- 1Departament de Bioquímica i Biologia Molecular, Estructura de Recerca Interdisciplinar en Biotecnologiaia i Biomedicina (ERI BIOTECMED), Universitat de València, Valencia, Spain
- 2Departamento de Biotecnología, Instituto de Agroquímica y Tecnología de Alimentos (IATA), Consejo Superior de Investigaciones Científicas (CSIC), Valencia, Spain
MicroRNAs contribute to the adaptation of plants to varying environmental conditions by affecting systemic mineral nutrient homeostasis. Copper and iron deficiencies antagonistically control the expression of Arabidopsis thaliana microRNA408 (miR408), which post-transcriptionally regulates laccase-like multicopper oxidase family members LAC3, LAC12, and LAC13. In this work, we used miR408 T-DNA insertion mutants (408-KO1 and 408-KO2) and a previously characterized transgenic line overexpressing miR408 (35S:408-14) to explore how miR408 influences copper- and iron-dependent metabolism. We observed that the altered expression of miR408 diminished plant performance and the activation of the iron-regulated genes under iron-deficient conditions. Consistently with the low expression of the miR408-target laccases, we showed that the vascular bundle lignification of the 35S:408-14 plants diminished. The decrease in the phenoloxidase and ferroxidase activities exhibited by wild-type plants under iron deficiency did not occur in the 408-KO1 plants, probably due to the higher expression of laccases. Finally, we observed that the hydrogen peroxide levels under iron starvation were altered in both the 408-KO1 and 35S:408-14 lines. Taken together, these results suggest that Arabidopsis plants with modified miR408 levels undergo multiple deregulations under iron-deficient conditions.
Introduction
Systemic signaling between roots and shoots is required to maintain mineral nutrient homeostasis in plants cultivated under varying environmental conditions. The nutrient itself, but also other molecules such as microRNAs, transmits and signals nutritional status information for whole plant adaptation (Sunkar et al., 2007; Kehr, 2013; Zhang et al., 2016; Chien et al., 2017). In Arabidopsis thaliana, a group of conserved small RNAs, denoted as Cu-miRNAs, are upregulated under copper (Cu) deficiency to target nonessential cuproproteins (Abdel-Ghany and Pilon, 2008). Cu-miRNAs miR397, miR398, and miR408 are highly conserved, which supports the importance of small RNA-mediated regulation in plant Cu homeostasis (Pilon, 2017). Apart from regulating local Cu deficiency responses, Cu-miRNAs also act as phloem-mediated systemic signals during Cu allocation and adaptation to metal deficiency (Buhtz et al., 2010; Kehr, 2013).
Experimental evidence indicates the interplay between Cu and iron (Fe) homeostases during different processes, including the antagonistic control of miRNA expression (Buhtz et al., 2010; Waters et al., 2012). miR398 regulates the mRNAs of genes CSD1 and CSD2, which encode cytosolic and chloroplastic Cu/Zn superoxide dismutases (Cu/ZnSODs), respectively (Yamasaki et al., 2007). Under Cu deficiency conditions, transcription factor SQUAMOSA-PROMOTER BINDING-LIKE PROTEIN 7 (SPL7) upregulates miR398 to replace Cu/ZnSOD with the Fe superoxide dismutase (FeSOD) counterpart, probably to economize Cu for essential cuproproteins such as plastocyanin (Yamasaki et al., 2007, 2009). Conversely under Fe deficiency conditions, the downregulation of miR398 contributes to FeSOD replacement with Cu/ZnSOD (Waters et al., 2012). Another antagonistically regulated miRNA by Cu and Fe deficiencies in phloem sap is miR408 (Buhtz et al., 2010), but its physiological significance remains uncovered. miR408 expression in Arabidopsis is abundant and spatially ubiquitous (Sunkar et al., 2006; Zhang and Li, 2013). miR408 is required for proper vegetative development and is involved in the adaptation to different abiotic stresses (Zhang and Li, 2013; Zhang et al., 2014; Ma et al., 2015). miR408 regulation under Cu deficiency is mediated by SPL7 through the binding to the GTAC motifs within the miR408 promoter (Yamasaki et al., 2009; Bernal et al., 2012; Zhang and Li, 2013). Besides, miR408 is also a target of HY5 (elongated Hypocotyl 5), a transcription factor that mediates responses to light (Zhang et al., 2014). miR408 overexpression partially compensates the effects in the spl7 and hy5 mutants under low Cu conditions by improving the plastocyanin function (Zhang and Li, 2013; Zhang et al., 2014). Based on its role in responses to light and Cu through the HY5-SPL7 gene network, miR408 has been proposed to act as an integrator of environmental signals in order to properly deliver Cu to plastocyanin for photosynthesis (Zhang et al., 2014). Thus a constitutive miR408 expression improves photosynthetic performance, increases the Cu content of chloroplasts, and improves biomass and seed yield in diverse plant species (Zhang et al., 2017; Pan et al., 2018; Song et al., 2018). Cupredoxin, plantacyanin, and uclacyanin mRNAs are miR408 targets (Abdel-Ghany and Pilon, 2008). These are blue cuproproteins that function as electron transfer shuttles between proteins (Nersissian et al., 1998; Choi and Davidson, 2011). miR408 targets the mRNAs of the LAC3, LAC12, and LAC13 genes encoding laccase-like multicopper oxidases (LMCOs) (LC, EC 1.10.3.2) (Abdel-Ghany and Pilon, 2008). LMCOs are extracellular glycoproteins that catalyze the oxidation of many substrates in vitro with simultaneous oxygen reduction (McCaig et al., 2005; Turlapati et al., 2011). Based on the wide range of substrates, it has been proposed that higher plant LMCOs could play more varied functions than initially expected (Reiss et al., 2013). Some LMCO functions could be involved in processes that affect Fe homeostasis, such as lignification, ferroxidase activity, and oxidative stress, which might account for their regulation under Fe deficiency through miR408.
Some LMCOs are involved in the oxidative polymerization of lignins from monolignols in secondary cell-wall formation (Berthet et al., 2011; Choi and Davidson, 2011; Zhao et al., 2013; Wang et al., 2014), and their activities are affected by miRNA expression (Lu et al., 2013). Cell-wall modifications through cross-linking have been suggested to affect metal chelation and mobilization (Le Gall et al., 2015; Curie and Mari, 2017). Among the potential interactions between Cu and Fe homeostases, we find metal competition for ligands during long-distance traffic under scarcity conditions (Alvarez-Fernández et al., 2014). In addition to laccases, the peroxidases that use hydrogen peroxide (H2O2) as a substrate also contribute to lignin biosynthesis in vivo, and H2O2 itself plays a role in cell-wall cross-linking and loosening (O’Brien et al., 2012; Kärkönen and Kuchitsu, 2015). H2O2 scavenging in the culture medium significantly decreases the amount of extracellular lignin formed in Norway spruce, and the inhibition of superoxide ( ) synthesis, or its dismutation to H2O2 by superoxide dismutases reduces lignin content (Kärkönen et al., 2002; Karlsson et al., 2005). Rice OsLAC3 has been shown to induce H2O2 accumulation, which affects the seed setting rate and mitochondria integrity in vascular tissues and root tips (Yu et al., 2017). Given the striking similarity between OsLAC3 and L-ascorbate oxidases, a role in oxidizing ascorbate, which drives to restrain H2O2 removal, has been proposed to explain the observed phenotypes. Reactive oxygen species (ROS) could also affect Cu-Fe interactions under metal deficiency conditions (Ravet and Pilon, 2013). The delicate balance between ROS, particularly H2O2, production and scavenging during metal stress is important for diverse signaling pathways, and there is evidence for a correlation between H2O2 and plant metal tolerance (Cuypers et al., 2016).
The role proposed for certain LMCOs as putative ferroxidases would be another miR480-controlled process that could participate in the interplay between Cu and Fe homeostases. LMCOs participate in Fe traffic in organisms other than plants (Hoopes and Dean, 2004). Some LMCO members could be involved in redox cycles, which are necessary for the mobilization and trafficking of Fe as they contain the residues expected for this purpose (Quintanar et al., 2007; Kosman, 2010a; Turlapati et al., 2011). Among other factors, the Fe redox state depends on the ratio between ferroxidase and ferrireductase activities (Kosman, 2010b, 2018). However, experimental evidence for LMCOs being involved in metal oxidation, by acting as ferroxidases, in plants is scarce (Müller et al., 2015). All plants, except grasses, acquire Fe after the reduction of Fe3+ chelates by a plasma membrane ferric chelate reductase which, in Arabidopsis, is encoded by FERRIC REDUCTASE 2 (FRO2) (Robinson et al., 1999). Upon reduction, Fe2+ is incorporated into the cell through a transporter encoded by IRON REGULATED TRANSPORTER 1 (IRT1) (Eide et al., 1996; Vert et al., 2002). IRT1 substrate availability depends on free external Fe (not bound to inorganic and organic complexes) and the Fe2+/Fe3+ratio, according to external redox status conditions and the enzymatic activity of ferrireductases. Helix-loop-helix type transcription factor FIT (bHLH29) is involved in Fe acquisition and remobilization (Colangelo and Guerinot, 2004). Other bHLH subgroup Ib factors (bHLH38, bHLH39, bHLH100, and bHLH101) could act in concert with FIT (Yuan et al., 2008; Wang et al., 2013). In the present work, we explore the effect of Fe deficiency on seedlings with altered miR408 levels by analyzing LMCO mRNA levels, enzymatic activities, and Fe deficiency responses.
Materials and Methods
Plant Growth Conditions and Treatments
Arabidopsis thaliana ecotype Columbia (Col-0) was used as the control wild type (WT). The three miR408 altered expression transgenic lines used herein, the miR408-overexpressing line 14 driven by the cauliflower mosaic virus 35S promoter (35S:408-14) and the two T-DNA insertion lines SALK_038860 (408-KO1) and SALK_121013.28.25.n (408-KO2), have been previously described (Zhang and Li, 2013; Ma et al., 2015). Seeds were surface-sterilized by sequential washes in 70% ethanol (5 min), bleach (5 min), and water (2 × 2 min) and were resuspended in 0.1% agar (w/v) and grown on plates containing 1/2 Murashige and Skoog (MS) medium supplemented with sucrose 1% (w/v). The 1/2 MS medium containing 1 μM CuSO4 and 50 μM Fe citrate was used for the metal sufficiency control conditions (50 μM Fe). Metal deficiency was obtained by home-made 1/2 MS with no added CuSO4 (0 μM Cu) or 5 μM Fe citrate for slight Fe deficiency (5 μM Fe) and 0 μM Fe citrate for severe Fe deficiency (0 μM Fe). For the assays of phenoloxidase and ferroxidase activities, 100 μM ferrozine, a Fe chelator, was included to provide severe deficiency growing conditions (-Fe). For severe Cu deficiency conditions, 100 μM of Cu chelator bathocuproine disulfonate (BCS) was added to the growth medium (-Cu). Intermediate photoperiodic conditions (12 h light, 20–23°C/12 h darkness, 16°C) were applied. Root length was measured by the Image J 1.42 q software.1
Chlorophyll and Hydrogen Peroxide Contents and Lignin Staining
The chlorophyll-a content of the Arabidopsis seedlings was determined by the trichlorometric method (Parsons and Strickland, 1963) and estimated by the equation of Lichtenthaler (1987). Hydrogen peroxide (H2O2) was detected by the brown polymerization product, formed by a reaction with diaminobenzidine tetrahydrochloride (DAB) (Jambunathan, 2010).
Lignin staining was done using 0.1% phloroglucinol saturated with HCl (Wiesner stain) (Liljegren, 2010). Between 5 and 7 seedlings of each genotype and condition were placed inside an Eppendorf tube with 700 μl of the reagent mixture to be incubated for 5 min. Afterward, seedlings were washed with sterile water and photographed.
Metal Content Determination
The fresh Arabidopsis material was washed once with 20 μM EDTA and three times with MilliQ H2O before being dried at 65°C for 2 days and digested with 65% (v/v) HNO3 and H2O2 30% (v/v) at 140°C. The digested samples were then diluted with Millipore H2O (Purelab Ultra). The Cu and Fe contents were determined by mass spectrometry with inductively coupled plasma (ICP-MS Agilent technologies) at the SCSIE (Universitat de València) using the manufacturer’s standard solutions for the calibration curves.
Gene Expression Analysis by Real-Time Quantitative PCR
The total RNA isolation, reverse transcription, and RT-qPCR analyses were performed as described in Carrió-Seguí et al. (2016). The forward (F) and reverse (R) sequences for the specific primers are shown in Table SI. To transform the fluorescent intensity measurements into relative mRNA levels, a two-fold dilution series of a mixture containing an equal amount of each cDNA sample was used, and standard curves were constructed for all the studied genes. The UBIQUITIN10 reference gene was used for data normalization. Each sample was analyzed in biological replicates, and the mean ratios ± SD were calculated.
Phenoloxidase and Ferroxidase Activities
Total proteins were extracted from the 7-day-old seedlings frozen in liquid nitrogen in the extraction buffer [400 mM NaCl, 2 mM MgCl2, 0.2% (p/v) sucrose, 20 mM Tris, PMSF 1 mM, pH 8.0, with HCl] at a ratio of 1:2 (p/v). Samples were centrifuged at 12,000 rpm for 10 min (4°C), and the supernatant was used as a crude extract. Total proteins were quantified (Bradford, 1976), and 500 μg were loaded with the nondenaturing loading buffer in the 12% SDS gels.
The phenoloxidase activity in gels was detected by using 3 mM p-phenylenediamine as the substrate (Lang et al., 2012). For the ferroxidase assay, the protocol of Hoopes and Dean (2004) was slightly modified as follows: gel was incubated for 1 h in 100 mM sodium acetate buffer, pH 5, with 5% (v/v) glycerol and 10 mM CuSO4, followed by a 1 h incubation period in 100 mM sodium acetate buffer, pH 5, with 0.4 mM FeSO4. After washing twice with distilled water and kept in the darkness at a relative humidity of 30% overnight, gel was revealed with 15 mM ferrozine. In the phenoloxidase assay, the bands around 65 kDa were quantified three times with the Image J 1.42 q software.1
Statistical Analyses
The statistical differences in gene expression analyses were identified by the pair-wise fixed reallocation randomization test (p < 0.05) (Pfaffl et al., 2002). One-way ANOVAs were performed for the other parameters. The significant differences between means were established after the Duncan test using the Infostat Statistics software, version 2018.2 Data are provided as the mean values ± SD of the different biological samples indicated in the figure legends. Asterisks indicate statistical differences (p < 0.05) in relation to the WT value.
Results
Phenotypic Characterization of Plants With an Altered miR408 Expression Under Iron Deficiency
The potential role of LMCOs in Fe homeostasis prompted us to conduct a physiological study of those plants with altered miR408 expression grown under Fe deficiency. To this end, the wild-type (WT) plants, a T-DNA mutant line (408-KO1), and a transgenic line overexpressing miR408 (35S:408-14) (Ma et al., 2015) were grown under Fe sufficiency (50 μM Fe) and under mild (5 μM Fe) and severe (0 μM Fe) Fe deficiency (Figures 1 and S1). The root length measurements indicated that only the 408-KO1 seedlings were slightly affected under the severe Fe deficiency conditions (Figure S1A). Regarding the chlorosis symptoms observed when Fe was scarce (Figure 1A; 0 μM Fe), the chlorophyll-a content of both the plants with altered miR408 levels was significantly lower than that of the WT under severe Fe deficiency (Figure 1B; 0 μM Fe). However under Fe sufficiency and mild Fe deficiency, the chlorophyll-a content of the three lines was roughly similar (Figure 1B). To ascertain whether the altered miR408 expression could influence metal content, Fe and Cu levels were measured in the aerial parts of the WT, 408-KO1, and 35S:408-14 lines (Figures 1C and S1B). As previously reported (Waters et al., 2012), the Cu levels in the WT seedlings were slightly higher under Fe deficiency compared to Fe sufficiency. Cu content did not change in the plants with altered miR408 expression under Fe deprivation compared to the control under these experimental conditions (Figure S1B). The Fe content in the 408-KO1 and 35S:408-14 lines remained essentially identical to the WT plants (Figure 1C). These results indicate that proper miR408 expression is necessary for Arabidopsis plants to adequately perform under iron-deficient conditions.
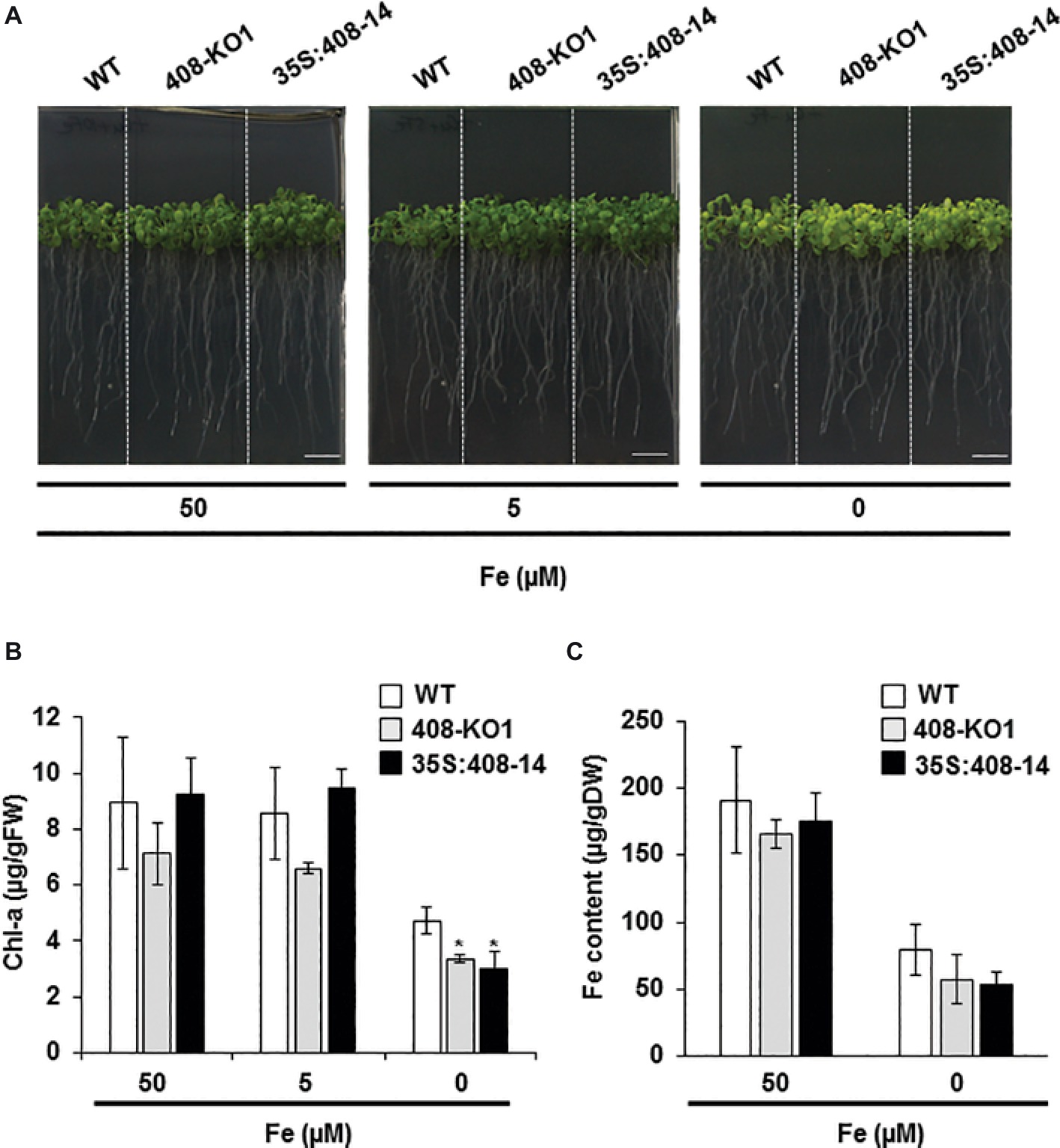
Figure 1. Phenotypic characterization under iron deficiency of the seedlings with altered miR408 expression. (A) Photographs of representative 15-day-old seedlings grown on 1/2 MS plates containing 50, 5, and 0 μM Fe citrate. (B) Chlorophyll-a content and (C) iron content in aerial tissues measured from the wild-type (WT), 408-KO1, and 35S:408-14 seedlings grown under the same conditions as indicated in panel A. Bars represent the means ± standard deviation of three biological replicates of n ≥ 15 plants for each genotype. Asterisks denote statistical differences (p < 0.05) compared to the WT value according to the Duncan test.
Lignification Under Iron Deficiency Is Affected in the Plants With Altered miR408 Expression
As certain LMCOs have been involved in the lignification process (Liang et al., 2006; Bonawitz and Chapple, 2010), we wondered whether there was a correlation between the degree of LMCO gene expression (LAC3, LAC12, and LAC13) and lignification. To this end, the WT, 408-KO1, and 35S:408-14 seedlings were grown under the control, slight, and severe Fe deficiency conditions (Figures 2 and S2). As previously reported (Rodríguez-Celma et al., 2016), we observed that the lignin staining of the WT vascular cylinder increased under the low Fe conditions (Figures 2 and S2). The lignification of the vascular bundles in the WT seedlings was more evident in the aerial part (Figure S2). The general reduction in lignin content observed in both the seedlings with altered miR408 expressions under the Fe deficiency conditions was noteworthy (Figures 2 and S2).
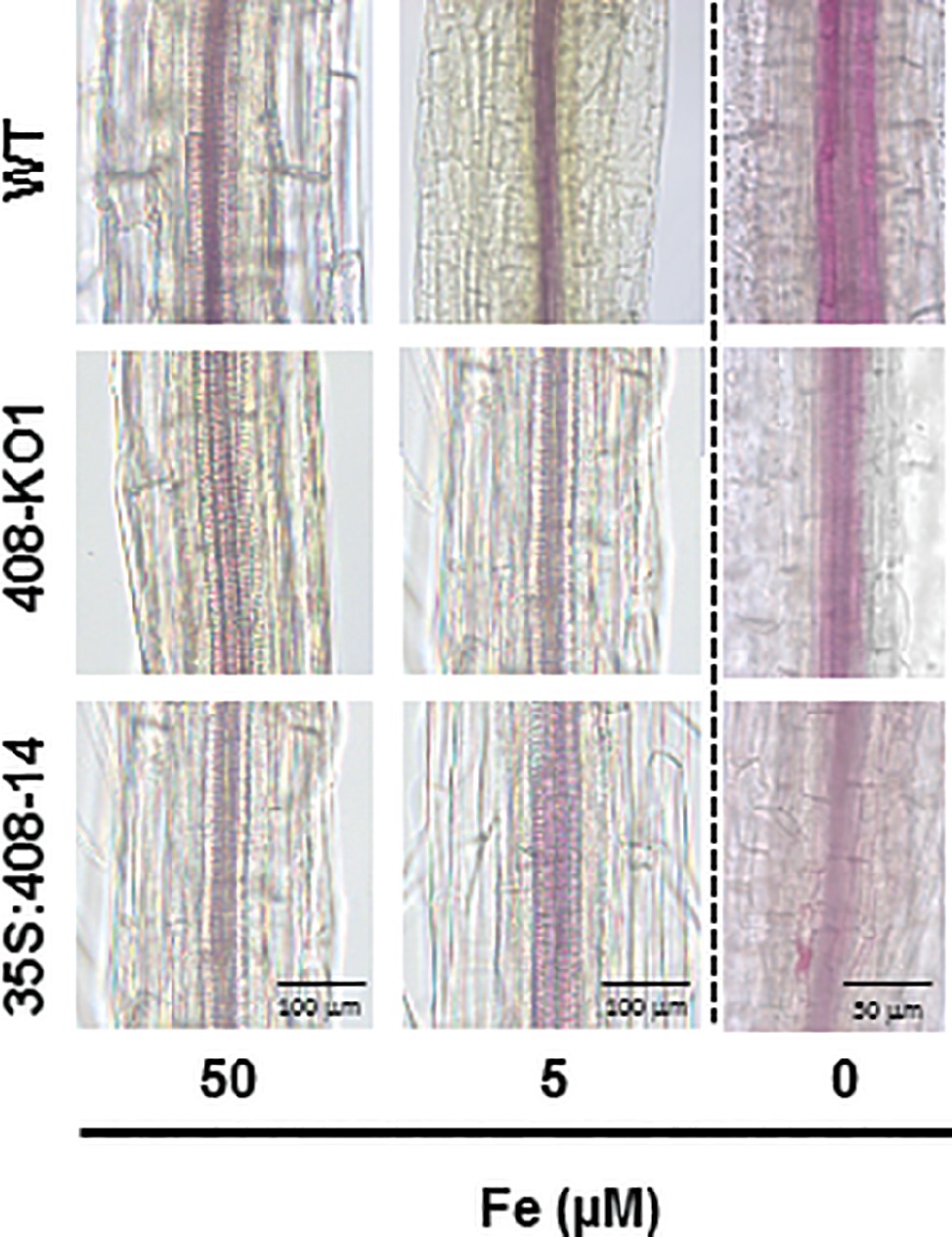
Figure 2. Lignin staining under iron deficiency conditions of the altered miR408 expression seedlings. Photographs of the representative lignin stained stems from the 20-day-old seedlings from the wild-type (WT), 408-KO1, and 35S:408-14 lines grown on 1/2 MS plates containing 50, 5, and 0 μM Fe citrate, pH 5.6. Stems (n ≥ 10 for each genotype) were stained with phloroglucinol saturated with HCl and photographed under a microscope. The length of the bars indicates 100 μm in 50 and 5 μM Fe citrate conditions and 50 μm in 0 μM Fe citrate.
To study the miR408 expression effects, we first corroborated that the miR408 levels were downregulated in the 408-KO1 and were overexpressed in the 35S:408-14 lines under our experimental conditions (Figure S3A). Previous studies had shown that miR408 is oppositely regulated by Fe and Cu deficiencies in phloem sap (Buhtz et al., 2010). In line with these data, miR408 expression increased in the WT seedlings under Cu deficiency, but decreased under the Fe deficiency conditions (Figure S3B). The expression of miR408 target transcript LAC3 was checked in the WT and miR408 mutant seedlings, with a second 408-KO line (408-KO2), under the control (1 μM Cu) and Cu-deficient conditions (0 μM Cu) (Figure S3C). As expected, the LAC3 mRNA levels lowered in response to Cu limitation in the WT seedlings. Moreover, its levels lowered in the miR408 overexpressing plants, but increased in the 408-KO mutants (Figure S3C), which indicates that the LAC3 target expression responded to both the miR408 levels and Cu deficiency in accordance with miR408 being upregulated. To address the target responses to Fe deficiency, the WT and mutant miR408 seedlings were grown under the control (50 μM Fe) and Fe deficiency (0 μM Fe) conditions, and the mRNA expression of miR408 targets LAC3, LAC12, LAC13, and plantacyanin (ARPN), encoding a cyanin (Yamasaki et al., 2007), was checked by RT-qPCR (Figure 3). In agreement with miR408 being downregulated, all the studied miR408 targets were upregulated by Fe deficiency in the WT plants (Figure 3). Moreover, the miR408 targets were upregulated in the 408-KO mutants and downregulated in the 35S:408-14 line under the metal sufficiency conditions, and the same results were also observed for Fe starvation (Figure 3). These results indicate that the miR408 mRNA targets under study are properly regulated by Fe limitation and respond accordingly to the altered miR408 expression under both the control and metal deficiency conditions (Figures 3 and S3C).
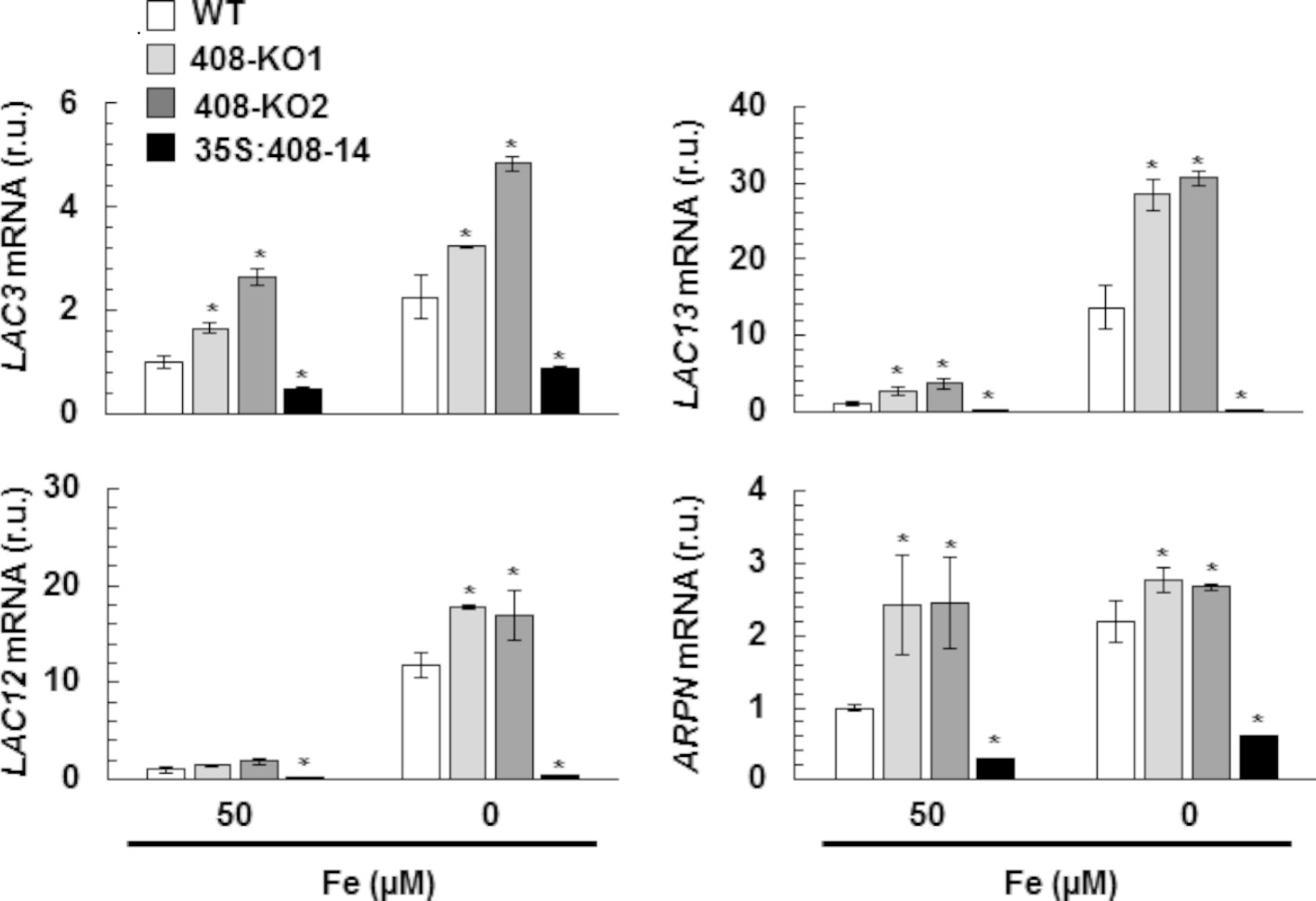
Figure 3. Gene expression of the miR408 targets under iron deficiency. LAC3, LAC12, LAC13, and ARPN relative expression. The 15-day-old wild-type (WT), 408-KO1, 408-KO2, and 35S:408-14 seedlings grown in 1/2 MS medium containing 50 and 0 μM Fe citrate. Total RNA was extracted and analyzed by RT-qPCR with specific oligonucleotides for LAC3, LAC12, LAC13, and ARPN. The UBIQUITIN10 gene was used for data normalization, and the expression is shown in relative units (r.u.). Values correspond to the arithmetic means (2−∆∆Ct) ± standard deviation of at least three biological replicates (n ≥ 25 plants). Asterisks denote significant differences for the same group of samples compared to the WT line (p < 0.05) based on the pair-wise fixed reallocation randomization test.
We analyzed whether the expression of the specific molecular markers involved in the lignin biosynthetic process (F6’H1´, β-GLU23, CCR1, and LAC17; Bonawitz and Chapple, 2010) were affected in the plants with the altered miR408 expression grown under Fe deficiency (Figure 4). Under the Fe-limited conditions, the expression of the studied lignification-related genes significantly reduced in the 408-KO lines compared to the WT plants (Figure 4). However in the 35S:408-14 line, the mRNA levels of the lignification-related genes increased under low Fe to reach the same levels observed in the WT, except for CCR1, which was not induced (Figure 4). As these genes are not direct miR408 targets, their downregulation in the 408-KO mutants is probably due to an indirect effect. With the 35S:408-14 line, the mRNA levels of the lignification-related genes increased when Fe was low, except for CCR1, which encodes a cinnamoyl-CoA reductase (Figure 4). These results show that miR408 influences the expression of the genes implicated in lignin biosynthesis.
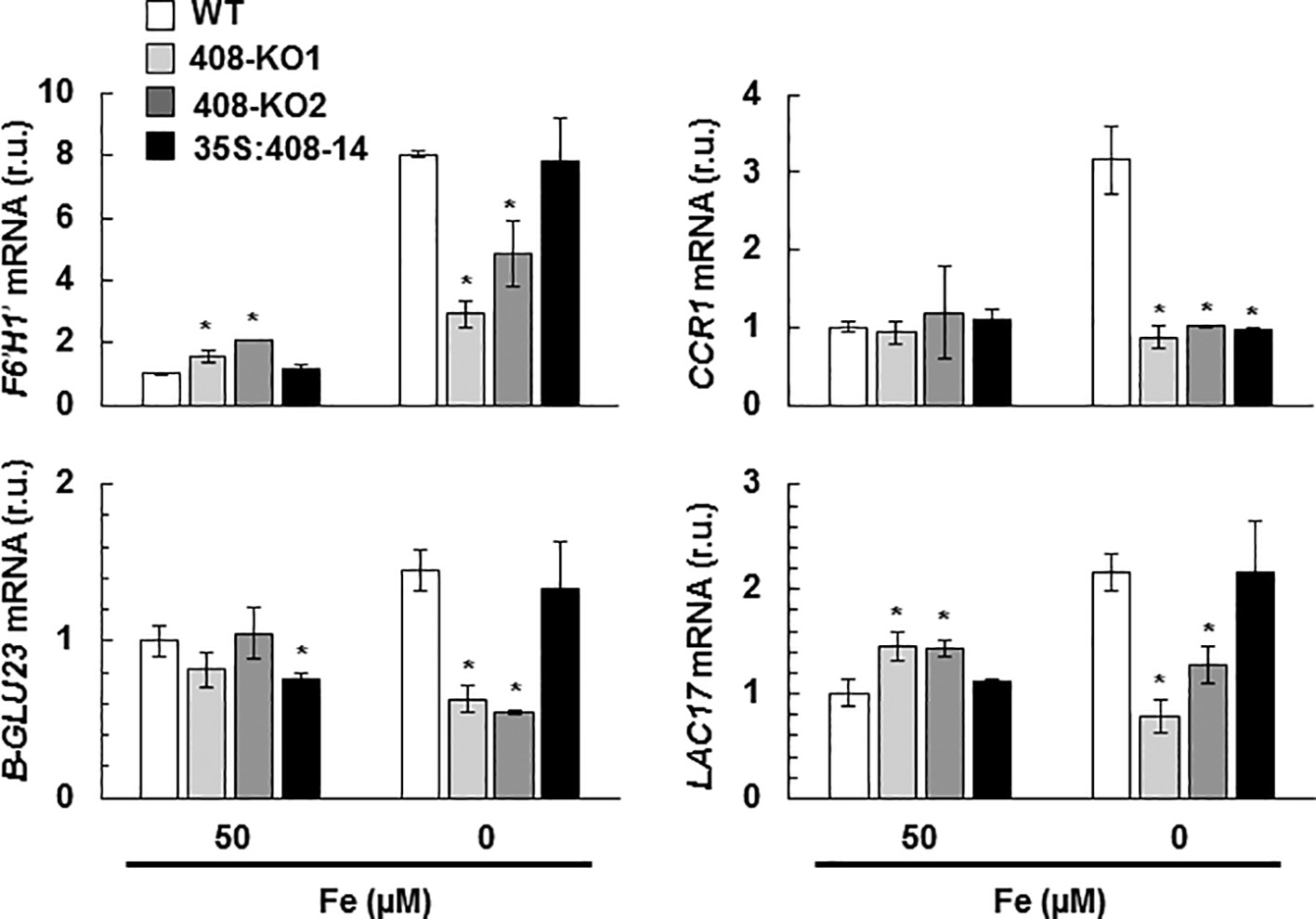
Figure 4. Gene expression of the lignification-related genes under iron deficiency in the seedlings with altered miR408 expression. F6′H1′, B-GLU23, LAC17, and CCR1 relative expression. The 15-day-old wild-type (WT), 408-KO1, 408-KO2, and 35S:408-14 seedlings grown in 1/2 MS medium containing 50 and 0 μM Fe citrate. Total RNA was extracted and analyzed by RT-qPCR with specific oligonucleotides for F6′H1′, B-GLU23, LAC17, and CCR1. The UBIQUITIN10 gene was used for data normalization, and the expression is shown in relative units (r.u.). Values correspond to the arithmetic means (2−∆∆Ct) ± standard deviation of at least three biological replicates (n ≥ 3). Asterisks depict significant differences for the same group of samples versus the WT line (p < 0.05) based on the pair-wise fixed reallocation randomization test.
Iron Deficiency Responses Are Affected in Altered miR408 Expression Plants
To determine how the plants with the altered miR408 expression perceived Fe status, the expression of a group of molecular Fe deficiency markers was analyzed under the Fe sufficiency and deficiency conditions. The genes involved in Fe remobilization and incorporation, such as FRO2, FRO3, and IRT1, and Fe-regulated Cu transporter COPT2 (Perea-García et al., 2013) were induced under Fe limitation in the WT plants (Figure 5). Unexpectedly, the expression of these Fe deficiency markers lowered in the plants with the altered miR408 expression grown under Fe scarcity (Figure 5). In order to check the regulation of the Fe deficiency responses, the expression of transcriptional activators bHLH39 and FIT (Colangelo and Guerinot, 2004; Yuan et al., 2008; Wang et al., 2013) was also analyzed (Figure 5). A differential response was observed in the 408-KO and 35S-408-14 lines. Whereas bHLH39 expression decreased by three times in the 408-KO lines, an 8-fold reduction in FIT expression took place in the 35S-408-14 line compared to the WT under Fe deprivation (Figure 5).
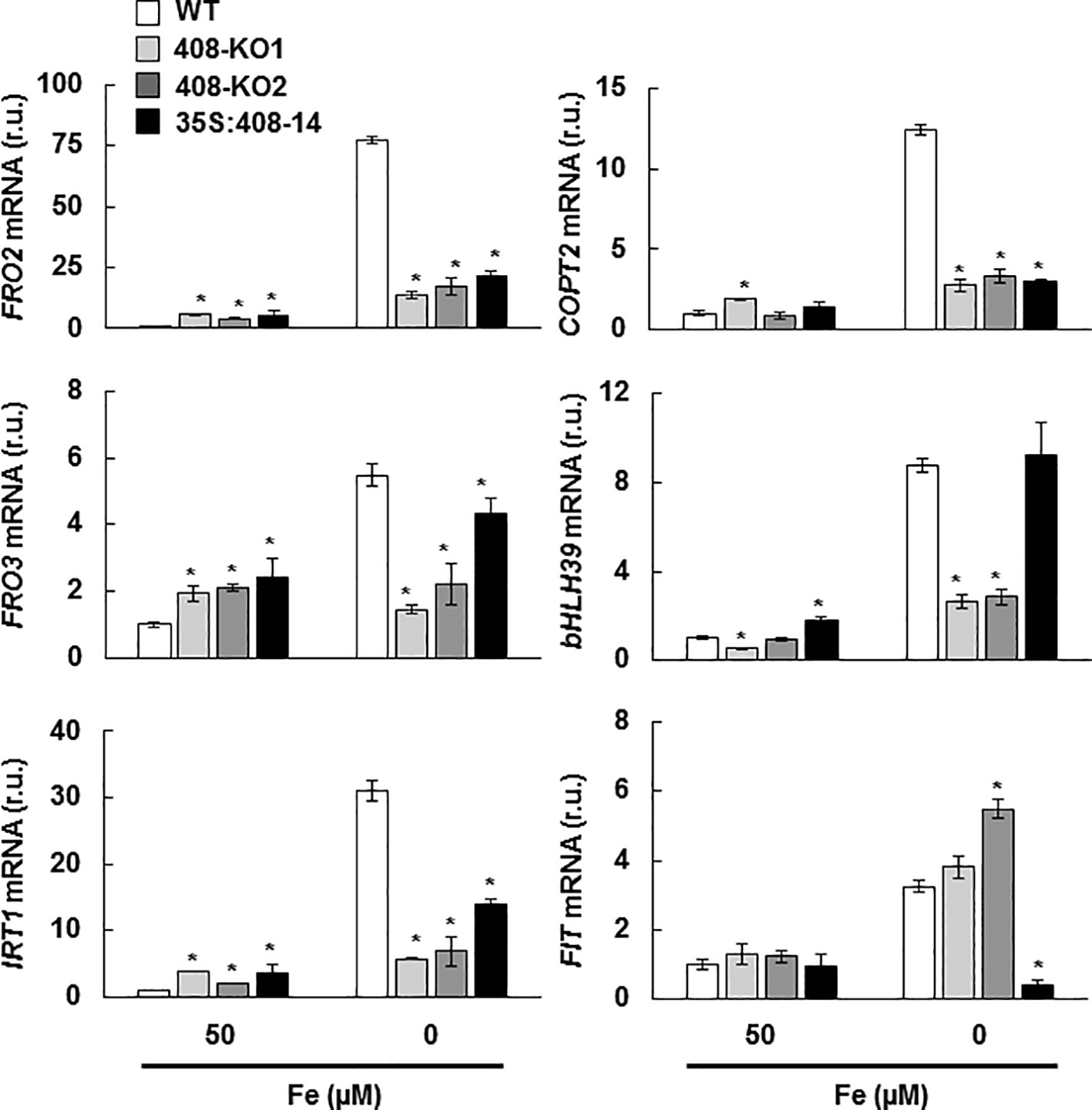
Figure 5. Expression of the metal homeostasis-related genes under iron deficiency in the seedlings with altered miR408 expression. FRO2, FRO3, IRT1, COPT2, bHLH39, and FIT1 relative expression. The 15-day-old wild-type (WT), 408-KO1, 408-KO2, and 35S:408-14 seedlings grown in 1/2 MS medium containing 50 and 0 μM Fe citrate. Total RNA was extracted and analyzed by RT-qPCR with specific oligonucleotides for FRO2, FRO3, IRT1, COPT2, bHLH39, and FIT1. The UBIQUITIN10 gene was used for data normalization and the expression is shown in relative units (r.u.). Values correspond to the arithmetic means (2−∆∆Ct) ± standard deviation of at least three biological replicates (n ≥ 25). Asterisks indicate significant differences for the same group of samples versus the WT line (p < 0.05) based on the pair-wise fixed reallocation randomization test.
Characterization of Phenoloxidase and Ferroxidase Activities in Plants With Altered miR408 Expression
The wide range of substrates that LMCOs can oxidize suggests that they could be involved in many other processes apart from lignification (Reiss et al., 2013). Therefore, we decided to determine phenoloxidase activity in gel in the WT and miR408 mutant seedlings grown under the control and Fe deficiency conditions (Lang et al., 2012; Figures 6A and S4). Remarkably, the phenoloxidase activity of the WT plants decreased under Fe deficiency (Figure 6A), despite us having shown that the miR408-dependent LMCO expression increased under these growth conditions (Figure 3). Phenoloxidase activity was slightly higher in mutants 408-KO than in the WT under Fe starvation (Figure 6A).
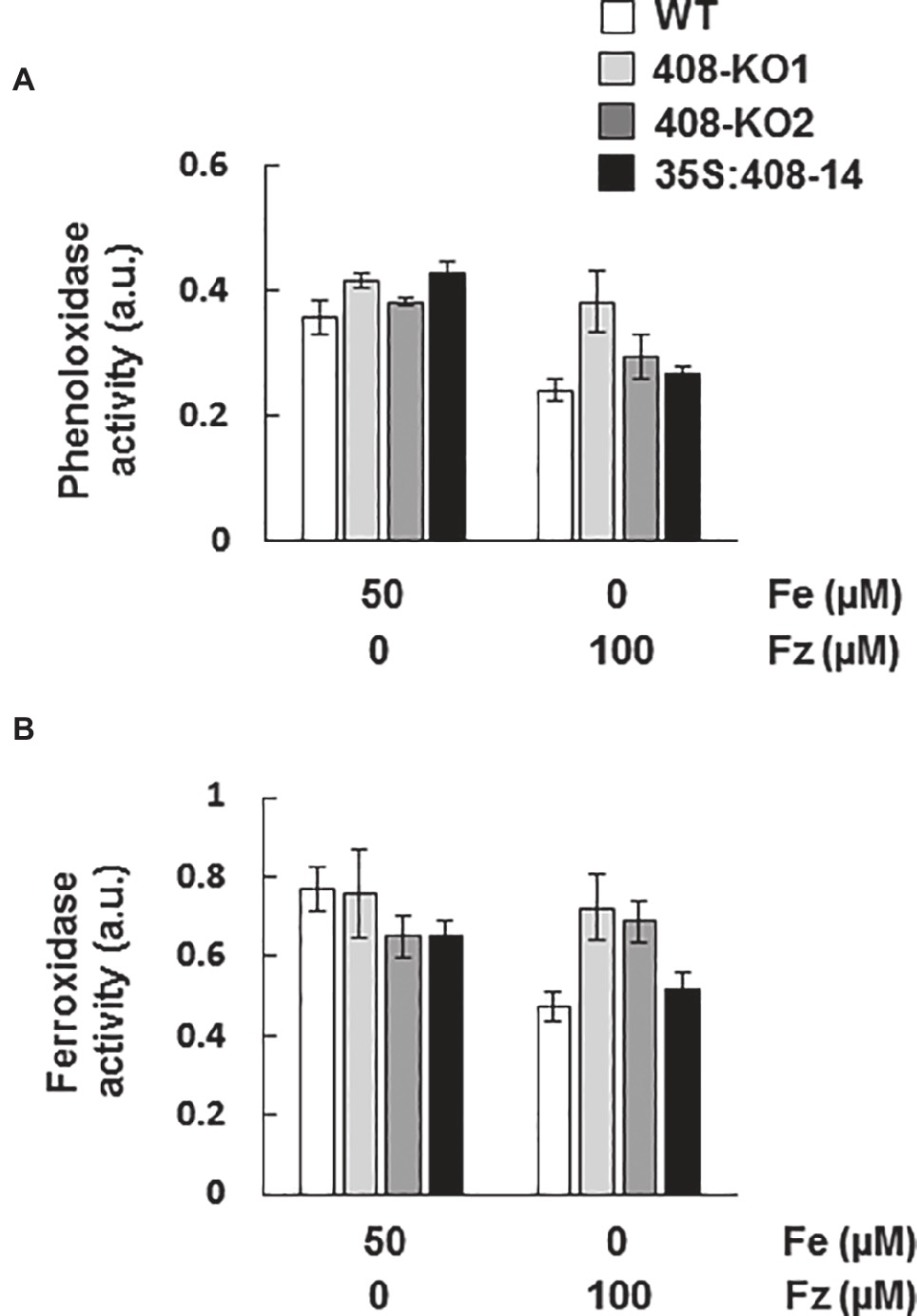
Figure 6. Phenoloxidase and ferroxidase activities in the seedlings with altered miR408 expression. (A) Phenoloxidase and (B) ferroxidase activities in the gels from the 7-day-old wild-type (WT), 408-KO1, 408-KO2, and 35S:408-14 seedlings grown on 1/2 MS plates containing 50 μM Fe citrate and 100 mM ferrozine. About 100 μg of total protein extraction were used for each sample. The relative intensities of the bands corresponding to the LMCO expected MW are shown as arbitrary units (a.u.). Values are the means ± SD of three technical replicates. A representative quantification of at least three independent experiments is shown.
All laccases exhibit the characteristic conserved Cu-binding domains of LMCOs. Apart from the different Cu-binding domains formed by histidine residues, Saccharomyces cerevisiae ferroxidase Fet3 (Askwith et al., 1994) possesses an essential aspartic residue (D409) within its ferroxidase domain (Quintanar et al., 2007). The alignment of the A. thaliana laccase sequences and yeast Fet3 showed that LAC3, LAC4, LAC5, LAC10, LAC11, LAC12, LAC13, and LAC17 were LMCOs which conserved the essential ferroxidase aspartic residue (Figure S5). Among the different Cu-miRNAs with LMCO mRNAs as targets (Abdel-Ghany and Pilon, 2008), miR408 is the only Cu-microRNA to target LMCO mRNAs, which encode proteins containing an aspartic residue potentially involved in Fe-binding. Thus, we hypothesized that miR408 expression could affect ferroxidase activity. To study this possibility, ferroxidase activity was checked in gels (Hoopes and Dean, 2004) in the same samples used to measure phenoloxidase activity (Figures 6B and S4). Similar to phenoloxidase, ferroxidase activity reduced under Fe deficiency in the WT plants (Figure 6B), despite the increase in the miR408-dependent LMCO mRNAs (Figure 3). It was noteworthy that ferroxidase activity did not decrease in mutants 408-KO (Figure 6B), probably due to the expression of laccases being higher than in the WT plants (Figure 3).
Hydrogen Peroxide Levels in Plants With Altered miR408 Expression
Previous data have shown that hydrogen peroxide (H2O2) increases in the vascular bundles of WT plants under Fe deficiency (Alvarez-Fernández et al., 2014). Thus, we decided to study H2O2 levels by diaminobenzidine tetrahydrochloride (DAB) staining in the 15-day-old seedling roots from the WT and miR408 mutants grown under the control and slight Fe deficiency conditions (Figure 7A). Under the control conditions, high H2O2 levels were observed in the vascular bundles of mutant 408-KO1, whereas the 35S:408-14 line displayed low levels versus the WT plants. Moreover, when Fe was scarce, the H2O2 levels increased in all the genotypes and were higher in mutant 408-KO1 and lower in the 35S:408-14 line compared to the WT line (Figure 7A). Therefore, we checked whether the expression of a set of oxidative stress genes involved in H2O2 metabolism was affected under Fe deprivation in the plants with altered miR408 expression (Figures 7B,C). We tested the expression of genes related either to H2O2 synthesis, such as SODs (CSD1, FSD1), or elimination, such as ascorbate oxidase MCO3 and catalase CAT2 (Cuypers et al., 2016). We observed that FSD1 expression significantly reduced in mutants miR408 compared to the WT plants under Fe sufficiency (Figure 7B). However under Fe deficiency, the most relevant result was the low expression of MCO3 and CAT2 compared to the WT in the plants with altered miR408 expression (Figure 7C). These data reveal that miR408 expression determines H2O2 levels and the expression of the genes related to oxidative stress.
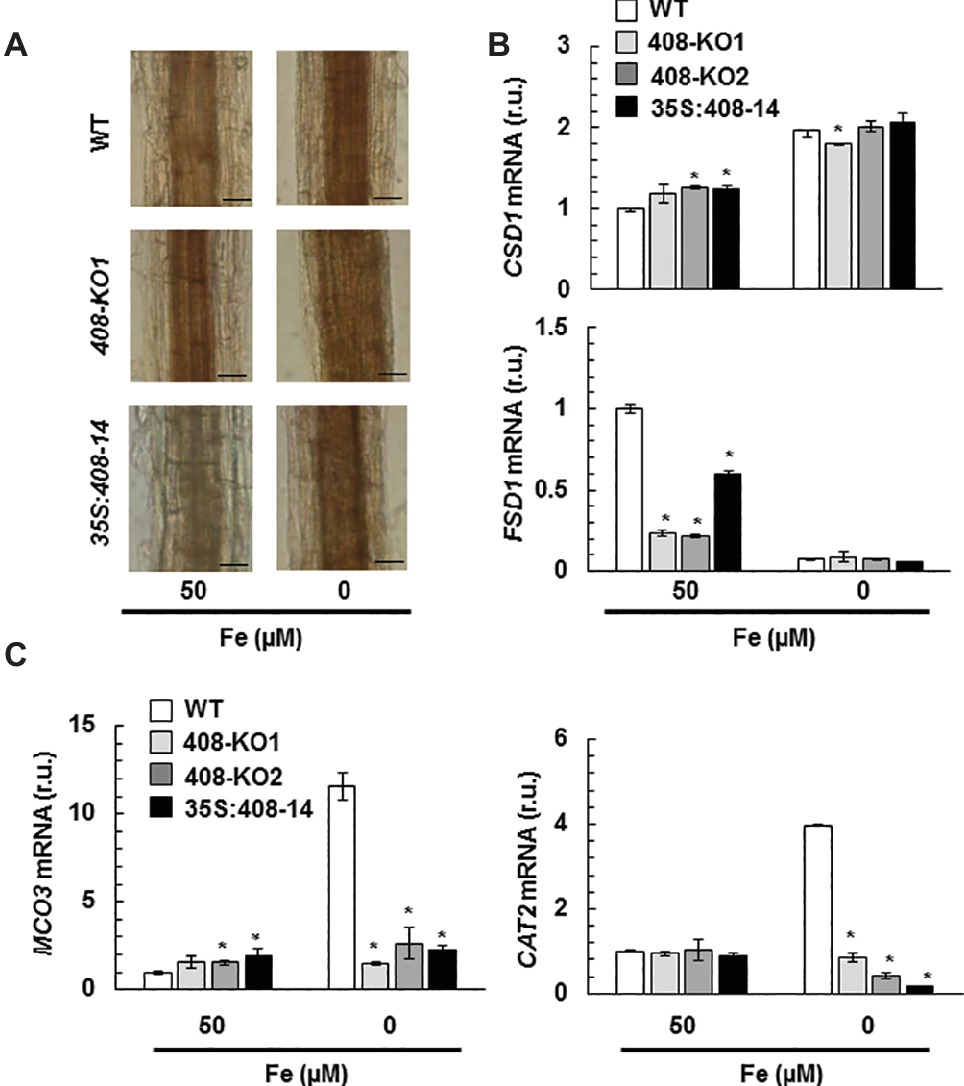
Figure 7. Hydrogen peroxide detection in the plants with altered miR408 expression. (A) Hydrogen peroxide detected in the roots of the 15-day-old wild-type (WT), 408-KO1, and 35S:408-14 seedlings grown in 50 and 5 μM Fe citrate media. Photographs of the representative roots, where a brown polymerization product was formed by the reaction of hydrogen peroxide with diaminobenzidine tetrahydrochloride. n = 15 plants were stained for each genotype and condition. The length of the bars indicates 100 μm in 50 and 5 μM Fe citrate. (B) FSD1 and CSD1 (C) CAT2 and MCO3 relative expression. The 7-day-old wild-type (WT), 408-KO1, 408-KO2, and 35S:408-14 seedlings grown in 1/2 MS medium containing 50 and 0 μM Fe citrate. Total RNA was extracted and analyzed by RT-qPCR with specific oligonucleotides for FSD1, CSD1, CAT2, and MCO3. The UBIQUITIN10 gene was used for data normalization, and the expression is shown in relative units (r.u.). Values correspond to the arithmetic means (2−∆∆Ct) ± standard deviation of at least three biological replicates (n ≥ 25 plants). Asterisks indicate significant differences for the same group of samples versus the WT line (p < 0.05) based on the pair-wise fixed reallocation randomization test.
Discussion
Previous work has shown the antagonistic effects of Cu and Fe deficiencies on Arabidopsis miR408 expression (Buhtz et al., 2010). In response to Cu starvation, SPL7 binds to Cu-responsive elements within the miR408 promoter and activates its expression (Yamasaki et al., 2009). As all miR408 targets are mRNAs that encode apoplastic cuproproteins, a role in Cu redistribution has been postulated for miR408 under Cu depletion (Abdel-Ghany and Pilon, 2008; Zhang et al., 2014). The mRNAs from the internal cuproproteins showed enhanced expression when miR408 was overexpressed and its targets putatively reduced (Ma et al., 2015). From this viewpoint, an apparently pernicious effect could take place under Fe deficiency because miR408 downregulation would increase the extracellular Cu quota versus the internal Cu, which could harm internal metalloprotein substitutions such as the FeSOD replacement for its Cu counterpart (Abdel-Ghany and Pilon, 2008). We ruled out a putative role of miR408 expression in metalloprotein substitution because no significant changes in SODs expression were observed under Fe deficiency in the plants with altered miR408 expression (Figure 7B).
The signaling pathway that drives to miR408 Fe deficiency repression and its physiological relevance remain unknown. miR408 is both induced and repressed when faced with different abiotic stresses (Ma et al., 2015). Indeed signaling during Cu starvation would display similarities to other stresses that induce miR408 expression, such as cold, salinity, and oxidative stress, whereas Fe deficiency signaling could converge with the stress signals that inhibit miR408 expression, such as drought and osmotic stress (Ma et al., 2015). Based on both the physiological and molecular effects that plants with altered miR408 expression showed under Fe limitation, the results offered herein could explain the antagonistic Fe and Cu regulation on miR408 expression (Figure 8). Both the 35S:408-14 overexpressing line and mutants 408-KO exhibited reduced chlorophyll-a content under low Fe conditions (Figure 1). According to previous results (Ma et al., 2015), 35S:408-14 plants are resistant to the type of stresses that induce miR408 expression and are sensitive to the stresses that inhibit its expression, among which we can now include Fe deficiency. However, whereas Fe sensitivity was observed in the 35S:408-14 seedlings, adult plants produced more seeds than the WT plants under Fe starvation (results not shown). Different possibilities were explored to understand these phenotypes. First, the role of the miR408 LMCO mRNA targets in the lignification process, which could affect metal translocation to the aerial part and shoot-to-root Fe deficiency signaling (Le Gall et al., 2015; Curie and Mari, 2017). Second, the potential function of LMCOs as ferroxidases and their influence on both Fe incorporation and mobilization. Finally, a putative role of LMCOs in oxidative stress and, more specifically, in H2O2 generation could affect metal transport.
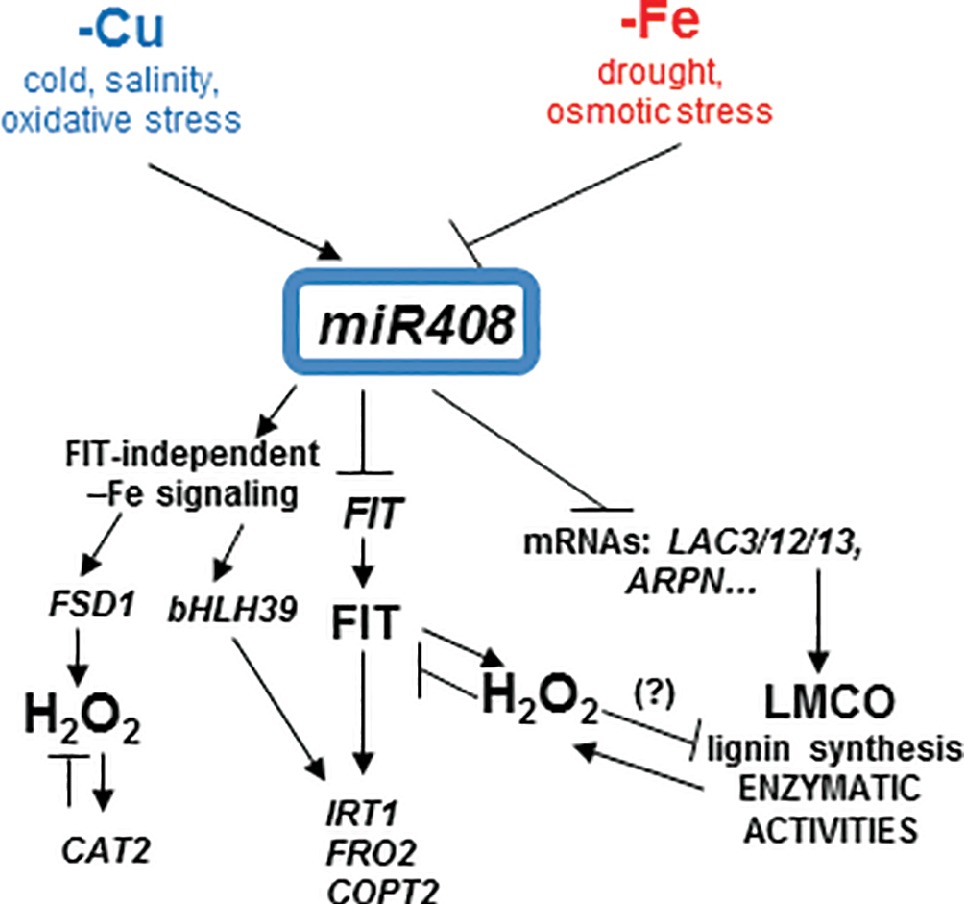
Figure 8. Model for the copper and iron homeostasis interplays through miR408 regulation. Schematic representation of the main miR408 effects on the Fe-deficiency responses observed in the present work. miR408 directly affects the expression of the target genes, such as the mRNA from LMCOs LAC3, LAC12, LAC13 (LAC3/12/13), and cyanins (i.e., ARPN) and their putative promiscuous LMCO role in lignin synthesis and enzymatic activities, such as phenoloxidase, ferroxidase, and ascorbate oxidase. In addition to other abiotic stresses, Cu and Fe deficiencies had the opposite effects on miR408 regulation and their subsequent enzymatic activities. The FIT-independent and FIT-dependent signaling pathways under Fe deficiency were induced or repressed by miR408, respectively. Fe deficiency induced oxidative stress and hydrogen peroxide (H2O2) could play a role in the observed miR408 effects. H2O2 could affect LMCO enzymatic activities, and at least part of the miR408 function could be able to counteract this effect under Fe deficiency.
Regarding the lignification process, some laccases, such as LAC4, LAC15, and LAC17, are directly involved in lignin synthesis in Arabidopsis (Liang et al., 2006; Berthet et al., 2011). Lignification is affected by miR857 and miR397 in both Arabidopsis and trees, such as Populus, where miRNAs act as negative regulators of laccase expression and are involved in the control of the secondary growth of vascular tissues (Lu et al., 2013; Wang et al., 2014; Zhao et al., 2015). Long-distance regulatory circuits have been identified for Fe deficiency signaling. Fe homeostasis is controlled at local and systemic levels by a wide range of signaling molecules, including ions, hormones, and metabolites (Giehl et al., 2009). Fe deficiency promotes increased lignification (Alvarez-Fernández et al., 2014). If laccases LAC3, LAC12, and LAC13 were involved in lignification, the repression of miR408 by Fe deprivation (Figure S3B), and the subsequent increase in LAC3, LAC12 and LAC13 expression (Figure 3), if redounded in increased protein activity, would lead to enhanced lignification under Fe deficiency, as was indeed observed (Figure 2). By applying the same reasoning, lignification should be higher in 408-KO mutants and lower in the 35S:408-14 line as LAC3, LAC12, and LAC13 expression was up- and downregulated, respectively (Figure 3). Instead, lignification under Fe deficiency was impaired in both 408-KO mutants and the 35S:408-14 line (Figure 2). However, we cannot rule out a direct role of the miR408 target LMCOs in the lignification process, and it is possible that their upregulated expression would cause reduced Cu availability for other LMCOs, including those involved in lignin biosynthesis. The inhibited expression of the key regulatory genes in the lignin pathway in both 408-KO mutants and the 35S:408-14 line (Figure 4) could account for the reduction observed in lignin in these lines.
Although both the 35S:408-14 line and the 408-KO mutants showed slightly increased responses in the expression of the Fe deficiency markers under Fe sufficiency, as well as a general defect under Fe deficiency, the molecular reasons behind these effects differed in both plant types. A reduced FIT expression was observed in the 35S:408-14 line under low Fe conditions, which suggests that low miR408 levels could be required for FIT expression and the subsequent Fe deficiency responses. Accordingly, FIT-independent Fe deficiency responses have been described for limited Cu, when miR408 expression is induced (Waters et al., 2012). On the contrary, 408-KO mutants displayed problems in bHLH39 expression, which could also account for their defective Fe deficiency responses. Different processes that are independent of FIT affect the expression of the Ib subgroup bHLH genes, including bHLH39. IVc subgroup members of the bHLH transcription factor family bind to the promoters of the Ib subgroup bHLH genes, and positively regulate Fe deficiency responses (Zhang et al., 2015). The dimethylation of histone H4R3 in the chromatin of the Ib subgroup bHLH genes is involved in Fe deficiency responses (Fan et al., 2014). Taken together, distinctive circumstances that depend on FIT or bHLH39 could be responsive of the defective Fe deficiency signaling in plants with high and low miR408 expression levels, respectively.
A significant decrease in both phenoloxidase and ferroxidase activities was observed under Fe deficiency in the WT plants (Figure 6), but its cause remains unsolved. These activities also reduced in the spl7 mutant, which exhibits exacerbated defects in root-to-shoot Fe translocation under low Cu conditions, but no responsive enzymes were identified (Bernal et al., 2012). In our study, Fe deficiency downregulated miR408 and led to the increase in miR408 target LMCO expression (Figure 3), which suggests a compensatory effect to counteract the negative effect of Fe scarcity on enzymatic activities. Thus, no decrease in phenoloxidase and ferroxidase activities was observed in mutants 408-KO at higher levels of miR408 target LMCOs. This result could indicate that both activities were affected by the miR408 expression levels, which would evidence a rather promiscuous range of activity for LMCOs, as previously suggested (Reiss et al., 2013).
LOW PHOSPHATE RESPONSE1 (LPR1), a cell wall-targeted ferroxidase, is involved in root growth inhibition when phosphate deficiency occurs by triggering Fe-stimulated apoplastic ROS generation and cell wall modifications, which impair cell-to-cell communication and meristem maintenance (Müller et al., 2015). Our results matched the concomitant increase in ferroxidase activity and ROS generation in mutant 408-KO under Fe scarcity conditions compared to the WT (Figures 6B, 7A). The results shown herein also agree with the proposed role of LMCOs functioning as ascorbate oxidases and, subsequently, with increasing H2O2 levels by competing with ascorbate peroxidases for reduced ascorbate (Yu et al., 2017). Hence according to the levels of the miR408 target LMCOs (Figures 3, 7A), H2O2 levels increased in mutant 408-KO and decreased in the 35S:408-14 line compared to the WT. Moreover, the fact that H2O2 levels increased in all cases under low Fe conditions (Figure 7A), which agrees with a higher LMCOs expression (Figure 3), could explain the previous studies in which Fe deficiency produced enhanced root H2O2 concentrations (Alvarez-Fernández et al., 2014). However, a role for other LMCOs, such as MCO3, that function as ascorbate oxidases (Yamamoto et al., 2005) cannot be ruled out because MCO3 expression was enhanced under Fe deficiency, but its expression considerably reduced in the plants with altered miR408 levels (Figure 7C).
Redox signaling has been shown to mediate microRNA expression (Sunkar et al., 2012; Jagadeeswaran et al., 2014). The results obtained with antioxidant activities in mutants 408-KO (Figures 7B,C) suggest that H2O2 accumulation under Fe deprivation could be due to the poor capacity to detoxify it (Jelali et al., 2014). H2O2 content was enhanced in an FIT-dependent manner under low Fe conditions, and the FIT protein was stabilized by H2O2 in the presence of zinc-finger transcription factor ZAT12, which demonstrates that H2O2 serves as a signal for Fe deficiency responses (Le et al., 2016). If the presence of FIT is a prerequisite for H2O2 accumulation in Fe-deficient roots, the difficulties shown by the 35S:408-14 overexpressing line to express FIT (Figure 5) would agree with the drop in H2O2 (Figure 7A). In addition, the fact that H2O2 participates in lignification would make lignin biosynthesis in these plants even more difficult (Figures 2 and S2).
One potential explanation to justify the reduced LMCO activities observed under Fe limitation comes from previous data, indicating that H2O2 inhibits the ferroxidase activity of the major human plasma multicopper oxidase ceruloplasmin (Shukla et al., 2006; Olivieri et al., 2011; Barbariga et al., 2015). Ceruloplasmin oxidation induces the structural changes that release Cu atoms from multicopper oxidase sites, which further increases oxidative stress (Shukla et al., 2006). Subsequently, reduced extracellular ferroxidase activity redounded in intracellular Fe retention (Olivieri et al., 2011). According to these data, it is tempting to speculate that increased oxidative stress under low Fe could also inactivate ferroxidase in Arabidopsis. If this were the case, plants would face a conflict under Fe deficiency as inhibited ferroxidase activity would make Fe mobilization difficult, while a subsequent Cu increase would further compete with scarce Fe for long-distance transport. In this scenario, the downregulation of miR408 and the subsequent increase in LMCOs expression could partially compensate for the negative effects of Fe depletion on ferroxidase activity. If miR408 mediates a process whose aim is to counteract the post-translational Fe deficiency effects on LMCO activities, the opposite miR408 regulation under Cu and Fe deficiencies could be justified.
In summary, these results suggest that Fe deficiency responses include an increase in oxidative stress, which comes in the form of H2O2, drives to enhanced lignification and affects shoot-to-root Fe signaling responses. In addition to their role in lignin biosynthesis, miR408 and probably other Cu-miRNAs that target LMCO mRNAs with putative promiscuous activities, such as ferroxidases and ascorbate oxidases, would interfere with Fe deficiency responses in a complex manner.
Data Availability
All datasets generated for this study are included in the manuscript and/or the Supplementary Files.
Author Contributions
LP and SP conceived the idea and wrote the manuscript. ÀC-S, LV-B, and OR-R performed the physiological and molecular experiments in the mutant plants. AP-G processed the data and helped to write the manuscript.
Funding
This work was supported by grant BIO2017-87828-C2-1-P from the Spanish Ministry of Economy, Industry and Competitiveness, and by FEDER funds from the European Union. ÀC-S and AP-G were recipients of predoctoral FPI and post-doctoral Juan de la Cierva Formación contracts, respectively, from the Spanish Ministry of Economy, Industry and Competitiveness.
Conflict of Interest Statement
The authors declare that the research was conducted in the absence of any commercial or financial relationships that could be construed as a potential conflict of interest.
Acknowledgments
We acknowledge the SCSIE (Universitat de València) for the ICP-MS and greenhouse services. The T-DNA mutants lines (408-KO1 and 408-KO2) and the transgenic line overexpressing miR408 (35S:408-14) were kindly provided by Ma et al. (2015).
Supplementary Material
The Supplementary Material for this article can be found online at: https://www.frontiersin.org/articles/10.3389/fpls.2019.00324/full#supplementary-material
Footnotes
References
Abdel-Ghany, S. E., and Pilon, M. (2008). MicroRNA-mediated systemic down-regulation of copper protein expression in response to low copper availability in Arabidopsis. J. Biolumin. Chemilumin. 283, 15932–15945. doi: 10.1074/jbc.M801406200
Alvarez-Fernández, A., Díaz-Benito, P., Abadía, A., López-Millán, A.-F., and Abadía, J. (2014). Metal species involved in long distance metal transport in plants. Front. Plant Sci. 5:105. doi: 10.3389/fpls.2014.00105
Askwith, C., Eide, D., Van Ho, A., Bernard, P. S., Li, L., Davis-Kaplan, S., et al. (1994). The FET3 gene of S. cerevisiae encodes a multicopper oxidase required for ferrous iron uptake. Cell 76, 403–410. doi: 10.1016/0092-8674(94)90346-8
Barbariga, M., Curnis, F., Andolfo, A., Zanardi, A., Lazzaro, M., Conti, A., et al. (2015). Ceruloplasmin functional changes in Parkinson’s disease-cerebrospinal fluid. Mol. Neurodegener. 10:59. doi: 10.1186/s13024-015-0055-2
Bernal, M., Casero, D., Singh, V., Wilson, G. T., Grande, A., Yang, H., et al. (2012). Transcriptome sequencing identifies SPL7-regulated copper acquisition genes FRO4/FRO5 and the copper dependence of iron homeostasis in Arabidopsis. Plant Cell 24, 738–761. doi: 10.1105/tpc.111.090431
Berthet, S., Demont-Caulet, N., Pollet, B., Bidzinski, P., Cézard, L., Le Bris, P., et al. (2011). Disruption of LACCASE4 and 17 results in tissue-specific alterations to lignification of Arabidopsis thaliana stems. Plant Cell 23, 1124–1137. doi: 10.1105/tpc.110.082792
Bonawitz, N. D., and Chapple, C. (2010). The genetics of lignin biosynthesis: connecting genotype to phenotype. Annu. Rev. Genet. 44, 337–363. doi: 10.1146/annurev-genet-102209-163508
Bradford, M. M. (1976). A rapid and sensitive method for the quantitation of microgram quantities of protein utilizing the principle of protein-dye binding. Anal. Biochem. 72, 248–254. doi: 10.1016/0003-2697(76)90527-3
Buhtz, A., Pieritz, J., Springer, F., and Kehr, J. (2010). Phloem small RNAs, nutrient stress responses, and systemic mobility. BMC Plant Biol. 10:64. doi: 10.1186/1471-2229-10-64
Carrió-Seguí, À., Romero, P., Sanz, A., and Peñarrubia, L. (2016). Interaction between ABA signaling and copper homeostasis in Arabidopsis thaliana. Plant Cell Physiol. 57, 1568–1582. doi: 10.1093/pcp/pcw087
Chien, P. S., Chiang, C. B., Wang, Z., and Chiou, T. J. (2017). MicroRNA-mediated signaling and regulation of nutrient transport and utilization. Curr. Opin. Plant Biol. 39, 73–79. doi: 10.1016/j.pbi.2017.06.007
Choi, M., and Davidson, V. L. (2011). Cupredoxins: a study of how proteins may evolve to use metals for bioenergetic processes. Metallomics 3, 140–151. doi: 10.1039/c0mt00061b
Colangelo, E. P., and Guerinot, M. L. (2004). The essential basic helix-loop-helix protein FIT1 is required for the iron deficiency response. Plant Cell 16, 3400–3412. doi: 10.1105/tpc.104.024315
Curie, C., and Mari, S. (2017). New routes for plant iron mining. New Phytol. 214, 521–525. doi: 10.1111/nph.14364
Cuypers, A., Hendrix, S., Amaral Dos Reis, R., De Smet, S., Deckers, J., Gielen, H., et al. (2016). Hydrogen peroxide, signaling in disguise during metal phytotoxicity. Front. Plant Sci. 7:470. doi: 10.3389/fpls.2016.00470
Eide, D., Broderius, M., Fett, J., and Guerinot, M. L. (1996). A novel iron-regulated metal transporter from plants identified by functional expression in yeast. Proc. Natl. Acad. Sci. U.S.A 93, 5624–5628.
Fan, H., Zhang, Z., Wang, N., Cui, Y., Sun, H., Liu, Y., et al. (2014). SKB1/PRMT5-mediated histone H4R3 dimethylation of Ib subgroup bHLH genes negatively regulates iron homeostasis in Arabidopsis thaliana. Plant J. 77, 209–221. doi: 10.1111/tpj.12380
Giehl, R. F., Meda, A. R., and von Wirén, N. (2009). Moving up, down, and everywhere: signaling of micronutrients in plants. Curr. Opin. Plant Biol. 12, 320–327. doi: 10.1016/j.pbi.2009.04.006
Hoopes, J. T., and Dean, J. F. (2004). Ferroxidase activity in a laccase-like multicopper oxidase from Liriodendron tulipifera. Plant Physiol. Biochem. 42, 27–33. doi: 10.1016/j.plaphy.2003.10.011
Jagadeeswaran, G., Li, Y. F., and Sunkar, R. (2014). Redox signaling mediates the expression of a sulfate-deprivation-inducible microRNA395 in Arabidopsis. Plant J. 77, 85–96. doi: 10.1111/tpj.12364
Jambunathan, N. (2010). Determination and detection of reactive oxygen species (ROS), lipid peroxidation, and electrolyte leakage in plants. Methods Mol. Biol. 639, 292–298. doi: 10.1007/978-1-60761-702-0_18
Jelali, N., Donnini, S., Dell’Orto, M., Abdelly, C., Gharsalli, M., and Zocchi, G. (2014). Root antioxidant responses of two Pisum sativum cultivars to direct and induced Fe deficiency. Plant Biol. 16, 607–614. doi: 10.1111/plb.12093
Kärkönen, A., Koutaniemi, S., Mustonen, M., Syrjänen, K., Brunow, G., Kilpeläinen, I., et al. (2002). Lignification related enzymes in Picea abies suspension cultures. Physiol. Plant. 114, 343–353. doi: 10.1034/j.1399-3054.2002.1140303.x
Kärkönen, A., and Kuchitsu, K. (2015). Reactive oxygen species in cell wall metabolism and development in plants. Phytochemistry 112, 22–32. doi: 10.1016/j.phytochem.2014.09.016
Karlsson, M., Melzer, M., Prokhorenko, I., Johansson, T., and Wingsle, G. (2005). Hydrogen peroxide and expression of hipI-superoxide dismutase are associated with the development of secondary cell walls in Zinnia elegans. J. Exp. Bot. 56, 2085–2093. doi: 10.1093/jxb/eri207
Kehr, J. (2013). Systemic regulation of mineral homeostasis by micro RNAs. Front. Plant Sci. 4:145. doi: 10.3389/fpls.2013.00145
Kosman, D. J. (2010a). Multicopper oxidases: a workshop on copper coordination chemistry, electron transfer, and metallophysiology. J. Biol. Inorg. Chem. 15, 15–28. doi: 10.1007/s00775-009-0590-9
Kosman, D. J. (2010b). Redox cycling in iron uptake, efflux, and trafficking. J. Biolumin. Chemilumin. 285, 26729–26735. doi: 10.1074/jbc.R110.113217
Kosman, D. J. (2018). The teleos of metallo-reduction and metallo-oxidation in eukaryotic iron and copper trafficking. Metallomics 10, 370–377. doi: 10.1039/c8mt00015h
Lang, M., Braun, C. L., Kanost, M. R., and Gorman, M. J. (2012). Multicopper oxidase-1 is a ferroxidase essential for iron homeostasis in Drosophila melanogaster. Proc. Natl. Acad. Sci. U.S.A 109, 13337–13342. doi: 10.1073/pnas.1208703109
Le, C. T., Brumbarova, T., Ivanov, R., Stoof, C., Weber, E., Mohrbacher, J., et al. (2016). Zinc finger of Arabidopsis thaliana12 (ZAT12) interacts with FER-like iron deficiency-induced transcription factor (FIT) linking iron deficiency and oxidative stress responses. Plant Physiol. 170, 540–557. doi: 10.1104/pp.15.01589
Le Gall, H., Philippe, F., Domon, J. M., Gillet, F., Pelloux, J., and Rayon, C. (2015). Cell wall metabolism in response to abiotic stress. Plants 4, 112–166. doi: 10.3390/plants4010112
Liang, M., Davis, E., Gardner, D., Cai, X., and Wu, Y. (2006). Involvement of AtLAC15 in lignin synthesis in seeds and in root elongation of Arabidopsis. Planta 224, 1185–1196. doi: 10.1007/s00425-006-0300-6
Lichtenthaler, H. K. (1987). Chlorophylls and carotenoids: pigments of photosynthetic biomembranes. Methods Enzymol. 148, 350–382.
Liljegren, S. (2010). Phloroglucinol stain for lignin. Cold Spring Harb. Protoc. 1:pdb.prot4954. doi: 10.1101/pdb.prot4954
Lu, S., Li, Q., Wei, H., Chang, M. J., Tunlaya-Anukit, S., Kim, H., et al. (2013). Ptr-miR397a is a negative regulator of laccase genes affecting lignin content in Populus trichocarpa. Proc. Natl. Acad. Sci. U.S.A 110, 10848–10853. doi: 10.1073/pnas.1308936110
Ma, C., Burd, S., and Lers, A. (2015). miR408 is involved in abiotic stress responses in Arabidopsis. Plant J. 84, 169–187. doi: 10.1111/tpj.12999
McCaig, B. C., Meagher, R. B., and Dean, J. F. (2005). Gene structure and molecular analysis of the laccase-like multicopper oxidase (LMCO) gene family in Arabidopsis thaliana. Planta 221, 619–636. doi: 10.1007/s00425-004-1472-6
Müller, J., Toev, T., Heisters, M., Teller, J., Moore, K. L., Hause, G., et al. (2015). Iron-dependent callose deposition adjusts root meristem maintenance to phosphate availability. Dev. Cell 33, 216–230. doi: 10.1016/j.devcel.2015.02.007
Nersissian, A. M., Immoos, C., Hill, M. G., Hart, P. J., Williams, G., Herrmann, R. G., et al. (1998). Uclacyanins, stellacyanins, and plantacyanins are distinct subfamilies of phytocyanins: plant-specific mononuclear blue copper proteins. Protein Sci. 7, 1915–1929. doi: 10.1002/pro.5560070907
O’Brien, J. A., Daudi, A., Butt, V. S., and Bolwell, G. P. (2012). Reactive oxygen species and their role in plant defence and cell wall metabolism. Planta 236, 765–779. doi: 10.1007/s00425-012-1696-9
Olivieri, S., Conti, A., Iannaccone, S., Cannistraci, C. V., Campanella, A., Barbariga, M., et al. (2011). Ceruloplasmin oxidation, a feature of Parkinson’s disease CSF, inhibits ferroxidase activity and promotes cellular iron retention. J. Neurosci. 31, 18568–18577. doi: 10.1523/JNEUROSCI.3768-11.2011
Pan, J., Huang, D., Guo, Z., Kuang, Z., Zhang, H., Xie, X., et al. (2018). Overexpression of microRNA408 enhances photosynthesis, growth, and seed yield in diverse plants. J. Integr. Plant Biol. 60, 323–340. doi: 10.1111/jipb.12634
Parsons, T., and Strickland, J. (1963). Discussion of spectrophotometric determination of marine-plant pigments, with revised equations for ascertaining chlorophyll-a and carotenoids. J. Mar. Res. 21, 105–156.
Perea-García, A., Garcia-Molina, A., Andrés-Colás, N., Vera-Sirera, F., Pérez-Amador, M. A., Puig, S., et al. (2013). Arabidopsis copper transport protein COPT2 participates in the cross talk between iron deficiency responses and low-phosphate signaling. Plant Physiol. 162, 180–194. doi: 10.1104/pp.112.212407
Pfaffl, M. W., Horgan, G. W., and Dempfle, L. (2002). Relative expression software tool (REST) for group-wise comparison and statistical analysis of relative expression results in real-time PCR. Nucleic Acids Res. 30:e36. doi: 10.1093/nar/30.9.e36
Quintanar, L., Stoj, C., Taylor, A. B., Hart, P. J., Kosman, D. J., and Solomon, E. I. (2007). Shall we dance? How a multicopper oxidase chooses its electron transfer partner. Acc. Chem. Res. 40, 445–452. doi: 10.1021/ar600051a
Ravet, K., and Pilon, M. (2013). Copper and iron homeostasis in plants: the challenges of oxidative stress. Antioxid. Redox Signaling 19, 919–932. doi: 10.1089/ars.2012.5084
Reiss, R., Ihssen, J., Richter, M., Eichhorn, E., Schilling, B., and Thöny-Meyer, L. (2013). Laccase versus laccase-like multi-copper oxidase: a comparative study of similar enzymes with diverse substrate spectra. PLoS One 8:e65633. doi: 10.1371/journal.pone.0065633
Robinson, N. J., Procter, C. M., Connolly, E. L., and Guerinot, M. L. (1999). A ferric-chelate reductase for iron uptake from soils. Nature 397, 694–697. doi: 10.1038/17800
Rodríguez-Celma, J., Lattanzio, G., Villarroya, D., Gutierrez-Carbonell, E., Ceballos-Laita, L., Rencoret, J., et al. (2016). Effects of Fe deficiency on the protein profiles and lignin composition of stem tissues from Medicago truncatula in absence or presence of calcium carbonate. J. Proteomics 140, 1–12. doi: 10.1016/j.jprot.2016.03.017
Shukla, N., Maher, J., Masters, J., Angelini, G. D., and Jeremy, J. Y. (2006). Does oxidative stress change ceruloplasmin from a protective to a vasculopathic factor? Atherosclerosis 187, 238–250. doi: 10.1016/j.atherosclerosis.2005.11.035
Song, Z., Zhang, L., Wang, Y., Li, H., Li, S., Zhao, H., et al. (2018). Constitutive expression of miR408 improves biomass and seed yield in Arabidopsis. Front. Plant Sci. 8:2114. doi: 10.3389/fpls.2017.02114
Sunkar, R., Chinnusamy, V., Zhu, J., and Zhu, J. K. (2007). Small RNAs as big players in plant abiotic stress responses and nutrient deprivation. Trends Plant Sci. 12, 301–309. doi: 10.1016/j.tplants.2007.05.001
Sunkar, R., Kapoor, A., and Zhu, J. K. (2006). Posttranscriptional induction of two Cu/Zn superoxide dismutase genes in Arabidopsis is mediated by downregulation of miR398 and important for oxidative stress tolerance. Plant Cell 18, 2051–2065. doi: 10.1105/tpc.106.041673
Sunkar, R., Li, Y. F., and Jagadeeswaran, G. (2012). Functions of microRNAs in plant stress responses. Trends Plant Sci. 17, 196–203. doi: 10.1016/j.tplants.2012.01.010
Turlapati, P. V., Kim, K. W., Davin, L. B., and Lewis, N. G. (2011). The laccase multigene family in Arabidopsis thaliana: towards addressing the mystery of their gene function(s). Planta 233, 439–470. doi: 10.1007/s00425-010-1298-3
Vert, G., Grotz, N., Dédaldéchamp, F., Gaymard, F., Guerinot, M. L., Briat, J. F., et al. (2002). IRT1, an Arabidopsis transporter essential for iron uptake from the soil and for plant growth. Plant Cell 14, 1223–1233. doi: 10.1105/tpc.001388
Wang, N., Cui, Y., Liu, Y., Fan, H., Du, J., Huang, Z., et al. (2013). Requirement and functional redundancy of Ib subgroup bHLH proteins for iron deficiency responses and uptake in Arabidopsis thaliana. Mol. Plant. 6, 503–513. doi: 10.1093/mp/sss089
Wang, C. Y., Zhang, S., Yu, Y., Luo, Y. C., Liu, Q., Ju, C., et al. (2014). MiR397b regulates both lignin content and seed number in Arabidopsis via modulating a laccase involved in lignin biosynthesis. Plant Biotechnol. J. 12, 1132–1142. doi: 10.1111/pbi.12222
Waters, B. M., McInturf, S. A., and Stein, R. J. (2012). Rossette iron deficiency transcript and microRNA profiling reveals links between copper and iron homeostasis in Arabidopsis thaliana. J. Exp. Bot. 63, 5903–5918. doi: 10.1093/jxb/ers239
Yamamoto, A., Bhuiyan, M. N., Waditee, R., Tanaka, Y., Esaka, M., Oba, K., et al. (2005). Suppressed expression of the apoplastic ascorbate oxidase gene increases salt tolerance in tobacco and Arabidopsis plants. J. Exp. Bot. 56, 1785–1796. doi: 10.1093/jxb/eri167
Yamasaki, H., Abdel-Ghany, S. E., Cohu, C. M., Kobayashi, Y., Shikanai, T., and Pilon, M. (2007). Regulation of copper homeostasis by micro-RNA in Arabidopsis. J. Biolumin. Chemilumin. 282, 16369–16378. doi: 10.1074/jbc.M700138200
Yamasaki, H., Hayashi, M., Fukazawa, M., Kobayashi, Y., and Shikanai, T. (2009). SQUAMOSA promoter binding protein-like7 is a central regulator for copper homeostasis in Arabidopsis. Plant Cell 21, 347–361. doi: 10.1105/tpc.108.060137
Yu, Y., Li, Q. F., Zhang, J. P., Zhang, F., Zhou, Y. F., Feng, Y. Z., et al. (2017). Laccase-13 regulates seed setting rate by affecting hydrogen peroxide dynamics and mitochondrial integrity in rice. Front. Plant Sci. 8:1324. doi: 10.3389/fpls.2017.01324
Yuan, Y., Wu, H., Wang, N., Li, J., Zhao, W., Du, J., et al. (2008). FIT interacts with AtbHLH38 and AtbHLH39 in regulating iron uptake gene expression for iron homeostasis in Arabidopsis. Cell Res. 18, 385–397. doi: 10.1038/cr.2008.26
Zhang, H., and Li, L. (2013). SQUAMOSA promoter binding protein-like7 regulated microRNA408 is required for vegetative development in Arabidopsis. Plant J. 74, 98–109. doi: 10.1111/tpj.12107
Zhang, J., Liu, B., Li, M., Feng, D., Jin, H., Wang, P., et al. (2015). The bHLH transcription factor bHLH104 interacts with IAA-LEUCINE RESISTANT3 and modulates iron homeostasis in Arabidopsis. Plant Cell 27, 787–805. doi: 10.1105/tpc.114.132704
Zhang, J. P., Yu, Y., Feng, Y. Z., Zhou, Y. F., Zhang, F., Yang, Y. W., et al. (2017). MiR408 regulates grain yield and photosynthesis via a phytocyanin protein. Plant Physiol. 175, 1175–1185. doi: 10.1104/pp.17.01169
Zhang, H., Zhao, X., Li, J., Cai, H., Deng, X. W., and Li, L. (2014). MicroRNA408 is critical for the HY5-SPL7 gene network that mediates the coordinated response to light and copper. Plant Cell 26, 4933–4953. doi: 10.1105/tpc.114.127340
Zhang, Z., Zheng, Y., Ham, B. K., Chen, J., Yoshida, A., Kochian, L. V., et al. (2016). Vascular-mediated signalling involved in early phosphate stress response in plants. Nat. Plants 2:16033. doi: 10.1038/nplants.2016.33
Zhao, Y., Lin, S., Qiu, Z., Cao, D., Wen, J., Deng, X., et al. (2015). MicroRNA857 is involved in the regulation of secondary growth of vascular tissues in Arabidopsis. Plant Physiol. 169, 2539–2552. doi: 10.1104/pp.15.01011
Keywords: Arabidopsis, hydrogen peroxide, iron deficiency, lignin, microRNA408
Citation: Carrió-Seguí À, Ruiz-Rivero O, Villamayor-Belinchón L, Puig S, Perea-García A and Peñarrubia L (2019) The Altered Expression of microRNA408 Influences the Arabidopsis Response to Iron Deficiency. Front. Plant Sci. 10:324. doi: 10.3389/fpls.2019.00324
Edited by:
Wolfgang Schmidt, Academia Sinica, TaiwanReviewed by:
Gang Liang, Xishuangbanna Tropical Botanical Garden (CAS), ChinaDiqiu Yu, Xishuangbanna Tropical Botanical Garden (CAS), China
Rongrong Guo, Guangxi Academy of Agricultural Science, China
Copyright © 2019 Carrió-Seguí, Ruiz-Rivero, Villamayor-Belinchón, Puig, Perea-García and Peñarrubia. This is an open-access article distributed under the terms of the Creative Commons Attribution License (CC BY). The use, distribution or reproduction in other forums is permitted, provided the original author(s) and the copyright owner(s) are credited and that the original publication in this journal is cited, in accordance with accepted academic practice. No use, distribution or reproduction is permitted which does not comply with these terms.
*Correspondence: Lola Peñarrubia, bG9sYS5wZW5hcnJ1YmlhQHV2LmVz;cGVuYXJydWJAdXYuZXM=
†Present address: Àngela Carrió-Seguí, Instituto de Biología Molecular y Celular de Plantas (CSIC-Universidad Politécnica de Valencia), Valencia, Spain
Omar Ruiz-Rivero, Instituto Valenciano de Investigaciones Agrarias (IVIA), Centro de Protección Vegetal y Biotecnología, Valencia, Spain
Laura Villamayor-Belinchón,Instituto de Medicina Genómica, Universitat de València, Valencia, Spain