- Department of Plant Agriculture, University of Guelph, Guelph, ON, Canada
Roles of the major polyamines (mPA), putrescine, spermidine, and spermine (Spm), in various developmental and physiological processes in plants have been well documented. Recently, there has been increasing focus on the link between mPA metabolism and defense response during plant-stress interactions. Empirical evidence is available for a unique role of Spm, distinct from the other mPA, in eliciting an effective defense response to (a)biotic stresses. Our understanding of the precise molecular mechanism(s) by which Spm modulates these defense mechanisms is limited. Further analysis of recent studies indicates that plant Spm functions differently during biotic and abiotic interactions in the regulation of oxidative homeostasis and phytohormone signaling. Here, we summarize and integrate current knowledge about Spm-mediated modulation of plant defense responses to (a)biotic stresses, highlighting the importance of Spm as a potent plant defense activator with broad-spectrum protective effects. A model is proposed to explain how Spm refines defense mechanisms to tailor an optimal resistance response.
Introduction
Polyamines are ubiquitous, small aliphatic polycations found in eukaryotic organisms. The major polyamines (mPA) in plants are the diamine putrescine (Put), the triamine spermidine (Spd) and the tetraamine spermine (Spm). They function in key developmental and physiological events such as embryogenesis, cell division, floral initiation, senescence and responses to stress (Evans and Malmberg, 1989; Galston and Sawhney, 1990). The biosynthesis and degradation of mPA are highly responsive to environmental stimuli (Liu et al., 2007). Several studies have reported that the three mPA mold plant responses to (a)biotic stresses (Bouchereau et al., 1999; Walters, 2000, 2003a,b; Urano et al., 2003; Alcázar et al., 2010; Minocha et al., 2014; Romero et al., 2018). However, there is evidence for the differential regulation of Spm/Spd and Put by stresses (see Shelp et al., 2018), and for a unique role of Spm, distinct from the other mPA, in the induction and formation of resistance responses to various types of (a)biotic stresses. For instance, Mitsuya et al. (2009) reported that Spm is the only mPA that effectively suppresses the multiplication of cucumber mosaic virus in Arabidopsis. Other research indicates that Spm strongly induces different defense-related genes in Arabidopsis seedlings, whereas similar doses of Put and Spd do not, and elevated levels of endogenous Spm are causally linked to higher tolerance to the bacterial pathogen Pseudomonas syringae and the oomycete Hyaloperonospora arabidopsidis (Marco et al., 2014). Similarly, among the mPA, only Spm strongly induces the two key defense-associated signaling molecules, nitric oxide and hydrogen peroxide (H2O2), in Nicotiana benthamiana, ultimately leading to resistance to the bacterial pathogen Xanthomonas campestris (Kim et al., 2013). An Arabidopsis mutant deficient in Spm biosynthesis exhibits hypersensitivity to salt and drought stresses, and the phenotype is mitigated by exogenous Spm, but not Put or Spd (Yamaguchi et al., 2006; Kusano et al., 2007). Together, these findings suggest that Spm is a stress-associated signaling molecule (Yamakawa et al., 1998) due to its unique role in inducing several components of the plant defense response, including: (i) genes coding for pathogenesis related (PR) and resistance (R) proteins (Yamakawa et al., 1998; Gonzalez et al., 2011); (ii) mitogen-activated protein kinases (MAPK) (Takahashi et al., 2003; Gonzalez et al., 2011); (iii) several defense-associated transcription factors (Mitsuya et al., 2009; Gonzalez et al., 2011); (iv) phytoalexin biosynthesis (Marco et al., 2014; Mo et al., 2015); and, (v) the hypersensitive response (HR) (Takahashi et al., 2004; Sagor et al., 2009). In this review, we summarize and integrate current knowledge on Spm-mediated refinement of plant defense responses to both biotic and abiotic stresses, and highlight the importance of Spm as a potent plant defense activator with broad-spectrum effects. In addition, a model is proposed to explain how Spm regulates various oxidative and hormone signaling pathways, which tailor an optimal defense response to various external stresses.
Spm Metabolism in Plants
Spm anabolism in plants involves two main routes (Shelp et al., 2012). The first is catalyzed by ornithine decarboxylase, which converts ornithine into Put, the main precursor for Spm biosynthesis. The second is a three-step pathway in which arginine is converted to agmatine by arginine decarboxylase, and then agmatine is converted to Put by agmatine imidohydrolase and carbamoylputrescine amidohydrolase. Put is then successively converted to Spd by Spd synthase, and then to Spm by Spm synthase. The latter reactions require the addition of aminopropyl groups, supplied from decarboxylated S-adenosylmethionine (SAM), which is a product of SAM decarboxylase (SAMDC). Spm catabolism involves flavin-containing PA oxidases (PAO), which catalyze two types of reactions, terminal oxidation and back-conversion. The terminal oxidation of Spm generates 4-N-(3-aminopropyl)-4-aminobutanal, 1,3-diaminopropane and H2O2. Alternatively, the back-conversion reaction converts Spm to Spd, and Spd to Put, resulting in the production of 3-aminopropanal and H2O2.
Spm Metabolism and Biotic Stresses
Spm Induces Oxidative Response
The HR reaction is defined as a type of rapid programmed cell death, which is induced by the generation of reactive oxygen species (ROS, such as H2O2) at the site of pathogen entry, leading to activation of several defense mechanisms that result in cessation of growth of the pathogen, typically biotrophic, and in protection of remaining plant tissue (Govrin and Levine, 2000; Jones and Dangl, 2006). It is generally believed that the HR reaction is effective against biotrophic pathogens only, but effectiveness of HR against necrotrophic pathogens such as Botrytis cinerea has also been reported (Asselbergh et al., 2007; Azami-Sardooei et al., 2010, 2013; Seifi et al., 2013). HR induction involves two major pathways: the host HR is mediated through specific recognition of certain microbes by the surveillance system of the host, namely R proteins (Keen, 1990); and, the non-host HR is non-specific, typically induced in response to a broad spectrum of pathogens in many plants (Heath, 2000). Interestingly, Yoda et al. (2003, 2009) demonstrated that PAO-mediated Spm oxidation strongly contributes to the onset of both host and non-host HRs triggered in tobacco plants by different pathogens, highlighting the importance of Spm catabolism in the regulation of the HR-dependent defense response.
Exogenous Spm induces the expression of several H2O2-dependent signaling components and transcription factors in Arabidopsis leaves, and results in HR-mediated resistance to cucumber mosaic virus (Mitsuya et al., 2009). The addition of a PAO inhibitor represses the activation of defense genes and alleviates ROS generation and HR, confirming that PAO is involved in the resistance response. Infiltration of tobacco leaf disks with Spm strongly decreases the growth of the biotrophic bacterial pathogen Pseudomonas viridiflava, but not the necrotrophic fungal pathogen, Sclerotinia sclerotiorum, and co-infiltration of Spm and a PAO inhibitor reverses this protective effect (Marina et al., 2008). Exogenous application of thermospermine, a structural isomer of Spm, induces resistance to P. viridiflava in Arabidopsis through PAO-mediated thermospermine oxidation (Marina et al., 2013). Apoplastic Spm accumulates in tobacco plants in response to infection by the (hemi)biotrophic bacterial pathogen P. syringae pv. tabaci, and PAO overexpression upregulates defense-related marker genes and cell wall-based defense responses, resulting in disease tolerance (Moschou et al., 2009). Similarly, overexpression of a cotton-derived PAO in Arabidopsis results in elevated levels of ROS and resistance to the necrotrophic vascular wilt fungus Verticillium dahlia (Mo et al., 2015). The resistance response is mainly mediated by the induction of MAPK and cytochrome P450, culminating in the accumulation of the Arabidopsis-specific phytoalexin camalexin (Mo et al., 2015). Exogenous Spm increases the disease resistance of Arabidopsis against P. viridiflava, which is compromised by the PAO inhibitor SL-11061 (Gonzalez et al., 2011). Together, these findings suggest that PAO is a key defense regulator, particularly in response to apoplastically-localized plant pathogens.
Mitochondrion Membrane Dysfunction
Spm induces apoptosis, a type of programmed cell death, in animal cells through the activation of a group of cell-death-inducing pathways, known as the caspase cascade, which entails the loss of mitochondrial membrane potential and leakage of electron-transfer-chain intermediates, such as cytochrome c, into the cytosol (Moffatt et al., 2000; Stefanelli et al., 2000). Similarly, plant mitochondria are known to play an important role in ROS generation and induction of HR during plant-pathogen interactions (Lam et al., 2001; Hatsugai et al., 2004; Van Breusegem and Dat, 2006). Notably, exogenous Spm induces mitochondrial membrane dysfunction (Takahashi et al., 2003) and the expression of two important defense-associated MAPK, which in turn induce a subset of HR-related genes such as HSR203J (Takahashi et al., 2004). Pre-treatment with bongkrekic acid, an inhibitor of the mitochondrial permeability transition pore, suppresses the induction of HR-related genes, confirming that mitochondrial dysfunction is involved in Spm-induced HR in tobacco leaves (Takahashi et al., 2004).
Hormonal Regulation
Several HR marker genes, such as HSR203J, are responsive to Spm, suggesting that it is involved in HR induction (Takahashi et al., 2004). These HR markers are also induced in NahG plants, which are highly deficient in the plant hormone salicylic acid (SA), suggesting that Spm-induced HR reaction is independent of the SA signaling pathway (Takahashi et al., 2004). This result is consistent with SA-independent, Spm-induced expression of PR proteins in tobacco (Yamakawa et al., 1998). However, several reports propose a link between JA-associated defense responses and Spm metabolism. For instance, exogenous Spm promotes JA biosynthesis in lima bean (Ozawa et al., 2009), and Spm synthase-overexpressing plants of Arabidopsis have elevated levels of endogenous Spm (two to threefold), resistance to the bacterial pathogen P. viridiflava, and expression of components of the JA-dependent defense signaling pathway such as ERF and Myb transcription factors (Gonzalez et al., 2011). Similarly, elevated levels of endogenous Spm in SAMDC-overexpression lines of Arabidopsis are associated with resistance to Hyaloperonospora arabidopidis and P. syringae and the induction of several defense-associated genes, such as PR and R proteins, as well as genes involved in JA biosynthesis, such as chloroplastic lipoxygenase and allene oxide synthase (Marco et al., 2014). Collectively, these findings suggest that JA signaling positively regulates Spm-mediated defense response to biotic stresses.
Spm Metabolism and Abiotic Stresses
Spm Activates Antioxidant Response
Elevated levels of endogenous Spm, as well as the exogenous application of Spm, induce tolerance to various abiotic stresses (Capell et al., 2004; Yamaguchi et al., 2006; Kusano et al., 2007). Fruits of the drought-tolerant tomato cultivar Zarina have elevated levels of endogenous Spm and activities of the antioxidant enzymes superoxide dismutase (SOD) and catalase (CAT), culminating in better tolerance to dehydration-induced oxidative stress (Sánchez-Rodríguez et al., 2016). Similarly, Spm application is associated with higher activities of SOD and CAT in pea plants, mitigating high-temperature-induced chlorophyll degradation (Todorova et al., 2016). Also, Spm induces the tolerance of mung bean seedlings to high temperature, drought or cadmium toxicity, and this is typically associated with elevated activities of SOD, CAT, glutathione S-transferase (GST) and glutathione reductase (GR), and levels of non-enzymatic antioxidants such as ascorbic acid and glutathione (GSH), culminating in reduced ROS accumulation (Nahar et al., 2016a,b). The application of Spm to wheat leaves alleviates oxidative damage caused by cadmium and copper excess, reduces the metal-induced ROS accumulation, and restores GR activity (Groppa et al., 2007). Likewise, Spm application to soybean leaves reduces osmotic-stress-induced losses in chlorophyll, carotenoid and protein levels, and increases the activities of CAT and SOD (Radhakrishnan and Lee, 2013). Stress tolerance, elevated activities of CAT, SOD and peroxidases, and elevated expression of heat shock proteins are found in Spm-treated seedlings of trifoliate orange exposed to combined drought and heat stresses (Fu et al., 2014). Together, this body of evidence suggests that Spm induces tolerance to oxidative stress caused by abiotic stresses through the activation of both non-enzymatic and enzymatic antioxidant pathways.
Hormonal Regulation
It has previously been shown that exogenous abscisic acid (ABA) upregulates expression of the mPA biosynthesis genes SAMDC and arginine decarboxylase (Urano et al., 2003), and the induction of these genes is significantly compromised in ABA-deficient mutants of Arabidopsis grown under drought stress (Alcázar et al., 2006a), suggesting a positive correlation between mPA biosynthesis and ABA-mediated response to cold, salt and drought stresses (Alcázar et al., 2010). Such a premise is supported by the existence of several abiotic stress-responsive elements (motifs), as well as, ABA-responsive elements in the promoters of mPA biosynthesis genes (Alcázar et al., 2006b). Notably, Spm treatment induces the expression of ABA-responsive element binding factors in trifoliate orange seedlings challenged by drought and heat stresses (Fu et al., 2014). Hence, crosstalk between Spm-mediated defense response to abiotic stresses and ABA-dependent signaling pathway is suggested.
Contrasting Roles of Spm During Oxidative/Antioxidant Responses
Many of the key reports on Spm-induced resistance discussed above are summarized in Table 1. Examination of the biochemical, transcriptional and molecular responses to (a)biotic stresses leads us to hypothesize dual roles for Spm in modulating the oxidative status of the plant cell. Spm seems to accumulate in response to both biotic and abiotic stresses, but this is followed by two different scenarios: (i) upon perception of biotic challenges, Spm “enhances” the oxidative response through the induction of ROS generation and HR: and (ii) upon perception of abiotic challenges, Spm “alleviates” oxidative damage through the stimulation of ROS-scavenging enzymes, leading to an antioxidant response. Figure 1 depicts the different players involved in the two scenarios. How a plant adopts such contrasting mechanisms in order to tailor an appropriate defense response merits further consideration.
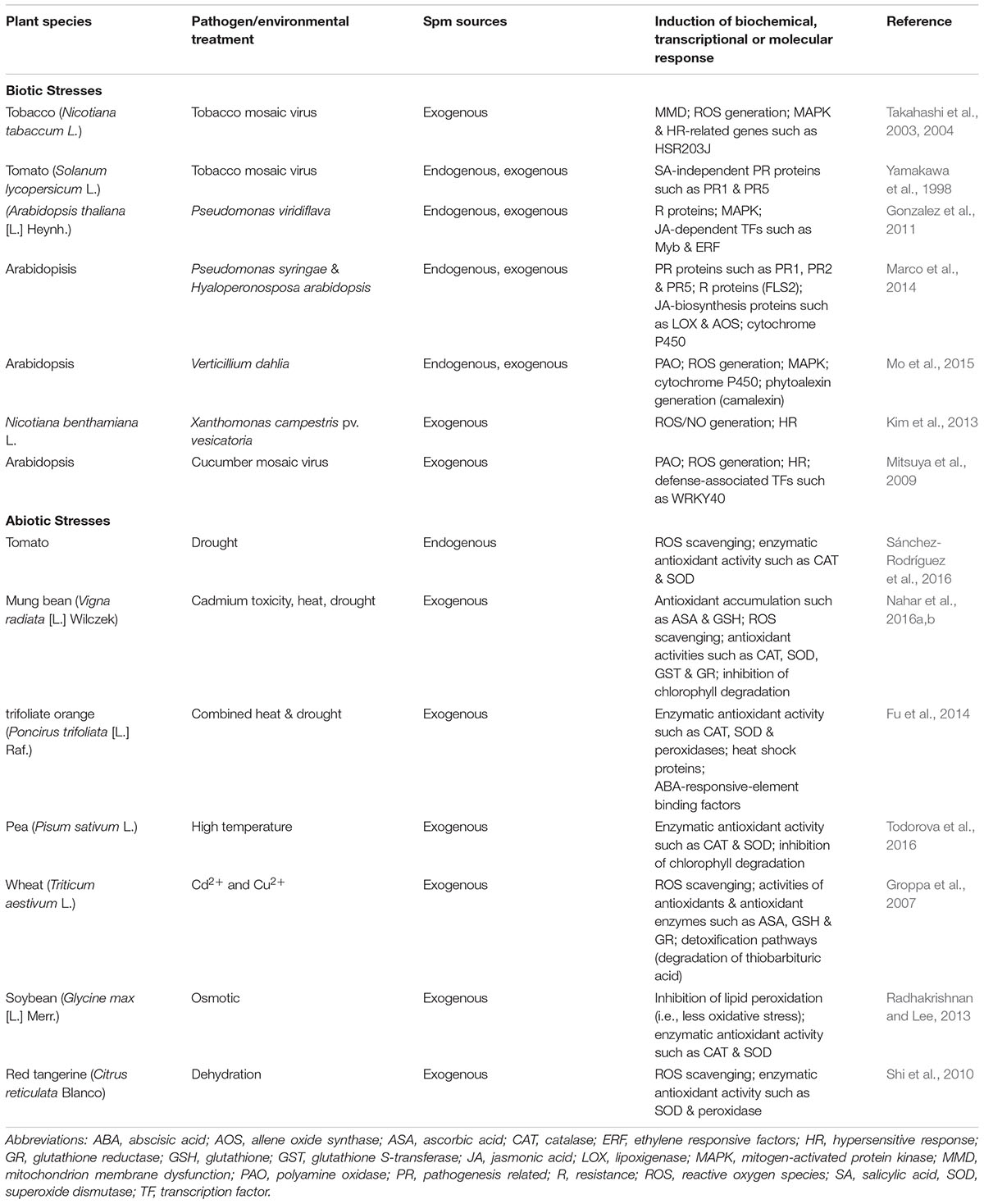
Table 1. Defense mechanisms associated with Spm-induced resistance against biotic and abiotic stresses.
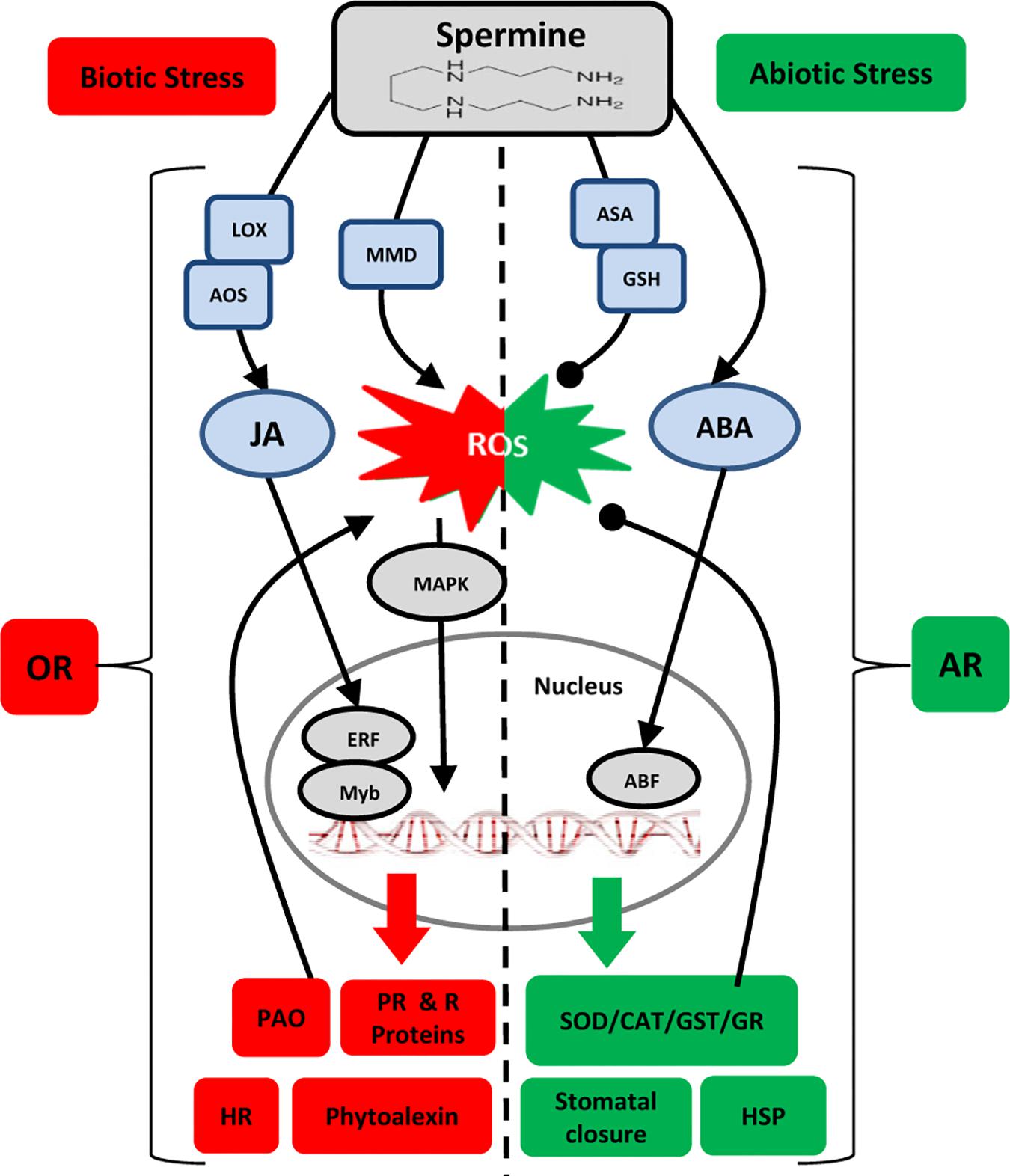
Figure 1. Model for interaction of Spm with plant responses to (a)biotic stresses. Lines ending in arrowheads and closed circles, respectively, indicate positive and negative impacts. Biotic Stress: OR, oxidative response; HR, hypersensitive response; MMD, mitochondrion membrane dysfunction; PAO, polyamine oxidase; JA, jasmonic acid; LOX, lipoxygenase; AOS, allene oxide synthase; MAPK, mitogen activated protein kinase; ERF, ethylene responsive factor; PR, pathogenesis related; R: resistance. Abiotic Stress: = AR, antioxidant response; ASA, ascorbic acid; GSH, glutathione; ABA, abscisic acid; ABF, abscisic acid-binding factor; SOD, superoxide dismutase; GR, glutathione reductase; CAT, catalase; GST, glutathione S-transferase; HSP, heat shock protein.
The oxidative response occurs immediately after successful recognition of the pathogen by the plant’s surveillance system, following a biphasic pattern (Wojtaszek, 1997). Phase-I consists of a rapid, transient, and low-amplitude burst of ROS generation, occurs within minutes after pathogen recognition, and is known to function as an upstream trigger of several defense-related signaling cascades. Phase-II occurs after few to several hours post recognition, consists of a sustained wave of ROS generation/accumulation of much higher amplitude, and plays a key role in inducing defense-associated genes and HR (Van Camp et al., 1998; De Gara et al., 2003; Torres et al., 2006). While the oxidative response to avirulent pathogens, successfully recognized by the plant’s immune system, generally exhibits a biphasic pattern of ROS accumulation, only phase-I is elicited in response to virulent pathogens that are able to avoid host recognition (Torres et al., 2006). With this in mind, it seems that PAO-mediated ROS generation (i.e., Spm oxidation) during incompatible plant-pathogen interactions exhibits the characteristics of a phase-II oxidative response, as previously proposed (Takahashi et al., 2004). Therefore, it can be posited that Spm oxidation under such conditions is not merely a metabolic feedback mechanism to maintain PA homeostasis, but beyond that, it functions as an important part of the plant immune system to provide the ROS necessary to fuel successful activation of defense genes and formation of HR.
The role of mPA as protective molecular chaperones (Jiménez-Bremont et al., 2014) might explain how Spm induces an antioxidative state in the plant tissue in response to abiotic stresses. The spatial separation of positive charges in PA at physiological pH could enable PA to bind negatively-charged molecules such as nucleic acids, phospholipids and proteins, thereby protecting the structure and function of these macromolecules from degradation and modification (Ruiz-Herrera et al., 1995; Martin-Tanguy, 2001; D’Agostino et al., 2005). This property would also enable the scavenging of free radicals and stabilization of intracellular membranes under stress conditions (Popovic et al., 1979; Groppa and Benavides, 2008; Alcázar et al., 2010; Radhakrishnan and Lee, 2013). This might also explain why mPA are abundant in green, young and actively growing tissues, whereas their titers dramatically decline in senescing organs (Galston and Sawhney, 1990; Del Duca et al., 2000). Considering that Spm contains four nitrogen groups, it could provide greater buffering capacity than Spd and Put (Shi et al., 2010). This is in agreement with previous studies that report exogenous Spm, unlike Spd and Put, has a potent anti-senescence effect on oat and lettuce leaves, as well as Jerusalem artichoketuber (Galston and Sawhney, 1990; Dondini et al., 2003; Serafini-Fracassini et al., 2010). Notably, elevated levels of Spm in an Arabidopsis mutant that lacks the PA back-conversion pathway, are associated with delayed dark-induced senescence, suggesting that Spm is a metabolic defense mechanism against senescence-induced oxidative stress and cell death (Sequera-Mutiozabal et al., 2016).
Concluding Remarks
Many natural and synthetic compounds are known to activate defense responses against a certain type of stress only, either biotic or abiotic. Those that confer protection against a wide range of both biotic and abiotic stresses are very rare, with silicon being an important exception (Van Bockhaven et al., 2013). In light of the empirical evidence reviewed above, it seems that Spm can be considered as another exceptional molecule with broad spectrum prophylactic effects against both types of stresses. Such effects are exerted through different passive (attributed to the physical and biochemical properties of Spm) and active (attributed to molecular functions of Spm) mechanisms. Given that Spm refines the defense response according to the biotic or abiotic nature of the stress by (i) promoting appropriate hormone-mediated signaling pathways, (ii) modulating oxidative/antioxidant responses, and (iii) inducing several defense-related genes (Figure 1), the notion that Spm functions as a plant defense activator becomes more plausible. Nevertheless, several important questions remain regarding these mechanisms. What are the nodes of convergence between Spm-induced signaling pathway and ABA/JA-mediated defense response during (a)biotic challenges? Which specific transcription factors or other transcription-regulating mechanisms control the Spm-induced defense gene activation? What are the regulatory mechanisms that control Spm-mediated oxidative homeostasis during biotic and abiotic stress responses? Considering the immense value of environmentally-friendly methods for plant stress management in sustainable crop production systems, the application of a multidisciplinary approach benefiting from molecular, biotechnological, and breeding strategies seems to be necessary to fully unlock the potential of Spm as a natural plant defense activator with broad-spectrum protective effects.
Author Contributions
HS conceived and wrote the manuscript. BS supervised the writing and edited the manuscript. Both authors read and approved the final manuscript.
Funding
This research was supported by funding from MITACS Canada, the Natural Sciences and Engineering Research Council (NSERC) Idea-to-Innovation Program, and NutriAg Ltd.
Conflict of Interest Statement
The authors declare that the research was conducted in the absence of any commercial or financial relationships that could be construed as a potential conflict of interest.
References
Alcázar, R., Cuevas, J. C., Patron, M., Altabella, T., and Tiburcio, A. F. (2006a). Abscisic acid modulates polyamine metabolism under water stress in Arabidopsis thaliana. Physiol. Plant 128, 448–455. doi: 10.1111/j.1399-3054.2006.00780.x
Alcázar, R., Marco, F., Cuevas, J. C., Patron, M., Ferrando, A., Carrasco, P., et al. (2006b). Involvement of polyamines in plant response to abiotic stress. Biotechnol. Lett. 28, 1867–1876. doi: 10.1007/s10529-006-9179-3
Alcázar, R., Altabella, T., Marco, F., Bortolotti, C., Reymond, M., Koncz, C., et al. (2010). Polyamines: molecules with regulatory functions in plant abiotic stress tolerance. Planta 231, 1237–1249. doi: 10.1007/s00425-010-1130-0
Asselbergh, B., Curvers, K., Franca, S. C., Audenaert, K., Vuylsteke, M., Van Breusegem, F., et al. (2007). Resistance to Botrytis cinerea in sitiens, an abscisis acid-deficient tomato mutant, involves timely production of hydrogen peroxide and cell wall modifications in the epidermis. Plant Physiol. 144, 1863–1877. doi: 10.1104/pp.107.099226
Azami-Sardooei, Z., Franca, S. C., De Vleesschauwer, D., and Höfte, M. (2010). Riboflavin induces resistance against Botrytis cinerea in bean, but not in tomato, by priming for a hydrogen peroxide-fuelled resistance response. Physiol. Mol. Plant Pathol. 75, 23–29. doi: 10.1016/j.pmpp.2010.08.001
Azami-Sardooei, Z., Seifi, H. S., De Vleesschauwer, D., and Höfte, M. (2013). Benzothiadiazole (BTH)-induced resistance against Botrytis cinerea is inversely correlated with vegetative and generative growth in bean and cucumber, but not in tomato. Australas. Plant Pathol. 42, 485–490. doi: 10.1007/s13313-013-0207-1
Bouchereau, A., Aziz, A., Larher, F., and Martin-Tanguy, J. (1999). Polyamines and environmental challenges: recent development. Plant Sci. 140, 103–125. doi: 10.1016/S0168-9452(98)00218-0
Capell, T., Bassie, L., and Christou, P. (2004). Modulation of the polyamine biosynthetic pathway in transgenic rice confers tolerance to drought stress. Proc. Natl. Acad. Sci. U.S.A. 101, 9909–9914. doi: 10.1073/pnas.0306974101
D’Agostino, L., Di Pietro, M., and Di Luccia, A. (2005). Nuclear aggregates of polyamines are supramolecular structures that play a crucial role in genomic DNA protection and conformation. FEBS J. 272, 3777–3787. doi: 10.1111/j.1742-4658.2005.04782.x
Del Duca, S., Dondini, L., Della Mea, M., Munoz de Rueda, P., and Serafini-Fracassini, D. (2000). Factors affecting transglutaminase activity catalysing polyamine conjugation to endogenous substrates in the entire chloroplast. Plant Physiol. Biochem. 38, 429–439. doi: 10.1016/S0981-9428(00)00761-0
De Gara, L., de Pinto, M. C., and Tommasi, F. (2003). The antioxidant systems vis-à-vis reactive oxygen species during plant–pathogen interaction. Plant Physiol. Biochem. 41, 863–870. doi: 10.1016/S0981-9428(03)00135-9
Dondini, L., Del Duca, S., Dall’Agata, L., Bassi, R., Gastaldelli, M., Della Mea, M., et al. (2003). Suborganellar localisation and effect of light on Helianthus tuberosus chloroplast transglutaminases and their substrates. Planta 217, 84–95. doi: 10.1007/s00425-003-0998-3
Evans, P. T., and Malmberg, R. L. (1989). Do polyamines have roles in plant development. Annu. Rev. Plant Physiol. Plant Mol. Biol. 40, 235–269. doi: 10.1146/annurev.pp.40.060189.001315
Fu, X. Z., Xing, F., Wang, N. Q., Peng, L. Z., Chun, C. P., Cao, L., et al. (2014). Exogenous spermine pretreatment confers tolerance to combined high-temperature and drought stress in vitro in trifoliate orange seedlings via modulation of antioxidative capacity and expression of stress-related genes. Biotechnol. Biotechnol. Equip. 28, 192–198. doi: 10.1080/13102818.2014.909152
Galston, A. W., and Sawhney, R. K. (1990). Polyamines in plant physiology. Plant Physiol. 94, 406–410. doi: 10.1104/PP.94.2.406
Gonzalez, M. E., Marco, F., Minguet, E. G., Carrasco-Sorli, P., Blázquez, M. A., Carbonell, J., et al. (2011). Perturbation of spermine synthase gene expression and transcript profiling provide new insights on the role of the tetraamine spermine in Arabidopsis defense against Pseudomonas viridiflava. Plant Physiol. 156, 2266–2277. doi: 10.1104/pp.110.171413
Govrin, E. M., and Levine, A. (2000). The hypersensitive response facilitates plant infection by the necrotrophic pathogen Botrytis cinerea. Curr. Biol. 10, 751–757. doi: 10.1016/S0960-9822(00)00560-1
Groppa, M. D., and Benavides, M. P. (2008). Polyamines and abiotic stress: recent advances. Amino Acids 34, 35–45. doi: 10.1007/s00726-007-0501-8
Groppa, M. D., Tomaro, M. L., and Benavides, M. P. (2007). Polyamines and heavy metal stress: the antioxidant behavior of spermine in cadmium- and copper-treated wheat leaves. Biometals 20, 185–195. doi: 10.1007/s10534-006-9026-y
Hatsugai, N., Kuroyanagi, M., Yamada, K., Meshi, T., Tsuda, S., Kondo, M., et al. (2004). A plant vacuolar protease, VPE, mediates virus-induced hypersensitive cell death. Science 305, 855–858. doi10.1126/science.1099859
Heath, M. C. (2000). “Hypersensitive Response-Related death,” in Programmed Cell Death in Higher Plants. eds E. Lam, H. Fukuda, J. Greenberg (Dordrecht: Springer Netherlands), 77–90. doi: 10.1007/978-94-010-0934-8_6
Jiménez-Bremont, J. F., Marina, M., Guerrero-González, M., de la L., Rossi, F. R., Sánchez-Rangel, D., Rodríguez-Kessler, M., et al. (2014). Physiological and molecular implications of plant polyamine metabolism during biotic interactions. Front. Plant Sci. 5:95. doi: 10.3389/fpls.2014.00095
Jones, J. D. G., and Dangl, J. L. (2006). The plant immune system. Nature 444, 323–329. doi: 10.1038/nature05286
Keen, N. T. (1990). Gene-fot-gene complementarity in plant-pathogen interactions. Annu. Rev. Genet. 24, 447–463. doi: 10.1146/annurev.ge.24.120190.002311
Kim, S. H., Kim, S. H., Yoo, S. J., Min, K. H., Nam, S. H., Cho, B. H., et al. (2013). Putrescine regulating by stress-responsive MAPK cascade contributes to bacterial pathogen defense in Arabidopsis. Biochem. Biophys. Res. Commun. 437, 502–508. doi: 10.1016/j.bbrc.2013.06.080
Kusano, T., Yamaguchi, K., Berberich, T., and Takahashi, Y. (2007). The polyamine spermine rescues Arabidopsis from salinity and drought stresses. Plant Signal. Behav. 2, 251–252. doi: 10.4161/psb.2.4.3866
Lam, E., Kato, N., and Lawton, M. (2001). Programmed cell death, mitochondria and the plant hypersensitive response. Nature 411, 848–853. doi: 10.1038/35081184
Liu, J.-H., Kitashiba, H., Wang, J., Ban, Y., and Moriguchi, T. (2007). Polyamines and their ability to provide environmental stress tolerance to plants. Plant Biotechnol. J. 24, 117–126. doi10.5511/plantbiotechnology.24.117
Marco, F., Busó, E., and Carrasco, P. (2014). Overexpression of SAMDC1 gene in Arabidopsis thaliana increases expression of defense-related genes as well as resistance to Pseudomonas syringae and Hyaloperonospora arabidopsidis. Front. Plant Sci. 5:115. doi: 10.3389/fpls.2014.00115
Marina, M., Maiale, S. J., Rossi, F. R., Romero, M. F., Rivas, E. I., Gárriz, A., et al. (2008). Apoplastic polyamine oxidation plays different roles in local responses of tobacco to infection by the necrotrophic fungus Sclerotinia sclerotiorum and the biotrophic bacterium Pseudomonas viridiflava. Plant Physiol. 147, 2164–2178. doi: 10.1104/pp.108.122614
Marina, M., Sirera, F. V., Rambla, J. L., Gonzalez, M. E., Blázquez, M. A., Carbonell, J., et al. (2013). Thermospermine catabolism increases Arabidopsis thaliana resistance to Pseudomonas viridiflava. J. Exp. Bot. 64, 1393–1402. doi: 10.1093/jxb/ert012
Martin-Tanguy, J. (2001). Metabolism and function of polyamines in plants: recent development (new approaches). Plant Growth Regulat. 34, 135–148. doi: 10.1023/A:1013343106574
Minocha, R., Majumdar, R., and Minocha, S. C. (2014). Polyamines and abiotic stress in plants: a complex relationship. Front. Plant Sci. 5:175. doi: 10.3389/fpls.2014.00175
Mitsuya, Y., Takahashi, Y., Berberich, T., Miyazaki, A., Matsumura, H., Takahashi, H., et al. (2009). Spermine signaling plays a significant role in the defense response of Arabidopsis thaliana to cucumber mosaic virus. J. Plant Physiol. 166, 626–643. doi: 10.1016/J.JPLPH.2008.08.006
Mo, H., Wang, X., Zhang, Y., Zhang, G., Zhang, J., and Ma, Z. (2015). Cotton polyamine oxidase is required for spermine and camalexin signalling in the defence response to Verticillium dahliae. Plant J. 83, 962–975. doi: 10.1111/tpj.12941
Moffatt, J., Hashimoto, M., Kojima, A., Kennedy, D. O., Murakami, A., Koshimizu, K., et al. (2000). Apoptosis induced by 1’-acetoxychavicol acetate in Ehrlich ascites tumor cells is associated with modulation of polyamine metabolism and caspase-3 activation. Carcinogenesis 21, 2151–2157. doi: 10.1093/carcin/21.12.2151
Moschou, P. N., Sarris, P. F., Skandalis, N., Andriopoulou, A. H., Paschalidis, K. A., Panopoulos, N. J., et al. (2009). Engineered polyamine catabolism preinduces tolerance of tobacco to bacteria and oomycetes. Plant Physiol. 149, 1970–1981. doi: 10.1104/pp.108.134932
Nahar, K., Hasanuzzaman, M., Alam, M. M., Rahman, A., Mahmud, J.-A., Suzuki, T., et al. (2016a). Insights into spermine-induced combined high temperature and drought tolerance in mung bean: osmoregulation and roles of antioxidant and glyoxalase system. Protoplasma 254, 445–460. doi: 10.1007/s00709-016-0965-z
Nahar, K., Rahman, M., Hasanuzzaman, M., Alam, M. M., Rahman, A., Suzuki, T., et al. (2016b). Physiological and biochemical mechanisms of spermine-induced cadmium stress tolerance in mung bean (Vigna radiata L.) seedlings. Environ. Sci. Pollut. Res. 23, 21206–21218. doi: 10.1007/s11356-016-7295-8
Ozawa, R., Bertea, C. M., Foti, M., Narayana, R., Arimura, G.-I., Muroi, A., et al. (2009). Exogenous polyamines elicit herbivore-induced volatiles in lima bean leaves: involvement of calcium, H2O2 and jasmonic acid. Plant Cell Physiol. 50, 2183–2199. doi: 10.1093/pcp/pcp153
Popovic, R. B., Kyle, D. J., Cohen, A. S., and Zalik, S. (1979). Stabilization of thylakoid membranes by spermine during stress-induced senescence of barley leaf discs. Plant Physiol. 64, 721–726. doi: 10.1104/PP.64.5.721
Radhakrishnan, R., and Lee, I.-J. (2013). Spermine promotes acclimation to osmotic stress by modifying antioxidant, abscisic acid, and jasmonic acid signals in soybean. J. Plant Growth Regulat. 32, 22–30. doi: 10.1007/s00344-012-9274-8
Romero, F. M., Maiale, S. J., Rossi, F. R., Marina, M., Ruíz, O. A., and Gárriz, A. (2018). “Polyamine metabolism responses to biotic and abiotic stress,” in Polyamines: Methods and Protocols. Methods in Molecular Biology, Vol. 1694, eds R. Alcázar and A. F. Tiburcio (New York, NY: Springer Business Media), 37–49. doi: 10.1007/978-1-4939-7398-9_3
Ruiz-Herrera, J., Ruiz-Medrano, R., and Domínguez, A. (1995). Selective inhibition of cytosine-DNA methylases by polyamines. FEBS Lett. 357, 192–196. doi: 10.1016/0014-5793(94)01360-D
Sagor, G. H. M., Cong, R.-Z., Berberich, T., Takahashi, H., Takahashi, Y., and Kusano, T. (2009). Spermine signaling in defense reaction against avirulent viral pathogen in Arabidopsis thaliana. Plant Signal. Behav. 4, 316–318. doi: 10.1016/j.jplph.2008.08.006
Sánchez-Rodríguez, E., Romero, L., and Ruiz, J. M. (2016). Accumulation of free polyamines enhances the antioxidant response in fruits of grafted tomato plants under water stress. J. Plant Physiol. 190, 72–78. doi: 10.1016/J.JPLPH.2015.10.010
Seifi, H. S., Curvers, K., De Vleesschauwer, D., Delaere, I., Aziz, A., and Höfte, M. (2013). Concurrent overactivation of the cytosolic glutamine synthetase and the GABA shunt in the ABA-deficient sitiens mutant of tomato leads to resistance against Botrytis cinerea. New Phytol. 199, 490–504. doi: 10.1111/nph.12283
Sequera-Mutiozabal, M. I., Erban, A., Kopka, J., Atanasov, K. E., Bastida, J., Fotopoulos, V., et al. (2016). Global metabolic profiling of Arabidopsis polyamine oxidase 4 (AtPAO4) loss-of-function mutants exhibiting delayed dark-induced senescence. Front. Plant Sci. 7:173. doi: 10.3389/fpls.2016.00173
Serafini-Fracassini, D., Di Sandro, A., and Del Duca, S. (2010). Spermine delays leaf senescence in Lactuca sativa and prevents the decay of chloroplast photosystems. Plant Physiol. Biochem. 48, 602–611. doi: 10.1016/j.plaphy.2010.03.005
Shelp, B. J., Bozzo, G. G., Trobacher, C. P., Zarei, C. P., Deyman, K. L., and Brikis, C. J. (2012). Hypothesis/review: contribution of putrescine to 4-aminobutyrate (GABA) production in response to abiotic stress. Plant Sci. 19, 130–135. doi: 10.1016/j.plantsci.2012.06.001
Shelp, B. J., Deyman, K. L., DeEll, J. R., and Bozzo, G. G. (2018). Polyamine homeostasis in apple fruit stored under multiple abiotic stresses. Can. J. Plant Sci. 99, 88–92. doi: 10.1139/CJPS-2018-0173
Shi, J., Fu, X.-Z., Peng, T., Huang, X.-S., Fan, Q.-J., and Liu, J.-H. (2010). Spermine pretreatment confers dehydration tolerance of citrus in vitro plants via modulation of antioxidative capacity and stomatal response. Tree Physiol. 30, 914–922. doi: 10.1093/treephys/tpq030
Stefanelli, C., Stanić, I., Zini, M., Bonavita, F., Flamigni, F., Zambonin, L., et al. (2000). Polyamines directly induce release of cytochrome c from heart mitochondria. Biochem. J. 347, 875–880. doi: 10.1042/BJ3470875
Takahashi, Y., Berberich, T., Miyazaki, A., Seo, S., Ohashi, Y., and Kusano, T. (2003). Spermine signalling in tobacco: activation of mitogen-activated protein kinases by spermine is mediated through mitochondrial dysfunction. Plant J. 36, 820–829. doi: 10.1046/j.1365-313X.2003.01923.x
Takahashi, Y., Uehara, Y., Berberich, T., Ito, A., Saitoh, H., Miyazaki, A., et al. (2004). A subset of hypersensitive response marker genes, including HSR203J, is the downstream target of a spermine signal transduction pathway in tobacco. Plant J. 40, 586–595. doi: 10.1111/j.1365-313X.2004.02234.x
Todorova, D., Katerova, Z., Shopova, E., Jodinskiene, M., Jurkoniene, S., and Sergiev, I. (2016). Responses of pea plants to heat stress and spermine treatment. Zemdirbyste Agriculture 103, 99–106. doi: 10.13080/z-a.2016.103.013
Torres, M. A., Jones, J. D. G., and Dangl, J. L. (2006). Reactive oxygen species signaling in response to pathogens. Plant Physiol. 141, 373–378. doi: 10.1104/PP.106.079467
Urano, K., Yoshiba, Y., Nanjo, T., Igarashi, Y., Seki, M., Sekiguchi, F., et al. (2003). Characterization of Arabidopsis genes involved in biosynthesis of polyamines in abiotic stress responses and developmental stages. Plant Cell Environ. 26, 1917–1926. doi: 10.1046/j.1365-3040.2003.01108.x
Van Breusegem, F., and Dat, J. F. (2006). Reactive oxygen species in plant cell death. Plant Physiol. 141, 384–390. doi: 10.1104/pp.106.078295
Van Bockhaven, J., De Vleesschauwer, D., and Höfte, M. (2013). Towards establishing broad-spectrum disease resistance in plants: silicon leads the way. J. Exp. Bot. 64, 1281–1293. doi: 10.1093/jxb/ers329
Van Camp, W., Van Montagu, M., and Inzé, D. (1998). H2O2 and NO: redox signals in disease resistance. Trends Plant Sci. 3, 330–334. doi: 10.1016/S1360-1385(98)01297-7
Walters, D. (2003b). Resistance to plant pathogens: possible roles for free polyamines and polyamine catabolism. New Phytol. 159, 109–115. doi: 10.1046/j.1469-8137.2003.00802.x
Walters, D. R. (2000). Polyamines in plant–microbe interactions. Physiol. Mol. Plant Pathol. 57, 137–146. doi: 10.1006/PMPP.2000.0286
Walters, D. R. (2003a). Polyamines and plant disease. Phytochemistry 64, 97–107. doi: 10.1016/S0031-9422(03)00329-7
Wojtaszek, P. (1997). Oxidative burst: an early plant response to pathogen infection. Acta Physiol. Plant 19, 581–589. doi: 10.1007/s11738-997-0057-y
Yamaguchi, K., Takahashi, Y., Berberich, T., Imai, A., Miyazaki, A., Takahashi, T., et al. (2006). The polyamine spermine protects against high salt stress in Arabidopsis thaliana. FEBS Lett. 580, 6783–6788. doi: 10.1016/j.febslet.2006.10.078
Yamakawa, H., Kamada, H., Satoh, M., and Ohashi, Y. (1998). Spermine is a salicylate-independent endogenous inducer for both tobacco acidic pathogenesis-related proteins and resistance against tobacco mosaic virus infection. Plant Physiol. 118, 1213–1222. doi: 10.1104/PP.118.4.1213
Yoda, H., Fujimura, K., Takahashi, H., Munemura, I., Uchimiya, H., and Sano, H. (2009). Polyamines as a common source of hydrogen peroxide in host- and nonhost hypersensitive response during pathogen infection. Plant Mol. Biol. 70, 103–112. doi: 10.1007/s11103-009-9459-0
Keywords: abiotic stress, biotic stress, defense response, defense activator, signaling, spermine
Citation: Seifi HS and Shelp BJ (2019) Spermine Differentially Refines Plant Defense Responses Against Biotic and Abiotic Stresses. Front. Plant Sci. 10:117. doi: 10.3389/fpls.2019.00117
Received: 18 December 2018; Accepted: 23 January 2019;
Published: 08 February 2019.
Edited by:
Rubén Alcázar, University of Barcelona, SpainReviewed by:
Thomas Berberich, Senckenberg Nature Research Society, GermanyAndrés Gárriz, Consejo Nacional de Investigaciones Científicas y Técnicas (CONICET), Argentina
Copyright © 2019 Seifi and Shelp. This is an open-access article distributed under the terms of the Creative Commons Attribution License (CC BY). The use, distribution or reproduction in other forums is permitted, provided the original author(s) and the copyright owner(s) are credited and that the original publication in this journal is cited, in accordance with accepted academic practice. No use, distribution or reproduction is permitted which does not comply with these terms.
*Correspondence: Barry J. Shelp, YnNoZWxwQHVvZ3VlbHBoLmNh