- 1Department of Plant Physiology, University of Agriculture of Krakow, Krakow, Poland
- 2Department of Grasslands, Institute of Technology and Life Sciences (ITP), Raszyn, Poland
- 3Department of Plant Physiology, Faculty of Agriculture and Biology, Warsaw University of Life Sciences (SGGW), Warsaw, Poland
One hundred and nine accessions of spring barley seedlings were phenotyped under soil drought conditions. Chlorophyll fluorescence induction (OJIP) parameters, leaf water content, relative turgidity, net assimilation rate (PN), and water use efficiency (WUE) of plants were measured. All the tested lines were genotyped by means of DArT sequencing (DArTseq) technology. For association mapping a 11,780 polymorphic DArTseq and 4,725 DArTseq SNP markers were used. Our results revealed dissimilar patterns of the relationships between OJIP-parameters under control and drought conditions. A high level of correlation between parameters characterizing Photosystem's II (PSII) energy trapping efficiency (Fv/Fm) and photochemical events downstream of PSII reaction center (e.g., Performance Index—PICSo) was observed only in the case of drought-treated plants. Generally, OJIP parameters were correlated with leaf water content (less in control). This correlation was weaker with WUE, and absent with PN. Under drought stress, 6,252 genotype × phenotype associations, which passed false discovery rate (FDR) verification, were found between all the studied phenotypic characteristics (23, including 19 OJIP parameters) and 2,721 markers. On the other hand, only 282 associations passed FDR test in the control. They comprised 22 phenotypic parameters and 205 markers. Probing for gene annotations of sequences was performed for markers associated with Fv/Fm for both drought and control, markers were associated with studied traits in both control and drought, as well as for markers associated with both OJIP and other physiological parameters in drought. Our work allowed us to conclude that drought treatment differentiates the studied lines through the revealing of relationships between water content and the damages to PSII reaction centers or different components of PSII energy transfer chain. Moreover, the former was not connected with net photosynthesis rate.
Introduction
Chlorophyll fluorescence measurements, including studies of induction kinetics in dark adapted samples followed by OJIP analysis, have been commonly used for studies of plant reaction to abiotic stresses (Kalaji et al., 2016). These include nitrogen deficiency (Cetner et al., 2017; Kalaji et al., 2017; Samborska et al., 2018) as well as low (Fracheboud et al., 1999; Rapacz et al., 2015) and high (Pastenes and Horton, 1996; Azam et al., 2015) temperature or salinity (Dabrowski et al., 2016). Also water stresses, like waterlogging (Bertholdsson et al., 2015) and especially water deficit (Li et al., 2006; Guo et al., 2008; Poormohammad Kiani et al., 2008; Czyczyło-Mysza et al., 2011; Wang et al., 2012), were widely studied using chlorophyll fluorescence techniques.
OJIP analysis may indicate electron fluxes between different components upstream, inside and downstream of PSII, thus they may indicate different points of drought-induced damages. Indeed it was possible to observe that the parameters of OJIP can very precisely characterize the state of leaf hydration (Živčák et al., 2008; Goltsev et al., 2012). However, the possible effects of water deficit on chlorophyll fluorescence parameters do not appear to be a simple reflection of the reduced energy demand from the dark photosynthesis phase.
The problem of drought tolerance is complex in general. Even the physiological and agronomic definition of drought tolerance varies. The physiological tolerance to drought requires the plant to maintain its vitality so that it can produce a minimum quantity of seeds or simply survive, while agronomic tolerance requires maintaining an economically significant yield (Schafleitner et al., 2007). Leaf hydration, critical for physiological tolerance, is not necessarily associated with drought tolerance in the agronomic sense, where the ability to maintain relatively high carboxylation is crucial for maintaining high productivity under drought (Blum, 2005; Ruggiero et al., 2017).
In our previous studies on tolerance to moderate water deficit for barley accessions with high carboxylation rates in drought under Middle-European conditions, we showed higher yielding potential in a dry environment (Rapacz et al., 2010; Wójcik-Jagła et al., 2012). However, no correlation between leaf hydration and net assimilation rate in drought was observed, considering both the lack of phenotypic correlations and the absence of common quantitative trait loci (QTLs) (Rapacz et al., 2010; Wójcik-Jagła et al., 2013). On the other hand, photosynthetic capacity in drought was clearly related to the expression level of the dehydrine encoding gene HVA1 (Rapacz et al., 2010; Wójcik-Jagła et al., 2012). This complex relationship was probably connected to the proposed mechanism of the induction of HVA1 expression in drought, which is triggered by intact changes of turgor in mesophyll cells sensed by the actin cytoskeleton (Wójcik-Jagła et al., 2012; Sniegowska-Swierk et al., 2015, 2016). To summarize, these studies showed that in barley, high photosynthetic activity can be maintained during moderate drought if the plants effectively protect the cells from dehydration irrespective of maintaining high turgidity at the cost of closing the stomata.
The basic effect of drought on photosynthesis is connected with stomatal limitation of carboxylation, where stomata closure results in a simultaneous decrease in water loss and CO2 uptake, which is the most challenging problem for breeding drought-tolerant cultivars (Lawson and Blatt, 2014; Flexas, 2016). Unfortunately, a higher water use efficiency (WUE) value results in lower stomatal conductance, which is often linked to a decrease of photosynthetic capacity, reducing productivity (Lawson and Blatt, 2014; Ruggiero et al., 2017). The stomatal limitation of photosynthesis in drought is the main, but not the only, effect of water deficit on photosynthesis. Metabolic impairment connected with deficiency of Calvin cycle intermediates, known as non-stomatal limitation, is observed under moderate drought conditions (Flexas and Medrano, 2002; Perlikowski et al., 2014).
Photosynthetic light-phase processes, directly studied by chlorophyll-fluorescence, are not considered as primary damage sites to photosynthetic apparatuses under water deficit (Kaiser, 1987; Cornic and Massacci, 1996). They are susceptible to secondary damage connected with photoinhibition, which occurs when the amount of energy absorbed by photosynthetic antennas exceeds the energy requirements of the dark photosynthesis phase (Sanda et al., 2011). In drought, this demand decreases mainly due to the stomata closing and, consequently, reactive oxygen species (ROS) production increases. Enhanced production of ROS in chloroplasts and the activation of hydrolytic enzymes in drought initiate damage of proteins, nucleic acids, and membrane lipids (Mittler, 2002). As a consequence, the photosynthetic electron transport chain is disturbed, but ROS are simultaneously signals necessary for maintaining redox homeostasis (Foyer and Shigeoka, 2011). Therefore, moderate drought can induce some adaptation of PSII observed as increasing energy fluxes for energy trapping and electron transfer (Kosmala et al., 2012). Similar reactions are also observed under heat or light excess conditions (Oukarroum et al., 2009; Pospíšil, 2016).
Decreased PSII activity under drought conditions is connected with cell membrane damage triggered by ROS and by disturbed lipid metabolism (Benhassaine-Kesri et al., 2002; Gallé and Feller, 2007). Additionally, dehydration affects cell turgor, causing mechanical damage to cellular membranes (Wolfe and Bryant, 1999). It has been proven that, unlike drought-sensitive plants, tolerant plants can maintain the integration of cell membranes in drought (Yu and Li, 2014). This phenomenon is connected with adaptive modifications of lipid composition in cellular membranes observed during water deficit in tolerant plants. It implies plastid membrane alterations, which in consequence greatly influences preserving transmembrane protein functions and thus eventually photosystem activities (Quartacci et al., 1995, 2000; Gigon et al., 2004; Degenkolbe et al., 2012; Perlikowski et al., 2016).
Plant drought stress is connected with the accumulation of low-molecular weight particles of complex function such as proline. Proline, which has long been considered a biochemical indicator of drought tolerance, can play an antioxidative and membrane-stabilizing function (Van Rensburg et al., 1993; Hayat et al., 2012). During drought, proline may affect the photosynthetic apparatus indirectly, through the osmotic action conducive to the maintenance of RWC, but also directly through the stabilization of cell membranes and RuBisCO.
Also ABA, which is a major plant hormone involved in plants' drought response and is considered as another biochemical indicator of drought tolerance, can affect both photosynthesis and chlorophyll fluorescence in drought by increasing relative water content in leaves (Corrêa de Souza et al., 2013; Mehrotra et al., 2014).
Despite the very well proved linkage between electron flows in PSII and leaf relative water content, the relationship between the latter and drought tolerance is still unclear. Therefore, we tried in this experiment to verify the hypothesis that the observed differences in chlorophyll fluorescence parameters in drought-treated plants are connected rather with leaf hydration and not with the efficiency of the carboxylation process. To confirm this hypothesis, in addition to the physiological tools, such as direct measurements of chlorophyll fluorescence, water status and gas exchange, we also attempted to screen the barley genome for the regions associated with OJIP parameters, gas exchange and water status in drought. We also tried to identify some genes affecting these relationships to suggest a possible explanation for this rather unexpected observation.
Materials and Methods
Plant Materials
The experiments were performed on the population of 109 accessions of barley consisting of doubled haploid-derived lines and F8 generation breeding materials from two breeding companies in Poland: HR Strzelce Ltd. group IHAR (Strzelce) and HR Danko sp. z o.o. (Choryń). The accessions were preselected by drought tolerance and targeted MAS, as described in detail elsewhere (Wójcik-Jagła et al., 2018). The complete list of accessions is available as Supplemental Material (Supplemental Table S1).
Plant Growth and Drought Treatment
Twelve seeds of each accession were sown in a pot (5 dm3) in a mixture of universal garden soil substrate (Ekoziem, Jurków, Poland) and sand (1:1, v:v). To allow subsequent measurements only at midday, 10 accessions per day were sown. Pots were randomized in a growth chamber set to 25°C/17°C (day/night). A photoperiod of 14/10 h was applied and the light (400 μmol m−2 s−1) was provided by high-pressure sodium (HPS) lamps (SON-T+ AGRO, Philips, Brussels, Belgium). The plants were watered to 10% of the soil dry weight (equal to 3 pF—logarithm from soil matrix potential, based on soil water retention curve), which was checked daily by weighing. Once a week, fertilization with Florovit multi-component fertilizer (Inco, Góra Kalwaria, Poland) was performed. Soil drought was applied at 4th leaf stage by decreasing watering to reach 4.0 pF (3.5% of the soil dry weight). At this pF, visual symptoms of reduced turgidity were visible in all accessions. The drought treatment lasted 10 days after reaching the required pF and soil moisture retention curve. Phenotypic measurements were taken 1 day before starting the soil water content decrease (control) and at the last day of drought treatment.
Phenotyping
The measurements and samplings were always performed between 11 a.m. and 2 p.m. because of diurnal changes expected for gas exchange and chlorophyll fluorescence.
Chlorophyll fluorescence measurements were taken in the middle part of the second leaf of 10 plants per accession. Measurements of the fast chlorophyll fluorescence induction kinetics were taken with Handy PEA (Hansatech, Kings Lynn, UK), with saturating flash intensity ca. 3,000 μmol m−2 s−1 and measurement time 1 s. Measurements of photo-induced chlorophyll fluorescence transients were used to calculate characteristics of the light phase of photosynthesis according to the OJIP algorithm (Strasser et al., 2004; Gururani et al., 2015). Measured and calculated parameters are listed in Table 1 and detailed calculation formulas can be found elsewhere (Gururani et al., 2015; Rapacz et al., 2015).
Plant gas exchange parameters were also measured in the middle part of the second barley leaves with a Ciras-3 infrared gas analyzer (PP Systems, MA, United States) equipped with a Universal Leaf Chamber (PLC6). The controlled conditions of the measurements were as follows: CO2 concentration of 400 μmol mol−1, relative humidity of 30%, irradiance of 500 μmol m−2 s−1, and leaf temperature of 25°C. The measurements were taken in 10 plants per accession. From the measured gas exchange parameters, two were used for further calculations: net CO2 assimilation rate (PN) and stomatal conductance (gs). Both were used for calculation of water use efficiency (WUE): WUEi = PN/gs (Flexas, 2016).
Measurements of leaf water status were performed on eight randomly selected first (the oldest) leaves of each accession. After cutting, the leaves were weighed (fresh weight; FW), placed in 25-ml closed tubes filled with water, and shaken in darkness. After 24 h the turgor weight (TW) was determined and the leaves were then dried for 48 h in paper envelopes at a temperature of 70°C in the laboratory dryer (Lumel, Zielona Góra, Poland). The dry weight (DW) was then determined. Relative water content (RWC) or relative turgidity was calculated as RWC [%] = (FW – DW)/(TW – DW) × 100% and water content (WC) as WC [g H2O/ g DW] = (FW – DW)/DW (Barrs, 1968).
Genotyping and Genome Wide Associations
One hundred and nine selected spring barley lines were genotyped using DArT sequencing technology (DArTseq) (https://www.diversityarrays.com/products-and-services/applications/), as previously described (Wójcik-Jagła et al., 2018). The genotyping resulted in 15,828 specific DArTseqs and 7,829 SNPs. The input data for the population's structure and association analysis were only markers with Polymorphism Information Content (PIC) > 18% (11,780 DArTseqs and 4,725 SNPs). Association analysis included the entire genome (GWAS). The analysis of the population structure was carried out using the STRUCTURE v. 2.3.4 software (Stanford University, California), (Pritchard et al., 2000). The following admixture model was selected: 10,000 cycles and 1,000 repetitions per cycle. The test was carried out 10 times for six possible subpopulations (K = 1–6). The true value of K parameter was determined in the manner described by Evanno et al. (2005). The marker-trait associations were determined using the TASSEL program with TASSEL software (Ithaca, New York, NY, United States) (Bradbury et al., 2007), as demonstrated previously (Wójcik-Jagła et al., 2018).
A minimum allele frequency of 0.05 was required for the genotype data. The degree of kinship of the population used in this study was strictly controlled, therefore we did not perform any correction for kinship. The general linear model (GLM) was used to determine the associations, finding the ordinary least squares solution for each marker-trait association (Bradbury et al., 2007). The probability level threshold was 0.001. The additive model was used for data analysis. Obtained probability values were corrected using the false discovery rate (FDR) (Benjamini and Hochberg, 1995) and Bonferroni correction (Dunn, 1958).
Annotations for Markers
The homology of selected barley DarTseq/SNP marker sequences with potential functional genes or proteins was confirmed with the BLASTn algorithm at http://blast.ncbi.nlm.nih.gov (Altschul et al., 1990) using discontiguous megablast as a selection program. The characterization of identified homologs with the highest similarity estimated on Qc (query cover) and Id (sequence identity) parameters was done with GeneBank datasets at https://www.ncbi.nlm.nih.gov/genbank/ (Benson et al., 2012). The identification of potential genes in the sequence of the barley genome was conducted by the ViroBLAST server at http://webblast.ipk-gatersleben.de (Deng et al., 2007) on a base from the barley high-confidence genes database (HC_genes_CDS_seq_2012). Moreover, functional annotations and gene ontology of selected sequences were confirmed by the sets from the UniProt and InterPro databases, located at http://www.uniprot.org/ (Magrane and Uniprot Consortium, 2011) and https://www.ebi.ac.uk/interpro/search/sequence-search (Mitchell et al., 2015), respectively. Conserved domain identification in marker sequences was confirmed by the NCBI Conserved Domain Database (CDD), located at https://www.ncbi.nlm.nih.gov/Structure/cdd/cdd.shtml (Marchler-Bauer et al., 2015).
Statistical Treatment of Phenotype Data
Data was processed using Statistica 13.1PL software (Statsoft, Tulsa, OK). Statistical significance of the drought effect on phenotypic data was checked by means of multifactor ANOVA in a General Linear Model (GLM) with accession and environment as factors. Normal distribution of the data was confirmed with histograms and Shapiro-Wilk testing. Pearson's correlation coefficients between phenotypic data were calculated based on the mean values for the accession. Principal component analyses (PCA) were performed by eigenvalue decomposition of a data correlation matrix.
Results
Drought significantly affected all the physiological parameters studied in this study. With exception to ETo/RC, DIo/RC, ϕEo and Ψo, the values of all parameters decreased under drought conditions, including an over 4-fold reduction of PN and 2-fold reduction in WC (Table 2). Among OJIP parameters, the relative magnitude of change was the highest in the case of TRo/CS and RC/CSm, which indicates that the drought mainly limited the number of active PSII reaction centers and thus the amount of trapped energy per leaf cross-section. All the changes were highly statistically significant, with exception of DIo/RC, which was significant at P = 0.05 (Supplemental Table S1). Net assimilation rate (PN) in both environments was not correlated with other physiological parameters with the exception of WUE, which was measured with the same method (Table 2). WC and RWC were correlated with WUE in drought only (Table 2). In well-watered plants, only some correlations between OJIP parameters and WC and RWC were observed (Table 2). This included negative correlations with phenomenological energy fluxes at different stages of electron transport (…/CS), RC/CSm and positive with Fv/Fm. The values of correlation coefficients were always under 0.5. Under drought stress, physiological parameters related to leaf water status directly (WC, RWC) or indirectly (WUE) were correlated with more OJIP parameters than in the control, but the values of correlation coefficients were still rather low. They were correlated with OJIP parameters related to PSII efficiency (e.g., Fv/Fm, Ψo, ϕEo, PIABS, PICSo, PICSm) and activity of single PSII reaction centers (…/RC) (Table 2). It may be suggested that the main reason for drought-induced changes in OJIP parameters is direct damage to Photosystem II (PSII) reaction centers or different components of the energy transfer chain in PSII. This may be additionally confirmed by the fact that the parameter characterizing energy trapping efficiency in PSII reaction centers (Fv/Fm), which is affected by PSII dysfunction, was highly correlated with the majority of OJIP parameters, including performance indexes (PIABS, PICSo, PICSm) only in drought and not under control conditions.
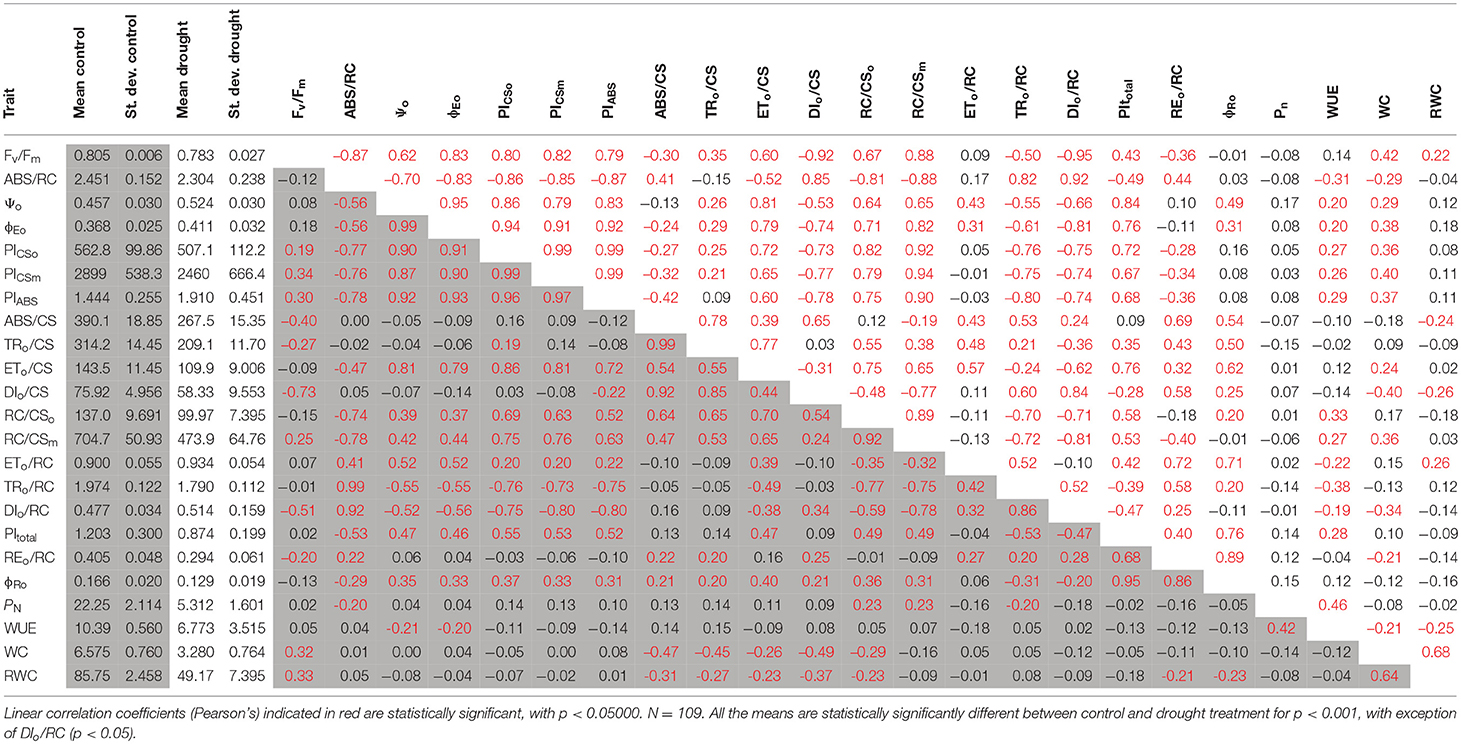
Table 2. Means, standard deviations and correlation matrix between studied phenotypic characteristics measured under control (shaded) and after 10 days of drought treatment (4 pF, no shading) conditions.
The various patterns of the relationships between different OJIP parameters, leaf water relations and PN in the control and drought-treated plants were also shown by principal component analysis (PCA) (Figure 1 and Supplementary Table S2). In both control and drought-treated plants, the first two principal components described much more than 50% of the total variation (60 and 70% for the control and drought-treated plants, respectively) and did not reveal physiological subpopulations of the studied accessions. Only some outliers, different for both environments, were present. In both environments, WC and RWC (in drought also WUE) discriminated the accessions in a similar way to that of Fv/Fm and DIo/CS (consider that higher values of DIo/CS indicated low energy trapping efficiency in PSII reaction centers as lower values of Fv/Fm do). ETo/RC had a very similar but weaker effect on the accession coordinates to those observed for WC and RWC in the control conditions. Under drought conditions, the accession discrimination effects of RWC, WC, and WUE were similar to those of all the performance indexes for energy conservation from exciton to the reduction of intersystem electron acceptors (PIABS, PICSo, PICSm) and the numbers of active PSII reaction centers (RC/CSo, RC/CSm). In both environments, net assimilation rate (PN) had a small effect on the differences between accessions, and it was similar to those of OJIP parameters describing efficiency of electron transport in PSII and at the PSI end acceptors side (as ETo/CS, ϕRo, or PItotal). On the other hand, the direction of accession discrimination for PN was similar to that of the number of active PSII reaction centers (RC/CSo, RC/CSm) in well-watered plants only.
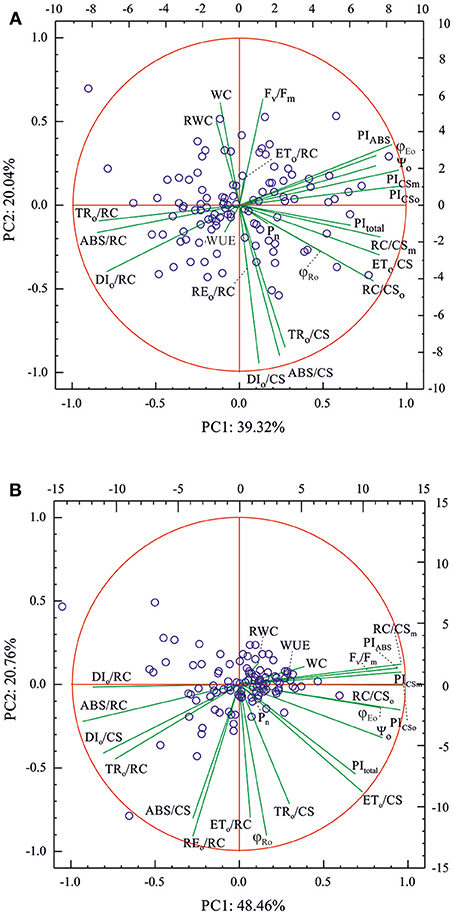
Figure 1. Biplots of principal component analysis (PCA) for OJIP parameters, leaf water relations (WC, RWC), WUE and PN (variables) measured in 109 barley accessions (cases) in (A) well-watering conditions (control) and (B) in drought. Left y and bottom x axes refer to variables and right y and top x axes for the cases, respectively.
In the control, 282 associations between phenotypes and markers, which passed false discovery rate (FDR) verification were found (Supplementary Table S3). They comprised 22 phenotypic parameters and 205 markers. On the other hand, under drought stress 6,252 genotype × phenotype associations passed the FDR test (Supplementary Table S4). These associations were found between all the studied phenotypic characteristics (23, including 19 OJIP parameters) and 2,721 markers.
Fv/Fm, describing the efficiency of energy trapping in PSII reaction centers, is the most commonly analyzed chlorophyll fluorescence parameter. In our study, it showed a similar relation with leaf water status in the control and drought, but it was correlated with efficiencies of further steps in photosynthetic energy transfer only in drought. Thus, it was chosen for testing for gene annotations of associated marker sequences (Tables 3, 4 and Supplementary Tables S5, S6). In well-watered plants, Fv/Fm values were associated with 20 markers, including 5 SNPs. Among them, 5 sequences were annotated to protein sequences in the UniProt database and only one with GO annotation for barley protein (proteolytic activity) (Table 3). On the other hand, in drought-treated plants Fv/Fm values were associated with 40 markers, among them 8 (including 5 SNPs) have GO annotations for proteins (Table 4). Four of them may possess protein kinase activity, three protein binding activity and one sequence (3432879) has 100% homology with wheat's 3-ketoacyl-CoA synthase, a transferase involved in the fatty acid biosynthesis process. Interestingly, another marker (3262177) associated with OJIP parameters in both environments shows 100% homology with the sequence of genes encoding the same enzyme in barley (Table 5). Thus, this protein may be important for energy conversion and transfer efficiency in thylakoids regardless of drought.
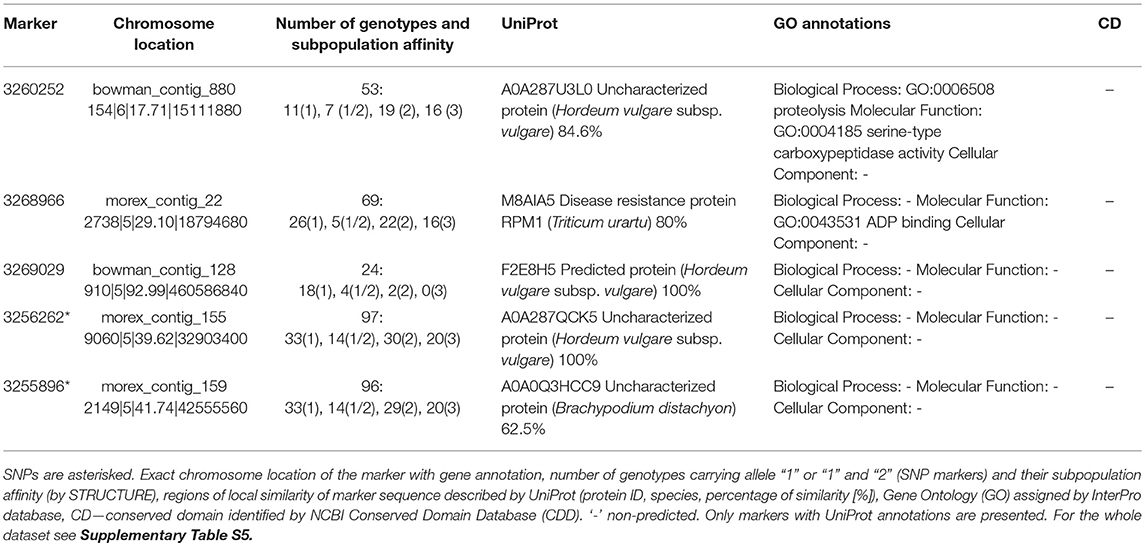
Table 3. Gene annotations of DArTseq and SNP markers associated with energy trapping efficiency in PSII (Fv/Fm) in well-watered barley (control).
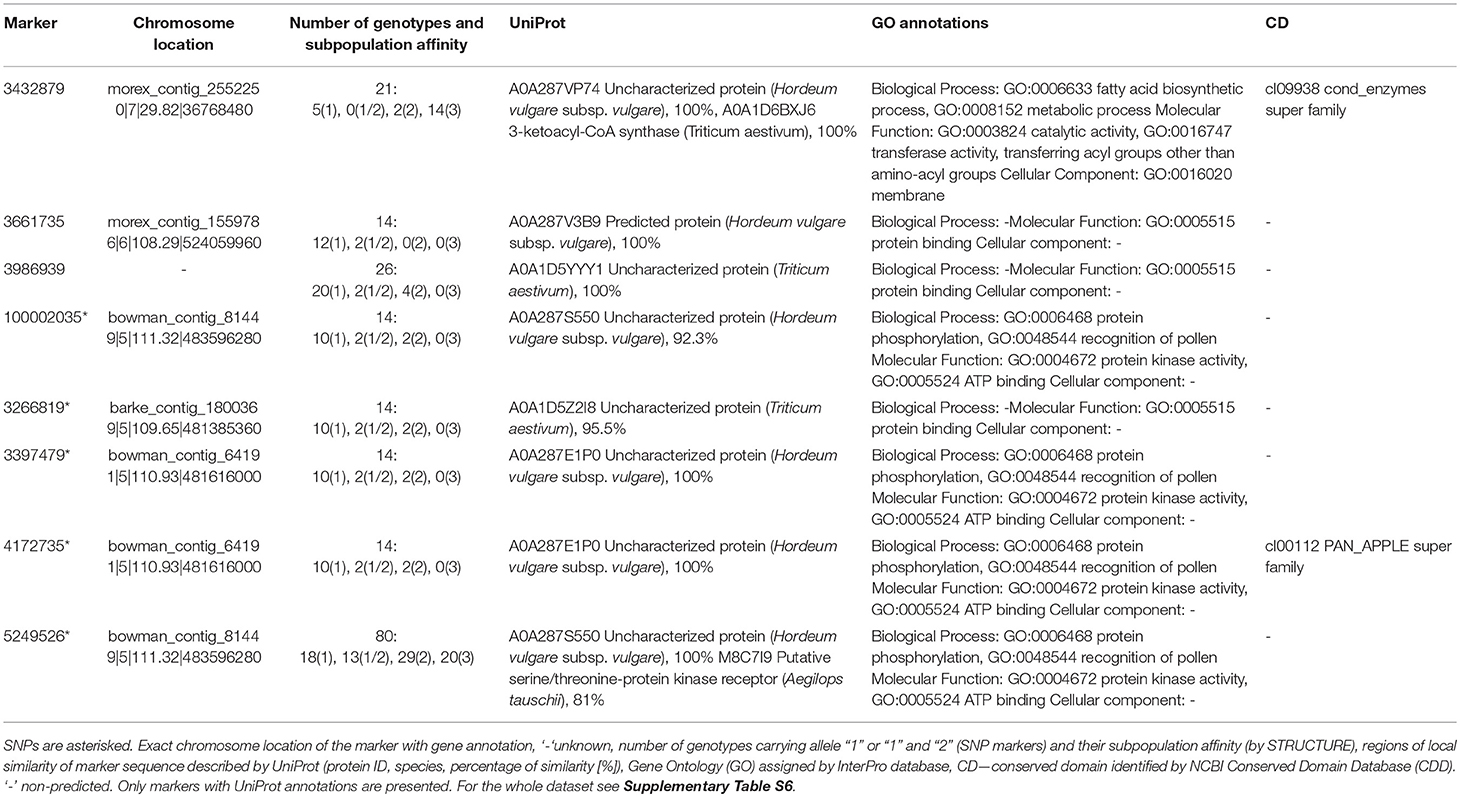
Table 4. Gene annotations of DArTseq and SNP markers associated with energy trapping efficiency in PSII (Fv/Fm) in barley plants under drought.
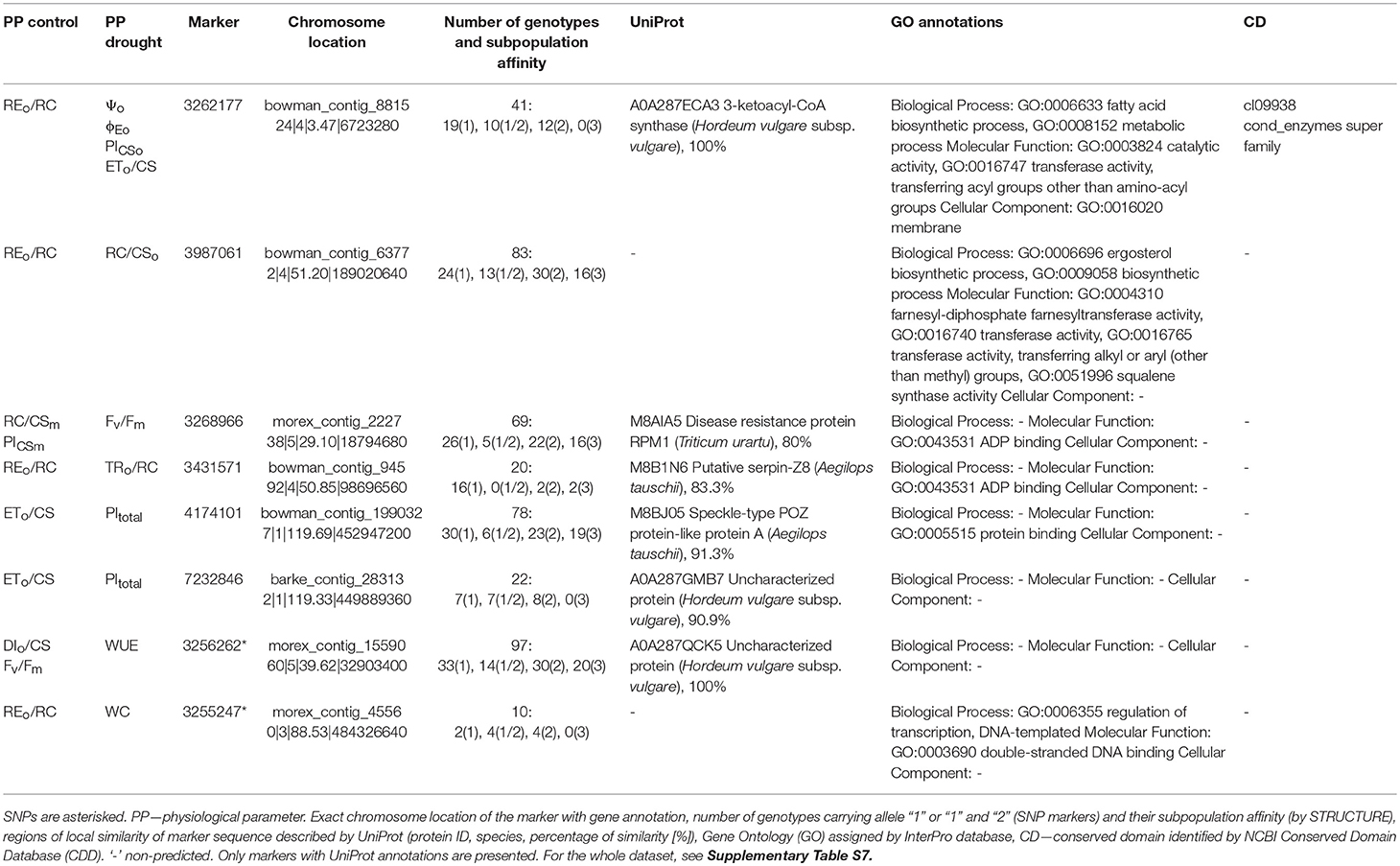
Table 5. Gene annotations of DArTseq and SNP markers associated with physiological parameters in both well-watered (control) and drought-treated barley plants.
Forty-one markers were associated with phenotypic traits, both in control and drought (28 with annotations found) (Supplementary Table S7). Under control conditions, eight of these common markers, including the aforementioned 3262177, were associated with electron flux reducing end electron acceptors at the Photosystem I (PSI) acceptor side per reaction center (RC) (REo/RC), while in drought-treated plants the same markers were associated with OJIP parameters characterizing upstream stages of the electron transport or even water content in leaves. Additionally, four further markers were associated with WUE in drought-treated plants while in the control, with some OJIP parameters. In eight cases, UniProt or/and GO annotations were identified for markers common for drought and well-watered plants (Table 5). With the exception of 3262177 and SNP 3256262 (putative DNA-binding transcription factor), other sequences were not fully characterized with respect to their function and/or with high sequence homology to barley proteins. In one case, GO molecular function of protein partially encoded by a marker indicated ergosterol biosynthesis (not synthesized in higher plants) and in other cases proteins with ADP or protein binding activities.
In the context of drought effect on plants, what is most interesting are the markers associated with physiological parameters belonging to at least two of the following three groups: gas exchange (WUE, PN), leaf water status (WC, RWC) and OJIP parameters (Table 6 and Supplementary Table S8). A total of 162 associations meeting these criteria were found (Supplementary Table S8). Among them, none of the PN-related sequences were found, which confirmed the very small relationship between net assimilation rate and both OJIP and leaf water status parameters in drought-treated barley. A total of 51 associations did not include OJIP parameters, and the associated sequences were common for RWC and WUE in 48 cases and in three cases for WC and RWC. Furthermore, 29 sequences associated with both WUE and RWC and additionally with OJIP parameters were found, which confirms a strong, authentic and not only statistical link between RWC and WUE, which are measured by completely different methods but were related to stomatal conductance. In any case, WC was associated with the same sequences as OJIP parameters. Among 112 associations common for OJIP parameters and WUE/RWC, all were related to the parameters connected with energy fluxes in single PSII reaction centers, mainly with TRo/RC and ABS/RC. In 25 cases, RWC/WUE shared the same associated markers with the number of active reaction centers (RC/CSo, RC/CSm), and in a further 9 cases also with at least one PSII performance index for energy conservation from exciton to the reduction of intersystem electron acceptors (PIABS, PICSo, PICSm). Only one case association common for OJIP parameters and other physiological characteristics concerned a parameter describing phenomenological energy fluxes per leaf cross-section, and in no case was there a parameter describing photochemical activity at the Photosystem I (PSI) acceptors side.
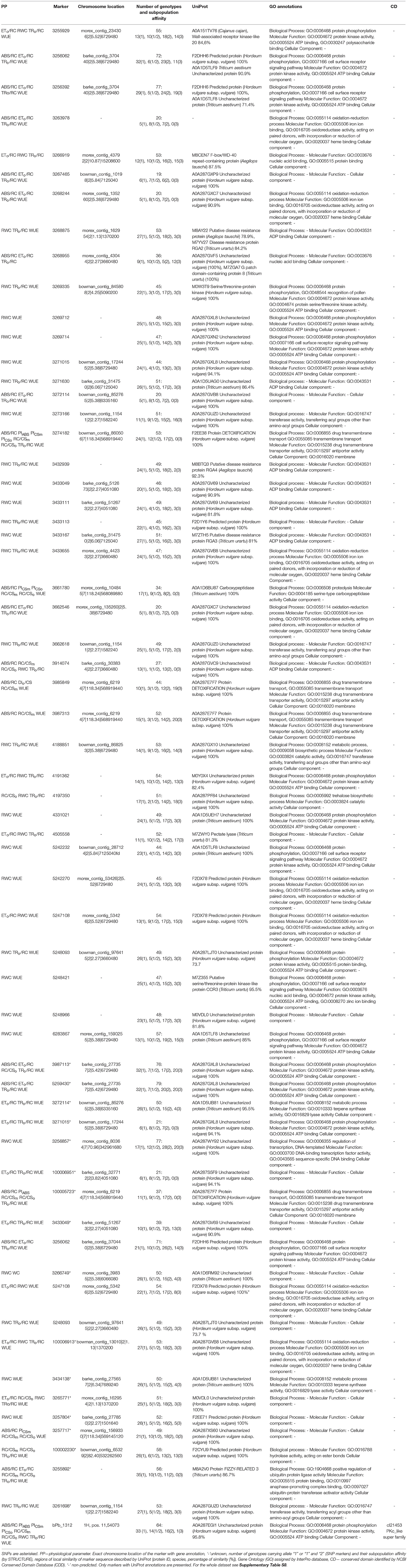
Table 6. Gene annotations of DArTseq and SNP markers associated with at least two physiological traits in barley plants under drought with the exception of the markers associated only with chlorophyll fluorescence parameters.
Among the 162 associations mentioned above, 67 were annotated to known sequences, of which 57 were registered in the UniProt and/or GO database (Table 6). Possible function of proteins encoded by these marker sequences may be clearly grouped. (1) Some of them may be involved in cell surface signal perception, signal transduction and broadly understood gene expression regulation. The sequence of marker 3255929 was annotated to cell wall-associated receptor kinase-like protein which may be responsible for polysaccharide signal perception. Three further markers-−3256062, 3256392 and probably 6283867—are parts of the coding sequence of the same gene encoding barley's protein (F2DHH6), which is involved in the cell surface receptor signaling pathway. The same biological process is also annotated for two additional putative barley protein kinases, encoded partially by the sequences 3269714 and 5242232. Signal recognition on the cell surface is predicted for the protein, which is barley serine/threonine-protein kinase involved in pollen recognition and is encoded in part by the sequence 3269335. Five markers, including three SNPs (3269712, 3271015; SNPs: 3987113, 5259430, 3271015) are different parts of the sequence of a protein with kinase activity (barleys A0A287GXL8). These SNP sequences were associated with WUE and some OJIP parameters, and the rest of markers with WUE and RWC. Sequence 5248421 was annotated to the CCR3 gene from Triticum urartu encoding protein involved in the cell surface receptor signaling pathway and possessing both protein kinase and (according to GO) nucleic acid binding activity. Also, two further markers are parts of the sequence of protein kinases: 4191362 and 4331021. Some of the kinases mentioned before may be involved not in signal transduction but metabolic activation. Some of the identified sequences encoded transcription factors (3266919 and SNP 3256857) and others are involved in proteolysis (3661780), including ubiquination (SNP 3255892).
(2) Other proteins encoded in part by the markers considered herein are involved in oxidation-reduction processes. They have very similar activity connected to binding iron ions and acting on paired donors with incorporation or reduction of molecular oxygen. Seven marker sequences matching coding regions of four proteins (three proteins are pointed by the pairs of markers) were found (markers: 3263978, 3268244 and 3662546, 5242270 and 5247108, 3433655 and SNP 100006913). Sequences of three of them have been assigned to cytochromes P450. The general role of cytochrome P450 is detoxification and, in our study, other candidate proteins with detoxification functions were identified. Barley protein A0A287E7F7 is considered by the UniProt database as a detoxification protein and, according to GO, is involved in transmembrane transport. Three markers (3985849, 3987313, SNP 100005723) associated with WUE and different chlorophyll fluorescence parameters were found within its sequence. Additionally, marker 3274182 was annotated to different protein with the same UniProt and GO categories.
(3) The next group consists of proteins involved probably in disease resistance (3268875, 3433167) or different metabolic processes, among which some may be connected to cell walls biosynthesis and hydrolysis. Sequence 4505558 was annotated to pectate lyase (in IPK barley database it is annotated to receptor-like kinase) and SNP 100002230 was a part of the sequence of barley's protein F2CYU9 with GO molecular function of hydrolase activity, acting on ester bonds (which may hydrolyze ester bonds between ferulic acid and sugars in cell walls). Trehalose biosynthesis was annotated to marker sequence 4197350. In turn, transferase activity was annotated to two different barley proteins, encoded partially in one case by the sequence 4188851 and in the other by sequences 3662618, 3273166 and SNP 326169. Terpene synthase activity (SNP 3434138) was also related to the physiological parameters discussed here. The rest of the sequences encoded uncharacterized proteins or proteins classified by GO for molecular function as ADP binding activity.
Another approach to determine a population's structure was to use only markers associated with the studied traits and being the parts of the sequences identified in databases as parts of potential genes (candidate gene subpopulations). In this approach, the population structure revealed four subpopulations (Figure 2). This structure was different from the one observed for the studied population with the whole marker set (“genome-wide” subpopulations). Only genome-wide subpopulation 3 was over-represented in candidate gene subpopulation 3. This indicates that distribution of candidate gene alleles was independent of genomic structure of the studied barley population and independent of the origin of the lines (Table S1).
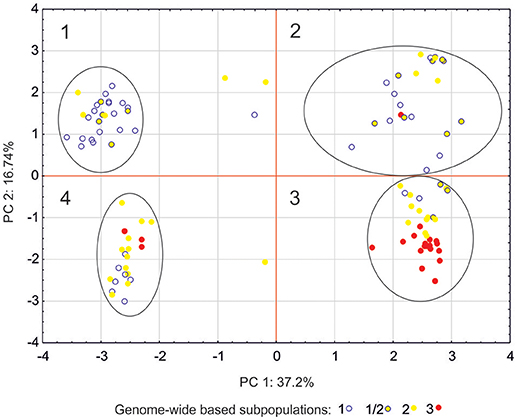
Figure 2. Principal component analysis (PCA) for markers associated with the studied traits and connected with the sequences identified in databases as a part of potential genes. Affinity of each genotype to one of the groupings (“candidate genes subpopulation”) can be found in Table S1.
Discussion
In the present study, the results of chlorophyll fluorescence measurements followed by OJIP testing corresponded well with leaf water status of both drought-treated and control plants of barley, but not with CO2 net assimilation rate. This relationship, at least in drought, may be a result of direct damaging of PSII reaction centers or other elements of the photosynthetic electron transport chain under conditions of leaf water deficit. It should be noted here that chlorophyll fluorescence parameters and CO2 assimilation were reported as not always closely related, especially under field or stress conditions already in the early stages of development of chlorophyll fluorescence measurement techniques (Genty et al., 1989; Edwards and Baker, 1993). In the present study, drought reduced the number of active reaction centers, while further steps for photosynthetic electron transfer were less affected. On the other hand, according to PCA analysis, PN, albeit poorly differentiated, discriminated our accessions similarly to PItotal in both environments, while only in the control in a way similar to quantum yield of reduction of end electron acceptors at the PSI acceptor side (ϕRo). This means that, although in both environments net assimilation rate is to some extent connected with overall photochemical efficiency, in the control it is more closely related to the activity of thylakoid electron end-acceptors.
Our study confirmed that drought-induced changes in OJIP parameters are very sensitive to changes in relative water content (RWC) in leaves. This parameter reflects the balance between water supply to the leaf tissue and transpiration rate, and thus it is considered as an important indicator of plants' water status (Schonfeld et al., 1988; Soltys-Kalina et al., 2016). Water use efficiency (WUE) is a critical measure that determines the balance between photosynthetic carbon assimilation and transpiration (Farquhar et al., 1982). In our study, in drought-treated plants WUE was negatively correlated with RWC and shared many common associations within the barley genome with RWC and OJIP parameters. The negative correlation means that plants losing less water in drought had problems with maintaining high PN, which is a typical problem the in breeding of drought tolerant crops (Lawson and Blatt, 2014; Ruggiero et al., 2017). On the other hand, in our study chlorophyll fluorescence parameters measured in drought-treated plants are not clearly connected with net assimilation rate, which is crucial for drought tolerance in the agronomic sense (Blum, 2005).
A possible explanation of this phenomenon may be suggested by the results obtained with the help of genome-wide associations (GWA). Unlike most studies using GWA to find new QTLs (He et al., 2017; Maulana et al., 2018), in our experiment we tried to identify genes in which polymorphisms of sequences associated with phenotypic traits connected with drought tolerance occur. GWA confirmed that in our population, drought-induced differences in chlorophyll fluorescence parameters were connected with water status of the leaves by the existence of some common associations with the genome. This highlights the fact that sensitivity of photosynthetic electron transport to water deficit is crucial for the observed differences between barley accessions. Markers associated in drought with both leaf water relations (RWC and WUE) and chlorophyll fluorescence parameters are correlated mostly with parameters describing the number and activities of single PSII reaction centers, while in the control the correlation is with those connected with further steps of photosynthetic electron transports. GWA is commonly used for unraveling the genetic basis of quantitative traits in populations occurring in natural conditions (Myles et al., 2009), but in our study we used this method to explain the physiological effects of drought observed in the population. For that reason, the population that we used was of a very specific kind. We selected accessions that were proven to have a very diverse response to drought—from highly tolerant to very susceptible. That explains why the difference in the number of obtained significant associations was so large for control and drought-treated plants (282 and 6,252, respectively).
Drought tolerance is a complex trait controlled by small effect genes, many of which have not yet been functionally characterized, which was also pointed out in our study (Fleury et al., 2010; Ruggiero et al., 2017). Candidate genes for leaf water relations and chlorophyll fluorescence parameters in the drought-treated plants selected herein encode proteins which are not directly involved in the control of chloroplast bioenergetics; however, they may be involved in its regulation and response to water deficit signal, with special focus to membranes and cell wall metabolism. Many of the candidate genes for RWC/WUE and chlorophyll fluorescence parameters in drought are protein kinases (e.g., F2DHH6, M0W3T9). This group of signal proteins may be involved both in the general drought stress signaling and in the regulation of stomatal control of water loss (Ruggiero et al., 2017). Special attention should be paid to kinases that may potentially act in the cell surface signal perception and/or be associated with cell walls (e.g., homolog of A0A151TV76). The former studies in barley showed that actin microfilament reorganization resulting from cell wall–plasma membrane interactions after RWC decrease are involved in ABA-independent drought signal recognition and response, including the expression of HVA1 dehydrine (Sniegowska-Swierk et al., 2016). Another candidate gene, encoding zinc finger transcription factor CCR3, is not only involved in cellular surface signal recognition but also in the control of lignin biosynthesis (Bi et al., 2011). Changes in lignin contents and composition are an important part of water loss regulation in drought (Moura et al., 2010). Candidate genes for drought response in the barley studied herein included also homologs of three multiple disease resistance genes (RGA2, RGA3, and RGA4). These proteins with homologs common in many plants are in general responsible for pathogen recognition and restriction of its growth (Liang et al., 2015). Mechanisms of this recognition is not clear but it may be connected with plasma membrane–cell wall adhesion, which has a very important function in pathogen detection and plant defense response (Underwood, 2012). Candidate gene F2CYU9 with GO molecular function of hydrolase activity, acting on ester bonds, may hydrolyze ester bonds between ferulic acid and sugars in cell walls. This process was previously identified as important for drought tolerance in cereals (Hura et al., 2012). Moreover, in triticale the content of cell wall-bound phenolics in drought share common loci (QCWPh.4B) with some OJIP parameters (Fv/Fm and ABS/CS).
Among the predicted enzymatic activities for proteins encoded in part by genes associated in our experiment with drought response in barley, trehalose synthesis was also identified. Trehalose was identified as an important element of plant drought tolerance (Fernandez et al., 2010).
Candidate genes were also found for associations common in both studied environments (drought and control). Most of them were annotated to posttranslational protein modifications or signal transduction as having protein kinases or protein binding activity, while one of the associated sequences has 100% homology with barley protein A0A287ECA encoding 3-ketoacyl-CoA synthase. The activity of these synthases is a part of log-chain fatty acid biosynthesis and in higher plants, including Arabidopsis and barley, they are involved in wax biosynthesis and cuticula formation (Weidenbach et al., 2014). This may explain their association with chlorophyll fluorescence parameters in drought where they strongly depend on plant water status. Neither can it be excluded that some changes in plant water status may also affect chlorophyll fluorescence in the control.
Studies of QTLs and markers, including GWAS/SNPs associated with chlorophyll fluorescence parameters, including drought treatment were performed before, show in general very complex interactions and multiple control of chlorophyll fluorescence parameters (Yin et al., 2010; Czyczyło-Mysza et al., 2011, 2013; Hao et al., 2012; Hura et al., 2017). However, direct comparison of these results with those obtained in our study is not possible because most of them were based on QTL linkage mapping using bi-parental populations, which means that most QTLs are population-specific and/or were performed with different species.
Conclusions
Drought treatment differentiated the studied accessions of barley through revealing relationships between water status of the leaf and its photosynthetic efficiency, measured by means of chlorophyll fluorescence parameters. Variations in these characteristics, however, were not directly connected with net photosynthesis rate. Thus, chlorophyll fluorescence measurements and OJIP testing seem to be reliable tools for estimating plant water status under drought, including RWC and WUE, but not its photosynthetic activity. Additionally, pointing to the candidate genes connected with RWC, WUE and chlorophyll fluorescence parameters, our results may contribute to the physiological and molecular dissection of drought response in barley, which is the first step of understanding the complex mechanisms that control drought tolerance for future molecular breeding programs (Mir et al., 2012).
Author Contributions
MR and JK designed the experiments. MW-J, AF, and JK conducted the experimental work. MW-J, AF, MR, and HK were responsible for data processing, bioinformatics, and statistical analysis. MR and HK interpreted the main results and drew the main conclusions. MR prepared the first version of the manuscript, but all the authors contributed in writing of the final version and approved the manuscript.
Conflict of Interest Statement
The authors declare that the research was conducted in the absence of any commercial or financial relationships that could be construed as a potential conflict of interest.
Acknowledgments
Plant phenotyping and genotyping were performed within project GENMARK (PBS1/A8/1/2012), supported by the National Centre for Research and Development (Poland). The further data recalculation, processing, manuscript writing and publication were supported by the Ministry of Science and Higher Education (Poland) within the framework of subsidies for maintenance of research potential granted for the Faculty of Agriculture and Economics of the Agricultural University in Kraków.
Supplementary Material
The Supplementary Material for this article can be found online at: https://www.frontiersin.org/articles/10.3389/fpls.2019.00078/full#supplementary-material
Supplementary Table S1. Population's structure obtained by using STRUCTURE software.
Supplementary Table S2. Statistics for phenotyping: F-test for comparison between phenotypic characteristics in the control and in drought; Variable contributions and principal component coordinates of cases (accessions) for PCA analysis showed in Figure 1A (control) and Figure 1B (drought).
Supplementary Table S3. Associations of DArTseq and SNP markers with physiological parameters in well-watered barley plants (control) after FDR correction.
Supplementary Table S4. Associations of DArTseq and SNP markers with physiological parameters in drought-treated barley plants after FDR correction.
Supplementary Table S5. Sequences and related data from NCBI, Gene Bank (protein ID) and IPK Barley databases for markers associated with energy trapping efficiency in PSII (Fv/Fm) in well-watered barley (control). Markers indicated in green are included in Table 3.
Supplementary Table S6. Sequences and related data from NCBI, Gene Bank (protein ID) and IPK Barley databases for markers associated with energy trapping efficiency in PSII (Fv/Fm) in barley plants under drought. Markers indicated in green are included in Table 4.
Supplementary Table S7. Sequences and related data from NCBI, Gene Bank (protein ID) and IPK Barley databases for markers associated with physiological parameters in both well-watered (control) and drought-treated barley plants. Markers indicated in green are included in Table 5.
Supplementary Table S8. Sequences and related data from NCBI, Gene Bank (protein ID) and IPK Barley databases for markers associated with at least two physiological traits in barley plants under drought with exception to the markers associated only with chlorophyll fluorescence parameters. Markers indicated in green are included in Table 6.
Abbreviations
Chlorophyll fluorescence parameters, see Table 1; FDR, false discovery rate; GO, gene ontology; gs, stomatal conductance; OJIPchlorophyll fluorescence transient—the letters OJIP refer to the specific time points in the induction curve; PN, net CO2 assimilation rate; PSI, PSII, photosystem I and II, respectively; ROS, reactive oxygen species; RWC, relative water content (relative turgidity); WC, water content; WUE, water use efficiency.
References
Altschul, S. F., Gish, W., Miller, W., Myers, E. W., and Lipman, D. J. (1990). Basic local alignment search tool. J. Mol. Biol. 215, 403–410. doi: 10.1016/S0022-2836(05)80360-2.
Azam, F. I., Chang, X., and Jing, R. (2015). Mapping QTL for chlorophyll fluorescence kinetics parameters at seedling stage as indicators of heat tolerance in wheat. Euphytica 202, 245–258. doi: 10.1007/s10681-014-1283-1.
Barrs, H. (1968). “Determination of water deficits in plant tissues,” in Water Deficits and Plant Growth, Vol. 1, ed T. Kozlowski (New York, NY; London: Academic Press), 235–368.
Benhassaine-Kesri, G., Aid, F., Demandre, C., Kader, J.-C., and Mazliak, P. (2002). Drought stress affects chloroplast lipid metabolism in rape (Brassica napus) leaves. Physiol. Plant. 115, 221–227. doi: 10.1034/j.1399-3054.2002.1150207.x
Benjamini, Y., and Hochberg, Y. (1995). Controlling the false discovery rate: a practical and powerful approach to multiple testing. J. R. Stat. Soc. Ser. B 57, 289–300. doi: 10.2307/2346101
Benson, D. A., Cavanaugh, M., Clark, K., Karsch-Mizrachi, I., Lipman, D. J., Ostell, J., et al. (2012). GenBank. Nucleic Acids Res. 41, D36–D42. doi: 10.1093/nar/gks1195
Bertholdsson, N.-O., Holefors, A., Macaulay, M., and Crespo-Herrera, L. A. (2015). QTL for chlorophyll fluorescence of barley plants grown at low oxygen concentration in hydroponics to simulate waterlogging. Euphytica 201, 357–365. doi: 10.1007/s10681-014-1215-0
Bi, C., Chen, F., Jackson, L., Gill, B. S., and Li, W. (2011). Expression of lignin biosynthetic genes in wheat during development and upon infection by fungal pathogens. Plant Mol. Biol. Report. 29, 149–161. doi: 10.1007/s11105-010-0219-8
Blum, A. (2005). Drought resistance, water-use efficiency, and yield potential - Are they compatible, dissonant, or mutually exclusive? Aust. J. Agric. Res. 56, 1159–1168. doi: 10.1071/AR05069
Bradbury, P. J., Zhang, Z., Kroon, D. E., Casstevens, T. M., Ramdoss, Y., and Buckler, E. S. (2007). TASSEL: software for association mapping of complex traits in diverse samples. Bioinformatics 23, 2633–2635. doi: 10.1093/bioinformatics/btm308
Cetner, M. D., Kalaji, H. M., Goltsev, V., Aleksandrov, V., Kowalczyk, K., Borucki, W., et al. (2017). Effects of nitrogen-deficiency on efficiency of light-harvesting apparatus in radish. Plant Physiol. Biochem. 119, 81–92. doi: 10.1016/J.PLAPHY.2017.08.016
Cornic, G., and Massacci, A. (1996). “Leaf Photosynthesis Under Drought Stress,” in Photosynthesis and the Environment, ed. N. R. Baker (Dordrecht: Springer Netherlands), 347–366. doi: 10.1007/0-306-48135-9_14
Corrêa de Souza, T., Magalhães, P. C., de Castro, E. M., de Albuquerque, P. E. P., and Marabesi, M. A. (2013). The influence of ABA on water relation, photosynthesis parameters, and chlorophyll fluorescence under drought conditions in two maize hybrids with contrasting drought resistance. Acta Physiol. Plant. 35, 515–527. doi: 10.1007/s11738-012-1093-9
Czyczyło-Mysza, I., Marcinska, I., Skrzypek, E., Chrupek, M., Grzesiak, S., Hura, T., et al. (2011). Mapping QTLs for yield components and chlorophyll a fluorescence parameters in wheat under three levels of water availability. Plant Genet. Resour. 9, 291–295. doi: 10.1017/S1479262111000207
Czyczyło-Mysza, I., Tyrka, M., Marcinska, I., Skrzypek, E., Karbarz, M., Dziurka, M., et al. (2013). Quantitative trait loci for leaf chlorophyll fluorescence parameters, chlorophyll and carotenoid contents in relation to biomass and yield in bread wheat and their chromosome deletion bin assignments. Mol. Breed. 32, 189–210. doi: 10.1007/s11032-013-9862-8
Dabrowski, P., Baczewska, A. H., Pawluśkiewicz, B., Paunov, M., Alexantrov, V., Goltsev, V., et al. (2016). Prompt chlorophyll a fluorescence as a rapid tool for diagnostic changes in PSII structure inhibited by salt stress in Perennial ryegrass. J. Photochem. Photobiol. B Biol. 157, 22–31. doi: 10.1016/J.JPHOTOBIOL.2016.02.001
Degenkolbe, T., Giavalisco, P., Zuther, E., Seiwert, B., Hincha, D. K., and Willmitzer, L. (2012). Differential remodeling of the lipidome during cold acclimation in natural accessions of Arabidopsis Thaliana. Plant J. 72, 972–982. doi: 10.1111/tpj.12007
Deng, W., Nickle, D. C., Learn, G. H., Maust, B., and Mullins, J. I. (2007). ViroBLAST: a stand-alone BLAST web server for flexible queries of multiple databases and user's datasets. Bioinformatics 23, 2334–2336. doi: 10.1093/bioinformatics/btm331
Dunn, O. J. (1958). Estimation of the means of dependent variables. Ann. Math. Stat. 29, 1095–1111. doi: 10.1214/aoms/1177706443
Edwards, G. E., and Baker, N. R. (1993). Can CO2 assimilation in maize leaves be predicted accurately from chlorophyll fluorescence analysis? Photosynth. Res. 37, 89–102. doi: 10.1007/BF02187468
Evanno, G., Regnaut, S., and Goudet, J. (2005). Detecting the number of clusters of individuals using the software STRUCTURE: a simulation study. Mol. Ecol. 14, 2611–2620. doi: 10.1111/j.1365-294X.2005.02553.x
Farquhar, G., O'Leary, M., and Berry, J. (1982). On the relationship between carbon isotope discrimination and the intercellular carbon dioxide concentration in leaves. Aust. J. Plant Physiol. 9:121. doi: 10.1071/PP9820121
Fernandez, O., Béthencourt, L., Quero, A., Sangwan, R. S., and Clément, C. (2010). Trehalose and plant stress responses: friend or foe? Trends Plant Sci. 15, 409–417. doi: 10.1016/j.tplants.2010.04.004
Fleury, D., Jefferies, S., Kuchel, H., and Langridge, P. (2010). Genetic and genomic tools to improve drought tolerance in wheat. J. Exp. Bot. 61, 3211–3222. doi: 10.1093/jxb/erq152
Flexas, J. (2016). Genetic improvement of leaf photosynthesis and intrinsic water use efficiency in C3 plants: why so much little success? Plant Sci. 251, 155–161. doi: 10.1016/J.PLANTSCI.2016.05.002
Flexas, J., and Medrano, H. (2002). Drought-inhibition of photosynthesis in C3plants: Stomatal and non-stomatal limitations revisited. Ann. Bot. 89, 183–189. doi: 10.1093/aob/mcf027
Foyer, C. H., and Shigeoka, S. (2011). Understanding oxidative stress and antioxidant functions to enhance photosynthesis. Plant Physiol. 155, 93–100. doi: 10.1104/pp.110.166181
Fracheboud, Y., Haldimann, P., Leipner, J., and Stamp, P. (1999). Chlorophyll fluorescence as a selection tool for cold tolerance of photosynthesis in maize (Zea mays L.). J. Exp. Bot. 50, 1533–1540. doi: 10.1093/jxb/50.338.1533
Gallé, A., and Feller, U. (2007). Changes of photosynthetic traits in beech saplings (Fagus sylvatica) under severe drought stress and during recovery. Physiol. Plant. 131, 412–421. doi: 10.1111/j.1399-3054.2007.00972.x.
Genty, B., Briantais, J.-M., and Baker, N. R. (1989). The relationship between the quantum yield of photosynthetic electron transport and quenching of chlorophyll fluorescence. Biochim. Biophys. Acta Gen. Subj. 990, 87–92. doi: 10.1016/S0304-4165(89)80016-9
Gigon, A., Matos, A.-R., Laffray, D., Zuily-Fodil, Y., and Pham-Thi, A.-T. (2004). Effect of drought stress on lipid metabolism in the leaves of Arabidopsis thaliana (ecotype Columbia). Ann. Bot. 94, 345–351. doi: 10.1093/aob/mch150
Goltsev, V., Zaharieva, I., Chernev, P., Kouzmanova, M., Kalaji, H. M., Yordanov, I., et al. (2012). Drought-induced modifications of photosynthetic electron transport in intact leaves: analysis and use of neural networks as a tool for a rapid non-invasive estimation. Biochim. Biophys. Acta Bioenerg. 1817, 1490–1498. doi: 10.1016/J.BBABIO.2012.04.018
Guo, P., Baum, M., Varshney, R. K., Graner, A., Grando, S., and Ceccarelli, S. (2008). QTLs for chlorophyll and chlorophyll fluorescence parameters in barley under post-flowering drought. Euphytica 163, 203–214. doi: 10.1007/s10681-007-9629-6
Gururani, M. A., Venkatesh, J., Ganesan, M., Strasser, R. J., Han, Y., Kim, J.-I., et al. (2015). In vivo assessment of cold tolerance through Chlorophyll-a fluorescence in transgenic zoysiagrass expressing mutant phytochrome A. PLoS ONE 10:e0127200. doi: 10.1371/journal.pone.0127200
Hao, D., Chao, M., Yin, Z., and Yu, D. (2012). Genome-wide association analysis detecting significant single nucleotide polymorphisms for chlorophyll and chlorophyll fluorescence parameters in soybean (Glycine max) landraces. Euphytica 186, 919–931. doi: 10.1007/s10681-012-0697-x
Hayat, S., Hayat, Q., Alyemeni, M. N., Wani, A. S., Pichtel, J., and Ahmad, A. (2012). Role of proline under changing environments: a review. Plant Signal. Behav. 7, 1456–1466. doi: 10.4161/psb.21949
He, Y., Wu, D., Wei, D., Fu, Y., Cui, Y., Dong, H., et al. (2017). GWAS, QTL mapping and gene expression analyses in Brassica napus reveal genetic control of branching morphogenesis. Sci. Rep. 7:15971. doi: 10.1038/s41598-017-15976-4
Hura, T., Hura, K., Dziurka, K., Ostrowska, A., and Aczek-Kwinta, R. B. ?, Grzesiak, M. (2012). An increase in the content of cell wall-bound phenolics correlates with the productivity of triticale under soil drought. J. Plant Physiol. 169, 1728–1736. doi: 10.1016/j.jplph.2012.07.012
Hura, T., Tyrka, M., Hura, K., Ostrowska, A., and Dziurka, K. (2017). QTLs for cell wall-bound phenolics in relation to the photosynthetic apparatus activity and leaf water status under drought stress at different growth stages of triticale. Mol. Genet. Genomics 292, 415–433. doi: 10.1007/s00438-016-1276-y
Kaiser, W. M. (1987). Effects of water deficit on photosynthetic capacity. Physiol. Plant. 71, 142–149. doi: 10.1111/j.1399-3054.1987.tb04631.x
Kalaji, H. M., Dabrowski, P., Cetner, M. D., Samborska, I. A., Łukasik, I., Brestic, M., et al. (2017). A comparison between different chlorophyll content meters under nutrient deficiency conditions. J. Plant Nutr. 40, 1024–1034. doi: 10.1080/01904167.2016.1263323
Kalaji, H. M., Jajoo, A., Oukarroum, A., Brestic, M., Zivcak, M., Samborska, I. A., et al. (2016). Chlorophyll a fluorescence as a tool to monitor physiological status of plants under abiotic stress conditions. Acta Physiol. Plant. 38:102. doi: 10.1007/s11738-016-2113-y
Kosmala, A., Perlikowski, D., Pawłowicz, I., and Rapacz, M. (2012). Changes in the chloroplast proteome following water deficit and subsequent watering in a high- and a low-drought-tolerant genotype of Festuca arundinacea. J. Exp. Bot. 63, 6161–6172. doi: 10.1093/jxb/ers265
Lawson, T., and Blatt, M. R. (2014). Stomatal size, speed, and responsiveness impact on photosynthesis and water use efficiency. Plant Physiol. 164, 1556–1570. doi: 10.1104/pp.114.237107
Li, R., Guo, P., Michael, B., Stefania, G., and Salvatore, C. (2006). Evaluation of chlorophyll content and fluorescence parameters as indicators of drought tolerance in barley. Agric. Sci. China 5, 751–757. doi: 10.1016/S1671-2927(06)60120-X
Liang, Y., Zhang, D.-Y., Ouyang, S., Xie, J., Wu, Q., Wang, Z., et al. (2015). Dynamic evolution of resistance gene analogs in the orthologous genomic regions of powdery mildew resistance gene MlIW170 in Triticum dicoccoides and Aegilops tauschii. Theor. Appl. Genet. 128, 1617–1629. doi: 10.1007/s00122-015-2536-7
Magrane, M., and Uniprot, Consortium (2011). UniProt Knowledgebase: a hub of integrated protein data. Database 2011:bar009-bar009. doi: 10.1093/database/bar009
Marchler-Bauer, A., Derbyshire, M. K., Gonzales, N. R., Lu, S., Chitsaz, F., Geer, L. Y., et al. (2015). CDD: NCBI's conserved domain database. Nucleic Acids Res. 43, D222–D226. doi: 10.1093/nar/gku1221
Maulana, F., Ayalew, H., Anderson, J. D., Kumssa, T. T., Huang, W., and Ma, X.-F. (2018). Genome-wide association mapping of seedling heat tolerance in winter wheat. Front. Plant Sci. 9:1272. doi: 10.3389/fpls.2018.01272
Mehrotra, R., Bhalothia, P., Bansal, P., Basantani, M. K., Bharti, V., and Mehrotra, S. (2014). Abscisic acid and abiotic stress tolerance – different tiers of regulation. J. Plant Physiol. 171, 486–496. doi: 10.1016/J.JPLPH.2013.12.007
Mir, R. R., Zaman-Allah, M., Sreenivasulu, N., Trethowan, R., and Varshney, R. K. (2012). Integrated genomics, physiology and breeding approaches for improving drought tolerance in crops. Theor. Appl. Genet. 125, 625–645. doi: 10.1007/s00122-012-1904-9
Mitchell, A., Chang, H.-Y., Daugherty, L., Fraser, M., Hunter, S., Lopez, R., et al. (2015). The InterPro protein families database: the classification resource after 15 years. Nucleic Acids Res. 43, D213–D221. doi: 10.1093/nar/gku1243
Mittler, R. (2002). Oxidative stress, antioxidants and stress tolerance. Trends Plant Sci. 7, 405–410. doi: 10.1016/S1360-1385(02)02312-9
Moura, J. C. M. S., Bonine, C. A. V., de Oliveira Fernandes Viana, J., Dornelas, M. C., and Mazzafera, P. (2010). Abiotic and biotic stresses and changes in the lignin content and composition in plants. J. Integr. Plant Biol. 52, 360–376. doi: 10.1111/j.1744-7909.2010.00892.x
Myles, S., Peiffer, J., Brown, P. J., Ersoz, E. S., Zhang, Z., Costich, D. E., et al. (2009). Association mapping: critical considerations shift from genotyping to experimental design. Plant Cell 21, 2194–2202. doi: 10.1105/tpc.109.068437
Oukarroum, A., Schansker, G., and Strasser, R. J. (2009). Drought stress effects on photosystem I content and photosystem II thermotolerance analyzed using Chl a fluorescence kinetics in barley varieties differing in their drought tolerance. Physiol. Plant. 137, 188–199. doi: 10.1111/j.1399-3054.2009.01273.x
Pastenes, C., and Horton, P. (1996). Effect of high temperature on photosynthesis in beans (I. Oxygen Evolution and Chlorophyll Fluorescence). Plant Physiol. 112, 1245–1251. doi: 10.1104/PP.112.3.1245
Perlikowski, D., Kierszniowska, S., Sawikowska, A., Krajewski, P., Eckhardt, Ä., and Kosmala, A. (2016). Remodeling of leaf cellular glycerolipid composition under drought and re-hydration conditions in grasses from the Lolium-Festuca complex. Front. Plant Sci. 7:1027. doi: 10.3389/fpls.2016.01027
Perlikowski, D., Kosmala, A., Rapacz, M., Kościelniak, J., Pawłowicz, I., and Zwierzykowski, Z. (2014). Influence of short-term drought conditions and subsequent re-watering on the physiology and proteome of Lolium multiflorum / Festuca arundinacea introgression forms, with contrasting levels of tolerance to long-term drought. Plant Biol. 16, 385–394. doi: 10.1111/plb.12074
Poormohammad Kiani, S., Maury, P., Sarrafi, A., and Grieu, P. (2008). QTL analysis of chlorophyll fluorescence parameters in sunflower (Helianthus annuus L.) under well-watered and water-stressed conditions. Plant Sci. 175, 565–573. doi: 10.1016/J.PLANTSCI.2008.06.002
Pospíšil, P. (2016). Production of reactive oxygen species by photosystem ii as a response to light and temperature stress. Front. Plant Sci. 7:1950. doi: 10.3389/fpls.2016.01950
Pritchard, J. K., Stephens, M., Rosenberg, N. A., and Donnelly, P. (2000). Association mapping in structured populations. Am. J. Hum. Genet. 67, 170–181. doi: 10.1086/302959
Quartacci, M. F., Pinzino, C., Sgherri, C., and Navari-Izzo, F. (1995). Lipid composition and protein dynamics in thylakoids of two wheat cultivars differently sensitive to drought. Plant Physiol. 108, 191–197. doi: 10.1104/PP.108.1.191
Quartacci, M. F., Pinzino, C., Sgherri, C. L. M., Dalla Vecchia, F., and Navari-Izzo, F. (2000). Growth in excess copper induces changes in the lipid composition and fluidity of PSII-enriched membranes in wheat. Physiol. Plant. 108, 87–93. doi: 10.1034/j.1399-3054.2000.108001087.x
Rapacz, M., Kościelniak, J., Jurczyk, B., Adamska, A., and Wójcik, M. (2010). Different patterns of physiological and molecular response to drought in seedlings of malt- and feed-type barleys (Hordeum vulgare). J. Agron. Crop Sci. 196, 9–19. doi: 10.1111/j.1439-037X.2009.00389.x
Rapacz, M., Sasal, M., Kalaji, H. M., and Kościelniak, J. (2015). Is the OJIP test a reliable indicator of winter hardiness and freezing tolerance of common wheat and triticale under variable winter environments? PLoS ONE 10:e0134820. doi: 10.1371/journal.pone.0134820
Ruggiero, A., Punzo, P., Landi, S., Costa, A., Van Oosten, M., and Grillo, S. (2017). Improving plant water use efficiency through molecular genetics. Horticulturae 3:31. doi: 10.3390/horticulturae3020031
Samborska, I. A., Kalaji, H. M., Sieczko, L., Goltsev, V., Borucki, W., and Jajoo, A. (2018). Structural and functional disorder in the photosynthetic apparatus of radish plants under magnesium deficiency. Funct. Plant Biol. 45, 668–679. doi: 10.1071/FP17241
Sanda, S., Yoshida, K., Kuwano, M., Kawamura, T., Munekage, Y. N., Akashi, K., et al. (2011). Responses of the photosynthetic electron transport system to excess light energy caused by water deficit in wild watermelon. Physiol. Plant. 142, 247–264. doi: 10.1111/j.1399-3054.2011.01473.x
Schafleitner, R., Gutierrez Rosales, R. O., Gaudin, A., Alvarado Aliaga, C. A., Martinez, G. N., Tincopa Marca, L. R., et al. (2007). Capturing candidate drought tolerance traits in two native Andean potato clones by transcription profiling of field grown plants under water stress. Plant Physiol. Biochem. 45, 673–690. doi: 10.1016/J.PLAPHY.2007.06.003
Schonfeld, M. A., Johnson, R. C., Carver, B. F., and Mornhinweg, D. W. (1988). Water relations in winter wheat as drought resistance indicators. Crop Sci. 28, 526–531. doi: 10.2135/cropsci1988.0011183X002800030021x
Sniegowska-Swierk, K., Dubas, E., and Rapacz, M. (2015). Drought-induced changes in the actin cytoskeleton of barley (Hordeum vulgare L.) leaves. Acta Physiol. Plant. 37:73. doi: 10.1007/s11738-015-1820-0
Sniegowska-Swierk, K., Dubas, E., and Rapacz, M. (2016). Actin microfilaments are involved in the regulation of HVA1 transcript accumulation in drought-treated barley leaves. J. Plant Physiol. 193, 22–25. doi: 10.1016/j.jplph.2016.02.006
Soltys-Kalina, D., Plich, J., Strzelczyk-Zyta, D., Sliwka, J., and Marczewski, W. (2016). The effect of drought stress on the leaf relative water content and tuber yield of a half-sib family of 'Katahdin'-derived potato cultivars. Breed. Sci. 66, 328–331. doi: 10.1270/jsbbs.66.328
Strasser, R., Tsimilli-Michael, M., and Srivastava, A. (2004). “Analysis of the Chlorophyll a Fluorescence Transient,” in Chlorophyll a Fluorescence: A Signature of Photosynthesis, eds. G. C. Papageorgiou and Govindjee (Dordrecht: Springer Netherlands), 321–362. doi: 10.1007/978-1-4020-3218-9_12
Underwood, W. (2012). The plant cell wall: a dynamic barrier against pathogen invasion. Front. Plant Sci. 3:85. doi: 10.3389/fpls.2012.00085
Van Rensburg, L., Krüger, G. H. J., and Krüger, H. (1993). Proline accumulation as drought-tolerance selection criterion: its relationship to membrane integrity and chloroplast ultrastructure in Nicotiana Tabacum L. J. Plant Physiol. 141, 188–194. doi: 10.1016/S0176-1617(11)80758-3
Wang, Z. X., Chen, L., Ai, J., Qin, H. Y., Liu, Y. X., Xu, P. L., et al. (2012). Photosynthesis and activity of photosystem II in response to drought stress in Amur Grape (Vitis amurensis Rupr.). Photosynthetica 50, 189–196. doi: 10.1007/s11099-012-0023-9.
Weidenbach, D., Jansen, M., Franke, R. B., Hensel, G., Weissgerber, W., Ulferts, S., et al. (2014). Evolutionary conserved function of barley and Arabidopsis 3-KETOACYL-CoA SYNTHASES in providing wax signals for germination of powdery mildew fungi. Plant Physiol. 166, 1621–1633. doi: 10.1104/pp.114.246348
Wójcik-Jagła, M., Fiust, A., Kościelniak, J., and Rapacz, M. (2018). Association mapping of drought tolerance-related traits in barley to complement a traditional biparental QTL mapping study. Theor. Appl. Genet. 131, 167–181. doi: 10.1007/s00122-017-2994-1
Wójcik-Jagła, M., Rapacz, M., Barcik, W., and Janowiak, F. (2012). Differential regulation of barley (Hordeum distichon) HVA1 and SRG6 transcript accumulation during the induction of soil and leaf water deficit. Acta Physiol. Plant. 34, 2069–2078. doi: 10.1007/s11738-012-1004-0
Wójcik-Jagła, M., Rapacz, M., Tyrka, M., Kościelniak, J., Crissy, K., and Zmuda, K. (2013). Comparative QTL analysis of early short-time drought tolerance in Polish fodder and malting spring barleys. Theor. Appl. Genet. 126, 3021–3034. doi: 10.1007/s00122-013-2190-x
Wolfe, J., and Bryant, G. (1999). Freezing, drying, and/or vitrification of membrane– solute–water systems. Cryobiology 39, 103–129. doi: 10.1006/cryo.1999.2195
Yin, Z., Meng, F., Song, H., He, X., Yu, D., Xu, X., et al. (2010). Mapping quantitative trait loci associated with chlorophyll a. Planta 231, 875–885. doi: 10.1007/s00425-009-1094-0
Yu, B., and Li, W. (2014). Comparative profiling of membrane lipids during water stress in Thellungiella salsuginea and its relative Arabidopsis thaliana. Phytochemistry 108, 77–86. doi: 10.1016/J.PHYTOCHEM.2014.09.012
Keywords: barley, candidate genes, chlorophyll fluorescence, DArTseq, drought, genome-wide association analysis, OJIP
Citation: Rapacz M, Wójcik-Jagła M, Fiust A, Kalaji HM and Kościelniak J (2019) Genome-Wide Associations of Chlorophyll Fluorescence OJIP Transient Parameters Connected With Soil Drought Response in Barley. Front. Plant Sci. 10:78. doi: 10.3389/fpls.2019.00078
Received: 25 September 2018; Accepted: 17 January 2019;
Published: 11 February 2019.
Edited by:
Vicent Arbona, University of Jaume I, SpainReviewed by:
Ildikó Karsai, Centre for Agricultural Research (MTA), HungaryAntonio Ferrante, University of Milan, Italy
Copyright © 2019 Rapacz, Wójcik-Jagła, Fiust, Kalaji and Kościelniak. This is an open-access article distributed under the terms of the Creative Commons Attribution License (CC BY). The use, distribution or reproduction in other forums is permitted, provided the original author(s) and the copyright owner(s) are credited and that the original publication in this journal is cited, in accordance with accepted academic practice. No use, distribution or reproduction is permitted which does not comply with these terms.
*Correspondence: Marcin Rapacz, cnJyYXBhY3pAY3lmLWtyLmVkdS5wbA==