- 1Department of Plant Biology, University of Georgia, Athens, GA, United States
- 2Department of Biological Sciences, University at Buffalo, Buffalo, NY, United States
- 3School of Biological Sciences, Nanyang Technological University, Singapore, Singapore
- 4Department of Botany and Plant Sciences, University of California, Riverside, Riverside, CA, United States
- 5Smithsonian Tropical Research Institute, Panama City, Panama
- 6Agricultural Biotechnology Research Center, Academia Sinica, Taipei, Taiwan
Crassulacean acid metabolism (CAM) photosynthesis is a modification of the core C3 photosynthetic pathway that improves the ability of plants to assimilate carbon in water-limited environments. CAM plants fix CO2 mostly at night, when transpiration rates are low. All of the CAM pathway genes exist in ancestral C3 species, but the timing and magnitude of expression are greatly altered between C3 and CAM species. Understanding these regulatory changes is key to elucidating the mechanism by which CAM evolved from C3. Here, we use two closely related species in the Orchidaceae, Erycina pusilla (CAM) and Erycina crista-galli (C3), to conduct comparative transcriptomic analyses across multiple time points. Clustering of genes with expression variation across the diel cycle revealed some canonical CAM pathway genes similarly expressed in both species, regardless of photosynthetic pathway. However, gene network construction indicated that 149 gene families had significant differences in network connectivity and were further explored for these functional enrichments. Genes involved in light sensing and ABA signaling were some of the most differently connected genes between the C3 and CAM Erycina species, in agreement with the contrasting diel patterns of stomatal conductance in C3 and CAM plants. Our results suggest changes to transcriptional cascades are important for the transition from C3 to CAM photosynthesis in Erycina.
Introduction
Crassulacean acid metabolism (CAM) is a carbon concentrating mechanism that evolved multiple times in response to CO2 limitation caused by water stress. In C3 species, stomata remain open during the day to assimilate atmospheric CO2, but water limitation can force stomata to close, resulting in impaired CO2 fixation at the expense of growth. When water stress is prolonged, stomatal closure in C3 plants can become debilitating. CAM species circumvent prolonged stomatal closure by opening stomata at night and fix CO2 nocturnally, when evapotranspiration rates are on average lower. CO2 is temporarily stored as malic acid in the vacuoles until day time, when stomata close and malic acid is moved back into the cytosol for decarboxylation. The resulting increase of CO2 levels near ribulose-1,5-bisphosphate carboxylase/oxygenase (RuBisCO) results in highly efficient CO2 reduction via C3 photosynthesis CAM is associated with a number of anatomical, physiological and genetic change, including alterations to leaf anatomy (Nelson and Sage, 2008; Zambrano et al., 2014), stomatal opening at night, and tight regulation of metabolic genes within day/night cycles. Despite the complexity of these evolutionary novelties, CAM plants are found in a wide range of plant families, including eudicot species in the Euphorbiaceae (Horn et al., 2014) and Caryophyllales (Guralnick et al., 1984; Winter and Holtum, 2011; Moore et al., 2017) and monocot lineages in Agavoideae (Abraham et al., 2016; Heyduk et al., 2016), Orchidaceae (Silvera et al., 2009, 2010), and Bromeliaceae (Crayn et al., 2004).
The CAM pathway is well-described biochemically (Holtum et al., 2005) and contemporary genomics approaches are beginning to shed light on the genetic basis of CAM (Cushman et al., 2008; Dever et al., 2015; Abraham et al., 2016) (Figure 1). As CO2 enters the chloroplast-containing cells as night, it is initially converted to HCO3- facilitated by a carbonic anhydrase (CA). HCO3- is then fixed by phosphoenolpyruvate carboxylase (PEPC) using phosphoenolpyruvate (PEP) as the substrate. Carboxylation of PEP results in oxaloacetate (OAA), which is subsequently converted to malic acid by malate dehydrogenase (MDH). Malic acid is then moved into the vacuole for storage. The vacuolar transporter of malic acid is not known for certain, although previous studies have pointed to aluminum-activated malate transporters (ALMT) as a candidate (Kovermann et al., 2007; Yang et al., 2017). During the day, the malic acid is released from the vacuoles either via a passive process or through as-yet undescribed transporter. The malic acid is then decarboxylated to CO2 and PEP using two decarboxylation pathways: NAD and/or NADP malic enzymes together with pyruvate, phosphate dikinase (PPDK), or MDH and phosphoenolpyruvate carboxykinase (PEPCK).
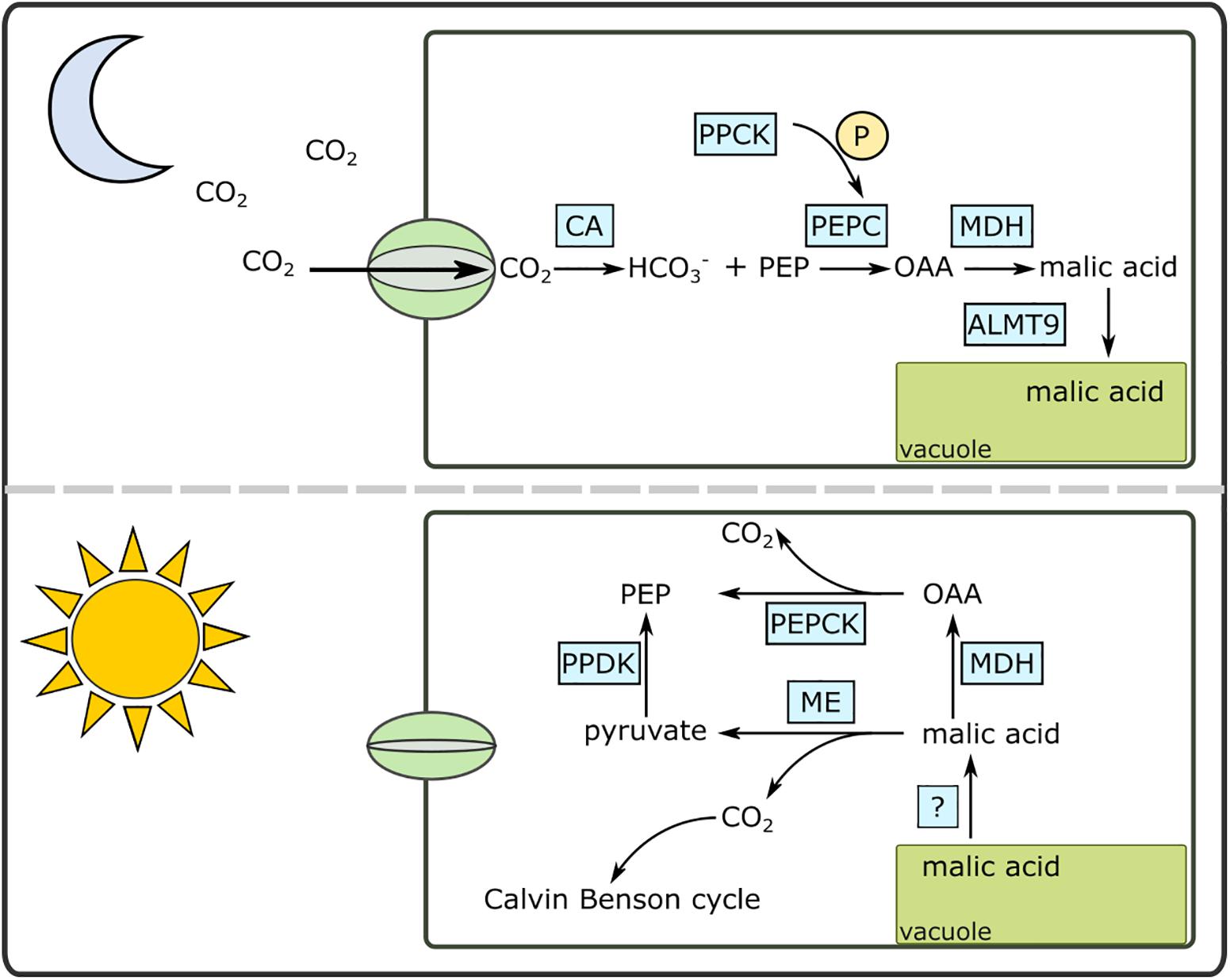
Figure 1. A simplified diagram of the Crassulacean acid metabolism (CAM) pathway under day and night conditions. ALMT9, aluminum activated malate transporter; CA, carbonic anhydrase; MDH, malate dehydrogenase; OAA, oxaloacetate; ME, malic enzyme (NAD or NADP); P, phosphate; PEPC, phosphoenolpyruvate carboxylase; PEPCK, PEP carboxykinase; PPCK, PEPC kinase; PPDK, pyruvate, phosphate dikinase.
While the roles of canonical CAM enzymes are considered novel in CAM species, they are all present in C3 ancestral species as well. As a result, the evolution of CAM likely involved alterations to gene copies, including changes to protein sequences and regulatory motifs. For example, PEPC in C3 species can play several roles depending on tissue type and developmental stage, including providing carbon backbones to the citric acid cycle and providing malate for cellular pH balance (Aubry et al., 2011; Winter et al., 2015), but its core function as a carboxylating enzyme remains unchanged in C3 and CAM species. Studies of molecular evolution of PEPC in CAM species have largely determined that the PEPC copy recruited for CAM has significantly higher nocturnal expression in CAM taxa over C3 (Lepiniec et al., 1994; Gehrig et al., 2001; Silvera et al., 2014; Ming et al., 2015; Zhang et al., 2016). In some cases, these CAM-specific PEPC gene copies have been shown to share sequence similarity across closely related but independently derived CAM taxa (Christin et al., 2014; Silvera et al., 2014). Additionally, genomic screens across many canonical CAM gene promoters have revealed an enrichment of circadian clock motifs (Ming et al., 2015), implicating alterations to transcription factor binding sites during CAM evolution. Because of the strong influence of the internal circadian clock on CAM (Hartwell, 2005), we might expect that genes involved in the CAM regulatory pathway should be controlled by a co-expressed circadian master regulator (Borland et al., 1999; Nimmo, 2000; Taybi et al., 2000).
Elucidation of regulatory changes requires comparative analysis between closely related C3 and CAM species, and this can be accomplished through RNA-Seq analyses. One of the largest plant families with multiple origins of CAM is the Orchidaceae; known for floral diversity and inhabiting a broad range of habitats, the evolution of CAM in predominantly epiphytic lineages may have also contributed to orchid diversity (Silvera et al., 2009). Epiphytic species constitute more than 70% of the Orchidaceae (Gravendeel et al., 2004; Chase et al., 2015), and many exhibit different degrees of CAM (Silvera et al., 2005). A large proportion of these exhibit weak CAM, whereby most CO2 is fixed by the C3 pathway. Weakly expressed CAM may represent an evolutionary end point, or may be an important intermediate step on the evolutionary path between constitutive C3 and constitutive CAM. Because many genera within the Orchidaceae include both C3 and weak/strong CAM species, the orchids are an attractive family to study the evolution of CAM photosynthesis. The subtribe Oncidiinae is one of the most diverse subtribes within Orchidaceae and it is part of a large epiphytic subfamily (Epidendroideae) in which CAM may have facilitated the expansion into the epiphytic habitat (Silvera et al., 2009). Despite the prevalence of CAM within the subtribe, the genus Erycina is particularly interesting because it has both CAM and C3 species. Erycina pusilla is a fast-growing CAM species with transformation capability and has the potential to be a model species for studying CAM photosynthesis in monocots (Lee et al., 2015). Comparative investigations of E. pusilla and its C3 relative, E. crista-galli, can therefore offer valuable insight into studying the evolution and regulation of CAM photosynthesis in the Orchidaceae. Through comparative, time-course RNA-Seq analysis of E. pusilla and E. crista-galli, we aim to understand (1) the changes in expression of core CAM genes between C3 and CAM Erycina species and (2) which regulatory changes are required for the evolution of CAM.
Materials and Methods
Plant Growth and RNA-Seq Tissue Collection
Erycina pusilla (L.) N. H. Williams and M. W. Chase (CAM) seedlings were cultivated on solid PSYP medium comprising 2 g/L Hyponex No. 1, 2 g/L tryptone, 20 g/L sucrose, 0.1 g/L citric acid, and 1 g/L active charcoal in flasks. The pH of the medium is adjusted to 5.4 before autoclaving and gelling with 3 g/L Phytagel. Plants were grown in 12-h day and 12-h night conditions over three independent dates in a growth chamber at the University at Buffalo, with temperatures set to 22–25°C and lights on at 6 a.m. for a 12-h photoperiod. Light intensity was between 95 and 110 μmol m-2 s-1. Leaf samples for RNA-sequencing were collected every 4 h directly from plants grown on sealed flasks for the first two experiments (January and February 2015, Set 1 and Set 2, respectively) and every 2 h from the final experiment (October 2015, Set 3), where both medium-sized and large plants were collected. Erycina pusilla is a miniature orchid species, limiting tissue availability, and therefore each genet was destructively sampled once at a randomly assigned time point. Leaf samples were flash frozen in liquid N2 and stored at -80°C.
Because Erycina species are considered miniatures and are therefore relatively small for destructive leaf sampling, we use individual genets as biological replicates at each time point.
Erycina crista-galli (Rchb.f.) N. H. Williams and M. W. Chase (C3) plants were wild-collected from Peña Blanca, District of Capira, Republic of Panama at 858 m above sea level, then grown and propagated in a commercial orchid greenhouse in Bajo Bonito, District of Capira, Republic of Panama. Plants were fertilized once a week alternatively with a 20–20–20 or 16–32–16 N–P–K fertilizer. Similarly sized and aged plants were moved into an environmental growth chamber at the Smithsonian Tropical Research Institute laboratories (Panama City, Panama) in April 2016, where they were allowed to acclimate for 48 h to the following conditions: 12-h light/dark cycle (lights on 6 a.m.), 25°C/22°C day/night temperatures, 60% humidity, and a light intensity of 30 μmol m-2 s-1, which is similar to the light intensity this species would experience naturally. Biological replicates (consisting of entire shoots without root tissue) were sampled every 4 h over a 24-h period, starting at ZT0 (lights on, 6 a.m.) with four replicates per time point. Tissue was flash frozen in liquid nitrogen and stored as described above for E. pusilla.
RNA was isolated from leaf tissue of both Erycina species using the RNeasy Plant Mini Kit (Qiagen). RNA samples were subsequently quantified via Nanodrop and checked for integrity with a Bioanalyzer v2100. RNA libraries were constructed using the Kapa mRNA stranded kit with a combinatorial barcoding scheme (Glenn et al., 2016). Libraries were sequenced on an Illumina NextSeq500 with PE75 reads, pooling 30–32 samples per run. A summary of the data can be found in Supplementary Table 1.
Gas Exchange
Individual shoots (leaves emerging from a common base) of E. pusilla and E. crista-galli species were individually sealed within a CQP 130 porometer gas-exchange cuvette (Walz, Effeltrich, Germany), located inside an environmental chamber (Environmental Growth Chambers, OH, United States) operating on 12 h light (6 a.m. to 6 p.m.) at 28°C, and 12 h dark (6 p.m. to 6 a.m.) cycle at 22°C. Light intensity inside the chamber was 230 μmol m-2 s-1. Plants were watered three to four times daily and humidity inside the growth chamber was maintained at near 60%. Continuous net CO2 exchange was measured for each plant for up to 8 day/night cycles with data points obtained every 4 min. For E. pusilla, water was withheld from one of the three plants measured (drought stress) between the fifth and the eighth day; regarding E. crista-galli, water was withheld from the third to the fifth day for one plant of the three measured. Data is presented for all three replicates of each of the two Erycina species in Supplementary Figure 1.
Titratable Acidity
Leaf samples from E. pusilla were collected for leaf acid titrations from the same plants used for RNA sequencing, grown on media as described above. Leaves from mature plants were collected every 4 h, flash frozen in liquid N2, weighed, and boiled in 20% ethanol and deionized water. Titratable acidity was measured as the amount of 0.002M NaOH required to neutralize the extract to a pH of 7. Because leaf tissue was limited for E. crista-galli plants, we conducted titrations on three plants that were not sampled for RNAseq to confirm their status as C3. Samples were collected in greenhouse conditions at dawn and dusk with three replicates at each time. Titratable acidity was measured as for E. pusilla but using 0.001M KOH.
Transcriptome Assembly
An initial de novo transcriptome was assembled from Sets 1 and 2 for E. pusilla sequences and from all samples sequenced for E. crista-galli using Trinity v2.0.6 (Haas et al., 2013). Reads were cleaned using Trimmomatic v0.36 (Bolger et al., 2014) and assemblies were made on in silico normalized reads. An initial evaluation of read mapping results from E. pusilla Sets 1, 2, and 3 showed a large degree of variation among replicates; to reduce this variation, we excluded the large plants from Set 3 from further analysis. These data were further reduced to include only four replicates per time points for a total of 24 samples. Replicates were chosen randomly. All reads for E. crista-galli were included in the analysis. Read mapping and abundance estimation for transcripts was conducted separately in each species using RSEM v1.3.0 (Li and Dewey, 2011) and Kallisto v0.42.5 (Bray et al., 2016). Transcripts with a transcripts per kilobase million mapped (TPM) < 2 were removed and the reads were re-mapped to the filtered assemblies.
Ortholog Circumscription and Isoform Filtering
To determine gene family circumscription and annotation, all transcripts were sorted into 14 orthogroup gene families from the genomes of the following: Amborella trichopoda, Ananas comosus, Arabidopsis thaliana, Asparagus officinalis, Brachypodium distachyon, Carica papaya, Dendrobium catenatum, Elaeis guineensis, Musa acuminata, Oryza sativa, Phalaenopsis equestris, Solanum lycopersicum, Sorghum bicolor, Spirodela polyrhiza, Vitis vinifera, and Zostera marina. Assembled transcripts were first used to query the genome database using blastx and sorted to gene families (orthogroups) based on best BLAST hit. The gene families were annotated by Arabidopsis members, using TAIR 101 classifications. Transcripts were retained only if they (1) were no longer than the longest homolog identified in the reference genomes used for gene family (orthogroup) circumscription, and (2) had a length no less than 50% of the minimum sequence length based on sequenced genome members of that gene family.
Trinity produces both gene components and subsidiary isoforms, which may represent true alternative splice isoforms or allelic or paralogous sequence variants. To mitigate dilution of read mappings to multiple isoforms, we instead used gene components (hereafter referred to as transcripts) for all further analyses (including gene level read mapping from RSEM). For gene tree estimation we took the longest isoform per component per orthogroup, using our minimum/maximum orthogroup filtered data set. Scripts for orthogroup sorting and filtering can be found at www.github.com/kheyduk/Erycina.
Time-Dependent Clustering
To incorporate time into our clustering analysis, we used R software package maSigPro v1.46.0 (Conesa et al., 2006; Nueda et al., 2014), which analyzes expression data for patterns across time by fitting each gene’s expression pattern to a polynomial using stepwise regression. Cross-normalized read counts and a negative binomial distribution for the generalized linear models were used. For each transcript, maSigPro estimated up to a fourth degree polynomial and tested the fit via ANOVA. Transcripts that had significantly time-structured expression (Benjamini and Hochberg adjusted p < 0.05) were retained while all others were removed from further analysis. Additionally, any genes considered overly influential based on DFBETAS diagnostic (how much an observation affects the estimate of a regression coefficient) (Belsley et al., 1980) were also removed. In total, 1,515 transcripts from E. pusilla and 505 transcripts from E. crista-galli were removed as influential genes.
The remaining transcripts that did show time-dependent expression (n = 7,066 in E. pusilla and n = 7,127 in E. crista-galli) were clustered by fuzzy clustering based on similarity in expression profiles. An optimal fuzzifier m, a parameter that determines how much clusters can overlap, was calculated in the Mfuzz package (Kumar and Futschik, 2007) of R for each species (m = 1.09 for both E. pusilla and E. crista-galli). The number of groups k for each species was determined by choosing a value which minimizes the within-group variance (Supplementary Figure 2); a k of 6 was used for both E. pusilla and E. crista-galli. Z-scores of normalized counts were calculated for each gene in each cluster, as well as a median cluster expression, for each species separately.
Gene Trees and Expression
Gene trees were estimated for PEPC and its kinase (PPCK) by first aligning nucleotide sequences from Erycina transcripts [longest open reading frame from Transdecoder v4.0.0 (Haas et al., 2013)] and their associated gene family members from the sequenced genomes using the coding sequence aligner in MUSCLE v3.8.425 (Edgar, 2004) within Aliview v1.23 (Larsson, 2014) then estimating trees using RAxML v8.2.11 (Stamatakis, 2006). Gene expression for genes of interest was plotted based on averaged transcripts per million mapped (TPM) for each replicate, scaled to the highest average TPM value.
Network Analysis
To identify regulatory candidates possibly involved in CAM and examine the relationships between genes within clusters, we used the ARACNe-AP v1.4 (Lachmann et al., 2016) algorithm to create networks of co-expressed transcripts from both species separately. Briefly, the algorithm randomly samples gene pairs and uses an adaptive partitioning approach to infer a pairwise mutual information (MI) statistic, or measure of statistical dependence, between them. This process is repeated iteratively for a specified number of bootstraps, while at each step removing indirect interactions. A final network is built based on the consensus of all bootstrap runs. Although ARACNe provides an option to specify transcription factors to generate a directed network by only considering interactions with a transcription factor source, we chose to generate an undirected gene co-expression network of genes that were significantly time-structured based on our maSigPro analysis, using 100 bootstrap replicates in ARACNe.
We imported network data into Cytoscape (Shannon et al., 2003) to generate visualizations and calculate network statistics. Nodes were color coded by their cluster membership and scaled to represent number of connections. Network statistics were exported and further analyzed at the orthogroup level. We calculated which orthogroups had the largest average difference in connectivity between the two species. We first calculated the average connectivity (number of directed edges, output from Cytoscape Network Analysis) for each orthogroup per species, then normalized these via Z-scores and subtracted the Z-score of the orthogroup in E. pusilla from that in E. crista-galli. We defined outliers – those orthogroups with the largest difference between species in connectivity – as orthogroups with Z-score differences greater than 1.5 times the interquartile range.
The outlier orthogroups with large changes to connectivity between species (Supplementary Table 3) were explored for genes of interest. The largest difference in connectivity was found in a E3 ubiquitin ligase gene family shown to play a role in ABA signaling, with the Arabidopsis homolog known as ring finger of seed longevity 1 (RSL1). To explore differences in network connections of RSL1 between the two species, we employed the diffusion algorithm (Carlin et al., 2017) in Cytoscape which finds strongly interactive nodes to a target of interest. For both species, we found the diffusion network for the RSL1 gene. Only a single gene copy of RSL1 was time-structured in E. crista-galli, but E. pusilla had two copies found in the ARACNe network. One gene copy had only a single connection to any other gene in the network and was not analyzed further. The other copy in E. pusilla, which had 72 directed connections, was used as the center of the diffusion network. Diffusion networks were compared for orthogroup content between species using a hypergeometric test. Gene Ontology (GO) terms were compared for the two RSL1 subnetworks and checked for enrichment using hypergeometric test (using all GO terms found in either ARACNe network as the universe), correcting for multiple testing with Benjamini and Hochberg significance correction.
Results
Gas Exchange Patterns and Titratable Acidity
Gas exchange data collected continuously showed net nighttime CO2 uptake in CAM E. pusilla under both well-watered conditions and while drought stressed (Figure 2A). C3 Erycina crista-galli displayed net CO2 uptake during the light period only. There was no net uptake of CO2 at night. Nonetheless, under drought stress, a slight decrease in respiratory loss of CO2 at night may indicate low levels of CAM cycling. Titration data collected from the same plants and at the time of RNA-sampling in E. pusilla confirms CAM function in the plants used for gene expression analysis, with a significant increase in leaf titratable acids occurring toward the end of the dark period (Figure 2A, 6 a.m.), and a reduction in total acids during the day period. Although the C3 E. crista-galli had higher overall levels of leaf acids, there was no significant diurnal fluctuation (Figure 2B).
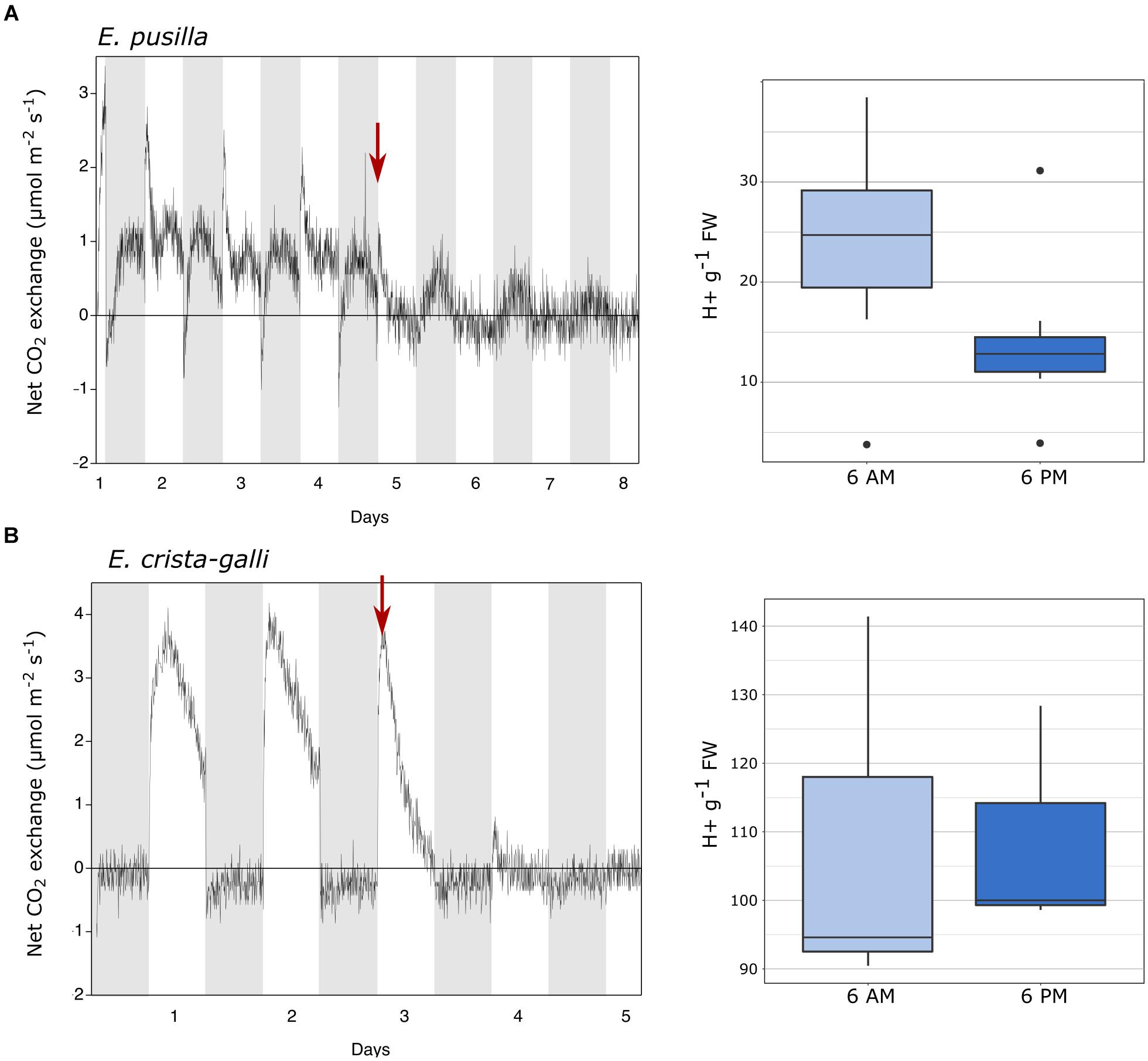
Figure 2. Gas exchange and titratable acidity for (A) Erycina pusilla and (B) Erycina crista-galli. Gas exchange is shown for a single plant; replicate plant gas exchange plots can be found in Supplementary Figure 1. Drought induction is indicated with a red arrow. Titrations are shown for dawn and dusk; full titratable acidity values are in Supplementary Table 2.
Clustering of Genes With Time-Structured Expression Profiles
After filtering by minimum/maximum length each transcript’s orthogroup, 23,596 and 26,437 genes were retained in E. pusilla and E. crista-galli, respectively. Both species had a similar number of genes that were significantly time-structured (∼7,000) according to maSigPro. Each species had best fit to k = 6 clusters, with three clusters showing nighttime biased expression and three with daytime bias (Figure 3). Expression of PEPC, the initial carboxylating enzyme in the CO2 fixation pathway at night, increased in expression just before the onset of darkness in the CAM species E. pusilla (Figure 4A). In contrast, there was a low, but significant, time-structured expression pattern of PEPC in the C3 species E. crista-galli (Figure 4B). The dedicated kinase, PPCK, which phosphorylates PEPC and allows it to function in the presence of malate, likewise showed a strong nocturnal increase in expression in the CAM species, with similar levels of expression in the C3 species (Figure 4B).
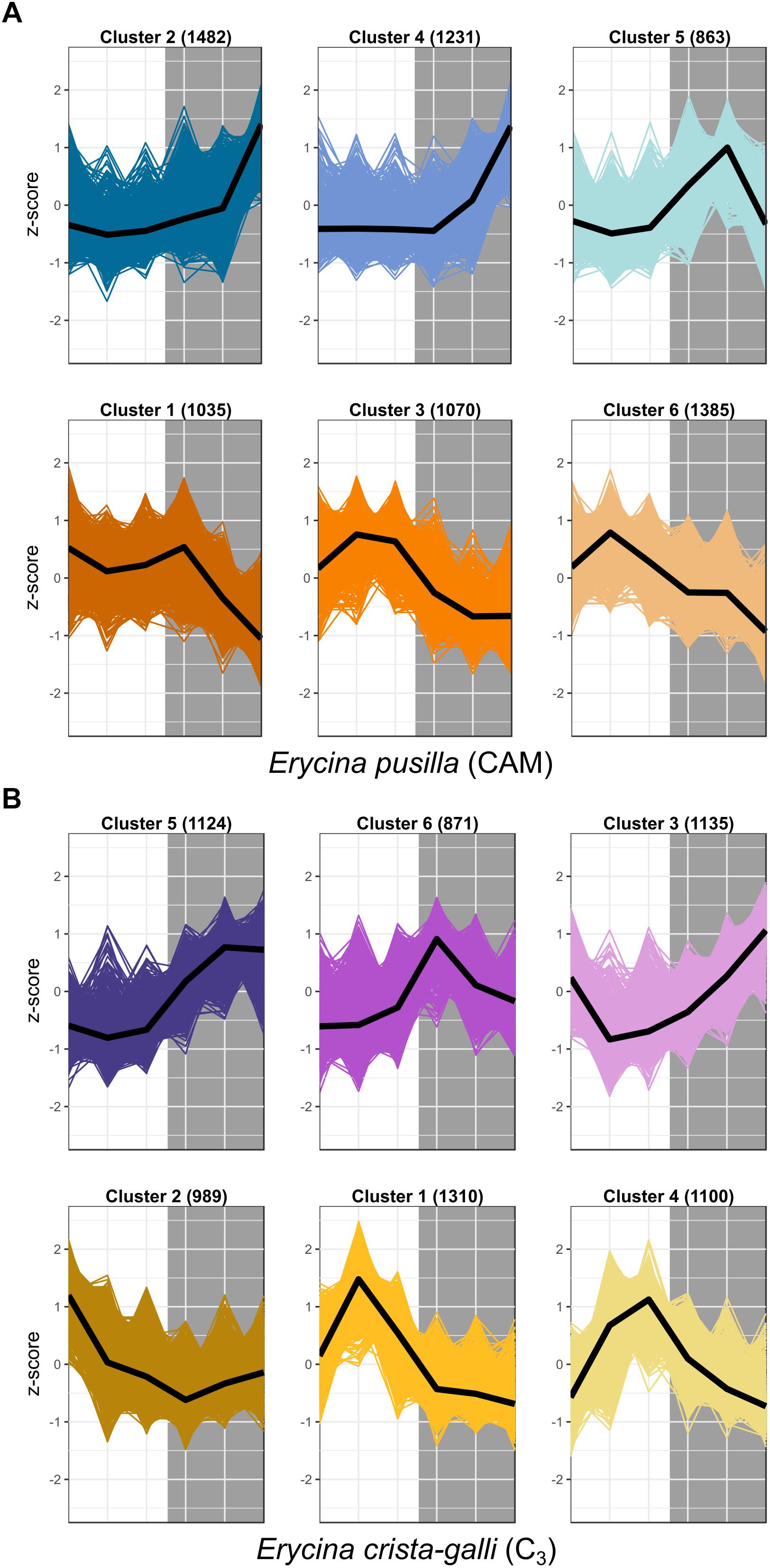
Figure 3. Expression Z-scores for each gene in each cluster for both (A) E. pusilla and (B) E. crista-galli, with median expression shown in the black line. Clusters represent co-expressed genes within each species’ time-structured transcripts. Cool colors (blue and purple) are clusters with nighttime biased expression, whereas warmer colors (orange and yellow) are clusters whose transcripts increase in expression during the day.
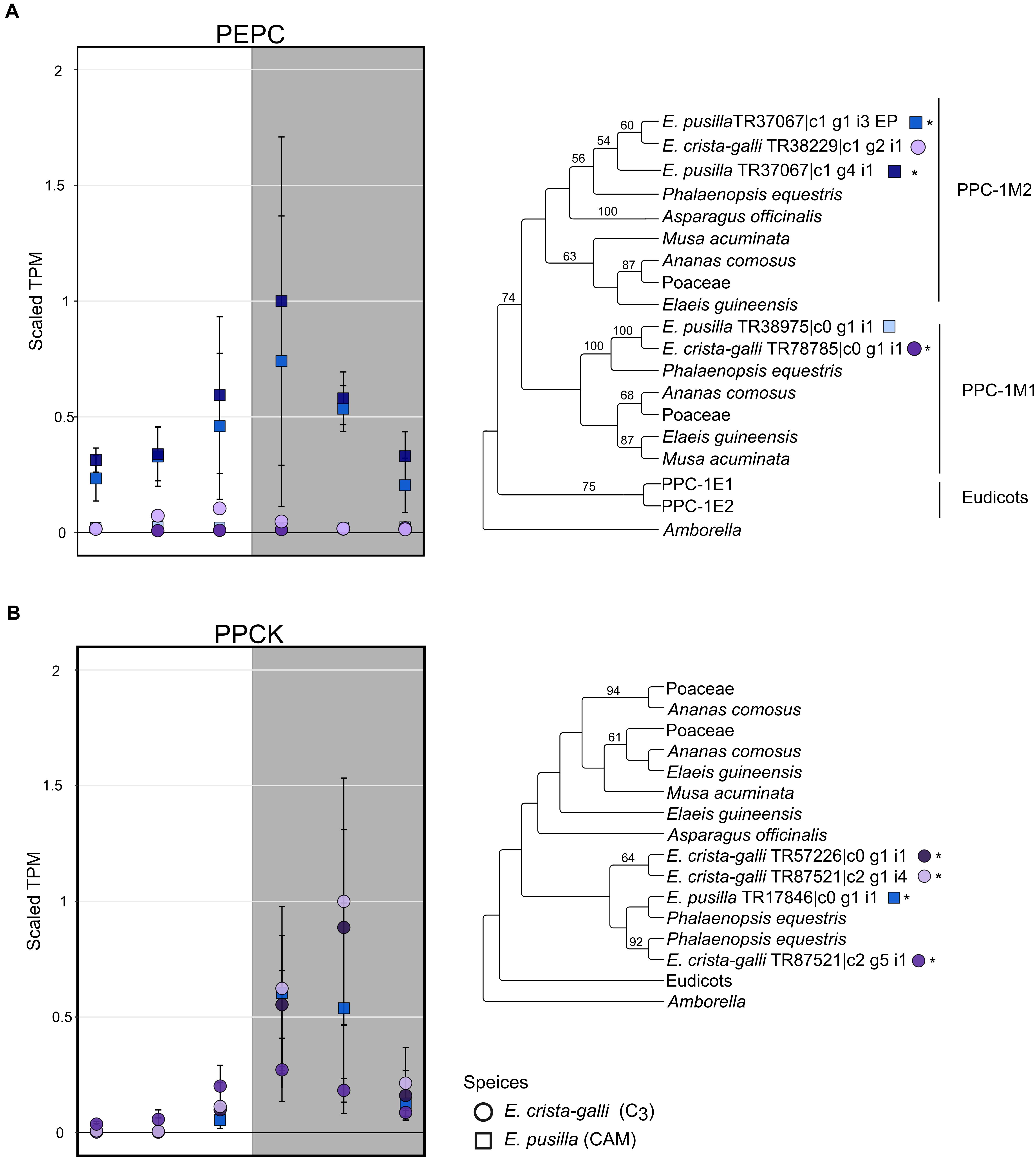
Figure 4. Expression and gene tree of (A) phosphoenolpyruvate carboxylase (PEPC) and (B) PEPC kinsase (PPCK) in E. pusilla (squares, blue tones) and E. crista-galli (circles, purple tones). Different shades of color represent different assembled gene copies, and only transcripts that had a predicted open reading frame are shown in both expression plots and gene trees; asterisks next to transcripts in the gene tree indicate those transcripts had significant variation in expression across time. Average TPM and standard deviation, scaled to the maximum mean TPM value across all copies and species, are plotted for all six time points, with the gray background indicating nighttime samples.
Network Comparisons
The network for C3 E. crista-galli had 119,338 directed connections between 4,828 nodes, whereas the CAM E. pusilla network was strikingly less connected, with only 76,071 connections between 4,591 nodes. Although the number of genes in each network was similar, overall connectivity of E. pusilla is easily seen in both the fewer number of connections as well as the mean number of connections (34.3 in E. pusilla vs. 50.3 in E. crista-galli). As expected, genes from the same co-expressed cluster (Figure 3) were grouped within the larger ARACNe network for each species (Figures 5A,C).
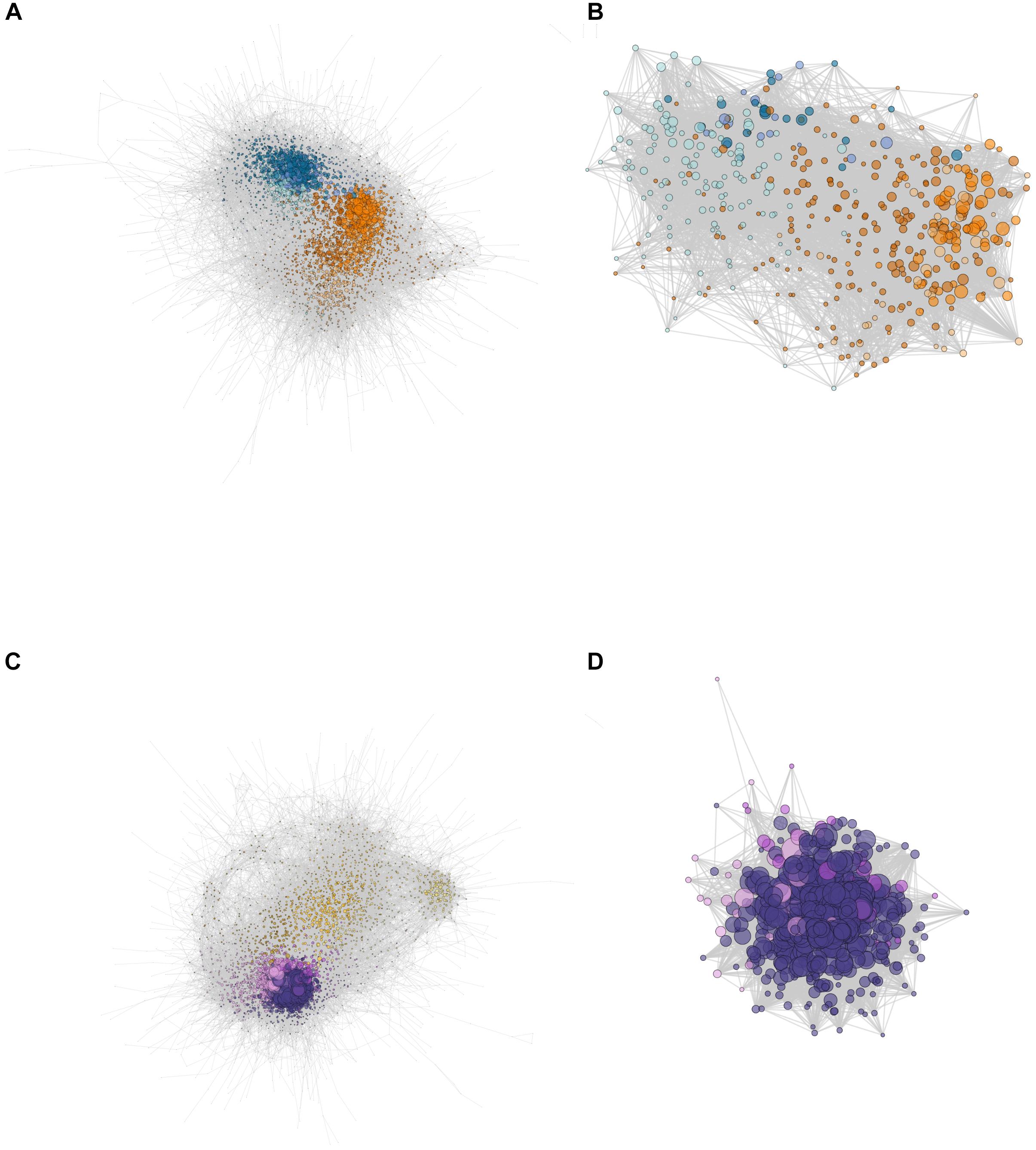
Figure 5. Network constructions of (A) E. pusilla and (C) E. crista-galli based on ARACNe network analysis, and the ring finger seed longevity (RSL1) subnetworks for (B) E. pusilla and (D) E. crista-galli calculated via the diffusion algorithm in Cytoscape. Colors correspond to Figure 3 cluster colors – cooler colors (blues in A and B and purples in C and D) are clusters that have an increase in expression at night and warmer colors (oranges in A and B and yellows in C and D) are clusters that have increases in expression during the day. Dots represent genes, scaled by the number of edges (maximum number of directed edges = 435).
Comparison of network connectivity of the time-structured genes found 149 outlier transcripts that had large species differences in the number of connected directed edges (Supplementary Figure 3); these were largely skewed toward increased connectivity in E. crista-galli (n = 90). Annotations of these outliers revealed a number of genes involved in stomatal opening/closing and ABA signaling. GO term enrichment indicates that outliers that skew toward more connectivity in C3 E. crista-galli were enriched for vacuolar and tonoplast membrane proteins and potassium and calcium transport. Genes that were more connected in CAM E. pusilla were enriched for genes involved in aldehyde dehydrogenase activity, among other functions (Supplementary Table 4).
A E3 ubiquitin ligase also known as ring finger of seed longevity 1 (RSL1) had the greatest difference in connectivity between the two species. RSL1 has been shown to be a negative regulator of ABA signaling (Bueso et al., 2014) and was chosen as a center node for comparison between the two species. The diffusion algorithm used to create subnetworks defaults to producing a subnetwork that is 10% of the total nodes in the larger network; as a result, both species subnetworks were roughly the same size, containing about 400 genes. However, the connectivity of those subnetworks differed greatly (Figures 5B,D); the C3 E. crista-galli RSL1 subnetwork contained 30,392 connections, whereas the CAM E. pusilla network had only 9,244. The subnetworks differed in their gene content as well (Supplementary Table 5). There contained 429 and 427 orthogroups in E. pusilla and E. crista-galli, respectively, but only 57 orthogroups were shared between the two [this was not significantly under-enriched via a hypergeometric test (p = 1)]. While all of the genes in the E. crista-galli RSL1 subnetwork were in night-biased expression clusters (485), E. pusilla had more subnetwork genes in day-biased clusters (298) than in night biased ones (162). GO term enrichment indicates both subnetworks are enriched for chloroplast, chloroplast stroma, and photosynthesis (Supplementary Table 6).
While both subnetworks were centered on RSL1 and generally were enriched for similar types of genes involved in chloroplast functions and photosynthesis, there were substantial differences in gene content between the networks. The focal gene RSL1 acts as a master negative regulator of ABA signaling pathway by targeting pyrabactin resistance 1 (PYR1) and PYR-like (PYL) ABA receptors for degradation. More generally its role in protein ubiquitination is relatively unknown. RSL1 was the third most connected node in the C3 E. crista-galli subnetwork (419 directed connections; most connected node had 423 connections). In CAM E. pusilla, RSL1 was 173rd out of 460 genes in connectivity. E. pusilla had a number of ABA responsive genes in its RSL1 subnetwork that E. crista-galli did not, including protein phosphatase 2C (PP2C), a gene encoding a member of the Snf1-related kinase family, plus a homolog of ABA Overly Sensitive 5. Recent work has shown that ABA responses in stomata including PYR/PYL and downstream genes are responsible for constitutive stomatal aperture, as well as stomatal responses to drought stress (Gonzalez-Guzman et al., 2012).
Erycina crista-galli, on the other hand, had a number of light sensing and circadian clock genes that CAM E. pusilla did not have in the RSL1 subnetwork, including lov kelch protein 2 (LKP2/ZTL gene family), time for coffee (TIC), phytochrome B/D (PHYB), and protein phosphatase 2A (PP2A) (Figure 6). LKP2/ZTL and PHYB are both involved in light sensing (blue and red/far-red, respectively). Specifically, LKP2/ZTL genes are thought to regulate light induced protein degradation via their function as E3 ligases (Demarsy and Fankhauser, 2009; Ito et al., 2012), and ztl mutants in Arabidopsis show a prolonged clock period under constant light due to the lack of degradation of clock components via ZTL (Somers et al., 2000; Más et al., 2003). TIC has been found to be responsible for the amplitude of the circadian clock but is not thought to be directly involved in light signaling (Hall et al., 2003). TIC is also implicated in daytime transcriptional induction via its association with the central circadian oscillator late elongated hypocotyl (LHY) (Ding et al., 2007). Finally, PP2A is a member of a large family of plant phosphoprotein phosphatases (PPP) with several cellular roles (Uhrig et al., 2013). PP2A specifically has been implicated in brassinosteroid signaling, light signaling via dephosphorylation of phototropin 2 (Tseng and Briggs, 2010), flowering time control (Kim et al., 2002), as well as the induction of CAM under certain abiotic stresses (Cushman and Bohnert, 1999).
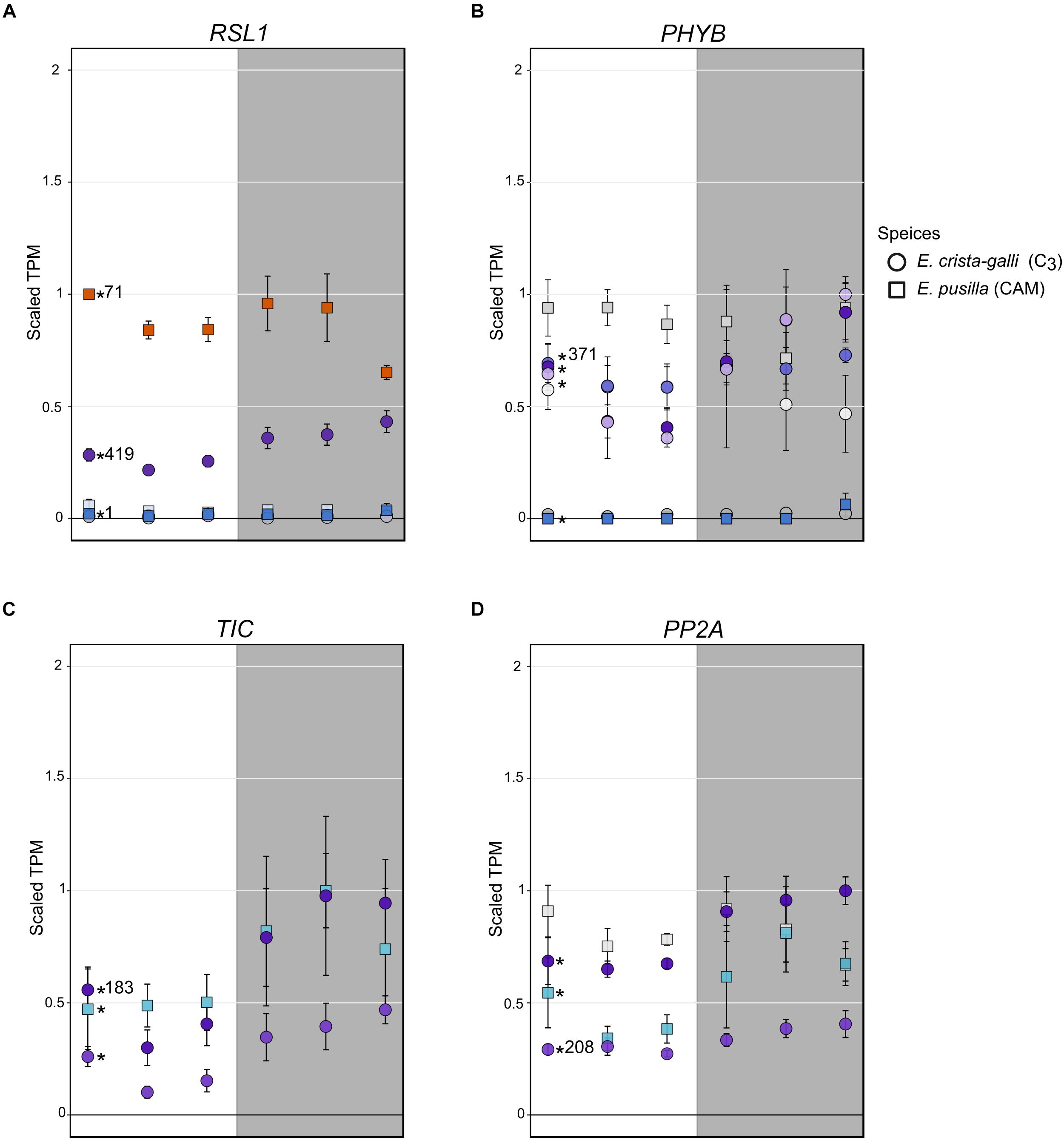
Figure 6. Expression of (A) ring finger of seed longevity 1 (RSL1), (B) phytochrome B/D (PHYB), (C) time for coffee (TIC), and (D) protein phosphatase 2A in E. pusilla (squares) and E. crista-galli (circles). Points are colored by which category of cluster they belong to: blues and purples are genes with increases in expression in the dark in E. pusilla and E. crista-galli, respectively, while oranges and yellows are genes with increases in daytime expression in E. pusilla and E. crista-galli, respectively (see Figure 3). Time-structured genes are marked with an asterisk, while those that were not significantly time-structured are shown in gray. Number of directed edges per gene is shown if they belonged to the RSL1 subnetwork. Average TPM and standard deviation, scaled to the maximum mean TPM value across all copies and species, are plotted for all six time points, with the gray background indicating nighttime samples.
Although the C3 E. crista-galli RSL1 subnetwork contained circadian and light sensing transcripts that were absent in the E. pusilla RSL1 subnetwork, these transcripts largely did not have different expression patterns between the two species with the exception of PHYB (Figure 6B). The contrasting gene content of the RSL1 subnetwork suggests light sensing and circadian regulatory cascades comprise large differences between C3 and CAM species, rather than levels of gene expression, which were quite similar.
Discussion
Shared Gene Expression Patterns
While traditionally it was thought that canonical CAM genes should have large differences in timing and magnitude of expression between C3 and CAM species, recent work has highlighted that between closely related C3 and CAM species, that may not always be the case (Heyduk et al., 2018b). Previous transcriptomic work in Orchidaceae showed large differences in nocturnal gene expression of core CAM genes between CAM and distantly related C3 orchids (Zhang et al., 2016). Here, however, comparison of closely related C3 and CAM taxa shows that both species have time-structured expression of certain CAM genes. For example, PEPC has time-structured expression in both the C3 and CAM species albeit at very different expression levels. While this alone says little about PEPC’s function in both species, the high nocturnal expression of PEPC’s dedicated kinase, PPCK, in both E. pusilla and E. crista-galli suggests that PEPC is being phosphorylated in both species and therefore has the need to function in the presence of malate.
It is worth noting that all C3 species have the genes involved in the CAM cycle. Many, including PEPC, function in anaplerotic reactions of the TCA cycle. PEPC has also been shown to have a role in malate production for osmotic regulation of stomatal aperture and in CO2 fixation in guard cells of tobacco (Asai et al., 2000; Daloso et al., 2015). Because most RNA-seq or gene expression studies to date in C3 species sample during the day, understanding how common nocturnal PEPC expression is across flowering plants will require more nighttime gene expression studies in C3 species, especially in lineages closely related to CAM species. While nearly all the genes in the CAM CO2 fixation pathway have known functions in C3 species, PPCK is a notable exception. Phosphorylation of PEPC by PPCK in CAM and C4 species is well-described (Nimmo et al., 1986; Jiao and Chollet, 1989; Taybi et al., 2000); malate and other organic acids act as negative regulators of PEPC, but phosphorylation of PEPC renders it immune to these negative effects. Thus, phosphorylated PEPC via PPCK is required for high levels of CAM and C4 malic acid production. In C3 species, however, there is no clear need for heavily phosphorylated PEPC, especially at night, making the expression of PEPC and PPCK in C3 E. crista-galli intriguing (though see Sullivan et al., 2004 and Fukayama et al., 2006 for work on nocturnal PPCK expression in soybean and rice, respectively).
Due to vastly different growth requirements for the two species compared in this study, physiology and subsequent mRNA levels were measured from plants grown in quite dissimilar condition. Erycina pusilla does well in flasks with media, whereas E. crista-galli requires lower light levels and more natural conditions. There is a relatively low level of circadian turnover of malic acid in E. pusilla, which either is its natural flux of nocturnal acids or is a result of growing on media with carbohydrates added. PEPC expression is significantly higher in the CAM E. pusilla, suggesting it is still relying on a CAM cycle while on media. Nevertheless, the differences in gene expression and network connectivity should be interpreted with care; future studies in Erycina and Orchidaceae more broadly will determine if these patterns are more generalizable.
Alterations in Regulatory Pathways Between C3 and CAM Orchids
Network analysis of co-expressed genes and subsequent comparisons between species can give insights not only into the changes in expression, but also the degree to which a given gene changes in connectivity between species. Extensive connectivity for a gene has long thought to be a signal of a “hub” or master regulatory gene – one that cannot experience large changes in timing or magnitude of expression without significant perturbations to the entire network. A major assumption of co-expression networks is that they rely on mRNA as an accurate predictor of downstream processes; while this is not always the case, recent work showed that although mRNA and protein networks differed in their gene content, they overlapped in gene ontologies and were predictive of pathway regulation in maize (Walley et al., 2016). Additionally, it has been shown that a correlation exists between connectivity of a gene within a network and the evolutionary conservation of the gene’s sequence across a number of flowering plant species (Masalia et al., 2017). It is therefore somewhat surprising to observe that as many as 149 gene families have large changes in connectivity between the two closely related Erycina species.
The 149 outlier gene families in Erycina were enriched for functions in protein degradation via phosphorylation and ubiquitination. While typically differences in phenotype are considered the result of changes in the abundance of gene products, our data highlight the importance of considering protein degradation as well. The differences in connectivity of protein degradation pathway genes were unbiased between species – in other words, genes involved in protein degradation have increased connectivity in both species. More interestingly, genes that had increased connectivity in C3 E. crista-galli were enriched for GO terms involved in potassium and chloride channels and membrane proteins associated with chloroplasts and vacuoles. Greater connectivity of such genes in the C3 species indicates an increased reliance on ion and metabolite fluxes. In stomatal guard cells, which make up a smaller portion of the whole-leaf transcriptome, these fluxes directly affect stomatal aperture and may play a role in alternative regulation of stomatal opening in C3 and CAM species.
Regulatory Changes in ABA, Light, and Clock Perception
Stomatal opening in CAM species has been vastly understudied, despite the opportunities it presents for understanding a fundamental biological process. In C3 species, stomatal opening is thought to be regulated by blue and red light inputs, whereas stomatal closing is driven by efflux of potassium cations. It remains largely unknown how stomata sense darkness, but experimental data have suggested a large role of CO2 concentrations on stomatal aperture (Cockburn et al., 1979). Draw down of CO2 concentrations at night in the intercellular airspace would result in stomatal opening, whereas high concentrations of CO2 from decarboxylation during the day may promote stomatal closure. CO2 concentrations undoubtedly play some role in the inverted stomatal aperture of CAM species, but more contemporary genomic work has implicated additional levels of regulation in CAM stomata (Cushman and Bohnert, 1997; Abraham et al., 2016; Wai et al., 2017). For example, ABA may be one signaling molecule for nocturnal stomatal closure (Desikan et al., 2004; Gonzalez-Guzman et al., 2012; Merilo et al., 2013). Gene expression results coupled with network analysis in Erycina indicate that both ABA signaling and light sensing are likely to be altered. Indeed, the gene with the largest difference in connectivity between species is RSL1, which encodes a E3 ubiquitin ligase known to function in stomatal response to ABA. Expression of RSL1 is higher in CAM E. pusilla and is clustered with day-biased genes (Figure 6A), whereas in C3 E. crista-galli expression is about half that in E. pusilla and slightly increases in expression during the dark period. These expression patterns are consistent with stomatal regulation between C3 and CAM species. In E. crista-galli nighttime ABA may play a larger role in drought-induced or nighttime stomatal closure than in the CAM E. pusilla, where the nocturnal stomatal closure driven by ABA signaling must be repressed to allow for nighttime CO2 acquisition. It is also unknown what causes daytime stomatal closure in CAM species; while high intracellular CO2 concentrations may play a role, so might ABA signaling. RSL1’s high connectivity to other genes in the subnetwork of E. crista-galli suggests that alterations to regulatory networks are also important for fully functional CAM.
Stomata, while highly responsive to ABA, are also strongly regulated by light inputs. The gene encoding phytochrome B (PHYB), a photoreceptor that both regulates transcriptional responses to red and far-red light as well as entrains the circadian clock (Goosey et al., 1997; Ni et al., 1999; Más et al., 2000), was differentially regulated and expressed in the two Erycina species (Figure 6B). While both species had copies of PHYB that showed time-structured expression, only E. crista-galli had a copy that was in the same network as RSL1 and had many connections to other genes in the same network. A single copy of PHYB was time-structured in CAM E. pusilla, and the expression level relative to copies in C3 E. crista-galli was quite low. Instead, the constitutively expressed copy of PHYB in E. pusilla had the highest expression, but what it’s role might be given constant expression across time is unclear. The stark difference in both connectivity and expression levels of PHYB in E. pusilla and E. crista-galli suggests that light-induced transcriptional regulation has a greater role in the C3 species, and that PHYB mediated transcriptional regulation in CAM species may be significantly reduced. Additionally, the presence of PP2A in the C3 E. crista-galli RSL1 subnetwork, but not in E. pusilla, further indicates that light induced responses are differentially regulated (although expression was similar between the two species, Figure 6D). PP2A is, among many other tasks, responsible for dephosphorylation of phototropin 2, which subsequently promotes stomatal opening (Tseng and Briggs, 2010; Uhrig et al., 2013).
Differences in light input sensing and signaling between C3 and CAM species is not surprising, but relatively little work has focused on this aspect of CAM biology. Previous work assessed light responses in the facultative CAM species Portulacaria afra (Lee and Assmann, 1992) and Mesembryanthemum crystallinum (Tallman et al., 1997) and showed that both species had reduced stomatal response to blue light signals when relying on the CAM cycle for carbon fixation (though see Ceusters et al., 2014). Additionally, M. crystallinum had reduced guard cell zeaxanthin production during the day in the CAM state compared to the C3 state. The reduction in zeaxanthin in the CAM state was shown to be a result of the downregulation of the pathway, rather than an aberration in guard cell chloroplasts. Changes to regulation and expression of PHYB in Erycina further support significantly altered light-induced pathways in CAM species relative to C3.
TIME FOR COFFEE (TIC) also stood out in its altered connectivity between the C3 and CAM species (Figure 6C). In C3 E. crista-galli, TIC was present in the RSL1 diffusion network whereas it was not for E. pusilla. TIC has been shown to be involved in maintaining the amplitude of the circadian clock in Arabidopsis (Hall et al., 2003; Ding et al., 2007), as well as regulating metabolic homeostasis and response to environmental cues (Sanchez-Villarreal et al., 2013). tic mutants in Arabidopsis showed large phenotypic effects ranging from late flowering to anatomical abnormalities. The tic mutants also showed extreme tolerance to drought, likely due to increased amounts of osmolytes such as proline and myo-inositol, as well as an accumulation of starch. As a result of the pleiotropic effects of TIC, altered network status of TIC in CAM E. pusilla compared to C3 E. crista-galli likely results a complex alteration in phenotype. In general, the mechanism that link the circadian clock and CAM photosynthesis are unknown (Boxall et al., 2005), and research to uncover circadian regulation of CAM is limited to transcriptomic studies. Gene expression comparisons between CAM Agave and C3 Arabidopsis revealed changes to the timing of expression of REVEILLE 1, a clock output gene that integrates the circadian network to metabolic activity (Yin et al., 2018). While it’s possible that changes to the timing of REVEILLE 1 are required for CAM evolution, expression differences may also be a result of lineage-specific changes to expression unrelated to CAM. Comparisons of closely related C3 and CAM species of Erycina suggest that alteration of transcriptional cascades from circadian oscillators may play a role in the evolution of CAM, rather than large scale changes to the timing or abundance of expression, but additional work to link clock outputs to the CAM phenotype are necessary.
Implications for the Evolution of CAM in Oncidiinae
Both CAM and C4 photosynthesis have evolved multiple times across the flowering plant phylogeny, suggesting that the evolution of these complex traits cannot be insurmountably difficult. Recent physiological work demonstrates that the evolution of anatomical traits required for CAM or C4 often predates the emergence of strong, constitutive carbon concentrating mechanisms (Christin et al., 2013; Heyduk et al., 2016). Other transcriptomic work has shown that closely related C3 and CAM species share the expression of canonical CAM genes, especially PEPC and PPCK as seen here in Erycina (Heyduk et al., 2018b). It has even been suggested that many C3 plants already have the nocturnal CAM cycle in place for fixation of respired CO2 and generation of amino acids (Bräutigam et al., 2017), but this alone is unlikely to entirely explain the repeated and relatively frequent emergence of CAM on the angiosperm phylogeny.
Indeed it appears that gene expression alone would not facilitate the large-scale transition from C3 to CAM. Recent comparative work across multiple C3 and C4 transcriptomes highlighted the recurrent co-option of highly expressed genes from C3 species into C4 (Moreno-Villena et al., 2018). The initial co-option of highly expressed gene copies happened early in the evolutionary trajectory between C3 and C4, and later steps included the refinement of C3 enzymes, including kinetic and tissue specificity. It is quite likely that a similar model of evolution holds for CAM, in that C3 relatives of CAM lineages have been shown to have similar expression patterns of canonical CAM genes (though, it is worth noting, they are not typically highly expressed in the C3 species). In Erycina, both C3 and CAM species share similar expression profiles for PEPC and PPCK, with the latter having nearly identical levels of expression in both taxa. It is possible that low levels of nocturnal CO2 fixation via PEPC evolved in the ancestor of both species for non-photosynthetic reasons; for example, tobacco leaves (Kunitake et al., 1959) and cotton ovules (Dhindsa et al., 1975) show various levels of carbon concentration, and fixation of cytosolic CO2 via PEPC is required to replenish the citric acid cycle (Aubry et al., 2011). Because these pathways already exist, slight upregulation of some components in a shared ancestor may have enabled the origins of CAM in certain lineages (Bräutigam et al., 2017).
However, gene expression by itself clearly does not make a species CAM, and further refinement is necessary. Refinement of CAM may take the form of improving secondary metabolic processes or genomic characteristics that allow for strong and constitutive CAM to exist. For example, fine tuning of carbohydrate turnover is necessary for CAM function (Ceusters et al., 2014; Borland et al., 2016). Experiments placing Mesembryanthemum crystallinum in CO2-free air at night resulted in a dampened CAM cycle (Dodd et al., 2003) and comparative RNA-seq in facultative CAM and C3/CAM comparisons has shown increased reliance on carbohydrate breakdown as CAM function increases (Brilhaus et al., 2016; Heyduk et al., 2018a). Circadian regulation of CAM genes in pineapple was shown to be the result of promoters that induce evening expression (Ming et al., 2015), and genes that link the clock to metabolic outputs had shifts in expression phasing between Agave (CAM) and Arabidopsis (C3) (Yin et al., 2018). Variation in timing and magnitude of expression of various light sensing and clock genes has been described not only here in Erycina, but also in Agave (Abraham et al., 2016). In all, the growing evidence suggests that the secondary processes that make a CAM plant, including stomatal regulation, light sensing and downstream transcriptional responses, and carbohydrate metabolism feedbacks, undergo fine-tuning along the evolutionary trajectory between C3 and CAM. Further work that characterizes closely related C3 and CAM species has the potential to greatly advance our understanding of the integration of nighttime CO2 acquisition with more complex regulatory pathways.
Data Availability
Raw sequence reads are available on NCBI Short Read Archive, under the BioProject PRJNA483943. All scripts used that are not part of existing programs are available at www.github.com/kheyduk.
Author Contributions
JL-M and VA conceived and led the project. KS and KW collected, phenotyped plants, and conducted continuous gas exchange measurements. KS additionally sampled E. crista-galli for RNA-seq. M-TC provided Erycina pusilla plants. VA, TL, KF, and T-HC grew and sampled Erycina pusilla individuals for RNA and physiology. KH and MH sequenced and analyzed the data and prepared the manuscript. JL-M oversaw general experimental design. All authors contributed to the final version of this manuscript.
Funding
This work was supported by NSF grants to JL-M, VA, and KS (DEB #1442199 and 1442190) and by Smithsonian Tropical Research Institute.
Conflict of Interest Statement
The authors declare that the research was conducted in the absence of any commercial or financial relationships that could be construed as a potential conflict of interest.
Acknowledgments
The authors would like to sincerely thank Orquideas Tropicales in Panama for generously donating Erycina crista-galli plants. Additional gratitude goes to Saravanaraj Ayyampalayam for assistance with data processing, Jeremy Ray for assistance with RNA preparation, and Rishi Masalia for giving feedback on figures.
Supplementary Material
The Supplementary Material for this article can be found online at: https://www.frontiersin.org/articles/10.3389/fpls.2018.02000/full#supplementary-material
Footnotes
References
Abraham, P. E., Yin, H., Borland, A. M., Weighill, D., Lim, S. D., De Paoli, H. C., et al. (2016). Transcript, protein and metabolite temporal dynamics in the CAM plant Agave. Nat. Plants 2:16178. doi: 10.1038/nplants.2016.178
Asai, N., Nakajima, N., Tamaoki, M., Kamada, H., and Kondo, N. (2000). Role of malate synthesis mediated by phosphoenolpyruvate carboxylase in guard cells in the regulation of stomatal movement. Plant Cell Physiol. 41, 10–15. doi: 10.1093/pcp/41.1.10
Aubry, S., Brown, N. J., and Hibberd, J. M. (2011). The role of proteins in C3 plants prior to their recruitment into the C4 pathway. J. Exp. Bot. 62, 3049–3059. doi: 10.1093/jxb/err012
Belsley, D. A., Kuuh, E., and Welsch, R. E. (1980). Regression Diagnostics: Identifying Influential Data and Sources of Collinearity. New York, NY: Wiley. doi: 10.1002/0471725153
Bolger, A. M., Lohse, M., and Usadel, B. (2014). Trimmomatic: a flexible trimmer for Illumina sequence data. Bioinformatics 30, 2114–2120. doi: 10.1093/bioinformatics/btu170
Borland, A. M., Guo, H.-B., Yang, X., and Cushman, J. C. (2016). Orchestration of carbohydrate processing for crassulacean acid metabolism. Curr. Opin. Plant Biol. 31, 118–124. doi: 10.1016/j.pbi.2016.04.001
Borland, A. M., Hartwell, J., Jenkins, G. I., Wilkins, M. B., Nimmo, H. G., Borland, A. M., et al. (1999). Metabolite control overrides circadian regulation of phosphoenolpyruvate carboxylase kinase and CO2 fixation in crassulacean acid metabolism. Plant Physiol. 121, 889–896. doi: 10.1104/pp.121.3.889
Boxall, S. F., Foster, J. M., Bohnert, H. J., Cushman, J. C., Nimmo, H. G., and Hartwell, J. (2005). Conservation and divergence of circadian clock operation in a stress-inducible crassulacean acid metabolism species reveals clock compensation against stress. Plant Physiol. 137, 969–982. doi: 10.1104/pp.104.054577
Bräutigam, A., Schlüter, U., Eisenhut, M., and Gowik, U. (2017). On the evolutionary origin of CAM photosynthesis. Plant Physiol. 174, 473–477. doi: 10.1104/pp.17.00195
Bray, N. L., Pimentel, H., Melsted, P., and Pachter, L. (2016). Near-optimal probabilistic RNA-seq quantification. Nat. Biotechnol. 34, 525–527. doi: 10.1038/nbt.3519
Brilhaus, D., Bräutigam, A., Mettler-Altmann, T., Winter, K., and Weber, A. P. M. (2016). Reversible burst of transcriptional changes during induction of crassulacean acid metabolism in Talinum triangulare. Plant Physiol. 170, 102–122. doi: 10.1104/pp.15.01076
Bueso, E., Rodriguez, L., Lorenzo-Orts, L., Gonzalez-Guzman, M., Sayas, E., Muñoz-Bertomeu, J., et al. (2014). The single-subunit RING-type E3 ubiquitin ligase RSL1 targets PYL4 and PYR1 ABA receptors in plasma membrane to modulate abscisic acid signaling. Plant J. 80, 1057–1071. doi: 10.1111/tpj.12708
Carlin, D. E., Demchak, B., Pratt, D., Sage, E., and Ideker, T. (2017). Network propagation in the cytoscape cyberinfrastructure. PLoS Comput. Biol. 13:e1005598. doi: 10.1371/journal.pcbi.1005598
Ceusters, J., Borland, A. M., Taybi, T., Frans, M., Godts, C., and De Proft, M. P. (2014). Light quality modulates metabolic synchronization over the diel phases of crassulacean acid metabolism. J. Exp. Bot. 65, 3705–3714. doi: 10.1093/jxb/eru185
Chase, M. W., Cameron, K. M., Freudenstein, J. V., Pridgeon, A. M., Salazar, G., van den Berg, C., et al. (2015). An updated classification of Orchidaceae. Bot. J. Linn. Soc. 177, 151–174. doi: 10.1111/boj.12234
Christin, P.-A., Arakaki, M., Osborne, C. P., Bräutigam, A., Sage, R. F., Hibberd, J. M., et al. (2014). Shared origins of a key enzyme during the evolution of C4 and CAM metabolism. J. Exp. Bot. 65, 3609–3621. doi: 10.1093/jxb/eru087
Christin, P.-A., Osborne, C. P., Chatelet, D. S., Columbus, J. T., Besnard, G., Hodkinson, T. R., et al. (2013). Anatomical enablers and the evolution of C4 photosynthesis in grasses. Proc. Natl. Acad. Sci. U.S.A. 110, 1381–1386. doi: 10.1073/pnas.1216777110
Cockburn, W., Ting, I. P., and Sternberg, L. O. (1979). Relationships between stomatal behavior and internal carbon dioxide concentration in crassulacean acid metabolism plants. Plant Physiol. 63, 1029–1032. doi: 10.1104/PP.63.6.1029
Conesa, A., Nueda, M. J., Ferrer, A., and Talón, M. (2006). maSigPro: a method to identify significantly differential expression profiles in time-course microarray experiments. Bioinformatics 22, 1096–1102. doi: 10.1093/bioinformatics/btl056
Crayn, D. M., Winter, K., and Smith, J. A. C. (2004). Multiple origins of crassulacean acid metabolism and the epiphytic habit in the neotropical family Bromeliaceae. Proc. Natl. Acad. Sci. U.S.A. 101, 3703–3708. doi: 10.1073/pnas.0400366101
Cushman, J. C., and Bohnert, H. J. (1997). Molecular genetics of crassulacean acid metabolism. Plant Physiol. 113, 667–676. doi: 10.1104/pp.113.3.667
Cushman, J. C., and Bohnert, H. J. (1999). Crassulacean acid metabolism: molecular genetics. Annu. Rev. Plant Physiol. Plant Mol. Biol. 50, 305–332. doi: 10.1146/annurev.arplant.50.1.305
Cushman, J. C., Tillett, R. L., Wood, J. A., Branco, J. M., and Schlauch, K. A. (2008). Large-scale mRNA expression profiling in the common ice plant, Mesembryanthemum crystallinum, performing C3 photosynthesis and crassulacean acid metabolism (CAM). J. Exp. Bot. 59, 1875–1894. doi: 10.1093/jxb/ern008
Daloso, D. M., Antunes, W. C., Pinheiro, D. P., Waquim, J. P., AraÚJo, W. L., Loureiro, M. E., et al. (2015). Tobacco guard cells fix CO 2 by both Rubisco and PEPcase while sucrose acts as a substrate during light-induced stomatal opening. Plant. Cell Environ. 38, 2353–2371. doi: 10.1111/pce.12555
Demarsy, E., and Fankhauser, C. (2009). Higher plants use LOV to perceive blue light. Curr. Opin. Plant Biol. 12, 69–74. doi: 10.1016/J.PBI.2008.09.002
Desikan, R., Cheung, M.-K., Clarke, A., Golding, S., Sagi, M., Fluhr, R., et al. (2004). Hydrogen peroxide is a common signal for darkness- and ABA-induced stomatal closure in Pisum sativum. Funct. Plant Biol. 31:913. doi: 10.1071/FP04035
Dever, L. V., Boxall, S. F., Kneøová, J., and Hartwell, J. (2015). Transgenic perturbation of the decarboxylation phase of crassulacean acid metabolism alters physiology and metabolism but has only a small effect on growth. Plant Physiol. 167, 44–59. doi: 10.1104/pp.114.251827
Dhindsa, R. S., Beasley, C. A., and Ting, I. P. (1975). Osmoregulation in cotton fiber: accumulation of potassium and malate during growth. Plant Physiol. 56, 394–398. doi: 10.1104/pp.56.3.394
Ding, Z., Millar, A. J., Davis, A. M., and Davis, S. J. (2007). Time for coffee encodes a nuclear regulator in the Arabidopsis thaliana circadian clock. Plant Cell 19, 1522–1536. doi: 10.1105/tpc.106.047241
Dodd, A. N., Griffiths, H., Taybi, T., Cushman, J. C., and Borland, A. M. (2003). Integrating diel starch metabolism with the circadian and environmental regulation of crassulacean acid metabolism in Mesembryanthemum crystallinum. Planta 216, 789–797. doi: 10.1007/s00425-002-0930-2
Edgar, R. C. (2004). MUSCLE: multiple sequence alignment with high accuracy and high throughput. Nucleic Acids Res. 32, 1792–1797. doi: 10.1093/nar/gkh340
Fukayama, H., Tamai, T., Taniguchi, Y., Sullivan, S., Miyao, M., and Nimmo, H. G. (2006). Characterization and functional analysis of phospho enol pyruvate carboxylase kinase genes in rice. Plant J. 47, 258–268. doi: 10.1111/j.1365-313X.2006.02779.x
Gehrig, H., Heute, V., and Kluge, M. (2001). New partial sequences of phosphoenolpyruvate carboxylase as molecular phylogenetic markers. Mol. Phylogenet. Evol. 20, 262–274. doi: 10.1006/mpev.2001.0973
Glenn, T. C., Nilsen, R., Kieran, T. J., Finger, J. W., Pierson, T. W., Bentley, K. E., et al. (2016). Adapterama I: Universal Stubs and Primers for Thousands of Dual-Indexed Illumina Libraries (iTru & iNext). Available at: http://biorxiv.org/content/early/2016/06/15/049114 [accessed May 31, 2017]
Gonzalez-Guzman, M., Pizzio, G. A., Antoni, R., Vera-Sirera, F., Merilo, E., Bassel, G. W., et al. (2012). Arabidopsis PYR/PYL/RCAR receptors play a major role in quantitative regulation of stomatal aperture and transcriptional response to abscisic acid. Plant Cell 24, 2483–2496. doi: 10.1105/tpc.112.098574
Goosey, L., Palecanda, L., and Sharrock, R. A. (1997). Differential patterns of expression of the arabidopsis PHYB, PHYD, and PHYE phytochrome genes. Plant Physiol. 115, 959–969. doi: 10.1104/PP.115.3.959
Gravendeel, B., Smithson, A., Slik, F. J. W., and Schuiteman, A. (2004). Epiphytism and pollinator specialization: drivers for orchid diversity? Philos. Trans. R. Soc. Lond. B Biol. Sci. 359, 1523–1535. doi: 10.1098/rstb.2004.1529
Guralnick, L. J., Rorabaugh, P. A., and Hanscom, Z. (1984). Seasonal shifts of photosynthesis in Portulacaria afra (L.) Jacq. Plant Physiol. 76, 643–646. doi: 10.1104/pp.76.3.643
Haas, B. J., Papanicolaou, A., Yassour, M., Grabherr, M., Philip, D., Bowden, J., et al. (2013). De novo transcript sequence reconstruction from RNA-Seq: reference generation and analysis with Trinity. Nat. Protoc. 8, 1494–1512. doi: 10.1038/nprot.2013.084
Hall, A., Bastow, R. M., Davis, S. J., Hanano, S., McWatters, H. G., Hibberd, V., et al. (2003). The time for coffee gene maintains the amplitude and timing of Arabidopsis circadian clocks. Plant Cell 15, 2719–2729. doi: 10.1105/tpc.013730
Hartwell, J. (2005). The co-ordination of central plant metabolism by the circadian clock. Biochem. Soc. Trans. 33, 945–948. doi: 10.1042/BST20050945
Heyduk, K., McKain, M. R., Lalani, F., and Leebens-Mack, J. (2016). Evolution of CAM anatomy predates the origins of crassulacean acid metabolism in the Agavoideae (Asparagaceae). Mol. Phylogenet. Evol. 105, 102–113. doi: 10.1016/j.ympev.2016.08.018
Heyduk, K., Ray, J. N., Ayyampalayam, S., and Leebens-Mack, J. (2018a). Shifts in gene expression profiles are associated with weak and strong crassulacean acid metabolism. Am. J. Bot. 105, 587–601. doi: 10.1002/ajb2.1017
Heyduk, K., Ray, J. N., Ayyampalayam, S., Moledina, N., Borland, A., Harding, S., et al. (2018b). Shared expression of Crassulacean acid metabolism (CAM) genes predates the origin of CAM in the genus Yucca. bioRxiv [Preprint]. doi: 10.1101/371344
Holtum, J. A. M., Smith, J. A. C., and Neuhaus, H. E. (2005). Intracellular transport and pathways of carbon flow in plants with crassulacean acid metabolism. Funct. Plant Biol. 32:429. doi: 10.1071/FP04189
Horn, J. W., Xi, Z., Riina, R., Peirson, J. A., Yang, Y., Dorsey, B. L., et al. (2014). Evolutionary bursts in Euphorbia (Euphorbiaceae) are linked with photosynthetic pathway. Evolution 68, 3485–3504. doi: 10.1111/evo.12534
Ito, S., Song, Y. H., and Imaizumi, T. (2012). LOV domain-containing F-box proteins: light-dependent protein degradation modules in arabidopsis. Mol. Plant 5, 573–582. doi: 10.1093/MP/SSS013
Jiao, J.-A., and Chollet, R. (1989). Regulatory seryl-phosphorylation of C4 phosphoenolpyruvate carboxylase by a soluble protein kinase from maize leaves. Arch. Biochem. Biophys. 269, 526–535. doi: 10.1016/0003-9861(89)90136-7
Kim, D.-H., Kang, J.-G., Yang, S.-S., Chung, K.-S., Song, P.-S., and Park, C.-M. (2002). A phytochrome-associated protein phosphatase 2A modulates light signals in flowering time control in Arabidopsis. Plant Cell 14, 3043–3056. doi: 10.1105/TPC.005306
Kovermann, P., Meyer, S., Hörtensteiner, S., Picco, C., Scholz-Starke, J., Ravera, S., et al. (2007). The Arabidopsis vacuolar malate channel is a member of the ALMT family. Plant J. 52, 1169–1180. doi: 10.1111/j.1365-313X.2007.03367.x
Kumar, L., and Futschik, M. E. (2007). Mfuzz: a software package for soft clustering of microarray data. Bioinformation 2, 5–7. doi: 10.6026/97320630002005
Kunitake, G., Stitt, C., and Saltman, P. (1959). Dark fixation of CO(2) by tobacco leaves. Plant Physiol. 34, 123–127. doi: 10.1104/PP.34.2.123
Lachmann, A., Giorgi, F. M., Lopez, G., and Califano, A. (2016). ARACNe-AP: gene network reverse engineering through adaptive partitioning inference of mutual information. Bioinformatics 32, 2233–2235. doi: 10.1093/bioinformatics/btw216
Larsson, A. (2014). AliView: a fast and lightweight alignment viewer and editor for large datasets. Bioinformatics 30, 3276–3278. doi: 10.1093/bioinformatics/btu531
Lee, D. M., and Assmann, S. M. (1992). Stomatal responses to light in the facultative Crassulacean acid metabolism species, Portulacaria afra. Physiol. Plant. 85, 35–42. doi: 10.1111/j.1399-3054.1992.tb05260.x
Lee, S. H., Li, C. W., Liau, C. H., Chang, P. Y., Liao, L. J., Lin, C. S., et al. (2015). Establishment of an Agrobacterium-mediated genetic transformation procedure for the experimental model orchid Erycina pusilla. Plant Cell. Tissue Organ. Cult. 120, 211–220. doi: 10.1007/s11240-014-0596-z
Lepiniec, L., Vidal, J., Chollet, R., Gadal, P., and Cretin, C. (1994). Phosphoenolpyruvate carboxylase: structure, regulation and evolution. Plant Sci. 99, 111–124. doi: 10.1016/0168-9452(94)90168-6
Li, B., and Dewey, C. N. (2011). RSEM: accurate transcript quantification from RNA-Seq data with or without a reference genome. BMC Bioinformatics 12:323. doi: 10.1186/1471-2105-12-323
Más, P., Devlin, P. F., Panda, S., and Kay, S. A. (2000). Functional interaction of phytochrome B and cryptochrome 2. Nature 408, 207–211. doi: 10.1038/35041583
Más, P., Kim, W.-Y., Somers, D. E., and Kay, S. A. (2003). Targeted degradation of TOC1 by ZTL modulates circadian function in Arabidopsis thaliana. Nature 426, 567–570. doi: 10.1038/nature02163
Masalia, R. R., Bewick, A. J., Burke, J. M., Irizarry, R., Bertranpetit, J., and Laayouni, H. (2017). Connectivity in gene coexpression networks negatively correlates with rates of molecular evolution in flowering plants. PLoS One 12:e0182289. doi: 10.1371/journal.pone.0182289
Merilo, E., Laanemets, K., Hu, H., Xue, S., Jakobson, L., Tulva, I., et al. (2013). PYR/RCAR receptors contribute to ozone-, reduced air humidity-, darkness-, and CO2-induced stomatal regulation. Plant Physiol. 162, 1652–1668. doi: 10.1104/pp.113.220608
Ming, R., VanBuren, R., Wai, C. M., Tang, H., Schatz, M. C., Bowers, J. E., et al. (2015). The pineapple genome and the evolution of CAM photosynthesis. Nat. Genet. 47, 1435–1442. doi: 10.1038/ng.3435
Moore, A., de Vos, J. M., Hancock, L. P., Goolsby, E., and Edwards, E. J. (2017). Targeted Enrichment of Large Gene Families for Phylogenetic Inference: Phylogeny and Molecular Evolution of Photosynthesis Genes in the Portullugo (Caryophyllales). Available at: http://biorxiv.org/content/early/2017/06/04/145995 [accessed June 19, 2017]
Moreno-Villena, J. J., Dunning, L. T., Osborne, C. P., and Christin, P.-A. (2018). Highly expressed genes are preferentially Co-opted for C4 photosynthesis. Mol. Biol. Evol. 35, 94–106. doi: 10.1093/molbev/msx269
Nelson, E. A., and Sage, R. F. (2008). Functional constraints of CAM leaf anatomy: tight cell packing is associated with increased CAM function across a gradient of CAM expression. J. Exp. Bot. 59, 1841–1850. doi: 10.1093/jxb/erm346
Ni, M., Tepperman, J. M., and Quail, P. H. (1999). Binding of phytochrome B to its nuclear signalling partner PIF3 is reversibly induced by light. Nature 400, 781–784. doi: 10.1038/23500
Nimmo, G. A., Nimmo, H. G., Hamilton, I. D., Fewson, C. A., and Wilkins, M. B. (1986). Purification of the phosphorylated night form and dephosphorylated day form of phosphoenolpyruvate carboxylase from Bryophyllum fedtschenkoi. Biochem. J. 239, 213–220. doi: 10.1042/BJ2390213
Nimmo, H. G. (2000). The regulation of phosphoenolpyruvate carboxylase in CAM plants. Trends Plant Sci. 5, 75–80. doi: 10.1016/S1360-1385(99)01543-5
Nueda, M. J., Tarazona, S., and Conesa, A. (2014). Next maSigPro: updating maSigPro bioconductor package for RNA-seq time series. Bioinformatics 30, 2598–2602. doi: 10.1093/bioinformatics/btu333
Sanchez-Villarreal, A., Shin, J., Bujdoso, N., Obata, T., Neumann, U., Du, S.-X., et al. (2013). TIME FOR COFFEE is an essential component in the maintenance of metabolic homeostasis in Arabidopsis thaliana. Plant J. 76, 188–200. doi: 10.1111/tpj.12292
Shannon, P., Markiel, A., Ozier, O., Baliga, N. S., Wang, J. T., Ramage, D., et al. (2003). Cytoscape: a software environment for integrated models of biomolecular interaction networks. Genome Res. 13, 2498–2504. doi: 10.1101/gr.1239303
Silvera, K., Santiago, L. S., Cushman, J. C., and Winter, K. (2009). Crassulacean acid metabolism and epiphytism linked to adaptive radiations in the orchidaceae. Plant Physiol. 149, 1838–1847. doi: 10.1104/pp.108.132555
Silvera, K., Santiago, L. S., Cushman, J. C., and Winter, K. (2010). The incidence of crassulacean acid metabolism in orchidaceae derived from carbon isotope ratios: a checklist of the flora of Panama and Costa Rica. Bot. J. Linn. Soc. 163, 194–222. doi: 10.1111/j.1095-8339.2010.01058.x
Silvera, K., Santiago, L. S., and Winter, K. (2005). Distribution of crassulacean acid metabolism in orchids of Panama: evidence of selection for weak and strong modes. Funct. Plant Biol. 32:397. doi: 10.1071/FP04179
Silvera, K., Winter, K., Rodriguez, B. L., Albion, R. L., and Cushman, J. C. (2014). Multiple isoforms of phosphoenolpyruvate carboxylase in the Orchidaceae (subtribe Oncidiinae): implications for the evolution of crassulacean acid metabolism. J. Exp. Bot. 65, 3623–3636. doi: 10.1093/jxb/eru234
Somers, D. E., Schultz, T. F., Milnamow, M., and Kay, S. A. (2000). Zeitlupe encodes a novel clock-associated PAS protein from arabidopsis. Cell 101, 319–329. doi: 10.1016/S0092-8674(00)80841-7
Stamatakis, A. (2006). RAxML-VI-HPC: maximum likelihood-based phylogenetic analyses with thousands of taxa and mixed models. Bioinformatics 22, 2688–2690. doi: 10.1093/bioinformatics/btl446
Sullivan, S., Jenkins, G. I., and Nimmo, H. G. (2004). Roots, cycles and leaves. expression of the phosphoenolpyruvate carboxylase kinase gene family in soybean. Plant Physiol. 135, 2078–2087. doi: 10.1104/pp.104.042762
Tallman, G., Zhu, J., Mawson, B. T., Amodeo, G., Nouhi, Z., Levy, K., et al. (1997). Induction of CAM in Mesembryanthemum crystallinum abolishes the stomatal response to blue light and light-dependent zeaxanthin formation in guard cell chloroplasts. Plant Cell Physiol. 38, 236–242. doi: 10.1093/oxfordjournals.pcp.a029158
Taybi, T., Patil, S., Chollet, R., and Cushman, J. C. (2000). A minimal serine/threonine protein kinase circadianly regulates phosphoenolpyruvate carboxylase activity in crassulacean acid metabolism-induced leaves of the common ice plant. Plant Physiol. 123, 1471–1482. doi: 10.1104/pp.123.4.1471
Tseng, T.-S., and Briggs, W. R. (2010). The Arabidopsis rcn1-1 mutation impairs dephosphorylation of Phot2, resulting in enhanced blue light responses. Plant Cell 22, 392–402. doi: 10.1105/tpc.109.066423
Uhrig, R. G., Labandera, A.-M., and Moorhead, G. B. (2013). Arabidopsis PPP family of serine/threonine protein phosphatases: many targets but few engines. Trends Plant Sci. 18, 505–513. doi: 10.1016/J.TPLANTS.2013.05.004
Wai, C. M., VanBuren, R., Zhang, J., Huang, L., Miao, W., Edger, P. P., et al. (2017). Temporal and spatial transcriptomic and microRNA dynamics of CAM photosynthesis in pineapple. Plant J. 92, 19–30. doi: 10.1111/tpj.13630
Walley, J. W., Sartor, R. C., Shen, Z., Schmitz, R. J., Wu, K. J., Urich, M. A., et al. (2016). Integration of omic networks in a developmental atlas of maize. Science 353, 814–818. doi: 10.1126/science.aag1125
Winter, K., and Holtum, J. A. M. (2011). Induction and reversal of crassulacean acid metabolism in Calandrinia polyandra: effects of soil moisture and nutrients. Funct. Plant Biol. 38, 576–582. doi: 10.1071/FP11028
Winter, K., Holtum, J. A. M., and Smith, J. A. C. (2015). Crassulacean acid metabolism: a continuous or discrete trait? New Phytol. 208, 73–78. doi: 10.1111/nph.13446
Yang, X., Hu, R., Yin, H., Jenkins, J., Shu, S., Tang, H., et al. (2017). The Kalanchoë genome provides insights into convergent evolution and building blocks of crassulacean acid metabolism. Nat. Commun. 8:1899. doi: 10.1038/s41467-017-01491-7
Yin, H., Guo, H.-B., Weston, D. J., Borland, A. M., Ranjan, P., Abraham, P. E., et al. (2018). Diel rewiring and positive selection of ancient plant proteins enabled evolution of CAM photosynthesis in Agave. BMC Genomics 19:588. doi: 10.1186/s12864-018-4964-7
Zambrano, V. A. B., Lawson, T., Olmos, E., Fernández-García, N., and Borland, A. M. (2014). Leaf anatomical traits which accommodate the facultative engagement of crassulacean acid metabolism in tropical trees of the genus Clusia. J. Exp. Bot. 65, 3513–3523. doi: 10.1093/jxb/eru022
Keywords: RNA-seq, transcriptomics, photosynthesis, time-course, gene network
Citation: Heyduk K, Hwang M, Albert V, Silvera K, Lan T, Farr K, Chang T-H, Chan M-T, Winter K and Leebens-Mack J (2019) Altered Gene Regulatory Networks Are Associated With the Transition From C3 to Crassulacean Acid Metabolism in Erycina (Oncidiinae: Orchidaceae). Front. Plant Sci. 9:2000. doi: 10.3389/fpls.2018.02000
Received: 02 October 2018; Accepted: 24 December 2018;
Published: 28 January 2019.
Edited by:
Anne MacLaren Borland, Newcastle University, United KingdomReviewed by:
Liangsheng Zhang, Fujian Agriculture and Forestry University, ChinaRobert VanBuren, Michigan State University, United States
Copyright © 2019 Heyduk, Hwang, Albert, Silvera, Lan, Farr, Chang, Chan, Winter and Leebens-Mack. This is an open-access article distributed under the terms of the Creative Commons Attribution License (CC BY). The use, distribution or reproduction in other forums is permitted, provided the original author(s) and the copyright owner(s) are credited and that the original publication in this journal is cited, in accordance with accepted academic practice. No use, distribution or reproduction is permitted which does not comply with these terms.
*Correspondence: Karolina Heyduk, a2Fyb2hleUBnbWFpbC5jb20=