- 1Research Group Sequestration and Detoxification in Insects, Max Planck Institute for Chemical Ecology, Jena, Germany
- 2Research Group Biosynthesis/NMR, Max Planck Institute for Chemical Ecology, Jena, Germany
- 3Department of Biochemistry, Max Planck Institute for Chemical Ecology, Jena, Germany
- 4Department of Bioorganic Chemistry, Max Planck Institute for Chemical Ecology, Jena, Germany
- 5Research Group Mass Spectrometry, Max Planck Institute for Chemical Ecology, Jena, Germany
The cabbage stem flea beetle (Psylliodes chrysocephala) is a key pest of oilseed rape in Europe, and is specialized to feed on Brassicaceae plants armed with the glucosinolate-myrosinase defense system. Upon tissue damage, the β-thioglucosidase enzyme myrosinase hydrolyzes glucosinolates (GLS) to form toxic isothiocyanates (ITCs) which deter non-adapted herbivores. Here, we show that P. chrysocephala selectively sequester GLS from their host plants and store these throughout their life cycle. In addition, P. chrysocephala metabolize GLS to desulfo-GLS, which implies the evolution of GLS sulfatase activity in this specialist. To assess whether P. chrysocephala can largely prevent GLS hydrolysis in ingested plant tissue by sequestration and desulfation, we analyzed the metabolic fate of 4-methylsulfinylbutyl (4MSOB) GLS in adults. Surprisingly, intact and desulfo-GLS together accounted for the metabolic fate of only 26% of the total ingested GLS in P. chrysocephala, indicating that most ingested GLS are nevertheless activated by the plant myrosinase. The presence of 4MSOB-ITC and the corresponding nitrile in feces extracts confirmed the activation of ingested GLS, but the detected amounts of unmetabolized ITCs were low. P. chrysocephala partially detoxifies ITCs by conjugation with glutathione via the conserved mercapturic acid pathway. In addition to known products of the mercapturic acid pathway, we identified two previously unknown cyclic metabolites derived from the cysteine-conjugate of 4MSOB-ITC. In summary, the cabbage stem flea beetle avoids ITC formation by specialized strategies, but also relies on and extends the conserved mercapturic acid pathway to prevent toxicity of formed ITCs.
Introduction
Crucifer-feeding insects encounter a potent chemical defense that consists of two components, the glucosinolates (GLS) and the β-thioglucoside hydrolase myrosinase (Halkier and Gershenzon, 2006). GLS are water-soluble β-thioglucoside-N-hydroxysulfates with a variable amino acid-derived side chain and occur almost exclusively in Brassicales plants (Fahey et al., 2001; Agerbirk and Olsen, 2012). While GLS are non-toxic, their hydrolysis by the myrosinase liberates noxious isothiocyanates (ITCs) with strong reactivity toward thiol- and amine-groups in peptides and proteins (Brown and Hampton, 2011). GLS and myrosinases are stored separately in the plant and ITCs are released rapidly in damaged tissue (Matile, 1980; Andréasson and Jørgensen, 2003). Feeding experiments with generalist as well as specialist insect herbivores have demonstrated negative effects of ITCs on growth, development, and survival (Li et al., 2000; Agrawal and Kurashige, 2003; Barth and Jander, 2006). For example, dietary ITCs depleted cysteine and glutathione levels and activated the catabolism of insect proteins in Spodoptera littoralis larvae, thereby leading to reduced larval weight gain (Jeschke et al., 2015).
Some plant species produce so-called specifier proteins that affect the products of GLS hydrolysis by promoting the formation of nitriles, epithionitriles, and thiocyanates (Burow and Wittstock, 2009; Kuchernig et al., 2012). Compared to ITCs, these compounds are less toxic to herbivores (Lambrix et al., 2001; Burow et al., 2006), but nitriles also play a role in direct and indirect plant defense (Mumm et al., 2008). Interestingly, a nitrile-specifier protein (NSP) was also identified in the larval gut of the cabbage white butterfly, Pieris rapae, where it efficiently prevents ITC formation in the ingested plant tissue (Wittstock et al., 2004). Within Pieridae, NSP activity was detected exclusively in crucifer-feeding species, which, together with phylogenetic analyses, demonstrated that NSPs represent an evolutionary key innovation that enabled a host plant shift from Fabales to Brassicales plants (Wheat et al., 2007; Edger et al., 2015).
Besides specifier proteins, insects evolved several other mechanisms to overcome the GLS-myrosinase defense system (reviewed in Winde and Wittstock, 2011; Jeschke et al., 2015). Larvae of the diamondback moth, Plutella xylostella, secrete a sulfatase enzyme into the gut lumen to convert ingested GLS to stable desulfo-GLS which are not a substrate of myrosinase (Ratzka et al., 2002). This GLS detoxification mechanism evolved convergently in two other generalist herbivores, the desert locust Schistocerca gregaria and the whitefly Bemisia tabaci, but the corresponding sulfatase enzymes in these two species are so far unknown (Falk and Gershenzon, 2007; Malka et al., 2016). Crucifer-feeding sawfly larvae (e.g., Athalia rosae) rapidly sequester GLS into the hemolymph where they are metabolized, and then excreted (Müller and Wittstock, 2005; Opitz et al., 2011; Abdalsamee et al., 2014). The cabbage aphid, Brevicoryne brassicae, and the striped flea beetle, Phyllotreta striolata, also accumulate high amounts of host plant GLS in their bodies to assemble their own GLS-myrosinase system using an insect myrosinase (Kazana et al., 2007; Beran et al., 2014). However, not all crucifer-specialists can prevent ITC formation during feeding. The herbivorous leaf-mining drosophilid Scaptomyza flava avoids plant tissues with high GLS concentration, and evolved a specific glutathione S-transferase to efficiently detoxify ITCs by conjugation to the tripeptide glutathione (GSH) (Gloss et al., 2014; Humphrey et al., 2016). Glutathione conjugates of ITCs are metabolized via the conserved mercapturic acid pathway to cysteinylglycine (CysGly), cysteine (Cys), and N-acetylcysteine (NAC) conjugates in S. flava. The mercapturic acid pathway plays a key role in the detoxification of dietary ITCs in generalist lepidopteran herbivores and molluscs as well, but the pathway end products may differ between species (Schramm et al., 2012; Falk et al., 2014; Jeschke et al., 2017).
In this study, we focus on the cabbage stem flea beetle, Psylliodes chrysocephala, a notorious pest of oilseed rape in Northern Europe (Williams, 2010; Zimmer et al., 2014). Both adults and larvae cause considerable crop damage by feeding on leaves and mining in stems and petioles of winter oilseed rape seedlings, respectively (Godan, 1951; Williams, 2010; Zimmer et al., 2014). Previous studies revealed that P. chrysocephala accept only GLS-containing plants as food (Bartlet and Williams, 1991), and that GLS stimulate adult feeding (Bartlet et al., 1994). Moreover, volatile GLS hydrolysis products attract adults in the field (Bartlet et al., 1992). These findings demonstrate that P. chrysocephala is highly adapted to GLS-containing plants, but how this specialist overcomes the ITC-based defense in its host plants is unknown. To answer this question, we analyzed whether the cabbage stem flea beetle is able to prevent GLS breakdown to ITCs, or metabolizes ITCs to avoid their toxicity.
Materials and Methods
Plants and Insects
Seeds of Brassica rapa cv. “Yu-Tsai-Sum” were purchased from Known-You Seed (Taiwan). Plants were cultivated in a controlled environment chamber (21°C, 55% relative humidity, 14-h light/ 10-h dark period). Arabidopsis thaliana Col-0 wild-type, A. thaliana myb28 × myb29 double-knockout (DKO) mutant plants which are devoid of aliphatic glucosinolates (GLS) (Sønderby et al., 2007), and A. thaliana tgg1 × tgg2 DKO mutant plants lacking myrosinase activity (Barth and Jander, 2006), were cultivated under short-day conditions in a controlled environment chamber (21°C, 55% relative humidity, 10-h light/ 14-h dark period).
The laboratory culture of P. chrysocephala was established in 2012 from adults collected in Laasdorf, Thuringia, Germany. Adults were reared on potted three- to four-week old B. rapa plants in a controlled environment chamber (24°C, 75% relative humidity, 16-h light/8-h dark period). Plants were exchanged every week. Plants with eggs were kept in a separate cage for larval development. After 4 weeks, the remaining plant material was cut and the soil with pupae was kept in plastic containers (9 l volume, Lock&Lock). Newly emerged beetles were collected from the soil containers every two to three days and reared on potted plants as described above.
Chemicals and Chemical Syntheses
4-Methylsulfinylbutyl (4MSOB) GLS was purchased from Phytoplan (Heidelberg, Germany) and 4-hydroxybenzyl (4OHBenzyl) GLS was isolated from seeds of Sinapis alba according to Thies (1988). GLS were converted to desulfo-glucosinolates using Helix pomatia sulfatase solution prepared according to Graser et al. (2001). Recombinant β-O-glucosidase from Caldocellum saccharolyticum was used to prepare 4MSOB cyanide from desulfo-4MSOB GLS as described in Wathelet et al. (2001). 4MSOB isothiocyanate (ITC) was purchased from Alexis Biochemicals (San Diego, USA). 4MSOB-amine, 4MSOB-ITC-glutathione (GSH), 4MSOB-ITC-cysteine (Cys), and 4MSOB-ITC-N-acetylcysteine were purchased from Santa Cruz Biotechnology (Dallas, USA). 4MSOB-ITC-cysteinylglycine (CysGly) was synthesized and purified as described by Kassahun et al. (1997) by using CysGly (Sigma-Aldrich, Munich, Germany) instead of GSH. 4MSOB-acetamide was synthesized from 4MSOB-amine as described by Song et al. (2005).
The conjugate of 4MSOB-ITC with U-13C and 15N-labeled L-cysteine (Sigma-Aldrich; purity 98%) was synthesized according to Kassahun et al. (1997) with the following modifications: 0.16 mmol of 4MSOB-ITC in 2.4 ml ethanol were mixed with 0.16 mmol U-13C15N-L-cysteine dissolved in 3.4 ml 50% (v/v) ethanol (pH 7.5). The mixture was stirred overnight under nitrogen atmosphere at room temperature. The 4MSOB-ITC-13N-Cys conjugate was purified by preparative HPLC using Agilent 1100 series equipment (Agilent Technologies, Böblingen, Germany) using a Supelcosil LC-18-DB Semi-Prep column (250 × 10 mm, 5 μm particle size, Supelco, Bellefonte, USA). Separation was accomplished using a mobile phase consisting of water as solvent A and acetonitrile as solvent B, with the flow rate set at 4 ml/min. The gradient was as follows: 10% (v/v) B (0.5 min), 10–30% (v/v) B (6.5 min), 30–100% (v/v) B (0.1 min), 100% B (v/v) (2.4 min), 100–10% (v/v) B (0.1 min), 10% (v/v) B (2.4 min), compound retention time (RT) 5.3 min.
The cyclic 4MSOB-ITC conjugate 2-(4-(methylsulfinyl)butylamino)-4,5-dihydrothiazole-carboxylic acid (4MSOB-ITC-Cyclic-Cys conjugate A) was synthesized by stirring a mixture of 0.1 mmol 4MSOB-ITC dissolved in 0.35 ml ethanol and 0.1 mmol of L-cysteine dissolved in 4.65 ml water (pH 5.8) at 80°C for 2 h. The cyclic product was isolated by preparative HPLC on a Supelcosil LC-18-DB Semi-Prep column (250 × 10 mm, 5 μm particle size, Supelco, Bellefonte, USA) using a mobile phase consisting of 0.05% (v/v) formic acid in water as solvent A and acetonitrile as solvent B, with the flow rate set at 4 ml/min. The gradient was as follows: 5–15.5% (v/v) B (7 min), 15.5–100% (v/v) B (0.1 min), 100% (v/v) B (1.9 min), 100–5% (v/v) B (0.1 min), 5% (v/v) B (3.9 min), compound RT 6.1 min.
The cyclic 4MSOB-ITC conjugate 4-hydroxy-3-(4-(methylsulfinyl)butyl)-2-thioxothiazolidine-4-carboxylic acid (4MSOB-ITC-Cyclic-Cys conjugate C) was synthesized by stirring a mixture of 14.1 μmol 4MSOB-ITC in 0.5 ml ethanol and 16.8 μmol mercaptopyruvate (Sigma-Aldrich; purity ≥97%) in 0.5 ml 50% (v/v) ethanol under nitrogen atmosphere at room temperature overnight. The product was purified by solid phase extraction (SPE) using a Chromabond® C18ec column (500 mg, Macherey-Nagel, Düren, Germany) followed by preparative HPLC using an EC 250/4.6 Nucleodur Sphinx RP column (250 × 4.6 mm, 5 μm particle size; Macherey-Nagel, Düren, Germany). The mobile phase consisted of 0.05% (v/v) formic acid in water as solvent A and acetonitrile as solvent B, with the flow rate set at 1 ml/min. The gradient was as follows: 5–41% (v/v) B (12 min), 41–100% (v/v) B (0.1 min), 100% (v/v) B (2.9 min), 100–5% (v/v) B (0.1 min), 5% (v/v) B (3.9 min), compound RT 10.5 min.
Sampling of Brassica rapa and Different Life Stages of P. chrysocephala
Leaves of intact four-week old B. rapa plants with six to seven true leaves, and of four-week old plants that had been damaged by P. chrysocephala adults for one week were harvested to analyze their GLS profiles (N = 5–8). From each plant, the second, third, and fourth fully expanded leaf was harvested separately, weighed, frozen in liquid N2, and freeze-dried. To analyze GLS in P. chrysocephala, eggs, larvae (stages L2 and L3), prepupae, pupae, and adults (newly emerged, one-, three-, seven-, and 14-day old fed on B. rapa) were collected from the laboratory rearing, weighed, frozen in liquid N2 and stored at −20°C until extraction (N = 7–9). Eggs were washed three times with water before they were frozen.
GLS Extraction and Analysis
The extraction and analysis of GLS from homogenized B. rapa leaves and P. chrysocephala samples was performed as described in Beran et al. (2014). 4OHBenzyl GLS was used as internal standard and desulfo-GLS were eluted from DEAE-Sephadex columns with 0.5 ml ultrapure water. Samples were stored at −20°C until analysis by HPLC-UV at 229 nm.
Data Analysis
The GLS concentrations in intact and damaged B. rapa plants were determined as the average GLS concentration of the three leaves per plant. The concentrations of individual GLS in undamaged and feeding-damaged plants were compared using Student's t-test or Mann-Whitney U-test if data were not normally distributed. The GLS profiles of intact and damaged B. rapa leaves and P. chrysocephala at different life stages were analyzed by principal component analysis (PCA) using the software MetaboAnalyst 3.0 (Xia and Wishart, 2011, 2016). Total GLS concentration was set to 100%, and the percentage of each GLS was calculated. Prior to analysis, data were cube-root transformed and mean centered. The result was visualized in score and loadings plots. Ellipsoids in the score plot correspond to the 95% confidence intervals of each group. Total GLS amount per individual, total GLS concentration, and percentages of individual GLS were compared using either analyses of variance (ANOVA) followed by the post-hoc Tukey HSD test, gamma generalized linear model, or the method of generalized least squares (nlme library, Pinheiro et al., 2017) depending on the variance homogeneity and the normality of residuals. If necessary, data were arcus-sinus-square-root transformed in order to achieve normality of residuals. For data analyzed with the generalized least squares method, the varIdent variance structure (which allows each group to have a different variance) was applied. P-values were obtained by removing the explanatory variable and comparison of models with likelihood ratio test (Zuur et al., 2009). To analyze which groups differed from each other, factor level reduction was applied (Crawley, 2013). Analyses were done in Sigma Plot 11.0 or in R 3.4.1 (R Core Team, 2017). Information about transformation, used method, and result of the statistical analysis for each GLS is summarized in Supplementary Table S1.
Quantitative Feeding Experiment With 4MSOB GLS
To analyze the metabolic fate of ingested GLS in P. chrysocephala adults, a quantitative feeding experiment was performed using 4MSOB GLS that is not present in the host plant B. rapa. A total of 250 nmol 4MSOB GLS were incorporated into detached leaves of the A. thaliana myb28 × myb29 DKO mutant as described in Schramm et al. (2012). Leaves were placed individually in 5 ml tubes (Eppendorf, Germany) with four newly emerged adults (N = 6). Beetles fed with an A. thaliana myb28 × myb29 DKO mutant leaf without additional GLS served as background control (N = 3), and leaves without beetles were used to determine the GLS recovery rate under feeding assay conditions (N = 4). After feeding for one day, the beetles were transferred to a new tube containing a non-spiked A. thaliana myb28 × myb29 DKO mutant leaf for an additional day to ensure that all plant material containing 4MSOB GLS had been digested and excreted. Remaining leaves were frozen in liquid N2 and freeze-dried. Feces were collected in 0.1% (v/v) formic acid in water, and stored at −20°C until extraction. Beetle and leaf samples were homogenized, extracted using 1 ml 50% (v/v) methanol in 0.1% (v/v) formic acid, and transferred to glass vials after centrifugation. Feces samples were homogenized and centrifuged, and the supernatant was transferred to a glass vial. Extracts of feces and the second non-spiked leaf were evaporated to dryness using a gentle nitrogen stream, re-dissolved in 100 μl of 50% (v/v) methanol in 0.1% (v/v) formic acid, and stored at −20°C until LC-MS/MS analysis.
Sequestration Experiment With A. thaliana tgg1 × tgg2 and Col-0 Wild-ype
A feeding experiment was performed to compare the accumulation of intact 4MSOB GLS in beetles which fed on the myrosinase-deficient tgg1 × tgg2 mutant and on Col-0 wild-type leaves, respectively. Two newly emerged adults were placed in a Petri dish with a moistened filter paper and a cut A. thaliana leaf from a five-week old plant (N = 24). On the next day, the remaining leaf tissue was weighed, frozen in liquid N2 and freeze-dried. Adults were supplied with a myb28 × myb29 leaf until they were weighed and frozen in liquid N2 on the next day. GLS present in leaves and adults were extracted and analyzed as described above. The 4MSOB GLS concentration in adults was determined as percentage relative to the 4MSOB GLS concentration in the corresponding leaf (set to 100%). The 4MSOB GLS concentration in the fed leaves of the two A. thaliana genotypes was compared by Student's t-test, and the relative accumulation of 4MSOB GLS in beetles was compared by Mann-Whitney U-test in Sigma Plot 11.0. To test whether beetles feed equally on the two different A. thaliana genotypes, the remaining leaf area after feeding of two newly emerged adults on a leaf disc (16 mm diameter) for five hours was analyzed using the software Fiji (Schindelin et al., 2012) (N = 27). The remaining leaf area was compared by Student's t-test in Sigma Plot 11.0.
Identification of 4MSOB GLS-Derived Metabolites in Feces of P. chrysocephala
To identify other 4MSOB GLS-derived metabolites, feces were analyzed after adults fed on cut leaves of A. thaliana Col-0 wild-type plants, leaves of the tgg1 × tgg2 and myb28 × myb29 DKO mutants, and myb28 × myb29 leaves spiked with 4MSOB GLS as described above, respectively (N = 3). Feces of six newly emerged adults that fed on one leaf for two days were collected and extracted in 200 μl 0.1% (v/v) formic acid (pH 3). Samples were stored at −20°C until HPLC-MS (ion trap) analysis.
In vivo Metabolism of 4MSOB-Amine and the 4MSOB-ITC-13N-Cys-conjugate in Adults
To elucidate the metabolic origin of previously unknown 4MSOB-ITC-derived metabolites which were identified in feces of P. chrysocephala, adults were forced to ingest 0.2 μl of 4MSOB-amine (74 mM), and 4MSOB-ITC-13N-Cys conjugate (33.5 mM), respectively (N = 3). Feces of five to ten beetles per replicate were collected after beetles fed on a cut myb28 × myb29 leaf for one day. Samples were extracted and stored as described in the previous section.
Identification and Qualitative Analyses of 4MSOB-ITC-Derived Metabolites in Aqueous Feces Samples by HPLC-MS
Comparative HPLC-MS (ion trap) analyses of aqueous feces extracts were carried out on an Agilent 1100 series HPLC (Agilent Technologies, Waldbronn, Germany) coupled to an Esquire 6000 ESI-Ion Trap mass spectrometer (Bruker Daltonics, Bremen, Germany) operated in alternating ionization mode in the range m/z 55–1000. Skimmer voltage −40 eV, capillary exit voltage −102.3 eV, capillary voltage 4,000 V, nebulizer pressure 35 psi, drying gas, 11 l/min; gas temperature 330°C. MS2 and MS3 spectra of candidate metabolites were obtained using the AutoMS mode of the software (Bruker Daltonics). Separation was accomplished using a Nucleodur Sphinx RP column (250 × 4.6 mm, 5 μm particle size, Macherey-Nagel) with a mobile phase consisting of 0.2% (v/v) formic acid in ultrapure water as solvent A and acetonitrile as solvent B with a flow rate of 1.0 ml/min at 25°C. The gradient was as follows: 0–100% (v/v) B (25 min), 100% B (3 min), 100–0% (v/v) B (0.1 min), 0% B (4.9 min). Eluent flow rate was diverted in a ratio of 4:1 before entering the ESI unit. Chromatograms were analyzed with the DataAnalysis and MetaboliteTools software packages from Bruker Daltonics. Candidate metabolites were further analyzed using MS/MS and high resolution mass spectrometry (see section UHPLC-ESI-MS/MS).
UHPLC-ESI-MS/MS
To determine the exact mass of candidate metabolites, ultra-high-performance liquid chromatography–electrospray ionization–tandem mass spectrometry (UHPLC–ESI–MS/MS) was performed with an Ultimate 3000 series RSLC (Dionex, Sunnyvale, CA, USA) and a LTQ-Orbitrap XL mass spectrometer (Thermo Fisher Scientific, Bremen, Germany). UHPLC was used applying an Acclaim C18 column (150 × 2.1 mm, 2.2 μm particle size, Dionex). Separation was accomplished using a mobile phase consisting of 0.1% (v/v) formic acid in ultrapure water as solvent A and 0.1% (v/v) formic acid in acetonitrile as solvent B with a flow rate of 300 μl/min at 25°C. The gradient was as follows: 0–100% (v/v) B (15 min), 100% B (5 min), 100–0% (v/v) B (0.1 min), 0% B (5 min). ESI source parameters were set to 4,000 V for spray voltage, 35 V for transfer capillary voltage at a capillary temperature of 275°C. Samples were measured in positive and negative ionization mode at a mass range of m/z 50–1000 using 30,000 m/Δm resolving power in the Orbitrap mass analyzer. Data were evaluated and interpreted using XCALIBUR software (Thermo Fisher Scientific).
Isolation of 4MSOB-ITC-Derived Metabolites Using Preparative HPLC
Three previously unknown 4MSOB-ITC-derived metabolites (m/z = 178 [M+H]+, RT 6.1 min; m/z = 295 [M-H]−, RT 7.2 min; m/z = 296 [M-H]−, RT 10.8 min) were isolated from feces extracts to elucidate their structure by nuclear magnetic resonance (NMR) spectroscopy. To obtain enough material for isolation, feces of 250 P. chrysocephala adults, which had fed on A. thaliana Col-0 wild-type plants for two consecutive days were collected and extracted in 0.1% (v/v) formic acid in water. The crude extract was purified and concentrated by SPE using a Chromabond® C18ec column (500 mg, Macherey-Nagel). The fraction obtained from elution with 20% (v/v) methanol was further fractionated by preparative HPLC using the method described for 4MSOB-ITC-Cyclic-Cys conjugate C. HPLC fractions were purified and concentrated by SPE and lyophilized. During the purification process we observed a spontaneous conversion of target metabolite m/z = 295 to metabolite m/z = 296 (4MSOB-ITC-Cyclic-Cys conjugate C). To obtain sufficient material of metabolite m/z = 295 for NMR, another feces extract was freeze-dried (ca. 40 mg feces dry weight) and extracted four times with 0.5 ml ultrapure water. Afterwards, a liquid-liquid extraction of the aqueous extract with dichloromethane was performed to remove 4MSOB-cyanide which co-eluted with the target metabolite. The final extract was fractionated by preparative HPLC and freeze-dried as described above. This procedure could not fully prevent the conversion of m/z = 295 to m/z = 296, but yielded a higher proportion of the target metabolite compared to the first attempt.
Structure Elucidation Using NMR Spectroscopy
NMR spectra were recorded on a Bruker Advance III HD 700 spectrometer, equipped with a cryoplatform and a 1.7 mm TCI microcryoprobe (Bruker Biospin GmbH, Rheinstetten, Germany). NMR tubes of 1.7 mm outer diameter were used for all measurements. All NMR spectra were recorded using MeOH-d3 as a solvent. Chemical shifts were referenced to the residual solvent peaks at δH 3.31 and δC 49.15, respectively. Data acquisition and processing were accomplished using TopSpin 3.2. (Bruker Biospin). Standard pulse programs as implemented in TopSpin were used for data acquisition.
Quantification of 4MSOB GLS and Derived Metabolites by LC-MS/MS
GLS and derived metabolites present in samples from the quantitative feeding experiment were quantified by liquid chromatography-tandem mass spectrometry (LC-MS/MS) using an Agilent 1200 HPLC system connected to an API3200 tandem mass spectrometer (AB Sciex, Darmstadt, Germany). Intact and desulfo-GLS were separated on a Nucleodur Sphinx RP column (250 × 4.6 mm, 5 μm particle size; Macherey-Nagel) using a mobile phase consisting of 0.2% (v/v) formic acid in ultrapure water as solvent A and acetonitrile as solvent B, at a flow rate of 1 ml/min. The gradient was as follows: 1.5% (v/v) B (1 min), 1.5–5% (v/v) B (5 min), 5–7% (v/v) B (2 min), 7–13.3% (v/v) B (4.5 min), 13.3–100% (v/v) B (0.1 min), 100% B (0.9 min), 100–1.5% (v/v) B (0.1 min), 1.5% (v/v) B (3.9 min). The presence of GLS was detected with the ionization source set to negative mode. Ionspray voltage was maintained at −4,500 eV. Gas temperature was set to 700°C, nebulizing gas to 70 psi, drying gas to 60 psi, curtain gas to 20 psi, and collision gas to 10 psi. Desulfo-GLS were detected in positive ionization mode. Ionspray voltage was maintained at 5,000 eV. Gas temperature was set to 700°C, nebulizing gas and drying gas to 60 psi, curtain gas to 35 psi, and collision gas to 5 psi. Hydrolysis products of 4MSOB GLS were separated on a Zorbax Eclipse XDB-C18 column (50 × 4.6 mm, 1.8 μm particle size; Agilent) using a mobile phase consisting of 0.05% (v/v) formic acid in ultrapure water as solvent A and acetonitrile as solvent B, at a flow rate of 1.1 ml/min. Separation of 4MSOB GLS hydrolysis products was achieved by using the following gradient: 3–15% (v/v) B (0.5 min), 15–85% (v/v) B (2 min), 85–100% (v/v) B (0.1 min), 100% B (0.9 min) 100–3% (v/v) B (0.1 min), 3% (v/v) B (2.4 min). Multiple-reaction monitoring (MRM) was used to monitor analyte parent ion-to-product ion formation (Supplementary Table S2). Compounds were quantified via external calibration curves (for sources of standards see section Chemicals and Chemical Syntheses). Data analysis was performed using Analyst Software 1.6 Build 3773 (AB Sciex).
Myrosinase Activity Assay
Crude protein extracts of L3 larvae and adults (14 days old and starved for one day) were analyzed for myrosinase activity. For each sample, three to four individuals were pooled, the fresh weight was determined, and the tissue was immediately frozen in liquid N2. Three samples were prepared for each life stage and were stored at −80°C until protein extraction. The tissue (ca. 15 mg) was homogenized in 600 μl chilled 20 mM 2-(N-morpholino)ethanesulfonic acid (MES) buffer (pH 6.5) supplemented with protease inhibitors (cOmplete EDTA-free; Roche) using a 2-ml potter tissue grinder. The crude extract was ultracentrifuged at 4°C for 45 min at 48,000 × g. To remove intact GLS and other compounds that might interfere with the enzyme activity assay, the supernatant was first applied to a 20 mg DEAE-Sephadex A-25 column equilibrated with extraction buffer. The flow-through was subsequently transferred to a Zeba™ Spin Desalting Column (7KDa MWCO 2 ml; Thermo Scientific) equilibrated with extraction buffer. Afterwards, the protein concentration was determined using the Bradford protein assay (Bio-Rad) according to the manufacturer's manual. Myrosinase activity assays were performed with two different GLS as substrates: allyl GLS (Roth) and 2-hydroxy-3-butenyl GLS (Phytoplan, Heidelberg, Germany). Enzyme assays were performed in dark 96-well plates using the Amplite Fluorimetric Glucose Quantitation Kit (AAT Bioquest, Sunnyvale, USA). Each assay consisted of 25 μl protein sample (1.25 μg protein), 25 μl substrate at 2 mM (dissolved in MES buffer, pH 6.5) and 50 μl assay reagent. Assays without substrate and without protein, respectively, served as background control. Two technical replicates were measured for each substrate and the corresponding controls. Fluorescence intensity (Ex/Em = 540 nm/590 nm) was monitored every min for 30 min using a Tecan Infinite 200 Reader (Crailsheim, Germany). Glucose amounts in each assay were calculated from a glucose standard curve (dissolved in MES buffer, pH 6.5) for each time point. The amount of released glucose in the activity assay was determined by subtracting the amount of glucose detected in both background controls.
Results
Glucosinolates Are Present in All Life Stages of P. chrysocephala
Chemical analyses of P. chrysocephala eggs, larvae, pupae, and adults revealed that GLS are present in all life stages at up to three-fold higher concentrations than detected in their rearing plant B. rapa (Figure 1; Table 1). GLS concentrations were similar in L3 larvae, pupae and adults (3.9 ± 0.9 μmol per g FW), but were significantly higher in eggs and L2 larvae (8.9 ± 1.3 and 5.5 ± 1.0 μmol GLS per g FW, respectively; N = 7–9; Figure 1; Supplementary Table S1).
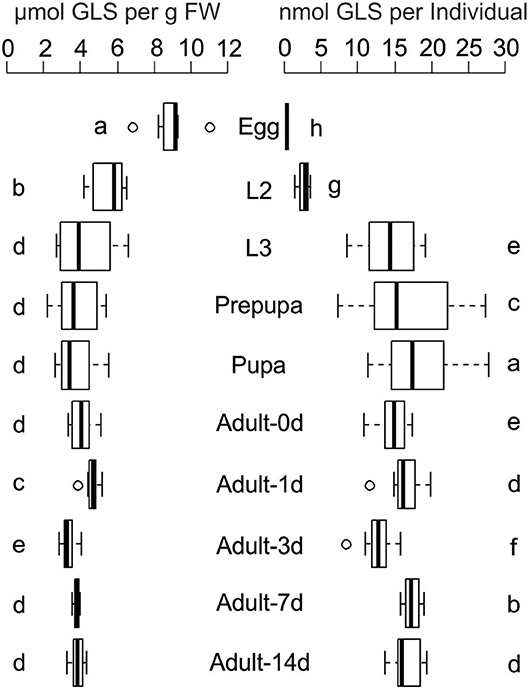
Figure 1. Glucosinolate (GLS) concentration and GLS amount per individual in all life stages of P. chrysocephala reared on B. rapa plants. Extracted GLS were analyzed by HPLC-UV, and GLS concentration is expressed per g fresh weight (FW). Different letters indicate significant differences between the life stages (N = 7–9). L2, second larval instar; L3, third larval instar. Details of statistical analyses are shown in Supplementary Table S1.
During development, the GLS amount per individual increased from 0.36 ± 0.04 nmol per egg to 18.4 ± 5.8 nmol in the pupa. The total GLS amount slightly decreased to 14.7 ± 2.3 nmol in newly emerged adults and remained at comparable levels in one-, three-, seven-, and 14-days old beetles which fed on B. rapa (N = 7–9; Figure 1; Supplementary Table S1).
All GLS detected in insect samples were also present in the host plant, but the relative composition of GLS in B. rapa and P. chrysocephala differed significantly (Figure 2A; Table 2, Supplementary Table S1). Eggs had a distinct GLS composition resembling that of the damaged plant, and were separated from all other developmental stages, which clustered together in a principal component analysis (Figure 2A). Later life stages were separated from plants and eggs because they contained a higher proportion of 2-hydroxy-3-butenyl GLS (up to 75%), and significantly less 3-butenyl-, and 4-pentenyl GLS (Figure 2B).
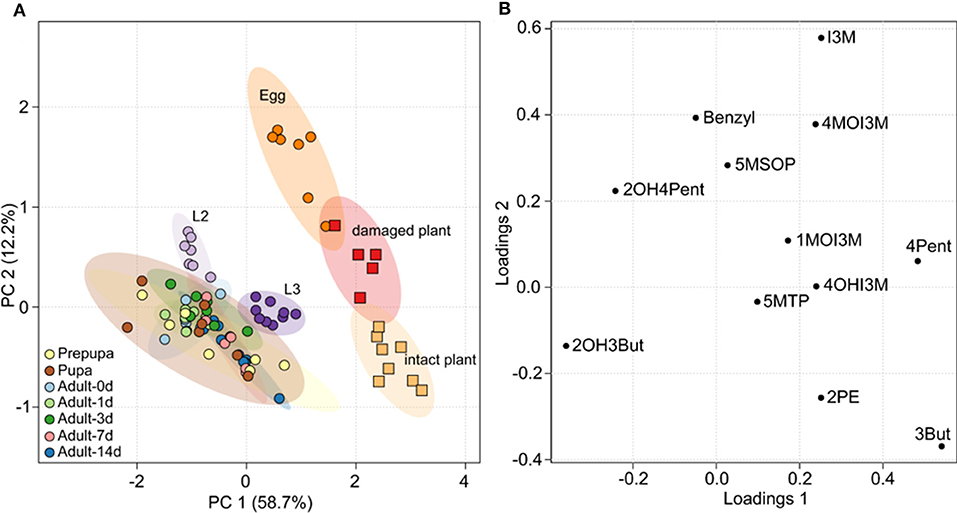
Figure 2. Principal component analysis (PCA) of the GLS composition in B. rapa and P. chrysocephala. GLS were extracted from intact and feeding damaged B. rapa leaves, and from all different life stages of P. chrysocephala (N = 5–9). GLS were analyzed by HPLC-UV and the percentage of each individual GLS was calculated from the total GLS concentration in each sample (Table 2). (A) PCA score plot; samples from P. chrysocephala are shown as circles, plant samples are shown as squares. Each circle or square represents the relative composition of all GLS in one replicate. The ellipsoids correspond to the 95% confidence intervals of each group. The GLS composition in P. chrysocephala larvae, prepupae, pupae and adults is very similar, but is separated from eggs and plants along principal component 1 (PC 1) which explains 58.7% of the total variability in the dataset. (B) PCA loadings plot showing the contribution of the individual GLS to the separation of the groups. 2-Hydroxy-3-butenyl-GLS (2OH3But), 3-butenyl GLS (3But), and 4-pentenyl GLS (4Pent) have the greatest influence on the separation of the groups along PC1. For abbreviations of GLS see legend of Table 1.
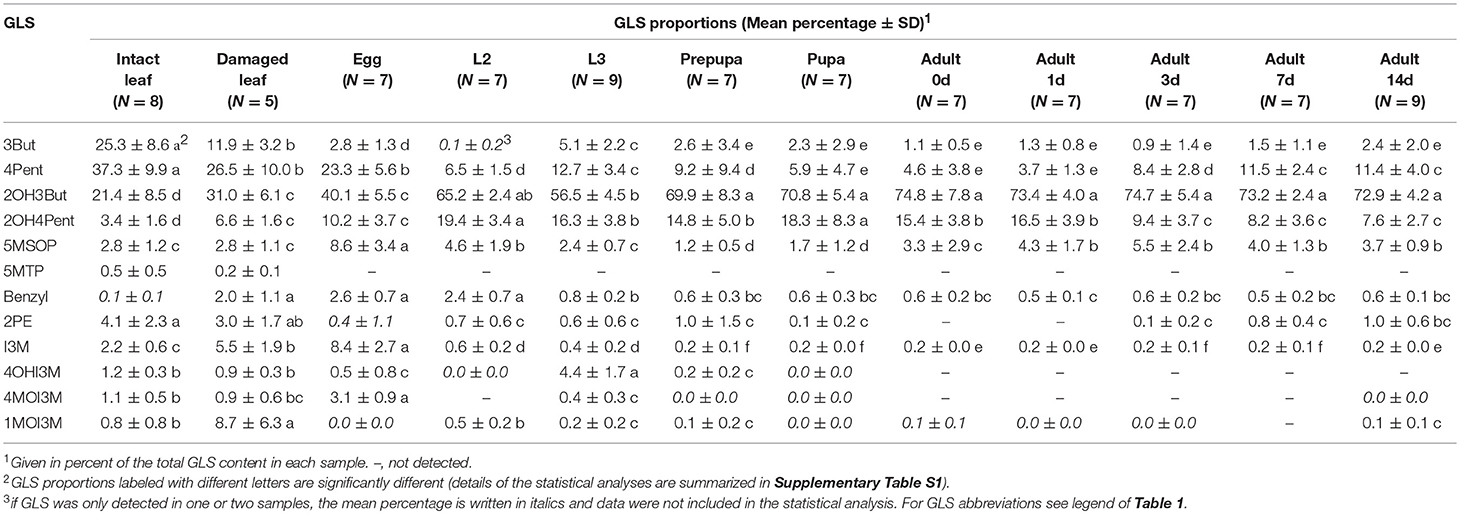
Table 2. Composition of GLS profiles of intact and feeding damaged B. rapa leaves and different life stages of P. chrysocephala.
To determine whether P. chrysocephala can activate sequestered GLS for their own defense, myrosinase activity assays were performed with crude protein extracts of larvae and adults. However, no myrosinase activity was detected under our assay conditions using allyl- and 2-hydroxy-3-butenyl GLS as substrates.
P. chrysocephala Partially Prevent GLS Hydrolysis
To assess how much of the total ingested GLS P. chrysocephala adults accumulate in their body, adults were fed for one day with detached leaves of the A. thaliana myb28 × myb29 mutant spiked with 250 nmol of 4MSOB GLS. The GLS recovery from control leaves without beetles was 93%, which demonstrates that only a small fraction of GLS was metabolized in leaves under assay conditions. The amount of ingested GLS was determined by quantifying the remaining amount of 4MSOB GLS in fed leaves. The quantification of 4MSOB GLS in adult beetles and feces samples revealed that adults accumulated 17% of the total ingested GLS in their body, and that only low amounts of intact 4MSOB GLS were present in feces samples (Table 3).
In addition to the intact GLS, desulfo-4MSOB GLS was present in body and feces samples, and this metabolite accounted for about 8% of the total GLS P. chrysocephala ingested (Table 3). Because GLS sequestration and desulfation explained the fate of only 26% of the total ingested GLS, we hypothesized that most ingested GLS are hydrolyzed by the plant myrosinase during feeding.
To further test the potential influence of plant myrosinase activity on the metabolic fate of GLS in P. chrysocephala, we compared the accumulation of 4MSOB GLS in adults that fed on leaves of the myrosinase-deficient A. thaliana tgg1 × tgg2 DKO mutant and the corresponding wild-type, respectively. Although adults fed equally on both A. thaliana genotypes (N = 27, Student's t-test, t = −0.53, P = 0.60) containing similar concentrations of 4MSOB GLS (N = 24, Student's t-test, t = 0.45, P = 0.66), beetles contained six times more 4MSOB GLS after feeding on tgg1 × tgg2 leaves than after feeding on leaves of wild-type plants (N = 24; Mann-Whitney U-test, U = 4.0, P < 0.001; Figure 3).
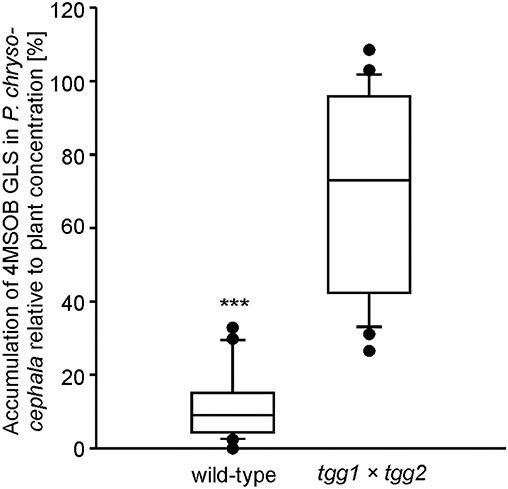
Figure 3. Amount of intact 4-methylsulfinylbutyl (4MSOB) GLS in P. chrysocephala adults after feeding on the myrosinase-deficient mutant (tgg1 × tgg2) or wild-type A. thaliana Col-0. Two newly emerged adults were allowed to feed on a detached leaf from a five-week old mutant or wild-type plant for 24 h, and subsequently on a detached leaf of the A. thaliana myb28 × myb29 mutant devoid of aliphatic GLS for 20 h. Extracted GLS from leaves and adults were analyzed by HPLC-UV. The 4MSOB GLS concentration per mg fresh weight in adults was determined and expressed relative to the 4MSOB GLS concentration in the corresponding leaf which was set to 100% (N = 24). ***P < 0.001.
P. chrysocephala Detoxify 4MSOB-ITC by Conjugation to Glutathione
In A. thaliana Col-0 wild-type leaves, tissue damage leads to the conversion of 4MSOB GLS to 4MSOB-ITC and minor amounts of the corresponding nitrile (Lambrix et al., 2001; Schramm et al., 2012). These expected GLS breakdown products were also present in our samples, and accounted for 2% and 9% of the total ingested GLS, respectively (Table 3). In agreement with previous studies on the metabolism of dietary ITCs in insect and molluscan herbivores (Schramm et al., 2012; Falk et al., 2014; Gloss et al., 2014; Jeschke et al., 2017), we found several 4MSOB-ITC-derived metabolites which resulted from the conjugation with GSH, i.e., ITC conjugates with GSH, CysGly and Cys, respectively. In addition, the cyclic Cys conjugate 2-(4-(methylsulfinyl)butylamino)-4,5-dihydrothiazole-carboxylic acid was detected, a metabolite that was previously discovered in the feces of molluscs (Falk et al., 2014) (Figure 4). The NAC conjugate of 4MSOB-ITC was not detected in P. chrysocephala. Together, these metabolites accounted for the metabolic fate of 6% of the total ingested GLS (Table 3). Moreover, ITC-GSH conjugates can be metabolized to amines and raphanusamic acid (Bednarek et al., 2009; Piślewska-Bednarek et al., 2018). Indeed, 4MSOB-amine was present in P. chrysocephala body and feces extracts and accounted for 5.5% of the total ingested GLS (Table 3; Supplementary Figure S1).
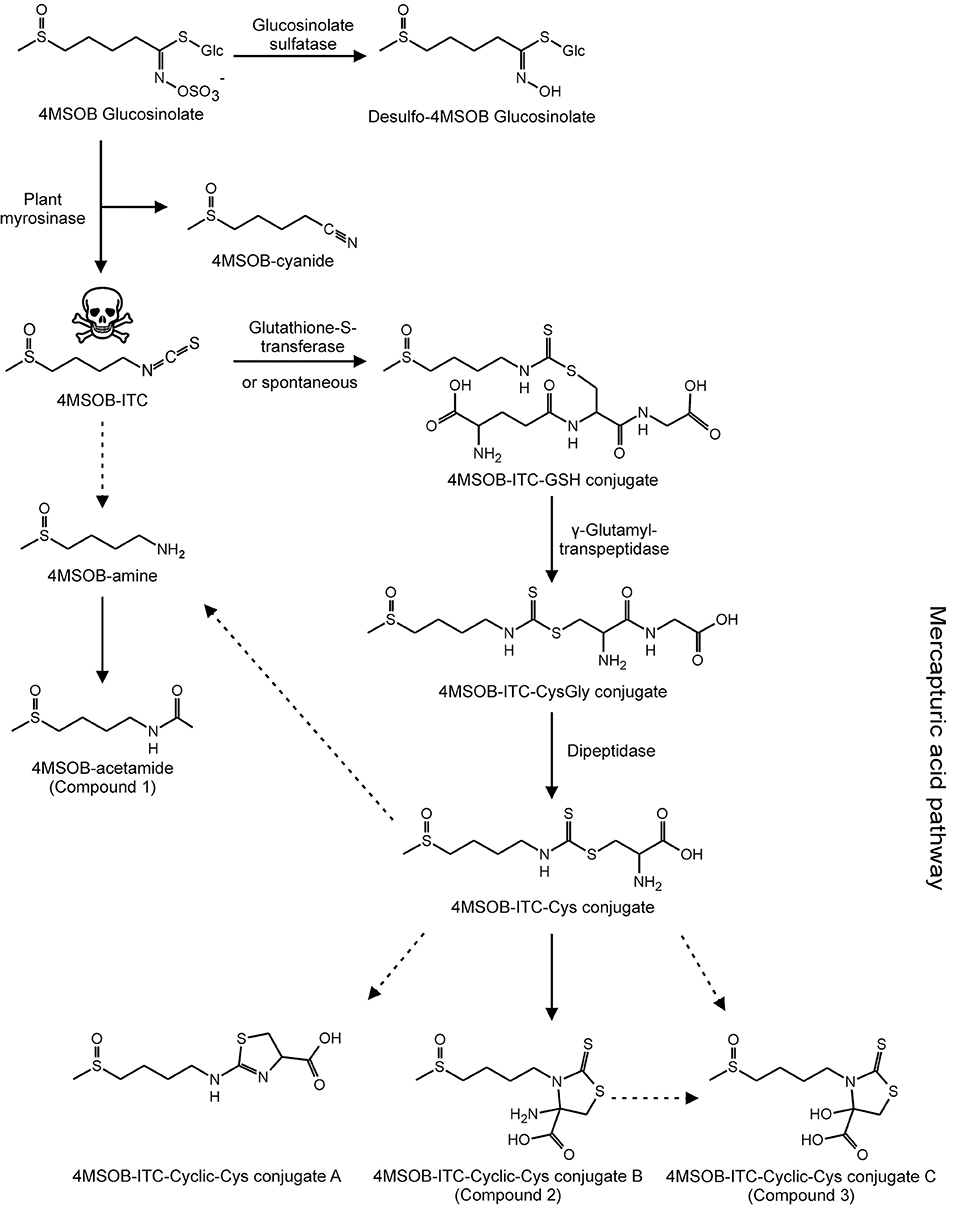
Figure 4. Metabolic fate of ingested 4MSOB GLS in P. chrysocephala adults. Ingested 4MSOB GLS is partially sequestered and additionally detoxified by desulfation. A fraction of ingested 4MSOB GLS is activated by the plant myrosinase to 4MSOB isothiocyanate (ITC) and the corresponding nitrile (4MSOB-cyanide). 4MSOB-ITC is detoxified by conjugation with glutathione (GSH). The ITC-GSH conjugate is metabolized via the mercapturic acid pathway to several cyclic ITC-Cys conjugates, i.e., 2-(4-(methylsulfinyl)butylamino)-4,5-dihydrothiazole-carboxylic acid (4MSOB-ITC-Cyclic-Cys conjugate A), 4-amino-3- (4-(methylsulfinyl)butyl)-2- thioxothiazolidine-4-carboxylic acid (4MSOB-ITC-Cyclic-Cys conjugate B, compound 2), and 4-hydroxy-3-(4-(methylsulfinyl)butyl)-2-thioxothiazolidine-4-carboxylic acid (4MSOB-ITC-Cyclic-Cys conjugate C, compound 3). Additionally, 4MSOB-amine and 4MSOB-acetamide (compound 1) were detected. Proposed metabolic steps are indicated with dashed arrows.
Identification of Previously Unknown Metabolites Derived From 4MSOB-ITC in Feces of P. chrysocephala
To search for other 4MSOB-ITC-derived metabolites, feces extracts of beetles that had fed on detached leaves of different A. thaliana genotypes (Col-0 wild-type, myb28 × myb29 lacking aliphatic GLS, 4MSOB GLS-spiked myb28 × myb29, and myrosinase-deficient tgg1 × tgg2) were compared by LC-MS. Peaks that were only detected when adults had fed on A. thaliana wild-type and 4MSOB GLS-spiked myb28 × myb29 leaves were further analyzed with high resolution mass spectrometry (HRMS) and MS/MS. This approach led to the identification of three previously unknown 4MSOB-ITC-derived metabolites, which were isolated from feces extracts by preparative HPLC to elucidate their structure with NMR.
Compound 1 showed a molecular ion peak of m/z 178.09012 ([M+H]+) which was consistent with the molecular formula C7H15O2NS ((C7H15O2NS+H)+ calculated (calcd) m/z 178.089625). NMR analyses revealed that this compound is 4MSOB-acetamide (Supplementary Table S3), and excreted and chemically synthesized 4MSOB-acetamide had the same LC retention time and mass spectrum (Supplementary Figure S2). However, the quantification of 4MSOB-acetamide by LC-MS/MS revealed that it was only a minor metabolite, accounting for >1% of the total ingested GLS in P. chrysocephala (Table 3).
To elucidate whether P. chrysocephala converts 4MSOB-amine to 4MSOB-acetamide in vivo, adults were fed aqueous 4MSOB-amine or water as a control. Afterwards, beetles were allowed to feed on A. thaliana myb28 × myb29 leaves to obtain feces for chemical analysis. After ingestion of 4MSOB-amine, adults excreted 4MSOB-acetamide and only traces of 4MSOB-amine, whereas both metabolites were not present in feces of adults, which had ingested water as a control (Figure 5).
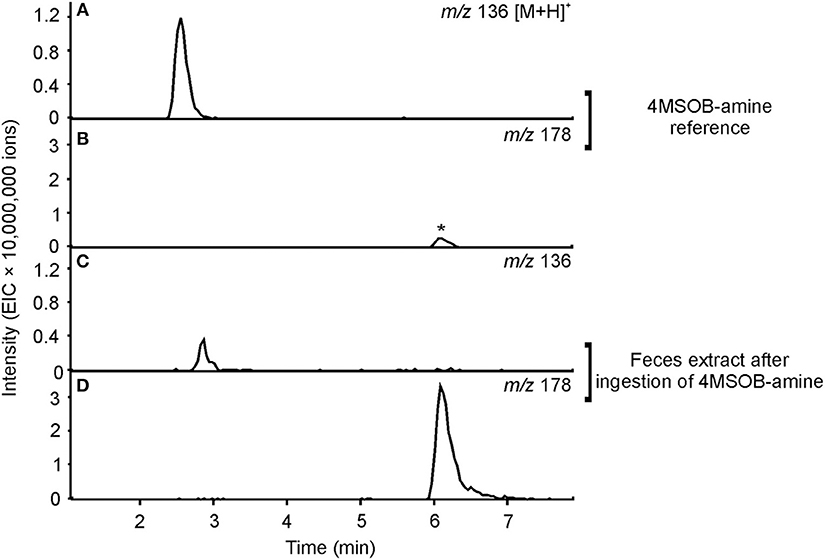
Figure 5. Metabolism of 4MSOB-amine in P. chrysocephala. Feces of adults which ingested 4MSOB-amine and subsequently fed on a detached leaf of the A. thaliana myb28 × myb29 mutant devoid of aliphatic GLS for 20 h were extracted with 0.1% (v/v) formic acid (pH 3). Feces extracts were analyzed by HPLC-MS (ion trap) in comparison to the 4MSOB-amine reference. Extracted ion chromatograms of 4MSOB-amine (m/z 136; A,C) and 4MSOB-acetamide (m/z 178; B,D) in positive ionization mode [M+H]+ are shown. A low amount of 4MSOB-acetamide was detected in the 4MSOB-amine reference (peak labeled with an asterisk).
Compound 2 showed a molecular ion peak of m/z 295.02459 ([M-H]−) which was consistent with the molecular formula C9H16O3N2S3 ((C9H16O3N2S3-H)− calcd m/z 295.025026). Tandem MS and NMR analyses revealed that compound 2 is a conjugate of 4MSOB-ITC which was assigned as 4-amino-3-(4-(methylsulfinyl)butyl)-2-thioxothiazolidine-4-carboxylic acid (4MSOB-ITC-Cyclic-Cys conjugate B; Supplementary Figure S3; Supplementary Table S4). During isolation by SPE and preparative HPLC, compound 2 was unstable and partially converted to compound 3, which indicated that both metabolites are related. Compound 3 showed a molecular ion peak of m/z 296.00901 ([M-H]−) which was consistent with the molecular formula C9H15O4NS3 ((C9H15O4NS3-H)− calcd m/z 296.00904). The structure of compound 3 was very similar to compound 2 and was assigned as 4-hydroxy-3-(4-(methylsulfinyl)butyl)-2-thioxothiazolidine-4-carboxylic acid (4MSOB-ITC-Cyclic-Cys conjugate C) according to LC-MS/MS and NMR analyses and by comparison to a chemically synthesized standard (Supplementary Figure S4; Supplementary Table S5). Mercaptopyruvic acid conjugates of benzenic ITCs were previously described in guinea-pigs, rabbits, and mice, and were shown to be derived from the ITC-Cys conjugate (Görler et al., 1982; Eklind et al., 1990). To test whether compounds 2 and 3 are derived from the ITC-Cys conjugate in P. chrysocephala, we performed a feeding experiment with the stable isotope labeled 13N-Cys conjugate of 4MSOB-ITC (m/z 301 [M-H]−). The labeled products were expected to have the same mass of the molecular ion (m/z 299 [M-H]−) because the 15N-labeled amino group at the C4-position in compound 2 is replaced with a hydroxyl group in compound 3 resulting in the loss of one labeled atom. Both 4MSOB-ITC conjugates were detected at the expected retention times of 7.1 min (compound 2) and 10.3 min (compound 3) with m/z 299 ([M-H]−) in feces extracts by LC-MS, confirming that they were derived from the labeled 4MSOB-ITC-Cys conjugate (Figure 6). These compounds were not detected in feces samples of beetles that ingested water as a control. Compounds 2 and 3 were thus named 4MSOB-ITC-Cyclic-Cys conjugate B and C, respectively (Figure 4).
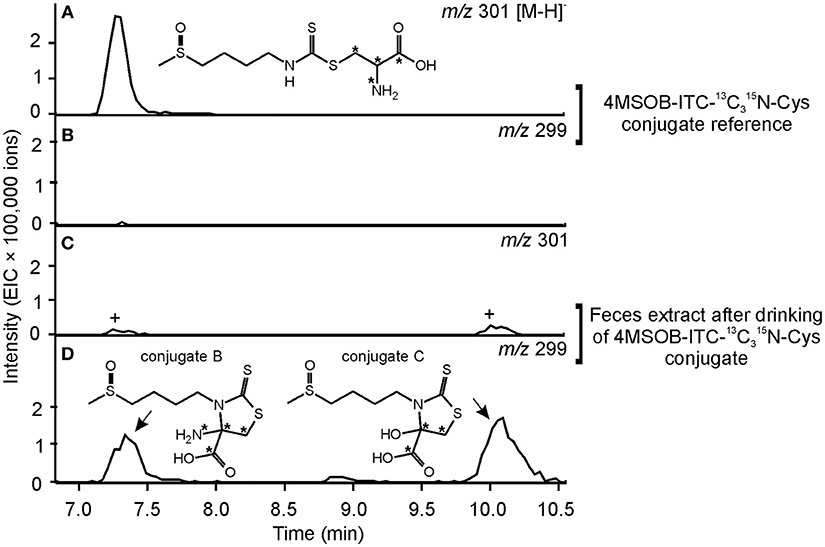
Figure 6. Metabolism of 4MSOB-ITC-13N-Cys in P. chrysocephala. Feces of adults which ingested 4MSOB-ITC-13N-Cys conjugate and subsequently fed on a detached leaf of the A. thaliana myb28 × myb29 mutant devoid of aliphatic GLS for 20 h were extracted with 0.1% (v/v) formic acid (pH 3). Feces extracts were analyzed by HPLC-MS (ion trap) in comparison to the 4MSOB-ITC-13C315N-Cys conjugate reference. Extracted ion chromatograms of 4MSOB-ITC-13C15N-Cys conjugate (m/z 301; A,C) and the labeled 4-amino-3-(4-(methylsulfinyl)butyl)-2-thioxothiazolidine-4-carboxylic acid (4MSOB-ITC-Cyclic-Cys conjugate B), and 4-hydroxy-3-(4-(methylsulfinyl)butyl)-2-thioxothiazolidine-4-carboxylic acid (4MSOB-ITC-Cyclic-Cys conjugate C) (m/z 299; B,D) in negative ionization mode [M–H]− are shown. 4MSOB-ITC-13N-Cys conjugate and 4MSOB-ITC-Cyclic-Cys conjugate B have the same retention time under the applied HPLC conditions. The peaks marked with “+” correspond to the 34S-isotopologues of the labeled 4MSOB-ITC-Cyclic-Cys conjugates B and C.
4MSOB-ITC-Cyclic-Cys conjugate B was not quantified because there was no synthetic standard available. The relative proportion of 4MSOB-ITC-Cyclic-Cys conjugate C in samples from the quantitative feeding experiment varied considerably and accounted for 11.6 ± 9.0% of the total ingested GLS (N = 6; Table 3).
All quantified derivatives of the mercapturic acid pathway together, including these novel metabolites, accounted for the metabolic fate of 17% of the total ingested GLS in our feeding experiment.
Discussion
The cabbage stem flea beetle, P. chrysocephala, is adapted to crucifer plants that are defended with the GLS-myrosinase system. Because feeding damage usually causes rapid enzymatic breakdown of GLS to toxic ITCs in the insect gut, we tested the hypothesis that P. chrysocephala can prevent GLS hydrolysis during feeding. Previously, we discovered a strong accumulation of GLS in the closely related flea beetle P. striolata, showing that adults at least partially prevent GLS activation in ingested plant tissue (Beran et al., 2014). The presence of GLS in all life stages of P. chrysocephala indicated a similar mechanism in this flea beetle species, but according to our quantitative analysis, less than 20% of the ingested 4MSOB GLS remained intact in adults. In addition, a minor proportion of 4MSOB GLS is metabolized by desulfation, a strategy that prevents enzymatic activation of GLS and requires activity of a sulfatase enzyme toward GLS. Such GLS sulfatase activity is known to be present in several specialist as well as generalist herbivores (Ratzka et al., 2002; Falk and Gershenzon, 2007; Opitz et al., 2011; Malka et al., 2016), but was not detected in P. striolata (Beran et al., 2014). Our results thus suggest that P. chrysocephala also possess GLS sulfatase activity. Compared to other adapted herbivores such as A. rosae and P. xylostella (Ratzka et al., 2002; Müller and Wittstock, 2005; Opitz et al., 2011; Jeschke et al., 2017), the proportion of sequestered and desulfated GLS in P. chrysocephala is low and thus does not prevent exposure to GLS hydrolysis products during feeding.
The ability to prevent GLS hydrolysis is not a prerequisite to feed or specialize on crucifers because herbivores may use other mechanisms to avoid ITC formation or toxicity. For example, cabbage white butterfly larvae excreted more than 90% of ingested 4MSOB GLS as the less toxic nitrile due to their gut-expressed NSP, whereas in other insects which do not possess endogenous NSP activity, 4MSOB GLS was mainly hydrolyzed to ITCs with only 9–15% nitrile (Gloss et al., 2014; Jeschke et al., 2017). In our quantitative study, the nitrile accounted for 9%, which also suggests the activation of a major proportion of ingested 4MSOB GLS (~65%) to ITCs. On the other hand, herbivory can induce the expression of NSP in rosette leaves of A. thaliana Col-0 leading to a higher percentage of the simple nitrile upon 4MSOB GLS hydrolysis (Burow et al., 2009). Indeed, free ITCs were present in low amounts (~2%), whereas conjugates of ITCs accounted for at least 17% of the metabolic fate of ingested GLS. This result confirms ITC formation and shows that P. chrysocephala metabolizes ITCs via the mercapturic acid pathway after conjugation to GSH. The conserved mercapturic pathway plays a key role in ITC metabolism for example in generalist lepidopteran larvae, but this detoxification strategy is associated with a high metabolic cost due to the loss of amino acids, in particular Cys (Schramm et al., 2012; Gloss et al., 2014; Jeschke et al., 2016, 2017).
In addition to the known GSH, CysGly, and Cys conjugates of 4MSOB-ITC, three different cyclic derivatives of Cys-conjugate were identified in P. chrysocephala. One of these (conjugate A) was previously detected in molluscs (Falk et al., 2014), whereas 4-amino-3-(4-(methylsulfinyl)butyl)-2-thioxothiazolidine-4-carboxylic acid (conjugate B) and 4-hydroxy-3-(4-(methylsulfinyl)butyl)-2-thioxothiazolidine-4-carboxylic acid (conjugate C; Figures 4, 6) are described for the first time. The formation of cyclic mercaptopyruvic acid conjugates (conjugate C) with other ITCs was previously reported in rodents (Görler et al., 1982; Eklind et al., 1990). The presence of the cyclic conjugates B and C in P. chrysocephala hints at a specific metabolism of ITCs in this beetle, because these conjugates were not detected in feeding studies with lepidopteran and dipteran herbivores using radiolabeled 4MSOB GLS (Gloss et al., 2014; Jeschke et al., 2017). Furthermore, the NAC conjugate of 4MSOB-ITC, a major product of ITC metabolism in S. flava (Gloss et al., 2014), was only found in traces in P. chrysocephala.
While the conversion of the ITC-Cys conjugate to the cyclic conjugate A can occur non-enzymatically (Kawakishi and Namiki, 1982), the formation of conjugates B and C is most likely enzymatic. Görler et al. proposed that the ITC-Cys conjugate is transaminated to the S-substituted mercaptopyruvate which cyclizes spontaneously following enolization to the thiazolidinethione (conjugate C). In P. chrysocephala, conjugate B may be formed by oxidation of the amino-group, and convert spontaneously to conjugate C as observed during the purification of conjugate B from feces extracts. It also remains an open question whether the cyclization enhances stability of the ITC conjugate compared to the thiocarbamoyl adduct which is known to be unstable under physiological conditions (Bruggeman et al., 1986; Nakamura et al., 2009).
We identified two other minor metabolites in P. chrysocephala that have so far not been reported as products of GLS metabolism in insects, i.e., 4MSOB-amine and its acetylated derivative 4MSOB-acetamide. By feeding adults with 4MSOB-amine, we demonstrated the conversion of 4MSOB-amine to 4MSOB-acetamide in vivo (Figure 5), but the precursor of 4MSOB-amine in P. chrysocephala is so far unknown. For example, amines may arise by non-enzymatic degradation of ITCs in aqueous solutions (Pechacek et al., 1997; Negrusz et al., 1998). In our experiments, 4MSOB-ITC remained stable in the extraction solvent in vitro, but might degrade to the amine in the beetle gut. In A. thaliana plants, the formation of indol-3-ylmethylamine depends on the conjugation of indol-3-ylmethyl-ITC to GSH and thus is a product of the mercapturic acid pathway (Bednarek et al., 2009; Piślewska-Bednarek et al., 2018). Furthermore, specific hydrolase enzymes may catalyze hydrolysis of ITCs to amines. Such an ITC hydrolase is for example present in a Pectobacterium strain that was isolated from the larval gut of the cabbage root fly, Delia radicum, where it possibly contributes to the detoxification of ITCs (Welte et al., 2016a,b). We currently investigate the possible role of the gut microbiome in ITC detoxification and formation of amines in P. chrysocephala.
Based on our results, it is unclear whether the conjugation to GSH represents the major detoxification strategy for dietary ITCs, because the metabolic fate of about 40% of the total ingested GLS remains unknown. Therefore, we cannot exclude the possibility that P. chrysocephala uses additional strategies to metabolize ITCs, nitriles, GLS, or desulfo-GLS. Feeding studies with radiolabeled GLS enable the identification of novel metabolites and are generally better suited to analyze the metabolic fate of ingested GLS because each compound can be quantified directly (Opitz et al., 2011; Gloss et al., 2014; Jeschke et al., 2017). Unfortunately, this approach failed with P. chrysocephala, because the amount of ingested radiolabeled 4MSOB GLS was too low. To clarify the importance of the mercapturic acid pathway in the detoxification of ITCs, we plan to manipulate this pathway via RNAi to analyze effects on ITC detoxification and fitness. Potential targets include glutathione S-transferases that catalyze the first step of the mercapturic acid pathway, although the conjugation of ITCs to GSH can also proceed non-enzymatically.
Independent of the fact that a relatively small proportion of ingested 4MSOB GLS was sequestered, the presence of GLS in all life stages of P. chrysocephala shows that this specialist is adapted to GLS. Both P. chrysocephala larvae and adults selectively sequester 2-hydroxy-3-butenyl GLS from their host plant B. rapa, but interestingly, the GLS composition of eggs differed from that of all other life stages and was more similar to that of B. rapa. For example, the proportion of indol-3-ylmethyl GLS in eggs was 42 times higher than in adults, suggesting an active transport mechanism for GLS into eggs. The presence of indolic GLS in eggs also demonstrates the uptake of indolic GLS from the gut into the body of adults, but nevertheless, GLS uptake may be selective and dependent on the GLS structure in P. chrysocephala. For example, the preferred uptake of 2-hydroxy-3-butenyl GLS compared to 3-butenyl GLS may lead to the observed GLS sequestration pattern in larvae and adults (Figure 2; Table 2). On the other hand, similar uptake rates for both GLS followed by enzymatic hydroxylation of 3-butenyl GLS would also change the relative proportions of both GLS. However, considering that 2-hydroxy-3-butenyl- and 3-butenyl GLS together account for less than 50% of the total GLS in B. rapa, but for up to 76% in P. chrysocephala adults (Table 2), enzymatic hydroxylation of 3-butenyl GLS alone would not explain the high proportion of 2-hydroxy-3-butenyl GLS in adults.
Interestingly, the flea beetle P. striolata selectively accumulates aliphatic GLS to ten-fold higher concentrations than P. chrysocephala (Beran et al., 2014), which indicates that the mechanism or efficiency of GLS uptake or turnover differs between both species. Moreover, P. striolata may use sequestered GLS for its defense against natural enemies as they express their own myrosinase (Beran et al., 2014), whereas enzyme assays with P. chrysocephala did not reveal endogenous myrosinase activity. Thus, the function of sequestered GLS in P. chrysocephala remains ambiguous.
Notably, the two flea beetle genera Phyllotreta and Psylliodes that use GLS-containing plants as hosts differ remarkably in their host plant range. While more or less all Phyllotreta species are specialized on crucifers, Psylliodes species are associated with host plants belonging to 27 different plant families (Jolivet and Hawkeswood, 1995). For example, about 65% of the middle European Psylliodes species feed on GLS-containing plants, while other species are specialized to feed on grasses or solanaceous plants that contain distinct defense compounds (Mohr, 1966). Thus, to answer the question how this complex GLS metabolism evolved in P. chrysocephala, a robust phylogeny of this genus and the subfamily Galerucinae is needed. Although some progress has been made on the phylogeny of the Galerucinae, the relationships at genus level are not well-resolved; yet, based on the available data it seems unlikely that Phyllotreta and Psylliodes are sister genera (Ge et al., 2012; Nie et al., 2018). Furthermore, the observed differences regarding GLS sequestration, insect myrosinase and GLS desulfation support the hypothesis that Phyllotreta and Psylliodes adapted independently from each other to GLS.
Conclusion
Most crucifer-specialist herbivores studied so far developed efficient strategies to prevent the breakdown of dietary GLS to reactive defense metabolites. Although the cabbage stem flea beetle selectively sequesters aliphatic GLS and additionally converts GLS to stable desulfo-GLS, this specialist does not avoid GLS hydrolysis completely. Instead, P. chrysocephala detoxifies ITCs by conjugation with glutathione via the conserved mercapturic acid pathway. From an evolutionary perspective, the utilization of a common detoxification pathway combined with specialized strategies preventing GLS hydrolysis is intriguing. Based on our results, we hypothesize that the ability to deactivate ITCs by conjugation with glutathione was a prerequisite to survive on crucifer plants, and subsequently enabled the evolution of GLS sequestration and detoxification mechanisms preventing ITC formation in crucifer-feeding Psylliodes species. At this background, we propose the genus Psylliodes as an excellent model to investigate molecular adaptations to chemical plant defenses that facilitate host shifts and thus drive the species diversification of herbivorous insects.
Author Contributions
FBer, CP, S-JA, SB, and MR conceived and designed the experiments. FBer, TS, CP, S-JA, FBet, AS, DV, SL, and MR performed the experiments. FBer, TS, CP, S-JA, DV, SB, SL, and MR analyzed the data. FBer and GK performed the statistical analysis. FBer wrote the manuscript. All authors commented on the manuscript.
Funding
This project was supported by the Max Planck Society and the International Max Planck Research School.
Conflict of Interest Statement
The authors declare that the research was conducted in the absence of any commercial or financial relationships that could be construed as a potential conflict of interest.
Acknowledgments
The authors thank the greenhouse team of the Max Planck Institute for plant cultivation, Susanne Donnerhacke for experimental help, and David G. Heckel and Jonathan Gershenzon for discussions.
Supplementary Material
The Supplementary Material for this article can be found online at: https://www.frontiersin.org/articles/10.3389/fpls.2018.01754/full#supplementary-material
References
Abdalsamee, M. K., Giampà, M., Niehaus, K., and Müller, C. (2014). Rapid incorporation of glucosinolates as a strategy used by a herbivore to prevent activation by myrosinases. Insect Biochem. Mol. Biol. 52, 115–123. doi: 10.1016/j.ibmb.2014.07.002
Agerbirk, N., and Olsen, C. E. (2012). Glucosinolate structures in evolution. Phytochemistry 77, 16–45. doi: 10.1016/j.phytochem.2012.02.005
Agrawal, A. A., and Kurashige, N. S. (2003). A role for isothiocyanates in plant resistance against the specialist herbivore Pieris rapae. J. Chem. Ecol. 29, 1403–1415. doi: 10.1023/A:1024265420375
Andréasson, E., and Jørgensen, L. B. (2003). Localization of plant myrosinases and glucosinolates. Integr. Phytochem. 37, 79–99. doi: 10.1016/S0079-9920(03)80019-9
Barth, C., and Jander, G. (2006). Arabidopsis myrosinases TGG1 and TGG2 have redundant function in glucosinolate breakdown and insect defense. Plant J. 46, 549–562. doi: 10.1111/j.1365-313X.2006.02716.x
Bartlet, E., Parsons, D., Williams, I. H., and Clark, S. J. (1994). The influence of glucosinolates and sugars on feeding by the cabbage stem flea beetle, Psylliodes chrysocephala. Entomol. Exp. Appl. 73, 77–83. doi: 10.1111/j.1570-7458.1994.tb01841.x
Bartlet, E., and Williams, I. H. (1991). Factors restricting the feeding of the cabbage stem flea beetle (Psylliodes chrysocephala). Entomol. Exp. Appl. 60, 233–238. doi: 10.1111/j.1570-7458.1991.tb01543.x
Bartlet, E., Williams, I. H., Blight, M. M., and Hick, A. J. (1992). “Responses of the oilseed rape pests, Ceutorhynchus assimilis and Psylliodes chrysocephala, to a mixture of isothiocyanates,” in Proceedings of the 8th International Symposium on Insect-Plant Relationships, eds. S. B. J. Menken, J. H. Visser, and P. Harrewijn (Dordrecht: Kluwer Academic Publishers), 103–104. doi: 10.1007/978-94-011-1654-1_29
Bednarek, P., Pislewska-Bednarek, M., Svatoš, A., Schneider, B., Doubsky, J., Mansurova, M., et al. (2009). A glucosinolate metabolism pathway in living plant cells mediates broad-spectrum antifungal defense. Science 323, 101–106. doi: 10.1126/science.1163732
Beran, F., Pauchet, Y., Kunert, G., Reichelt, M., Wielsch, N., Vogel, H., et al. (2014). Phyllotreta striolata flea beetles use host plant defense compounds to create their own glucosinolate-myrosinase system. Proc. Natl. Acad. Sci. U.S.A. 111, 7349–7354. doi: 10.1073/pnas.1321781111
Brown, K. K., and Hampton, M. B. (2011). Biological targets of isothiocyanates. Biochim. Biophys. Acta Gen. Subjects 1810, 888–894. doi: 10.1016/j.bbagen.2011.06.004
Bruggeman, I. M., Temmink, J. H. M., and Vanbladeren, P. J. (1986). Cytotoxicity of mercapturic acid pathway sulfur conjugates derived from benzyl and allyl isothiocyanate. Hum. Toxicol. 5, 139–139.
Burow, M., Losansky, A., Müller, R., Plock, A., Kliebenstein, D. J., and Wittstock, U. (2009). The genetic basis of constitutive and herbivore-induced ESP-independent nitrile formation in Arabidopsis. Plant Physiol. 149, 561–574. doi: 10.1104/pp.108.130732
Burow, M., Müller, R., Gershenzon, J., and Wittstock, U. (2006). Altered glucosinolate hydrolysis in genetically engineered Arabidopsis thaliana and its influence on the larval development of Spodoptera littoralis. J. Chem. Ecol. 32, 2333–2349. doi: 10.1007/s10886-006-9149-1
Burow, M., and Wittstock, U. (2009). Regulation and function of specifier proteins in plants. Phytochem. Rev. 8, 87–99. doi: 10.1007/s11101-008-9113-5
Edger, P. P., Heidel-Fischer, H. M., Bekaert, M., Rota, J., Gloeckner, G., Platts, A. E., et al. (2015). The butterfly plant arms-race escalated by gene and genome duplications. Proc. Natl. Acad. Sci. U.S.A. 112, 8362–8366. doi: 10.1073/pnas.1503926112
Eklind, K. I., Morse, M. A., and Chung, F. L. (1990). Distribution and metabolism of the natural anticarcinogen phenethyl isothiocyanate in A/J mice. Carcinogenesis 11, 2033–2036. doi: 10.1093/carcin/11.11.2033
Fahey, J. W., Zalcmann, A. T., and Talalay, P. (2001). The chemical diversity and distribution of glucosinolates and isothiocyanates among plants. Phytochemistry 56, 5–51. doi: 10.1016/S0031-9422(00)00316-2
Falk, K. L., and Gershenzon, J. (2007). The desert locust, Schistocerca gregaria, detoxifies the glucosinolates of Schouwia purpurea by desulfation. J. Chem. Ecol. 33, 1542–1555. doi: 10.1007/s10886-007-9331-0
Falk, K. L., Kästner, J., Bodenhausen, N., Schramm, K., Paetz, C., Vassão, D. G., et al. (2014). The role of glucosinolates and the jasmonic acid pathway in resistance of Arabidopsis thaliana against molluscan herbivores. Mol. Ecol. 23, 1188–1203. doi: 10.1111/mec.12610
Ge, D., Gómez-Zurita, J., Chesters, D., Yang, X., and Vogler, A. P. (2012). Suprageneric systematics of flea beetles (Chrysomelidae: Alticinae) inferred from multilocus sequence data. Mol. Phylogenet. Evol. 62, 793–805. doi: 10.1016/j.ympev.2011.11.028
Gloss, A. D., Vassão, D. G., Hailey, A. L., Dittrich, A. C. N., Schramm, K., Reichelt, M., et al. (2014). Evolution in an ancient detoxification pathway is coupled with a transition to herbivory in the Drosophilidae. Mol. Biol. Evol. 31, 2441–2456. doi: 10.1093/molbev/msu201
Godan, D. (1951). Über Nahrungs- und Brutpflanzen des Rapserdflohs (Psylliodes chrysocephala L.). Anzeiger für Schädlingskunde 24, 81–84. doi: 10.1007/BF02311555
Görler, K., Krumbiegel, G., Mennicke, W. H., and Siehl, H. U. (1982). The metabolism of benzyl isothiocyanate and its cysteine conjugate in guinea-pigs and rabbits. Xenobiotica 12, 535–542. doi: 10.3109/00498258209038932
Graser, G., Oldham, N. J., Brown, P. D., Temp, U., and Gershenzon, J. (2001). The biosynthesis of benzoic acid glucosinolate esters in Arabidopsis thaliana. Phytochemistry 57, 23–32. doi: 10.1016/S0031-9422(00)00501-X
Halkier, B. A., and Gershenzon, J. (2006). Biology and biochemistry of glucosinolates. Annu. Rev. Plant Biol. 57, 303–333. doi: 10.1146/annurev.arplant.57.032905.105228
Humphrey, P. T., Gloss, A. D., Alexandre, N. M., Villalobos, M. M., Fremgen, M. R., Groen, S. C., et al. (2016). Aversion and attraction to harmful plant secondary compounds jointly shape the foraging ecology of a specialist herbivore. Ecol. Evol. 6, 3256–3268. doi: 10.1002/ece3.2082
Jeschke, V., Gershenzon, J., and Vassão, D. G. (2015). “Metabolism of glucosinolates and their hydrolysis products in insect herbivores,” in Recent Advances in Phytochemistry, The Formation, Structure, and Activity of Phytochemicals ed R. Jetter (Heidelberg; New York, NY; Dordrecht; London: Springer International Publishing). 163–194. doi: 10.1007/978-3-319-20397-3
Jeschke, V., Gershenzon, J., and Vassão, D. G. (2016). A mode of action of glucosinolate-derived isothiocyanates: detoxification depletes glutathione and cysteine levels with ramifications on protein metabolism in Spodoptera littoralis. Insect Biochem. Mol. Biol. 71, 37–48. doi: 10.1016/j.ibmb.2016.02.002
Jeschke, V., Kearney, E. E., Schramm, K., Kunert, G., Shekhov, A., Gershenzon, J., et al. (2017). How glucosinolates affect generalist lepidopteran larvae: growth, development and glucosinolate metabolism. Front. Plant Sci. 8:1995. doi: 10.3389/fpls.2017.01995
Jolivet, P. H., and Hawkeswood, T. J. (1995). Host-Plants of Chrysomelidae of the World. Leiden: Backhuys Publishers.
Kassahun, K., Davis, M., Hu, P., Martin, B., and Baillie, T. (1997). Biotransformation of the naturally occurring isothiocyanate sulforaphane in the rat: identification of phase I metabolites and glutathione conjugates. Chem. Res. Toxicol. 10, 1228–1233. doi: 10.1021/tx970080t
Kawakishi, S., and Namiki, M. (1982). Oxidative cleavage of the disulfide bond of cystine by allyl isothiocyanate. J. Agric. Food Chem. 30, 618–620. doi: 10.1021/jf00111a056
Kazana, E., Pope, T. W., Tibbles, L., Bridges, M., Pickett, J. A., Bones, A. M., et al. (2007). The cabbage aphid: a walking mustard oil bomb. Proc. R. Soc. Lond. Series B Biol. Sci. 274, 2271–2277. doi: 10.1098/rspb.2007.0237
Kuchernig, J. C., Burow, M., and Wittstock, U. (2012). Evolution of specifier proteins in glucosinolate-containing plants. BMC Evol. Biol. 12:127. doi: 10.1186/1471-2148-12-127
Lambrix, V., Reichelt, M., Mitchell-Olds, T., Kliebenstein, D. J., and Gershenzon, J. (2001). The Arabidopsis epithiospecifier protein promotes the hydrolysis of glucosinolates to nitriles and influences Trichoplusia ni herbivory. Plant Cell 13, 2793–2807. doi: 10.1105/tpc.13.12.2793
Li, Q., Eigenbrode, S. D., Stringham, G. R., and Thiagarajah, M. R. (2000). Feeding and growth of Plutella xylostella and Spodoptera eridania on Brassica juncea with varying glucosinolate concentrations and myrosinase activities. J. Chem. Ecol. 26, 2401–2419. doi: 10.1023/A:1005535129399
Malka, O., Shekhov, A., Reichelt, M., Gershenzon, J., Vassão, D. G., and Morin, S. (2016). Glucosinolate desulfation by the phloem-feeding insect Bemisia tabaci. J. Chem. Ecol. 42, 230–235. doi: 10.1007/s10886-016-0675-1
Matile, P. (1980). The mustard oil bomb - compartmentation of the myrosinase system. Biochem. Physiol. Pflanzen 175, 722–731. doi: 10.1016/S0015-3796(80)80059-X
Mohr, K.-H. (1966). “Chrysomelidae,” in Die Käfer Mitteleuropas, eds H. Freude, K. W. Harde, and G. A. Lohse (Krefeld: Goecke und Evers Verlag Krefeld), 95–299.
Müller, C., and Wittstock, U. (2005). Uptake and turn-over of glucosinolates sequestered in the sawfly Athalia rosae. Insect Biochem. Mol. Biol. 35, 1189–1198. doi: 10.1016/j.ibmb.2005.06.001
Mumm, R., Burow, M., Bukovinszkine'kiss, G., Kazantzidou, E., Wittstock, U., Dicke, M., et al. (2008). Formation of simple nitriles upon glucosinolate hydrolysis affects direct and indirect defense against the specialist herbivore, Pieris rapae. J. Chem. Ecol. 34, 1311–1321. doi: 10.1007/s10886-008-9534-z
Nakamura, T., Kawai, Y., Kitamoto, N., Osawa, T., and Kato, Y. (2009). Covalent modification of lysine residues by allyl isothiocyanate in physiological conditions: Plausible transformation of isothiocyanate from thiol to amine. Chem. Res. Toxicol. 22, 536–542. doi: 10.1021/tx8003906
Negrusz, A., Moore, C. M., Mcdonagh, N. S., Woods, E. F., Crowell, J. A., and Levine, B. S. (1998). Determination of phenethylamine, a phenethyl isothiocyanate marker, in dog plasma using solid-phase extraction and gas chromatography-mass spectrometry with chemical ionization. J. Chromatogr. B Biomed. Sci. Appl. 718, 193–198. doi: 10.1016/S0378-4347(98)00354-5
Nie, R. E., Breeschoten, T., Timmermans, M. J. T. N., Nadein, K., Xue, H. J., Bai, M., et al. (2018). The phylogeny of Galerucinae (Coleoptera: Chrysomelidae) and the performance of mitochondrial genomes in phylogenetic inference compared to nuclear rRNA genes. Cladistics 34, 113–130. doi: 10.1111/cla.12196
Opitz, S. E. W., Mix, A., Winde, I. B., and Müller, C. (2011). Desulfation followed by sulfation: metabolism of benzylglucosinolate in Athalia rosae (Hymenoptera: Tenthredinidae). Chembiochem 12, 1252–1257. doi: 10.1002/cbic.201100053
Pechacek, R., Velisek, J., and Hrabcova, H. (1997). Decomposition products of allyl isothiocyanate in aqueous solutions. J. Agric. Food Chem. 45, 4584–4588. doi: 10.1021/jf970316z
Pinheiro, J., Bates, D., Debroy, S., Sarkar, D., and Team, R. C. (2017). nlme: Linear and Nonlinear Mixed Effects Models. R package version 3.1-131 [Online]. Available online at: https://CRAN.R-project.org/package=nlme
Piślewska-Bednarek, M., Nakano, R. T., Hiruma, K., Pastorczyk, M., Sanchez-Vallet, A., Singkaravanit-Ogawa, S., et al. (2018). Glutathione transferase U13 functions in pathogen-triggered glucosinolate metabolism. Plant Physiol. 176, 538–551. doi: 10.1104/pp,0.17.01455
R Core Team (2017). R: A Language and Environment for Statistical Computing. [Online]. Vienna, Austria: R Foundation for Statistical Computing. Available online at: https://www.R-project.org/
Ratzka, A., Vogel, H., Kliebenstein, D. J., Mitchell-Olds, T., and Kroymann, J. (2002). Disarming the mustard oil bomb. Proc. Natl. Acad. Sci. U.S.A. 99, 11223–11228. doi: 10.1073/pnas.172112899
Schindelin, J., Arganda-Carreras, I., Frise, E., Kaynig, V., Longair, M., Pietzsch, T., et al. (2012). Fiji: an open-source platform for biological-image analysis. Nat. Methods 9, 676–682. doi: 10.1038/nmeth.2019
Schramm, K., Vassão, D. G., Reichelt, M., Gershenzon, J., and Wittstock, U. (2012). Metabolism of glucosinolate-derived isothiocyanates to glutathione conjugates in generalist lepidopteran herbivores. Insect Biochem. Mol. Biol. 42, 174–182. doi: 10.1016/j.ibmb.2011.12.002
Sønderby, I. E., Hansen, B. G., Bjarnholt, N., Ticconi, C., Halkier, B. A., and Kliebenstein, D. J. (2007). A systems biology approach identifies a R2R3 MYB gene subfamily with distinct and overlapping functions in regulation of aliphatic glucosinolates. PLos ONE 2:e1322. doi: 10.1371/journal.pone.0001322
Song, L., Morrison, J. J., Botting, N. P., and Thornalley, P. J. (2005). Analysis of glucosinolates, isothiocyanates, and amine degradation products in vegetable extracts and blood plasma by LC-MS/MS. Anal. Biochem. 347, 234–243. doi: 10.1016/j.ab.2005.09.040
Thies, W. (1988). Isolation of sinigrin and glucotropaeolin from cruciferous seeds. Eur. J. Lipid Sci. Technol. 90, 311–314. doi: 10.1002/lipi.19880900806
Wathelet, J. P., Iori, R., Leoni, O., Rollin, P., Mabon, N., Marlier, M., et al. (2001). A recombinant beta-O-glucosidase from Caldocellum saccharolyticum to hydrolyse desulfo-glucosinolates. Biotechnol. Lett. 23, 443–446. doi: 10.1023/A:1010322322867
Welte, C. U., de Graaf, R. M., van den Bosch, T. J., Op den Camp, H. J., van Dam, N. M., and Jetten, M. S. (2016a). Plasmids from the gut microbiome of cabbage root fly larvae encode SaxA that catalyses the conversion of the plant toxin 2-phenylethyl isothiocyanate. Environ. Microbiol. 18, 1379–1390. doi: 10.1111/1462-2920.12997
Welte, C. U., Rosengarten, J. F., de Graaf, R. M., and Jetten, M. S. M. (2016b). SaxA-mediated isothiocyanate metabolism in phytopathogenic pectobacteria. Appl. Environ. Microbiol. 82, 2372–2379. doi: 10.1128/AEM.04054-15
Wheat, C. W., Vogel, H., Wittstock, U., Braby, M. F., Underwood, D., and Mitchell-Olds, T. (2007). The genetic basis of a plant-insect coevolutionary key innovation. Proc. Natl. Acad. Sci. U.S.A. 104, 20427–20431. doi: 10.1073/pnas.0706229104
Williams, I. H. (2010). “The major insect pests of oilseed rape in Europe and their management: an overview,” in Biocontrol-Based Integrated Management of Oilseed Rape Pests, ed I.H. Williams (Dordrecht; Heidelberg; London; New York, NY: Springer), 1–43. doi: 10.1007/978-90-481-3983-5
Winde, I., and Wittstock, U. (2011). Insect herbivore counteradaptations to the plant glucosinolate-myrosinase system. Phytochemistry 72, 1566–1575. doi: 10.1016/j.phytochem.2011.01.016
Wittstock, U., Agerbirk, N., Stauber, E. J., Olsen, C. E., Hippler, M., Mitchell-Olds, T., et al. (2004). Successful herbivore attack due to metabolic diversion of a plant chemical defense. Proc. Natl. Acad. Sci. U.S.A. 101, 4859–4864. doi: 10.1073/pnas.0308007101
Xia, J., and Wishart, D. S. (2011). Metabolomic data processing, analysis, and interpretation using MetaboAnalyst. Curr. Protoc. Bioinformatics 14:14.10. doi: 10.1002/0471250953.bi1410s34
Xia, J., and Wishart, D. S. (2016). Using MetaboAnalyst 3.0 for comprehensive metabolomics data analysis. Curr. Protoc. Bioinformatics 55, 14.10.1–14.10.91. doi: 10.1002/cpbi.11
Zimmer, C. T., Müller, A., Heimbach, U., and Nauen, R. (2014). Target-site resistance to pyrethroid insecticides in German populations of the cabbage stem flea beetle, Psylliodes chrysocephala L. (Coleoptera: Chrysomelidae). Pestic. Biochem. Physiol. 108, 1–7. doi: 10.1016/j.pestbp.2013.11.005
Keywords: Psylliodes chrysocephala, glucosinolate, sequestration, detoxification, cabbage stem flea beetle, isothiocyanate, glutathione
Citation: Beran F, Sporer T, Paetz C, Ahn S-J, Betzin F, Kunert G, Shekhov A, Vassão DG, Bartram S, Lorenz S and Reichelt M (2018) One Pathway Is Not Enough: The Cabbage Stem Flea Beetle Psylliodes chrysocephala Uses Multiple Strategies to Overcome the Glucosinolate-Myrosinase Defense in Its Host Plants. Front. Plant Sci. 9:1754. doi: 10.3389/fpls.2018.01754
Received: 26 September 2018; Accepted: 12 November 2018;
Published: 07 December 2018.
Edited by:
Dorothea Tholl, Virginia Tech, United StatesReviewed by:
Meike Burow, University of Copenhagen, DenmarkUte Wittstock, Technische Universitat Braunschweig, Germany
Copyright © 2018 Beran, Sporer, Paetz, Ahn, Betzin, Kunert, Shekhov, Vassão, Bartram, Lorenz and Reichelt. This is an open-access article distributed under the terms of the Creative Commons Attribution License (CC BY). The use, distribution or reproduction in other forums is permitted, provided the original author(s) and the copyright owner(s) are credited and that the original publication in this journal is cited, in accordance with accepted academic practice. No use, distribution or reproduction is permitted which does not comply with these terms.
*Correspondence: Franziska Beran, ZmJlcmFuQGljZS5tcGcuZGU=