- 1Leibniz-Institute of Vegetable and Ornamental Crops (IGZ), Großbeeren, Germany
- 2Agriculture Botany Department, Faculty of Agriculture, Alexandria University, Alexandria, Egypt
- 3Max Planck Institute for Chemical Ecology, Jena, Germany
How interactions between plants, the rhizosphere, and contaminated soil affect environmental sustainability is still under research. We tested the effects of two root endophytic fungi, the arbuscular mycorrhiza fungus (AMF) Rhizophagus irregularis and the beneficial endophyte Serendipita indica, on sweet basil (Ocimum basilicum) growing on soil contaminated with lead and copper in a pot experiment under defined greenhouse conditions. Both fungi caused an increase in shoot and root dry weight of sweet basil plants under all conditions and decreased the amount of lead in shoots. The amount of copper was reduced by S. indica, while the AM fungus showed this effect only when the soil is contaminated with both copper and lead. Furthermore the AMF, but not the endophyte S. indica caused a strong increase on the concentrations of the essential oils linalool and eucalyptol even on sweet basil growing on contaminated soils. Hence, cultivating sweet basil in combination with beneficial fungi in case of difficult environmental conditions could be of interest for industry located in countries with widespread land pollution, because quantity and quality of plants are increased while the amount of heavy metals is generally reduced.
Introduction
As a result of urbanization and industrialization, heavy metal pollution of the soil is a serious problem. It is especially important that soil pollution is kept at a low level that is below the mandatory toxicity limits, when cultivating food crops, or medicinal plants such as sweet basil. In particular, lead (Pb) pollution is a global problem due to its use as an additive in fertilizers and gasoline since the 1920s. On the one hand, there is a trend toward the protection of the environment from lead. But on the other hand, most sewage sludge and other fertilizers used in agriculture are still loaded with lead. Plant uptake is the most important gateway of heavy metals into the food chain and thus into the human body (Guerra et al., 2012). Although lead accumulated in soil is not readily absorbed by plant roots, studies show that it inhibits plant metabolic processes such as root growth, photosynthesis, and water uptake (Sharma and Dubey, 2005; Fahr et al., 2013). Thus, lead is still one of the main sources of environmental pollution and impacts agroecosystems, the environment, and, consequently, human health.
In contrast to lead, copper (Cu) is not easily accumulated in the food chain and thus the toxicity to humans and other mammals is relatively low. Additionally, copper is an essential element to plants and is needed for seed production, lignin synthesis, and the biosynthesis of different enzymes. However its concentrations are often elevated in the soil due to the widespread use of copper-containing fungicides, pesticides, and herbicides, especially in soils used for citrus and grapevine production (He et al., 2005). Plants are in general very sensitive to even slight increases in tissue copper contents. At high doses, copper inhibits root morphology, enhances reactive oxygen species (ROS) production in the plant, and induces the expression levels of genes related to an oxidative stress response (Rastogi et al., 2017).
The annual herb sweet basil (Ocimum basilicum, L.) is one of the best studied plants among the 150 Ocimum species. It belongs to the family of the Lamiaceae and is of high economic interest. It is mainly cultivated as a spice for the food industry, but also to use its essential oil in cosmetics, pharmaceuticals, and pesticides. The essential oil of sweet basil mostly consists of monoterpenes, sesquiterpenes, and phenylpropanoids with the monoterpene linalool and the phenylpropene methyl chavicol (Estragole) being the major compounds (Lee et al., 2005). Some aromatic plants are exclusively grown for the production of essential oils and Zheljazkov et al. (2006) showed that aromatic plants can be grown in heavy metal enriched soils without the risk of metal transfer into the oils.
Extensive previous research exists on different varieties and cultivars of sweet basil under the influence of beneficial fungi such as AMF, with respect to the biosynthesis and composition of essential oils, and the generation of biomass and plant fitness (Copetta et al., 2006; Toussaint et al., 2007; Zuccarini and Okurowska, 2008). AMF are particularly important to improve the quality of medicinal plants such as sweet basil. The symbionts can be used to enhance nutrient uptake and alter secondary metabolite content (Harrier, 2001). The AM fungus Rhizophagus irregularis (formerly known as Glomus intraradices (Krüger et al., 2012)) is widely used in research on AM symbiosis, in particular on how it supports plant fitness (Smith and Read, 2008). Going one step further, Battini et al. (2016) described the impact of dual inoculation with AMF and its associated bacteria and found an improvement of nutraceutical value of sweet basil. Nevertheless, little research has been initiated on the impact of beneficial fungi on sweet basil under heavy metal stress. However many investigations of the responses of other plants to heavy metal stress inoculated with AMF exist and different plant mechanisms, including the role of mycorrhizal fungi for heavy metal tolerance are surveyed by Hall (2002). Ingle et al. (2017) showed that AMF play an important role in the alleviation of cadmium stress in chickpea plants. In sweet basil, Prasad et al. (2011) discovered that R. irregularis enhance the metal concentration in shoot tissues grown in soil with a low dose of metals, whereas this effect was reciprocal in soil with a high amount of heavy metals.
In addition, the antioxidative plant defense to heavy metals is widely described and the protective impact of AMF in the case of heavy metal stress is also discussed in the literature (Schützendübel and Polle, 2002; Sytar et al., 2013; Shahid et al., 2017). Similarly, previous research exits on the response of AMF itself to heavy metals as well as its capability to decrease heavy metal stress in other plants. R. irregularis is able to grow in media with copper concentrations that are lethal to a majority of other organisms (Tamayo et al., 2014) and the effect of decreasing heavy metals in plants is an important aspect for the application of AMF in sustainable agricultural systems (Schreiner and Bethlenfalvay, 1995).
The mutualistic endophytic fungus Serendipita indica (formerly known as Piriformospora indica) is also widely used in plant-fungus interaction studies. This soil borne fungus originates from the Indian Thar desert and was initially described in Verma et al. (1998). It colonizes roots of diverse plant species and is known to promote growth and disease resistance in its hosts (Varma et al., 1999; Waller et al., 2005). S. indica alters the secondary metabolites of many plants of economic importance and, for example, promotes the uptake of iron and copper in the sugarcane ratoon crop (Varma et al., 2012). A recent study shows that S. indica is able to protect Oryza sativa from arsenic toxicity (Mohd et al., 2017).
In this study we used the two beneficial fungi, R. irregularis and S. indica, to investigate their interaction with sweet basil growing in Pb and Cu-contaminated soil. We analyzed sweet basil performance concerning biomass, P uptake, heavy metal accumulation and essential oil content. Because plants in natural or anthropogenic terrestrial ecosystems are usually not dealing with only one single factor at time, we choose a total factorial design for our study.
Materials and Methods
This experiment was carried out in the greenhouse at the Leibniz Institute for Vegetables and Ornamental Crops, Erfurt, Germany in 2015.
Experimental Design
A fully crossed-four factor design was chosen with three heavy metal treatments, two root colonizing fungi and controls. The AM fungus R. irregularis and the root-endophytic fungus S. indica were used. The treatment combinations were arranged in a randomized block design with 9 replicates.
Plant Cultivation
The seeds of O. basilicum were obtained from the Medicinal and Aromatic plants Department of the Agricultural Research center, Ministry of Agriculture, Cairo, Egypt. Seeds were germinated in specific peat for small plant cultivation (Pikiererde, Einheitserdewerke Werkverband e.V., Sinntal-Altengronau, Germany) at 25: 18°C (day: night) and irrigated three times a week with 65% holding capacity of water. Four weeks after sowing, single seedlings were transferred to pots (20 cm × 30 cm) filled with 2700 mL of substrate (50% peat and 50% autoclaved sand) and fertilized twice per month with 100 mL/plant low phosphorous mineral fertilizer (Flory 2, which contains 1% P, 15% N, 5% P2O2, 25% K2O, and 2% MgO, Germania Care GmbH, Germany).
Treatments
At transplanting, plants were treated either with R. irregularis, S. indica or both simultaneously. AM plants received viable propagules of R. irregularis (INOQ GmbH, Schnega, Germany) inoculum (sand contained about 220 mycorrhizal units/cm3) at 10% v/v mixed throughout the soil. One mycorrhizal unit is a spore, a hyphae or a mycorrhizal root fragment. Control plants were treated with the same amount of sand without any microorganisms in order to create the same soil conditions. S. indica was grown on PDA plates for 2 weeks (Carl Roth, Karlsruhe, Germany). A small hyphal plug was transferred in 500 mL conical flasks with liquid complete media (CM: 50 mL 20x salt solution (120 g NaNO3, 10.4 g KCl, 10.4 g MgSO4 × 7 H2O, and 30 g KH2PO4 in 1 L), 20 g glucose, 2 g peptone, 1 g yeast extract, 1 g casamine acids and 1 mL microelements (6 g MnCl2, 1.5 g H3BO3, 2.65 g ZnSO4 × 7 H2O, 750 mg KI, 2.4 mg NaMO4 × 2 H2O, 130 mg CuSO4 × 5 H2O dissolved in 1 L) and incubated for 3 weeks in the dark at 24°C. This liquid fungal culture was blended in a kitchen blender to obtain a mixture as homogeneous as possible. Afterward, this liquid suspension of S. indica hyphae and spores was used to soak plant roots of sweet basil. Control sweet basil seedling received the same amount of autoclaved (121°C for 20 min) S. indica suspension (mock treatment). The application of lead nitrate [Pb(NO3)2 × 7 H2O, Carl Roth, Karlsruhe, Germany] solution and/or copper sulfate (CuSO4 × 5 H2O, Carl Roth, Karlsruhe, Germany) solution to the potting substrate was conducted either separately for the single heavy metal treatment (400 mg/L) or in combination (200 mg/L each). An amount of 400 mg/L was chosen because higher amounts induced plant toxicity. Plants in the control group remained untreated. One week after transferring the seedlings to the pots and inoculating them with the fungi, the plants were irrigated every day with 100 ml of the stock solution (400 mg/L) containing the appropriate metal ions over a period of 10 days, to avoid a shock for the plants and to gradually achieve 400 mg total heavy metal amount in the soil. Thereafter plants were irrigated with tap water. The factorial design of the experiment resulted in total in 16 treatments (see Table 1), each with 9 replicates.
Harvest
The plants were harvested 5 weeks after transplanting and inoculating. This time period was chosen to keep the plants from flowering. Shoots and roots were separated and the plant height (cm), the shoot fresh weight (g/plant), and the number of leaves were measured. Aliquots of 300 mg root material were stored in 70% ethanol to evaluate the mycorrhization through microscopy and histology, and 100 mg of root material was frozen in liquid nitrogen to assess S. indica colonization with PCR. Shoots and roots dry weights were determined after drying in an oven at 65°C for 3 days. Samples were ground in a high speed rotor mill (Pulverisette 14, Fritsch, Idar-Oberstein, Germany) to measure lead, copper and oil content in shoots and roots and the phosphorus content in the shoots. Since oil values were compared to each other without the need of the absolute values, a potential loss of volatiles during the drying process was acceptable.
Histology, Microscopy and Detection of R. irregularis in Colonized Roots
Root samples were cut into small pieces of (1–2 cm), bleached in 10% KOH for 15 min at 90°C, washed with water, and submerged in 2N HCl for 2 min. Then they were stained with Trypan blue (0.05%) in lactoglycerol for 15 min at 90°C in a water bath and bleached in lactic acid according to Koske and Gemma (1989). Ten pieces were put on slides and observed under a light microscope with 200 times magnification. AM fungal structures (hyphae, arbuscular, and vesicles) were estimated quantitatively according to Biermann and Linderman (1981). For the histological sections, roots, dehydrated by increasing the ethanol concentrations from 70 to 99% and embedded in paraffin, were cut into 6–12 μm sections using a Leica RM2155 microtome (Leica, Wetzlar, Germany) and placed on a microscope slide. Furthermore, the staining method W-3A described by Wacker (2006) was used. The samples were stained with 1% acridine red for 5–15 min and washed 15 min with distilled water to stain the cell walls blue. The second staining was done with 1% acriflavine for 5–15 s and washed with distilled water for 5 s to stain the fungal hyphae yellow. The last staining was done with 2% astra blue for 30–180 s and then washed off completely for 10–20 s with distilled water to stain other parts of the cell wall red. For the staining solutions see: https://www.waldeck-ms.de/de/produktion/farbstoffe-und-farbstoffloesungen/. The samples were examined with an Olympus BX53 light microscope (Olympus, Tokio, Japan).
Phosphorus Concentration
Phosphorus (P) uptake was analyzed according to a modified method after Goehler and Drews (1978), Ehrenberger (1991), and Ghanem et al. (2014). Dried and pulverized randomly selected samples of shoot tissues (250 mg per sample) were subjected to an acid digestion (adding 2 mL of 65% HNO3 directly to the sample with 2 mL of 30% hydrogen peroxide and 5 mL H2O placed outside the sample vessel) at 200°C for 15 min in a microwave (μPREP-A microwave system, MLS GmbH, Leutkirch, Germany). The digested solution was diluted to 50 mL with deionized water. To an aliquot of 5 mL of the sample solution, 10 mL of vanadate–molybdate reagent (Sigma-Aldrich, Steinheim, Germany) was added. After 5 min incubation, P concentration was determined at 440 nm using a V-550 spectrophotometer (Jasco GmbH, Grossumstadt, Germany). Results were calculated in mg P × g-1 dry weight.
Determination of Lead (Pb) and Copper (Cu) Concentrations in Shoots and Roots
Dried and finely ground plant material from randomly selected plants (0.5 g) was digested with ten milliliters of concentrated HNO3 (ultrapure 65%) by incubating overnight at room temperature and heating for 4 h at 120°C. The temperature was then increased to 140°C until 1 mL of acid remained and samples were filtered after cooling. Pb and Cu concentrations were determined with atomic absorption spectrophotometry (Analyst 400 Perkin Elmer, England) (Jackson, 1967). Results were calculated in mg Pb or Cu, respectively × kg-1 dry weight.
Essential Oil Measurement
Linalool, methyl chavicol, eucalyptol, and eugenol were extracted from 50 mg of dry herb tissue with 600 μL of TBME (tetra butyl methyl ether) containing isobutylbenzene as internal standard (1.3 μg/μL). The extract was prepared by continuous shaking for 3 h, removed and washed with 500 μL of 0.1M (NH4)2CO3, pH 8.0. After centrifuging for 5 min at 17,000 g, the organic upper phase was passed through a Pasteur pipette, filled with 50 mg of anhydrous Na2SO4 and 50 mg of silica held in place with a plug of glass wool.
The extracted sample was analyzed by gas chromatography - mass spectrometry (GC-MS) to confirm peak identities and quantified with GC using flame ionization detection (FID). GC analysis was carried out with a Zebron ZB-5 capillary column (Phenomenex, 5% phenyl-95% dimethylpolysiloxane film, 30 m long × 0.25 mm id, 0.25 μm film thickness). Split less injection of 1 μl sample was performed using an injector temperature of 220°C. The instrument was programmed with an initial temperature of 40°C for 3 min, which was increased at a rate of 2°C/min up to 80°C. The temperature was then increased at a rate of 5°C/min to 160°C, following by a rate of 60°C/min up to 300°C. Hydrogen was used as carrier gas at a constant flow of 2 mL/min. The MS scan was set at 50 to 350 m/z, starting after a 5 min solvent delay. Ionization was done through an electron impact at the standard 70 eV. The mass selective detector transfer line heater was at 230°C, the quadrupole of the MS at 150°C and the MS source at 230°C. Compounds were identified by the comparison of their GC retention times and mass spectra with those of authentic standards. To quantify the compounds, a five point calibration was obtained with the authentic standard (Bosch et al., 2014).
RNA Isolation and PCR Analysis
Fresh root material was transferred directly into liquid nitrogen and stored at -80°C for the detection of S. indica activity by RT-PCR. Frozen material (100 mg) was ground with a tissue homogenizer (Precellys 24, VWR International GmbH, Darmstadt, Germany) at -12°C. Total RNA was extracted with the RNeasy plant Mini Kit from Qiagen (Qiagen, Hilden, Germany) according to manufacturer’s instructions, including the on-column-DNAse treatment for 30 min. The quality and quantity of the RNA was monitored with the NanoDrop 2000 (VWR International GmbH, Darmstadt, Germany). 500 ng of RNA was transcribed to cDNA in a final volume of 10 μl with the QuantiTect reverse transcription kit from Qiagen (Qiagen, Hilden, Germany) according to manufacturer’s instructions. Synthesized cDNA was used as a template for PCR to detect S. indica with ITS primers (forward: CAACACATGTGCACGTCGAT; reverse: CCAATGTGCATTCAGAACGA). As control, a fragment of the actin-encoding gene of O. basilicum was amplified [forward: AGATTCCTCCAGCAAATCTTTCTC; reverse: CTTTCTGGTGGAACAGCATCAA (Rastogi et al., 2014)].
Statistical Analysis
With the program package Statistica (version 12, Tulsa, OK, United States) an analysis of variance (ANOVA) was conducted. Tukey HSD (honestly significant difference) was performed at p ≤ 0.05 to detect significant interactions between factors while taking into account the fact that multiple comparisons are necessary due to the experimental design. Lead and copper treatment was considered as single factor (except for Figure 1), respectively and each S. indica and R. irregularis inoculation was also considered as a single factor, respectively. For detailed information see Supplementary Tables S1–S9. If no significant interaction between all factors was observed, the mean values were compared pairwise at p ≤ 0.05 with Student’s t-Test. All data are shown as mean values with standard errors.
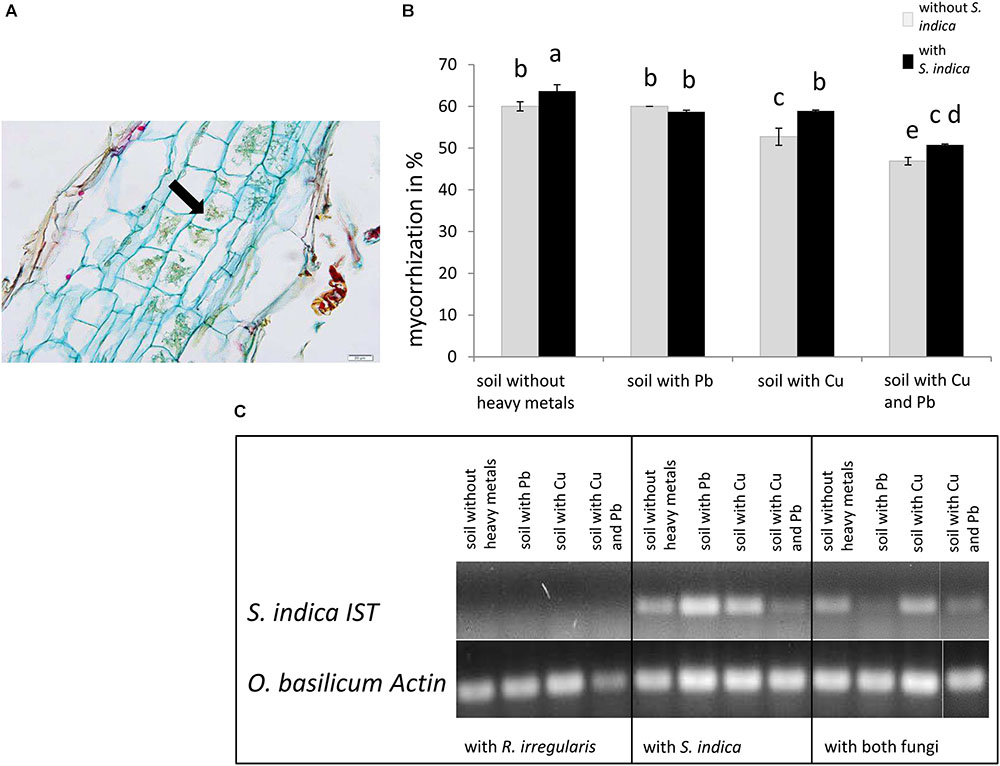
FIGURE 1. Colonization of sweet basil roots with Rhizophagus irregularis and Serendipita indica after 5 weeks of inoculation. (A) Histological section of a mycorrhizal sweet basil root stained with acridine red, acriflavine and astra blue. The arrow indicates cells with arbuscules. Scale bar: 20 μm. (B) Mycorrhizal colonization in %. Gray bars indicate sweet basil plants only inoculated with the AM fungus R. irregularis while black bars indicate plants inoculated with both, R. irregularis and S. indica. Data show means of three individual plants and ten 1 cm root segments per plant with standard error. Bars topped by the same letter do not differ significantly at p ≤ 0.05 according to Tukey’s HSD test (see Supplementary Table S1), where all factors do interact, calculating heavy metal treatment as one factor. (C) Qualitative detection of S. indica in sweet basil roots with PCR, using the ITS gene of S. indica. Representative samples are shown, which are either inoculated with R. irregularis or S. indica.
Results
Fungal Colonization
Mycorrhization was detected and quantified in the roots by staining, controls remain untreated and no colonization was observed (Figures 1A,B and data not shown). The highest level of mycorrhization was discovered in plants inoculated with both fungi on soil neither treated with copper nor with lead with 63% AM fungal structures in root pieces (Figure 1B). Only the simultaneous application of copper and lead significantly decreased mycorrhization. When both fungi were present, the mycorrhization decreased significantly in lead contaminated soil compared to normal soil. The lowest mycorrhizal colonization with only 46% was visible when only R. irregularis was present and the soil was contaminated with both heavy metals (Figure 1B). In general we found that mycorrhization increased with the presence of S. indica, except in lead contaminated soil. Furthermore the mycorrhization intensity decreased when the soil was contaminated with copper but not with lead.
Growth Parameters
In order to test the impact of the two fungi and heavy metal treatments on sweet basil growth, different growth parameters were measured 5 weeks after inoculation. These parameters were the plant height, the number of leaves per plant, the shoot fresh weight (Supplementary Figures S1, S2), and the shoot and root dry weight (Figure 2). No obvious symptoms (Figure 2A) and no change in dry matter were visible on the plant parts of sweet basil growing on heavy metal contaminated soil (Figures 2B,C). Within our 144 plants we saw only 5 plants randomly distributed in the treatments with obvious disease symptoms (data not shown). An overall trend was visible that both fungi promoted an increase in biomasses (Figure 2). More precisely, sweet basil shoot dry weights were not changing with heavy metal treatment, but were significantly increased if roots were colonized with either S. indica or with R. irregularis. Interestingly, the increase was not significant when both fungi were present, in case of the combined Cu and Pb treatment and no heavy metal treatment. However, both fungi increased fresh weight significantly, although less than for the single fungal treatment (Supplementary Figure S2). Even under heavy metal treatment, shoot dry weight was significantly increased when the plants were mycorrhizal with R. irregularis. In contrast, S. indica conferred a growth promotion only in uncontaminated soil and soil contaminated with lead (Figure 2B). When both fungi were present in heavy metal contaminated soil, the effect resembled the mycorrhization effect.
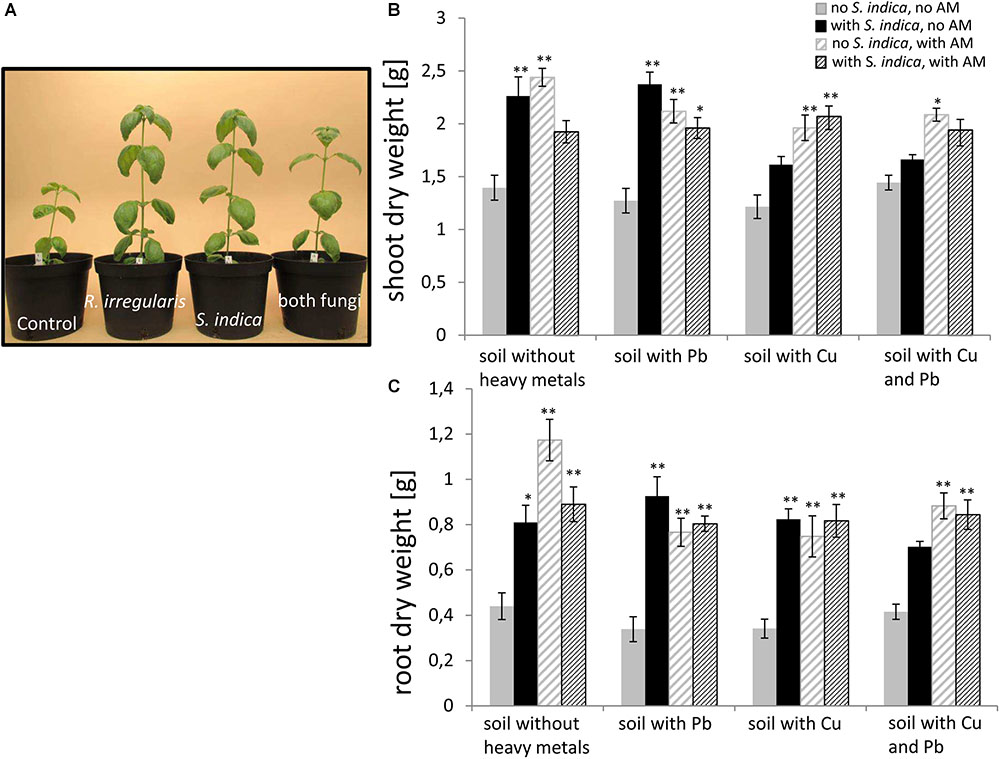
FIGURE 2. (A) Representative sweet basil plants inoculated with different fungi growing in uncontaminated soil. (B) Sweet basil shoot and (C) root dry weight after 5 weeks inoculation with the AM fungus R. irregularis, with S. indica, and with both fungi under different heavy metal treatments. Gray bars indicate non-inoculated sweet basil plants (control), black bars indicate plants inoculated only with S. indica, dashed gray bars indicate plants inoculated only with R. irregularis, and dashed black bars indicate plants inoculated with both fungi. Bars represent the mean out of nine individual plants with standard error. Asterisks indicate a significant difference compared to the respective non-inoculated control at the different heavy metal treatment according to Tukey’s HSD test (see Supplementary Table S2), ∗p ≤ 0.05, ∗∗p ≤ 0.001.
Root dry weight showed similar effects (Figure 2C); except that S. indica treated plants had significantly higher root dry weight also when the soil was contaminated with copper. Also, shoot fresh weight, plant height and number of leaves showed similar responses to heavy metals and root colonization with a few minor exceptions (Supplementary Figures S1, S2).
Phosphorus (P) Uptake
Since it is known that AMF increase the P uptake (P concentration [mg/g] × DW [g]) in plants, we measured the P concentration in the dried shoot material to confirm the mycorrhizal effect and to examine the effect of S. indica under the different heavy metal treatments. Mycorrhizal plants untreated with heavy metals showed a significant increase in P uptake (Figure 3). This effect was also visible when the soil was contaminated with copper but not when the soil was contaminated with lead or both heavy metals. Plants inoculated with S. indica showed a tendency to have a higher P uptake in uncontaminated soil and soil contaminated with lead or copper, respectively. When both fungi were present, the effect disappeared in non-contaminated soils, but resembled the mycorrhization effect, if heavy metals were present.
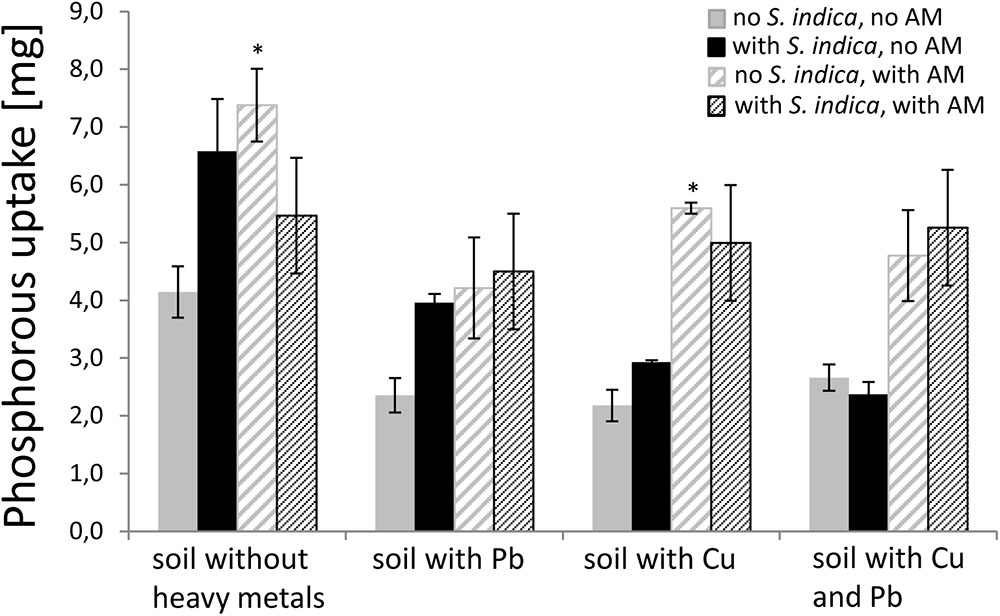
FIGURE 3. P uptake (P content × dry weight) in sweet basil shoots 5 weeks after inoculation with the AM fungus R. irregularis, with S. indica, and with both fungi under different heavy metal treatments. Bars are patterned as described for Figure 2. Asterisks indicate a significant difference compared to the respective non-inoculated control at the different heavy metal treatment according to Tukey’s HSD test (see Supplementary Table S3), ∗p ≤ 0.05
Lead and Copper Concentration in Shoots and Roots
No lead was found in the plants growing on uncontaminated soil and in the soil treated with copper. Less than 0.3 mg/kg copper was found in the plant material harvested from uncontaminated soil and in the soil treated with lead (data not shown).
The lead concentration in sweet basil shoots decreased significantly when the plants were colonized with R. irregularis or S. indica, with the lowest amount being measured when both fungi were present (Figure 4A). The lead concentration in the shoot decreased from 12,98 mg/kg without fungal inoculation to 6,32mg/kg when the plants were inoculated with both fungi. No differences were found in the lead concentrations in the roots. When the soil was contaminated with both heavy metals, fungal inoculations had a similar effect in the shoots, however plants colonized with both fungi did not show a lower value compared to the mycorrhizal plants. In contrast to the roots growing on lead contaminated soil, the roots on soil contaminated with both heavy metals showed an increase in lead concentration when inoculated with the fungi (Figure 4A).
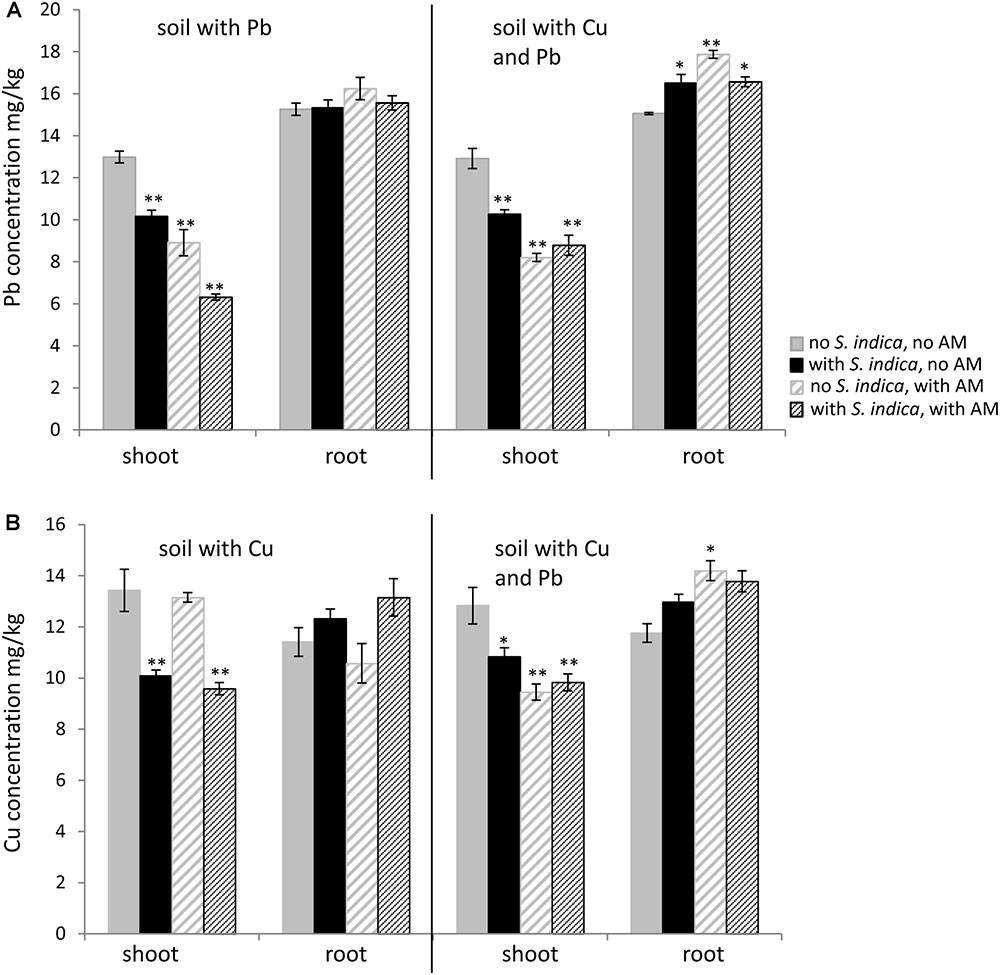
FIGURE 4. Lead (Pb) and copper (Cu) concentration in sweet basil roots and shoots 5 weeks after inoculation with the AM fungus R. irregularis, with S. indica and with both fungi under different heavy metal treatments. (A) Pb concentration in shoots and roots which grow on soil contaminated with Pb and both heavy metals, respectively. (B) Cu concentration in shoots and roots which grow on soil contaminated with Cu and both heavy metals, respectively. Bars are patterned as described for Figure 2 and represent the mean out of three plants with standard error. Asterisks indicate a significant difference compared to the respective non-inoculated control at the different heavy metal treatments according to Tukey’s HSD test (see Supplementary Tables S4, S5), ∗p ≤ 0.05, ∗∗p ≤ 0.001.
Interestingly the copper concentration in the shoots decreased only when the plants were inoculated with S. indica, not when they were only mycorrhizal (Figure 4B). In this case the copper concentration in the shoots remained stable at 13 mg/kg. Fungal treatments did not have any effect on the copper concentration in the roots when the soil was contaminated with copper. When both heavy metals were present in the soil, the copper concentration decreased in shoots for all fungal treatments (Figure 4B). The copper concentration in the roots increased significantly only when both heavy metals were present and when the roots were mycorrhizal.
Essential Oils in Sweet Basil
Linalool, eugenol, eucalyptol and methyl chavicol were measured by GC in sweet basil shoots. Remarkably, the linalool concentration was increased only when the plants were mycorrhizal. Linalool levels increased even when the soil was contaminated with either lead or copper, but not when both heavy metals were present (Figure 5A). The eugenol concentration was impaired when the roots were colonized with S. indica, but this effect was only significant when the soil was contaminated with lead. R. irregularis seemed to have no effect on the eugenol concentration (Figure 5B). The effect of mycorrhization on eucalyptol resembled the effect on linalool. It increased when the plants grow in uncontaminated soil and in soil containing lead or copper. But eucalyptol did not increase in mycorrhizal plants when both heavy metals were present in the soil (Figure 5C). The methylchavicol concentration was dramatically increased when the roots were mycorrhizal. This effect was only visible in uncontaminated soil and not in the soils contaminated with heavy metals (Figure 5D).
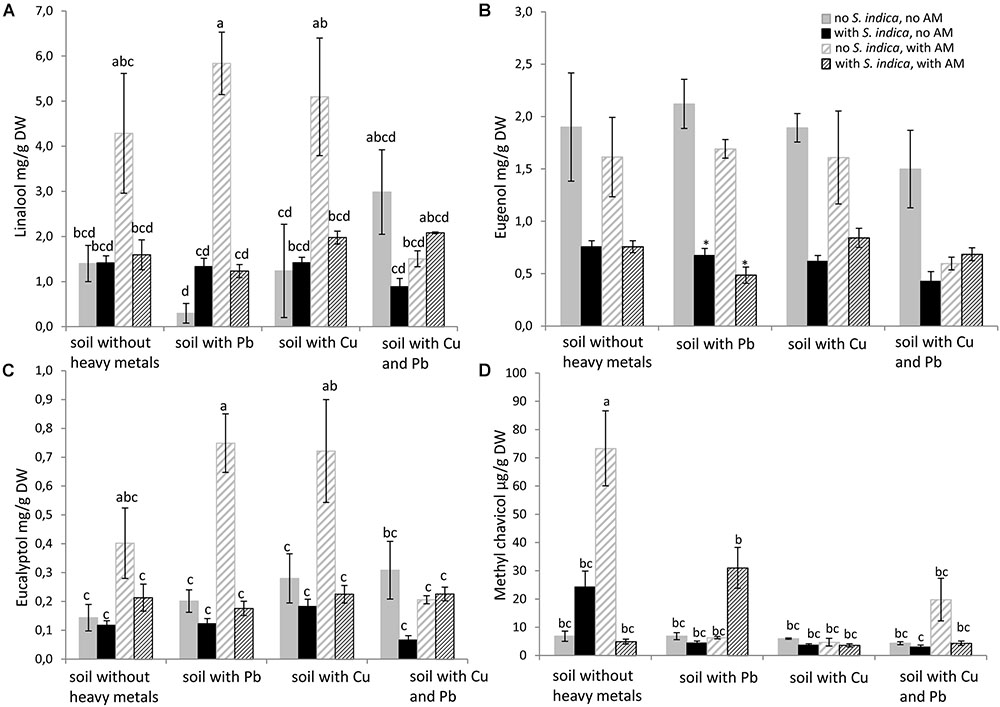
FIGURE 5. Essential oil concentration in sweet basil shoots 5 weeks after inoculation with the AM fungus R. irregularis, with S. indica and with both fungi under different heavy metal treatments. (A) Linalool concentration, (B) Eugenol concentration, (C) Eucalyptol concentration, (D) Methyl chavicol concentration. Bars are patterned as described for Figure 2 and represent the mean out of three plants with standard error. Letters indicate a significant difference at p ≤ 0.05 according to Tukey’s HSD test where all factors do interact. Asterisks indicate a significant difference compared to the respective non-inoculated control at the different heavy metal treatments according to Tukey’s HSD test (see Supplementary Tables S6, S7), ∗p ≤ 0.05.
Discussion
Fungal Colonization
The majority of vegetable and ornamental crops are host plants for AM fungi (AMF). Growth promotion of plants after inoculation with AMF is known for diverse species, including O. basilicum (Toussaint et al., 2008; Baum et al., 2015). The effect of AMF on O. basilicum is, however, species specific. Of the previously analyzed AMF Funneliformis mosseae, Gigaspora margarita and Gigaspora rosea, only F. mosseae induced significant increase in total biomass of O. basilicum plants (Copetta et al., 2006). Contributing to this literature, our study showed that sweet basil is a very good host for R. irregularis and hence the fungal inoculation might help producers to improve sweet basil growth and quality and support agricultural sustainability.
It is known that soil conditions and the presence of heavy metals negatively influence the interaction of beneficial fungi with plants (Yang et al., 2016). Prasad et al. (2011) found different mycorrhization levels in sweet basil due to the application of various heavy metals (Cr, Cd, Pb, and Ni) at different concentrations. Yet, lead had no influence on the mycorrhization level in sweet basil roots in our study, although we chose a high lead concentration. This might be due to the fact that R. irregularis seems to be less active in our experimental setup which leads to a reduced phosphorous uptake of the plant shown in Figure 3. In contrast, copper decreased the mycorrhization level to around 50%. Copper accumulation in the soil often originates from copper containing fungicides. These are used against pathogenic fungi of the aerial parts of the plant (Brunetto et al., 2016) which in turn might harm the microbial community in the soil.
Interestingly, the mycorrhization level increased with the simultaneous inoculation with S. indica in all treatments, except when lead was present in the soil (Figure 1). For tomato, Sartipnia et al. (2013) showed an increased activity of antioxidant enzymes after lead treatment. Such a defense response might influence colonization of beneficial fungi also in sweet basil. Additionally, Gill et al. (2016) reported a different colonization pattern of S. indica compared to AMF: They described a biphasic root colonization strategy with a biotrophic colonization pattern where an immune suppression was considered as a precondition for successful root colonization, although this is very host specific (Lahrmann et al., 2013; Gill et al., 2016). Hence we speculate that the S. indica-conferred immune suppression might enhance the colonization of AMF in our experiment. This is supported by the fact that wild lettuce inoculated with both mycorrhizal and endophytic fungi enjoyed more benefits than that with a single fungus in a polluted environment (Wazny et al., 2018). The mechanisms and degree of interaction and/or competition between R. irregularis and S. indica open new research opportunities for the future.
Growth Parameters, Lead and Copper Concentration in Plant Matter and Phosphorous Uptake
Growth Parameters
Although not always significant, there was a general trend of increased growth of sweet basil in the presence of the used fungi (Figure 2 and Supplementary Figures S1, S2). This is in agreement with other studies showing that beneficial fungi improve plant growth also in disturbed and polluted sites (Christie et al., 2004; Prasad et al., 2011).
Lead
Even though both fungi improve growth in shoots and roots when the soil is contaminated with lead, the increase in growth is lower for R. irregularis treated plants compared to plants treated with S. indica (Figure 2). Yang et al. (2016) also discovered a decreased growth promotion with different lead concentrations on mycorrhizal Robinia pseudoacacia. Interestingly, in our experiment, lead had no influence on the growth promoting effect of S. indica. This is in agreement with observations that barley plants inoculated with S. indica are more resistant to lead than the non-colonized controls (Karimi et al., 2011). Depending on the host plant, S. indica might be more suited for plant benefits than AM fungi when the soil is contaminated with lead.
It has been proposed that AM fungi suppress salicylic acid (SA) signaling during later stages of colonization allowing a biotrophic interaction (Cameron et al., 2013). In contrast, the early biotrophic stage of S. indica colonization is followed by a programmed cell death-associated later stage (Lahrmann and Zuccaro, 2012). This later stage might be accompanied by SA accumulation, and SA-regulated genes are induced by S. indica under drought stress (Zhang et al., 2018a). This difference during late stages of colonization might explain the better performance of S. indica-inoculated plants under lead stress, since an alleviative effect of SA has been shown when wheat seedlings were treated with 200 mg/L lead (Song et al., 2012). Furthermore S. indica might be able to recruit jasmonic acid signaling to counterbalance the salicylic acid defense pathway in Arabidopsis (Jacobs et al., 2011). Therefore it might be interesting to measure the activity of oxidative stress enzymes if they are altered like Song et al. (2012) did for wheat. Both fungi are important players when plants deal with oxidative stress (Schützendübel and Polle, 2002; Nath et al., 2016), but if and how this happens in our experimental setup needs further investigations.
Copper
S. indica was not able to significantly improve growth of the shoots of sweet basil when copper was present in the soil. For barley, Karimi et al. (2011) showed that the growth promoting effect was dependent on copper levels in the soil. Sweet basil inoculated with R. irregularis and grown in copper contaminated soil still showed a significant growth promotion on the shoots and the roots in our experiment (Figure 2). Plant biomass, root density, chlorophyll, carotenoid, and protein content significantly increased in Glycine max. with the inoculation of Penicillium funiculosum LHL06 to counteract copper stress by secreting bioactive gibberellins to bind the metal into stable complexes (Khan and Lee, 2013). In contrast it is known that gibberellins have a negative effect on arbuscule formation in pea and tomato (Foo et al., 2013; Martín-Rodríguez et al., 2016). Copper has no influence on sweet basil shoot dry weight in the presence of S. indica. In contrast to AM fungi, S. indica colonizes plant roots mainly extracellularly. We therefore hypothesize that copper, which can act as a fungicide, harms the fungus and thus limits its growth promoting effects to the shoot. This still needs to be verified by, for example, determining the colonization level of S. indica under different heavy metal stresses.
Heavy Metal Concentration
AMF colonizing plant roots were shown to reduce the uptake of heavy metals into plant cells (Hildebrandt et al., 2007). This can take place by changing the soil structure and chemical stabilization of metals in the soil (Gaur and Adholeya, 2004), decreasing the risk of toxicity to plants (Gonzalez-Chavez et al., 2009). In contrast, Dietterich et al. (2017) found no effects of AMF colonization on plant metal uptake, whereas a promotion of heavy metal uptake with AMF and S. indica was described for sunflower (Shahabivand et al., 2017; Zhang et al., 2018b). Furthermore, Park et al. (2016) showed that peat moss supports mobility and bioavailability of lead and copper. This indicates that the effect of AMF to heavy metal toxicity in plants is highly dependent on the plant host species, the fungal isolate and the soil structure.
Lead
For plants, it is known that most of the lead taken up remains in the roots (Kumar et al., 1995). In our experiment we saw that the lead concentration significantly decreased in the shoots but not in the roots inoculated with AMF (Figure 4). Different effects were described in the shoots for mycorrhizal plants growing on contaminated soil. Rydlová and Vosátka (2003) observed that mycorrhizal Agrostis capillaris took up more lead, whereas Yang et al. (2016) showed that different mycorrhizal plant species took up less lead when contamination is very high. For Origanum majorana it was shown that the lead content was reduced in shoots and roots when inoculated with the AM fungi F. mosseae, but lead content increased when infected with Claroideoglomus claroideum (Hristozkova et al., 2015). Surprisingly, in our experiment the lead concentration in the inoculated sweet basil roots increased, when both heavy metals were present in the soil. This effect was more significant in mycorrhizal roots than in roots inoculated with S. indica. The ability of S. indica to lower the pH in the surrounding media is also known for the rhizosphere in general (Lin et al., 2004; Ngwene et al., 2016). A decrease of the pH might lead to a higher absorption of lead, as shown for Oryza sativa (Lin et al., 2004). In contrast, the lead availability is reduced in the Pelargonium hortorum rhizosphere, when other heavy metals with antagonistic effects, such as copper, are present (Orroño et al., 2012). Since there are no results in the literature on the interaction between mycorrhizal fungi and S. indica, we can only speculate that in our experiments both fungi have an additive effect to decrease the uptake of lead by the shoots. Further investigations are needed to verify this conjecture.
Copper
Given our setup, the copper concentration in the sweet basil shoots decreased significantly when the roots are inoculated with S. indica but not when they are mycorrhizal. The exact mechanisms responsible for this difference are unknown at present. One explanation could be that some fungi are able to use avoidance mechanisms or compartmentalization strategies to detoxify metal cations (Meharg, 2003). The mycelia architecture of R. irregularis alters considerably under copper concentrations, at amounts lethal to other organisms (Tamayo et al., 2014; Ruscitti et al., 2017). Although Tamayo et al. (2014) revealed the presence of 30 metal transporters for copper, iron (Fe), and zinc (Zn) in R. irregularis, the exact function and purpose of these transporters in the R. irregularis membranes are unknown. Our results indicate that a comparison between the copper transporters in R. irregularis and S. indica might be helpful to explain the different effects of the two fungi on copper uptake by sweet basil.
Phosphorous Uptake
We showed that the mycorrhization effect on sweet basil roots was impaired by lead and not by copper (Figure 3). The ability of S. indica to support plant nutrition is still under investigation. Ngwene et al. (2016) found that S. indica is able to solubilize phosphate from inorganic phosphorus by lowering the pH, rather than an enzymatic effect. Our results show that S. indica impairs the mycorrhizal effect in the uncontaminated soil, which is in contradiction to our finding that plants inoculated with S. indica had a higher mycorrhizal colonization. Therefore this increase in mycorrhizal colonization may not have a biological relevance.
Essential Oil Concentrations
Many studies investigate the composition and biosynthesis of essential oils in sweet basil. We observed a significant interaction between the fungi and the heavy metal treatments on the linalool, eucalyptol and methyl chavicol concentrations (Figure 5). A clear increase in linalool and eucalyptol concentration was visible when the roots were inoculated with R. irregularis irrespective of the heavy metal treatment. Even though, the mechanisms are not very well studied, we suspect that the increase of certain essential oils in sweet basil inoculated with AMF might be due to increased nutrient uptake. However, Copetta et al. (2006) and Khaosaad et al. (2006) suggested that an altered P uptake is not be responsible for variations observed in the essential oil production in plants belonging to the Lamiaceae family. A possible explanation might be that methyl chavicol and eugenol belong to the phenylpropenes which have a different biosynthesis pathway than the terpenes linalool and eucalyptol. Prasad et al. (2011) found an increase in linalool content in the presence of lead, but methyl chavicol levels were unaffected in their study. Even though they examined O. basilicum in a pot experiment, differences in their experimental conditions such as soil structure, plant age and heavy metal content prevent direct comparison to our results. Moreover, Hristozkova et al. (2015) found that different isolates of F. mosseae and C. claroideum have changing effects on the composition of essential oils in O. majorana, supporting our hypothesis that the AM fungus is the active component affecting essential oil biosynthesis. Research on the ability of fungi to produce bioactive fungal metabolites is of high interest for the production of organic crops (Rai et al., 2014).
In contrast to our results, two studies showed that S. indica colonized herbs, such as fennel and Thymus vulgaris, harbor higher amounts of essential oil (Dolatabadi et al., 2011a,b). As we mentioned above, the effects of S. indica are very host dependent. Our results indicate that S. indica might not be suitable to increase the essential oil content in sweet basil. In fact, S. indica decreased the eugenol concentration.
Perspectives and Conclusion
A tremendous amount of literature is available on the role of phytohormones related to plant-microbe interactions. Results range from how mycorrhizal fungi develop and colonize plant roots – which in some cases are mutated in specific phytohormone pathways – to how this has an impact on plant fitness. The more research was done the more complex the situation became (Pieterse et al., 2014). There is also ample research on the effects of plant phytohormones to heavy metal stress (Ben Massoud et al., 2018). Our experimental setup has the potential to shed light on many of these processes if phytohormone levels and specific gene expression levels measurements are included. Using the resulting data checking a putative connection to the essential oil production of sweet basil seems worthwhile, as it is known that these secondary metabolites serve as defense and signal compounds. Furthermore, their biosynthesis is also regulated by phytohormones.
The industrially used herb O. basilicum represents a suitable host for both the beneficial fungi R. irregularis and S. indica. Both fungi have the ability to increase shoot and root weights after inoculation. Particularly, these increases were also visible in the presence of heavy metals in the soil. Linalool and Eucalyptol concentrations were dramatically changed in the presence of R. irregularis but not in the presence of S. indica. Furthermore both fungi protected sweet basil shoots from lead uptake, but only S. indica was able to protect the plants from copper uptake. For this reason, the use of beneficial fungi might be a helpful tool to lower heavy metal concentration in final agricultural products and to improve essential oil contents.
Author Contributions
MS, AA, and PF initiated the research. MS and PF designed the experiments. MS performed the experiments. IC directed the molecular work and supervised the execution of the experiments. EP and LW measured the essential oils. MS, PF, and IC analyzed the data. IC, MS, PF, and LW wrote the manuscript.
Funding
MS was supported by the Cultural Affairs and Missions Sector of the Ministry of Higher Education of Egypt. This work was funded by the Ministries of Consumer Protection, Food and Agriculture of the Federal Republic of Germany, of the States of Brandenburg and of Thüringen and by the Max Planck Society.
Conflict of Interest Statement
The authors declare that the research was conducted in the absence of any commercial or financial relationships that could be construed as a potential conflict of interest.
Acknowledgments
We thank Monika Graff and the greenhouse team for support and Katrin Schulz and Katja Krüger for excellent technical assistance.
Supplementary Material
The Supplementary Material for this article can be found online at: https://www.frontiersin.org/articles/10.3389/fpls.2018.01726/full#supplementary-material
References
Battini, F., Turrini, A., Sgherri, C., Malorgio, F., and Quartacci, M. F. (2016). Dual inoculation with AMF and associated bacteria improves nutraceutical value of sweet basil grown under commercial conditions. Agrochimica 60, 81–99. doi: 10.12871/0021857201623
Baum, C., El-Tohamy, W., and Gruda, N. (2015). Increasing the productivity and product quality of vegetable crops using arbuscular mycorrhizal fungi: A review. Sci Hortic 187, 131–141. doi: 10.1016/j.scienta.2015.03.002
Ben Massoud, M., Sakouhi, L., Karmous, I., Zhu, Y., El Ferjani, E., Sheehan, D., et al. (2018). Protective role of exogenous phytohormones on redox status in pea seedlings under copper stress. J. Plant Physiol. 221, 51–61. doi: 10.1016/j.jplph.2017.11.014
Biermann, B., and Linderman, R. G. (1981). Quantifying vesicular-arbuscular mycorrhizae: A proposed method towards standardization. New Phytol 87, 63–67. doi: 10.1111/j.1469-8137.1981.tb01690.x
Bosch, M., Wright, L. P., Gershenzon, J., Wasternack, C., Hause, B., Schaller, A., et al. (2014). Jasmonic acid and its precursor 12-oxophytodienoic acid control different aspects of constitutive and induced herbivore defenses in tomato. Plant Physiol. 166, 396–410. doi: 10.1104/pp.114.237388
Brunetto, G., Bastos, de Melo GW, Terzano, R., Del, Buono D, Astolfi, S., Tomasi, N., et al. (2016). Copper accumulation in vineyard soils: Rhizosphere processes and agronomic practices to limit its toxicity. Chemosphere 162, 293–307. doi: 10.1016/j.chemosphere.2016.07.104
Cameron, D. D., Neal, A. L., van Wees, S. C., and Ton, J. (2013). Mycorrhiza-induced resistance: more than the sum of its parts? Trends Plant Sci 18, 539–545. doi: 10.1016/j.tplants.2013.06.004
Christie, P., Li, X., and Chen, B. (2004). Arbuscular mycorrhiza can depress translocation of zinc to shoots of host plants in soils moderately polluted with zinc. Plant Soil 261, 209–217. doi: 10.1023/B:PLSO.0000035542.79345.1b
Copetta, A., Lingua, G., and Berta, G. (2006). Effects of three AM fungi on growth, distribution of glandular hairs, and essential oil production in Ocimum basilicum L. var. Genovese. Mycorrhiza 16, 485–494. doi: 10.1007/s00572-006-0065-6
Dietterich, L. H., Gonneau, C., and Casper, B. B. (2017). Arbuscular mycorrhizal colonization has little consequence for plant heavy metal uptake in contaminated field soils. Ecol Appl 27, 1862–1875. doi: 10.1002/eap.1573
Dolatabadi, H. K., Goltapeh, E. M., Jaimand, K., Rohani, N., and Varma, A. (2011a). Effects of Piriformospora indica and Sebacina vermifera on growth and yield of essential oil in fennel (Foeniculum vulgare) under greenhouse conditions. J. Basic Microbiol. 51, 33–39. doi: 10.1002/jobm.201000214
Dolatabadi, H. K., Goltapeh, E. M., Moieni, A., Jaimand, K., Sardrood, B. P., and Varma, A. (2011b). Effect of Piriformospora indica and Sebacina vermifera on plant growth and essential oil yield in Thymus vulgaris in vitro and in vivo experiments. Symbiosis 53, 29–35. doi: 10.1007/s13199-010-0104-0
Ehrenberger, F. (1991). Quantitative organische Elementaranalyse. VCH Verlagsgesellschaft, Weinheim 103, 1555–1555. doi: 10.1002/ange.19911031144
Fahr, M., Laplaze, L., Bendaou, N., Hocher, V., El Mzibri, M., Bogusz, D., et al. (2013). Effect of lead on root growth. Front Plant Sci 4:175. doi: 10.3389/fpls.2013.00175
Foo, E., Ross, J. J., Jones, W. T., and Reid, J. B. (2013). Plant hormones in arbuscular mycorrhizal symbioses: an emerging role for gibberellins. Ann. Bot. 111, 769–779. doi: 10.1093/aob/mct041
Gaur, A., and Adholeya, A. (2004). Prospects of arbuscular mycorrhizal fungi in phytoremediation of heavy metal contaminated soils. Curr Sci India 86, 528–534. doi: 10.1007/s10653-008-9159-8
Ghanem, G., Ewald, A., Zerche, S., and Hennig, F. (2014). Effect of root colonization with Piriformospora indica and phosphate availability on the growth and reproductive biology of a Cyclamen persicum cultivar. Sci Hortic 172, 233–241. doi: 10.1016/j.scienta.2014.04.022
Gill, S. S., Gill, R., Trivedi, D. K., Anjum, N. A., Sharma, K. K., Ansari, M. W., et al. (2016). Piriformospora indica: Potential and significance in plant stress tolerance. Front Microbiol 7:332. doi: 10.3389/fmicb.2016.00332
Goehler, F., and Drews, M. (1978). Che∗mische Betriebslaboratorien in Gewächshausanlagen. Iga Ratgeber. Akademie der Landwirtschaftswissenschaften der DDR 1–54.
Gonzalez-Chavez, M. C., Carrillo-Gonzalez, R., and Gutierrez-Castorena, M. C. (2009). Natural attenuation in a slag heap contaminated with cadmium: the role of plants and arbuscular mycorrhizal fungi. J. Hazard. Mater. 161, 1288–1298. doi: 10.1016/j.jhazmat.2008.04.110
Guerra, F., Trevizam, A. R., Muraoka, T., Marcante, N. C., and Canniatti-Brazaca, S. G. (2012). Heavy metals in vegetables and potential risk for human health. Sci Agric 69, 54–60. doi: 10.1590/S0103-90162012000100008
Hall, J. L. (2002). Cellular mechanisms for heavy metal detoxification and tolerance. J. Exp. Bot. 53, 1–11. doi: 10.1093/jexbot/53.366.1
Harrier, L. A. (2001). The arbuscular mycorrhizal symbiosis: A molecular review of the fungal dimension. J. Exp. Bot. 52, 469–478. doi: 10.1093/jexbot/52.suppl_l.469
He, Z. L., Yang, X. E., and Stoffella, P. J. (2005). Trace elements in agroecosystems and impacts on the environment. J. Trace Elem. Med. Biol. 19, 125–140. doi: 10.1016/j.jtemb.2005.02.010
Hildebrandt, U., Regvar, M., and Bothe, H. (2007). Arbuscular mycorrhiza and heavy metal tolerance. Phytochemistry 68, 139–146. doi: 10.1016/j.phytochem.2006.09.023
Hristozkova, M., Geneva, M., Stancheva, I., Boychinova, M., and Djonova, E. (2015). Aspects of mycorrhizal colonization in adaptation of sweet marjoram (Origanum majorana L.) grown on industrially polluted soil. Turk J Biol 39, 461–468. doi: 10.3906/biy-1408-47
Ingle, A. P., Rathod, D., Brestic, M., Kalaji, H. M., and Rai, M. (2017). “Biophysical phenotyping as an essential tool for understanding host–microbe interaction,” in Modern Tools and Techniques to Understand Microbes, eds A. Varma and A. K. Sharma (Berlin: Springer International Publishing), 65–80. doi: 10.1007/978-3-319-49197-4_4
Jacobs, S., Zechmann, B., Molitor, A., Trujillo, M., Petutschnig, E., Lipka, V., et al. (2011). Broad-spectrum suppression of innate immunity is required for colonization of Arabidopsis roots by the fungus Piriformospora indica. Plant Physiol. 156, 726–740. doi: 10.1104/pp.111.176446
Karimi, F., Sepehri, M., Afyuni, M., and Hajabbasi, M. A. (2011). “Role of Piriformospora indica in barley (Hordeum vulgare L.) resistance to cadmium, lead and copper,” in Proceedings of the 12th International Conference on Environmental Science and Technology, (Rhodes).
Khan, A. L., and Lee, I.-J. (2013). Endophytic Penicillium funiculosum LHL06 secretes gibberellin that reprograms Glycine max L. growth during copper stress. BMC Plant Biol 13:86. doi: 10.1186/1471-2229-13-86
Khaosaad, T., Vierheilig, H., Nell, M., Zitterl-Eglseer, K., and Novak, J. (2006). Arbuscular mycorrhiza alter the concentration of essential oils in oregano (Origanum sp. Lamiaceae). Mycorrhiza 16, 443–446. doi: 10.1007/s00572-006-0062-9
Koske, R. E., and Gemma, J. N. (1989). A modified procedure for staining roots to detect VA mycorrhizas. Mycol. Res. 92, 486–488. doi: 10.1016/S0953-7562(89)80195-9
Krüger, M., Krüger, C., Walker, C., Stockinger, H., and Schüßler, A. (2012). Phylogenetic reference data for systematics and phylotaxonomy of arbuscular mycorrhizal fungi from phylum to species level. New Phytol 193, 970–984. doi: 10.1111/j.1469-8137.2011.03962.x
Kumar, P., Dushenkov, V., Motto, H., and Raskin, I. (1995). Phytoextraction - the use of plants to remove heavy-metals from soils. Environ. Sci. Technol. 29, 1232–1238. doi: 10.1021/es00005a014
Lahrmann, U., Ding, Y., Banhara, A., Rath, M., Hajirezaei, M. R., Döhlemann, S., et al. (2013). Host-related metabolic cues affect colonization strategies of a root endophyte. Proc. Natl. Acad. Sci. U.S.A. 110, 13965–13970. doi: 10.1073/pnas.1301653110
Lahrmann, U., and Zuccaro, A. (2012). Opprimo ergo sum-evasion and suppression in the root endophytic fungus Piriformospora indica. Mol. Plant Microbe Interact. 25, 727–737. doi: 10.1094/MPMI-11-11-0291
Lee, S.-J., Umano, K., Shibamoto, T., and Lee, K.-G. (2005). Identification of volatile components in basil (Ocimum basilicum L.) and thyme leaves (Thymus vulgaris L.) and their antioxidant properties. Food Chem 91, 131–137. doi: 10.1016/j.foodchem.2004.05.056
Lin, Q., Chen, Y. X., He, Y. F., and Tian, G. M. (2004). Root-induced changes of lead availability in the rhizosphere of Oryza sativa L. Agric, Ecosyst Environ 104, 605–613. doi: 10.1016/j.agee.2004.01.001
Martín-Rodríguez, J. A., Huertas, R., Ho-Plágaro, T., Ocampo, J. A., Turečková, V., Tarkowská, D., et al. (2016). Gibberellin-abscisic acid balances during arbuscular mycorrhiza formation in tomato. Front Plant Sci 7:1273. doi: 10.3389/fpls.2016.01273
Meharg, A. A. (2003). The mechanistic basis of interactions between mycorrhizal associations and toxic metal cations. Mycol. Res. 107, 1253–1265. doi: 10.1017/S0953756203008608
Mohd, S., Shukla, J., Kushwaha, A. S., Mandrah, K., Shankar, J., Arjaria, N., et al. (2017). Endophytic fungi Piriformospora indica mediated protection of host from arsenic toxicity. Frontiers in Microbiology 8:754. doi: 10.3389/fmicb.2017.00754
Nath, M., Bhatt, D., Prasad, R., Gill, S. S., Anjum, N. A., and Tuteja, N. (2016). Reactive oxygen species generation-scavenging and signaling during plant-arbuscular mycorrhizal and Piriformospora indica interaction under stress condition. Front Plant Sci 7:1574. doi: 10.3389/fpls.2016.01574
Ngwene, B., Boukail, S., Söllner, L., Franken, P., and Andrade-Linares, D. R. (2016). Phosphate utilization by the fungal root endophyte Piriformospora indica. Plant Soil 405, 231–241. doi: 10.1007/s11104-015-2779-8
Orroño, D. I., Schindler, V., and Lavado, R. S. (2012). Heavy metal availability in Pelargonium hortorum rhizosphere: Interactions, uptake and plant accumulation. J Plant Nutr 35, 1374–1386. doi: 10.1080/01904167.2012.684129
Park, J. H., Lee, S. J., Lee, M. E., and Chung, J. W. (2016). Comparison of heavy metal immobilization in contaminated soils amended with peat moss and peat moss-derived biochar. Environmental science Processes & impacts 18, 514–520. doi: 10.1039/c6em00098c
Pieterse, C. M., Zamioudis, C., Berendsen, R. L., Weller, D. M., Van Wees, S. C., and Bakker, P. A. (2014). Induced systemic resistance by beneficial microbes. Annu. Rev. Phytopathol. 52, 347–375. doi: 10.1146/annurev-phyto-082712-102340
Prasad, A., Kumar, S., Khaliq, A., and Pandey, A. (2011). Heavy metals and arbuscular mycorrhizal (AM) fungi can alter the yield and chemical composition of volatile oil of sweet basil (Ocimum basilicum L.). Biol Fertility Soils 47, 853. doi: 10.1007/s00374-011-0590-0
Rai, M., Rathod, D., Agarkar, G., Dar, M., Brestic, M., Pastore, G. M., et al. (2014). Fungal growth promotor endophytes: a pragmatic approach towards sustainable food and agriculture. Symbiosis 62, 63–79. doi: 10.1007/s13199-014-0273-3
Rastogi, A., Zivcak, M., Sytar, O., Kalaji, H. M., He, X., Mbarki, S., et al. (2017). Impact of metal and metal oxide nanoparticles on plant: A critical review. Frontiers in chemistry 5:78. doi: 10.3389/fchem.2017.00078
Rastogi, S., Meena, S., Bhattacharya, A., Ghosh, S., Shukla, R. K., Sangwan, N. S., et al. (2014). De novo sequencing and comparative analysis of holy and sweet basil transcriptomes. BMC Genomics 15:588. doi: 10.1186/1471-2164-15-588
Ruscitti, M., Arango, M., and Beltrano, J. (2017). Improvement of copper stress tolerance in pepper plants (Capsicum annuum L.) by inoculation with arbuscular mycorrhizal fungi. Theor Exp Plant Physiol 29, 37–49. doi: 10.1007/s40626-016-0081-7
Rydlová, J., and Vosátka, M. (2003). Effect of Glomus intraradices isolated from Pb-contaminated soil on Pb uptake by Agrostis capillaris is changed by its cultivation in a metal-free substrate. Folia Geobot 38, 155–165. doi: 10.1007/BF02803148
Sartipnia, N., Khavari-Nejad, R.-A., Babaeizad, V., Nejad-Sattari, T., and Najafi, F. (2013). Effect of Piriformospora indica on antioxidant enzymes activity of tomato (Lycopersicon esculentum Mill) under lead stress. Int J Biosci 3, 55–64. doi: 10.12692/ijb/3.12.55-64
Schreiner, R. P., and Bethlenfalvay, G. J. (1995). Mycorrhizal interactions in sustainable agriculture. Crit. Rev. Biotechnol 15, 271–285. doi: 10.3109/07388559509147413
Schützendübel, A., and Polle, A. (2002). Plant responses to abiotic stresses: heavy metal-induced oxidative stress and protection by mycorrhization. J. Exp. Bot. 53, 1351–1365. doi: 10.1093/jexbot/53.372.1351
Shahabivand, S., Parvaneh, A., and Aliloo, A. A. (2017). Root endophytic fungus Piriformospora indica affected growth, cadmium partitioning and chlorophyll fluorescence of sunflower under cadmium toxicity. Ecotoxicol. Environ. Saf 145, 496–502. doi: 10.1016/j.ecoenv.2017.07.064
Shahid, M., Shamshad, S., Rafiq, M., Khalid, S., Bibi, I., Niazi, N. K., et al. (2017). Chromium speciation, bioavailability, uptake, toxicity and detoxification in soil-plant system: A review. Chemosphere 178, 513–533. doi: 10.1016/j.chemosphere.2.017.03.074
Sharma, P., and Dubey, R. S. (2005). Lead toxicity in plants. Braz J Plant Physiol 17, 35–52. doi: 10.1590/S1677-04202005000100004
Song, W. Y., Zheng, A. Z., Shao, H. B., Chu, L. Y., Brestic, M., and Zhang, Z. B. (2012). The alleviative effect of salicylic acid on the physiological indices of the seedling leaves in six different wheat genotypes under lead stress. Plant Omics 5, 486–493. doi: 10.1002/clen.201200310
Sytar, O., Kumar, A., Latowski, D., Kuczynska, P., Strzalka, K., and Prasad, M. N. V. (2013). Heavy metal-induced oxidative damage, defense reactions, and detoxification mechanisms in plants. Acta Physiol Plant 35, 985–999. doi: 10.1007/s11738-012-1169-6
Tamayo, E., Gómez-Gallego, T., Azcón-Aguilar, C., and Ferrol, N. (2014). Genome-wide analysis of copper, iron and zinc transporters in the arbuscular mycorrhizal fungus Rhizophagus irregularis. Front Plant Sci 5:547. doi: 10.3389/fpls.2014.00547
Toussaint, J. P., Kraml, M., Nell, M., Smith, S. E., Smith, F. A., Steinkellner, S., et al. (2008). Effect of Glomus mosseae on concentrations of rosmarinic and caffeic acids and essential oil compounds in basil inoculated with Fusarium oxysporum f.sp. basilici. Plant Pathol 57, 1109–1116. doi: 10.1111/j.1365-3059.2008.01895.x
Toussaint, J. P., Smith, F. A., and Smith, S. E. (2007). Arbuscular mycorrhizal fungi can induce the production of phytochemicals in sweet basil irrespective of phosphorus nutrition. Mycorrhiza 17, 291–297. doi: 10.1007/s00572-006-0104-3
Varma, A., Savita, V., Sudha., Sahay, N., Bütehorn, B., and Franken, P. (1999). Piriformospora indica, a cultivable plant-growth-promoting root endophyte. Appl. Environ. Microbiol. 65, 2741–2744.
Varma, A., Sherameti, I., Tripathi, S., et al. (2012). “13 The Symbiotic Fungus Piriformospora indica: Review,” in Fungal Associations, ed. B. Hock (Berlin: Springer), 231–254. doi: 10.1007/978-3-642-30826-0_13
Verma, S., Varma, A., Rexer, K.-H., Franken, P., Verma, S., Sarbhoy, A., et al. (1998). Piriformospora indica, gen. et sp. nov., a new root-colonizing fungus. Mycologia 90, 896–903. doi: 10.2307/3761331
Wacker, R. (2006). Eine neue und einfache Methode zur polychromatischen Anfärbung von Paraffinschnitten pflanzlicher Gewebe für Durchlicht- und Fluoreszenzmikroskopie. Mikrokosmos 95, 210–212.
Waller, F., Achatz, B., Baltruschat, H., Fodor, J., Becker, K., Fischer, M., et al. (2005). The endophytic fungus Piriformospora indica reprograms barley to salt-stress tolerance, disease resistance, and higher yield. Proc. Natl. Acad. Sci. U.S.A. 102, 13386–13391. doi: 10.1073/pnas.0504423102
Wazny, R., Rozpadek, P., Jedrzejczyk, R. J., Sliwa, M., Stojakowska, A., Anielska, T., et al. (2018). Does co-inoculation of Lactuca serriola with endophytic and arbuscular mycorrhizal fungi improve plant growth in a polluted environment? Mycorrhiza 28, 235–246. doi: 10.1007/s00572-018-0819-y
Yang, Y., Liang, Y., Han, X., Chiu, T.-Y., Ghosh, A., Chen, H., et al. (2016). The roles of arbuscular mycorrhizal fungi (AMF) in phytoremediation and tree-herb interactions in Pb contaminated soil. Sci Rep 6, 20469. doi: 10.1038/srep20469
Zhang, W., Wang, J., Xu, L., Wang, A., Huang, L., Du, H., et al. (2018a). Drought stress responses in maize are diminished by Piriformospora indica. Plant Signal. Behav. 13, e1414121. doi: 10.1080/15592324.2017.1414121
Zhang, Y., Hu, J., Bai, J., Wang, J., Yin, R., Wang, J., et al. (2018b). Arbuscular mycorrhizal fungi alleviate the heavy metal toxicity on sunflower (Helianthus annuus L.) plants cultivated on a heavily contaminated field soil at a WEEE-recycling site. Sci Total Environ 62, 282–290. doi: 10.1016/j.scitotenv.2018.01.331
Zheljazkov, V. D., Craker, L. E., and Xing, B. (2006). Effects of Cd, Pb, and Cu on growth and essential oil contents in dill, peppermint, and basil. Environ. Exp. Bot. 58, 9–16. doi: 10.1016/j.envexpbot.2005.06.008
Keywords: arbuscular mycorrhizal fungi, Rhizophagus irregularis, Ocimum basilicum, Serendipita indica, Piriformospora indica, heavy metal, soil pollution
Citation: Sabra M, Aboulnasr A, Franken P, Perreca E, Wright LP and Camehl I (2018) Beneficial Root Endophytic Fungi Increase Growth and Quality Parameters of Sweet Basil in Heavy Metal Contaminated Soil. Front. Plant Sci. 9:1726. doi: 10.3389/fpls.2018.01726
Received: 30 May 2018; Accepted: 06 November 2018;
Published: 27 November 2018.
Edited by:
Andrea Genre, Università degli Studi di Torino, ItalyReviewed by:
Marian Brestic, Slovak University of Agriculture, SlovakiaSudhakar Srivastava, Banaras Hindu University, India
Copyright © 2018 Sabra, Aboulnasr, Franken, Perreca, Wright and Camehl. This is an open-access article distributed under the terms of the Creative Commons Attribution License (CC BY). The use, distribution or reproduction in other forums is permitted, provided the original author(s) and the copyright owner(s) are credited and that the original publication in this journal is cited, in accordance with accepted academic practice. No use, distribution or reproduction is permitted which does not comply with these terms.
*Correspondence: Iris Camehl, Y2FtZWhsQGlnemV2LmRl
†Present address: Louwrance Peter Wright, Zeiselhof Research Farm, Menlo Park, South Africa