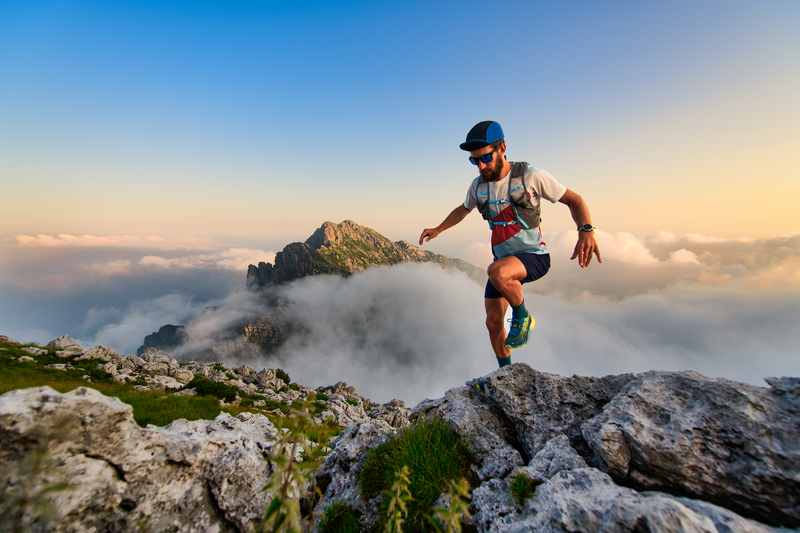
94% of researchers rate our articles as excellent or good
Learn more about the work of our research integrity team to safeguard the quality of each article we publish.
Find out more
REVIEW article
Front. Plant Sci. , 28 November 2018
Sec. Plant Pathogen Interactions
Volume 9 - 2018 | https://doi.org/10.3389/fpls.2018.01692
Roots are important organs for plant survival. In recent years, clear differences between roots and shoots in their respective plant defense strategies have been highlighted. Some putative gene markers of defense responses usually used in leaves are less relevant in roots and are sometimes not even expressed. Immune responses in roots appear to be tissue-specific suggesting a compartmentalization of defense mechanisms in root systems. Furthermore, roots are able to activate specific defense mechanisms in response to various elicitors including Molecular/Pathogen Associated Molecular Patterns, (MAMPs/PAMPs), signal compounds (e.g., hormones) and plant defense activator (e.g., β-aminobutyric acid, BABA). This review discusses recent findings in root defense mechanisms and illustrates the necessity to discover new root specific biomarkers. The development of new strategies to control root disease and improve crop quality will also be reviewed.
In the natural environment, plants are continuously exposed to diverse pathogens that affect crop production and food security. In the last decade, considerable progress has been made to understand the molecular and cellular interactions between pathogens and plants (Lai and Mengiste, 2013; Zhang et al., 2013). The cell wall represents the first line of plant defense acting as a preformed barrier against pathogen invasion. Activation of inducible defense reactions is based on the plant’s ability to detect the presence of microorganisms through the recognition of highly conserved molecular patterns called MAMPs (Microbe-Associated Molecular Patterns). These conserved patterns are foreign as they derive directly from either non-pathogenic microorganisms (Henry et al., 2012) or from pathogenic microorganisms, e.g., bacterial flagella (Chinchilla, 2006), peptidoglycan (Gust et al., 2007), fungal chitin (Zipfel and Felix, 2005). These pathogenic derived patterns are commonly referred to as PAMPs (Pathogen Associated Molecular Patterns). Plants also recognize endogenous signals released by the plant itself, under pathogen pressure (Klarzynski and Fritig, 2001) or during abiotic stress (Choi and Klessig, 2016). These biotic and abiotic stress related patterns are called DAMPs (Damage-Associated Molecular Patterns) (Boller and Felix, 2009). All of these molecular patterns, also known as general elicitors (Henry et al., 2012), are recognized by pattern recognition receptors (PRRs) present at the cell surface (Newman et al., 2013; De Lorenzo et al., 2018). In this review, the generic term elicitor refers to any compound that triggers plant responses in various ways: MAMPs/PAMPs/DAMPs, signaling compounds (salicylic acid, methyl-jasmonate), priming agents and plant defense activators (PDAs) used for agricultural practices (e.g., β-aminobutyric acid, BABA). The perception of such elicitors triggers the activation of a non-host resistance known as Pathogen- or Pattern-Triggered Immunity (PTI) (Jones and Dangl, 2006; Bigeard et al., 2015). PTI contributes to host defense against a broad range of pathogens. The current knowledge around plant immunity is predominantly focused on the aerial part of the plant. However, the root is the organ that encounters the highest microorganism density and diversity being within the rhizosphere (Torsvik and Ovreas, 2002; Jacobs et al., 2011). In this review, we aim to provide new insights regarding root immune system responses to PAMPs. Furthermore, the ability of pathogen mimicking molecules, referred as PDAs on stimulating root defense mechanisms will be discussed.
The rhizosphere is highly enriched in microorganisms with up to 106–109 bacteria, 105–106 fungi and 101–102 nematodes per gram of soil (Watt et al., 2006; Mendes et al., 2013). These organisms can be either beneficial, such as plant growth-promoting rhizobacteria (PGPR) (Beneduzi et al., 2012), or pathogenic, such as Fusarium spp. Soil-borne such as Fusarium, Pythium, or Phytophthora infect the roots of a variety of crops resulting in significant economic losses. Thus, roots represent an important opportunistic entryway for a number of soil pathogens. Pathogens can penetrate roots through natural apertures present at the junction between the main and lateral roots such as epidermal cracks (Perrine-Walker et al., 2007) or through young growing tissues which lack secondary cell walls (Okubara and Paulitz, 2005). Amongst pathogenic organisms, vascular pathogens penetrate the root system to infect and cause symptoms within the aerial parts of host plants such as their leaves. For example, the fungus Colletotrichum graminicola, responsible of maize anthracnose is able to infect roots even though it is generally not considered to be a root disease pathogen (Sukno et al., 2008). In rice, the fungus Magnaporthe grisea (Sesma and Osbourn, 2004), typically thought to be a foliar pathogen, was able to spread from infected roots to leaves. Fusarium oxysporum f. sp. vasinfectum and Verticillium longisporum infect root vascular tissue (Dowd et al., 2004) and moves upwards to the foliage (Iven et al., 2012). It is therefore essential for roots to detect soil pathogens and initiate defense responses to limit pathogen infection and spread. Although root infections have a negative impact on crop production (Okubara and Paulitz, 2005), most plant defense studies are focused on leaves (Balmer and Mauch-Mani, 2013) rather than on roots. To date, only few investigations are dedicated to root defenses as root systems are more complex to study because of their general inaccessibility. In addition, soil-borne microorganisms are not easily culturable in vitro. Less than 1% of soil microorganisms are currently characterized and culturable under laboratory conditions (Singh et al., 2004).
Recent studies have highlighted that roots are able to perceive the presence of pathogens and induce the PTI response. Induction of defense mechanisms is shown to occur in response to a wide range of elicitors including the flagellin-derived peptide elicitor Flagellin22 (Flg22), fusaric acid, peptidoglycan (PGN), Methyl-jasmonate (MeJA) and extracts from Pectobacterium atrosepticum (Millet et al., 2010; Plancot et al., 2013; Gotté et al., 2015, 2016; Koroney et al., 2016). Treatment with Flg22 was shown to enhance resistance to microbial invasion in roots by inducing reactive oxygen species (ROS) accumulation, callose deposition and the production of antimicrobial compounds during PTI response (Millet et al., 2010; Tran T.M. et al., 2016). These defense responses clearly show that root cells are able to perceive elicitors, suggesting the presence of cell-surface receptors.
To confirm the existence of MAMPs/PAMPs receptors, the root responses were investigated in an Arabidopsis fls2 mutant lacking a functional Flg22 receptor. The PTI response to Flg22 was completely abolished in the fls2 mutant confirming the existence of specific PRRs in Arabidopsis roots (Gómez-Gómez and Boller, 2000; Gómez-Gómez, 2004; Robatzek et al., 2006; Millet et al., 2010). It has been shown that all Arabidopsis root tissues, including the root-cap derived border-like cells (BLCs), have the capacity to activate immune responses (Plancot et al., 2013; Wyrsch et al., 2015). This suggests that Flg22 receptor (FLS2) is present in all root tissues. Further studies demonstrated significant differences in the expression of the gene FLS2 in various root tissues (Beck et al., 2014). Indeed, intensity of the responses observed was more pronounced within the inner root tissues believed to be due to enriched receptor distribution and density in the endodermis and stele cells (Beck et al., 2014; Wyrsch et al., 2015). However, even if the FLS2 promoter activity is mainly present in the root stele, the expression of FLS2 expanded to cortical and epidermal cell layers when roots are grown under non-sterile or biotic stress conditions (Beck et al., 2014; Wyrsch et al., 2015). Moreover, FLS2 expression is also shown to be regulated in a developmental-dependent manner (Beck et al., 2014). For example, the FLS2 promoter activity is highly expressed in primordia and in growing lateral roots. However when these lateral roots reach a mature developmental stage, the FLS2 expression became restricted to the vascular cylinder of the developing lateral roots (Beck et al., 2014). Therefore, these findings suggest that the expression of MAMPs receptors are limited and restricted to the most vulnerable sites for pathogen entryway. Activation of PTI was therefore induced only when PAMPs perception recognizes a significant specific threat to the plant itself. This is especially true when MAMPs/PAMPs are detected in the pericycle or in the vascular system. However, PAMPs perception by roots was also reported to differ depending on the elicitor type (e.g., fusaric acid, chitin or mycelium extract from Fusarium oxysporum) (Millet et al., 2010; Plancot et al., 2013) and according to the specific plant species under study (Tran T. et al., 2016).
In the rhizosphere, roots are constantly exposed to MAMPs. Therefore, roots need to distinguish between microbial “friend or foe,” i.e., recognizing beneficial microbes from pathogenic microbes. It is thus necessary that the root system modulates the defense responses that are associated with their recognition in order to avoid a constant and energetically expensive activation of the PTI. Consequently, beneficial microbes have to escape recognition by the root receptors in order to establish root interactions. One hypothesis is that FLS2 expression in roots can be sufficiently low to allow beneficial bacteria to colonize root tissue without triggering PTI defense responses (Millet et al., 2010). Both beneficial and pathogen micro-organisms are able to modulate root immune responses suggesting a fine-tuned molecular dialog. Such interaction is complex because it involves the interactions with beneficial microbes, which the plant must encourage without threatening its own survival. Interactions between microbiota and roots were recently well reviewed in Hacquard et al., 2017. For more details, the readers are invited to refer to this paper.
Previous studies performed in roots indicated that PTI includes molecular events such as the production of ROS (Poncini et al., 2017), transcriptional reprogramming (Boller and Felix, 2009; Millet et al., 2010), callose deposition (Millet et al., 2010) and modified extensin distribution within the cell wall (Ribeiro, 2006; Wojtasik et al., 2011; Plancot et al., 2013; Figure 1A). Phytohormones including salicylic acid (SA), jasmonic acid (JA), and ethylene (ET), are also implicated in root defense against pathogens (Tytgat et al., 2013; Papadopoulou et al., 2018). However, the antagonistic interactions of the two hormones JA and SA reported in leaves did not always follow the infection process in, for example, the roots of Arabidopsis thaliana (Badri et al., 2008; Attard et al., 2010). In addition, the regulation of defense genes by hormonal elicitation was reported to be organ-specific and differed both quantitatively and qualitatively between aerial and below ground organs in the non-model plants Brassica oleracera and Brassica rapa (Tytgat et al., 2013; Papadopoulou et al., 2018). Differences regarding the diversity and levels of antimicrobial compound produced occur between roots and leaves suggesting that different regulation mechanisms exist for defense in roots. For example in rice, the expression of typical defense-related genes such as pathogenesis related-proteins (PR-1, PR-10) are rapidly, but transiently, transcribed during the early stages of root infection whereas in leaves the same transcripts continue to accumulate to high levels during later stages of infection (Marcel et al., 2010). Consequently, PR-1 which is a characteristic salicylic acid (SA) marker highly expressed in Arabidopsis leaves, is found to be produced at very low concentrations in maize (Balmer et al., 2013) and rice (Marcel et al., 2010) in response to pathogen attack. In B. rapa, PR-1 shows also differential regulation in response to hormonal elicitation in both organs (Papadopoulou et al., 2018) confirming that shoots and roots can adopt specific defense responses (Tytgat et al., 2013). Other important proteins controlling plant responses to pathogen are found in leaves such as JA markers (plant defensin: PDF1.2), ET markers (amino-cyclopropane-carboxylate oxidase myrosinase binding protein: ACCO), glucosinolate markers (enzyme involves in glucosinolate biosynthesis: CYP79B2). However, these same biomarkers are not significantly induced in Arabidopsis roots (Badri et al., 2008; Attard et al., 2010; Lyons et al., 2015) suggesting they are leaf specific. Taking glucosinolate biosynthesis marker genes as an example we consider their differential expression in roots versus leaves. Glucosinolates are major nitrogen and sulfur containing secondary metabolites that alleviate biotic and abiotic stress in plant species belonging to Brassicaceae (Martínez-Ballesta et al., 2013; Augustine and Bisht, 2015). As compared to leaves, JA induces glucosinolate biosynthesis genes very weakly in roots as glucosinolates are found to be constitutively present in the below ground organs (i.e., roots) of B. oleracera (Tytgat et al., 2013). It is suggested that the constitutive high levels of glucosinolates in roots is an efficient approach to counteract rhizosphere pathogen attack versus an inducible synthesis approach. Similarly, the Endoplasmic Reticulum (ER)-bodies are reported to be constitutively produced in roots of Arabidopsis thaliana whereas their formation in leaves is only found upon wounding or MeJA treatment (Matsushima, 2002). ER-bodies are organized into network structures that accumulate defense proteins such as β-glucosidase (Nakano et al., 2014). In stress-free conditions, ER-bodies are reported to occur in roots (Nakano et al., 2014, 2017). However, these root ER-bodies are not uniformly formed and have been reported to be absent in some tissues including the quiescent zone, the endodermis and the stele (Gotté et al., 2015). Interestingly, exogenous application of JA or MeJA in roots were shown to have an effect on both the morphology and enzymatic activity of the ER-bodies (Gotté et al., 2015). Such data suggest that ER-bodies are part of the PTI response in roots. Based on these studies, there is a need to carefully select specific gene markers, both according to the plant species and the organs (e.g., aerial or belowground organs) under investigation.
FIGURE 1. Overview of global and tissue-specific defense responses in Arabidopsis thaliana root. (A) Early PAMPs-triggered immunity (PTI) are common to leaves and roots. Production of ROS, callose deposition, an increase of defense genes leading to cell wall modification have been described in leaves and roots tissues. However, differences are noticed: the antagonistic effect of Salicylic acid (SA) and Jasmonic acid (JA) is not observed in A. thaliana root in response to Phytophthora parasitica infection (Attard et al., 2010). Markers of SA (PR-1), JA (PDF1.2) and ET (ACCO) expressed in leaves are also less relevant in roots (Badri et al., 2008; Attard et al., 2010; Lyons et al., 2015). (B) Root responses show tissue-specificity. In this schematic representation of a root, different zones can be distinguished: DZ, differentiation zone; PZ, root hair zone; EZ, elongation zone; MZ, meristematic zone; RC and BLCs, root cap and border-like cells. Millet et al. (2010), used various defense gene promotors in fusion to GUS in order to evaluate the defense gene expression in the different zone of the root after elicitation with various MAMPs. In response to chitin elicitation, gene expression occurred throughout the entire mature zones (DZ and PZ). In contrast, with Flg22 and PGN, the response is restricted to EZ. In this work, callose deposition was also studied. Furthermore, in root caps, it has been shown that in response to Flg22, expression of defense genes such as WRKY11 and HEL was restricted to the peripheral RC cells (Poncini et al., 2017). This compartmentalized response might suggest tissue-specific elicitor receptors localization. SA, Salicylic acid; JA, Jasmonic acid; ET, Ethylene; PR-1, Pathogenesis related proteins 1; PDF1.2, Plant defensin family 1.2; ACCO, Amino-cyclopropane-carboxylate oxidase myrosinase binding protein; CYP71A12, Cytochrome P450 family 71 polypeptide; MYB51, Transcription factor for the regulation of indole-glucosinolate biosynthesis; WRKY11, Negative regulator of basal resistance; AT5G25260, Nodulin-like protein of unknown function; ACS6 : 1-aminocyclopropane-1-carboxylate synthase 6; HEL, Hevein-like protein; ZAT12, Zinc-finger protein; PER5, Peroxidase superfamily protein; Flg22, Flagellin fragment of 22 amino-acid; PGN, Peptidoglycan. : Pathogen microorganisms
: Beneficial microorganisms. Copyright by the American Society of Plant Biologists.
Many pathogens are reported to infect their hosts through specific regions of the root termed root zones (RZs) (Gunawardena and Hawes, 2002; Oren et al., 2003; Cannesan et al., 2011). This suggests that root tissues differ in their susceptibility to pathogens and therefore their inherent resistance capacities. For example, spatiotemporal events in tomato roots are important to limit Ralstonia solanacearum from spreading and infecting the entire plant (Caldwell et al., 2017). In pea, Aphanomyces euteiches infection is restricted in roots suggesting the existence of tissue specific responses (Cannesan et al., 2011). The differential sensitivities found in root tissues can be partially explained by the spatial localization of pisatin, a phytoalexin that accumulates in response to pathogen infection. It was shown that pisatin accumulates mainly in RZs that escaped pathogen infection [e.g., root cap cells (RC) and differentiation zone (DZ)]. Pisatin concentration is however significantly lower in the more susceptible elongation zone (EZ) (Cannesan et al., 2011). The greater susceptibility of the root EZ is explained by the yougth of elongating cells which have not fully developed protective tissue such as their epidermis barrier (Wyrsch et al., 2015). Thus, innate immunity responses appeared to be enhanced in EZs as compared to the other parts of the root system (Millet et al., 2010; Poncini et al., 2017). In Arabidopsis roots, Flg22 or PGN are elicitors derived from bacteria that trigger callose deposition in the EZ (Millet et al., 2010; Figure 1B). The expression of defense genes such as the cytochrome P450 CYP71A12 and the transcription factor MYB51, that are involved in the biosynthesis compound toxic to pathogens, are activated after Flg22 treatment in EZs (Millet et al., 2010). These genes are also expressed in response to root-knot nematodes in EZs (Teixeira et al., 2016). In contrast, in response to chitin, an elicitor derived from fungal cell walls, CYP71A12, MYB51 expression and callose deposition was observed in all mature parts of the RZ (Millet et al., 2010). In addition, early MAMP-signaling marker WRKY11 and the ROS markers ZAT12 and PER5 were also reported to be mainly induced in the EZ and DZ (Poncini et al., 2017). Indeed, YFP marker fusion constructs expressed in the EZ were only visible in the epidermal layer without elicitor treatment and expressed in the central tissues few hours after elicitor treatment (e.g., pMYB51::YFPN and pZAT12::YFPN). Whereas in the root tip, YFP fluorescence was restricted to the peripherical root cap cells (e.g., pWRKY11::YFPN and pHEL::YFPN) (Poncini et al., 2017). These data suggest that PTI induction can be restricted to specific root tissue zones that are critical for successful infection by invading pathogen (Millet et al., 2010; Teixeira et al., 2016).
Newly generated tissues are preferential zones of infection by soil pathogens. However, most of the nascent root tips remain uninfected even if other root tissues develop lesions (Wen et al., 2006). Indeed, the root tip inoculated with Nectria haematococca remained devoid of infection whereas the EZ was almost entirely infected. (Gunawardena and Hawes, 2002). This root tip tissue-specific resistance is correlated spatially with the presence of border cells (BCs) surrounding the root cap periphery (Driouich et al., 2013; Koroney et al., 2016). These BCs, which are individually separated from the root tip immediately after water contact (Hawes et al., 2000) are embedded in a thick mucilage and respond to both biotic (Driouich et al., 2013) and abiotic stresses (Miyasaka and Hawes, 2001; Cai et al., 2011). Several studies have demonstrated that BCs act as a physical and chemical barrier to fungi (Gunawardena and Hawes, 2002; Cannesan et al., 2011), bacteria (Hawes and Pueppke, 1987), zoospores (Goldberg et al., 1989), and nematodes infection (Zhao et al., 2000). These studies suggest a special role for the root cap and associated tissues in plant defense (Hawes et al., 2016; Tran T.M. et al., 2016). Therefore, it was suggested that BCs act as “sentries” providing specialized protection of the root cap and root meristems (Plancot et al., 2013). Root BCs were shown to attract pathogens such as oomycetes and nematodes (via chemotaxis) in order to subsequently neutralize them using antimicrobial mucilage traps (Zhao et al., 2000; Hawes et al., 2016). It is reported that root BCs attract zoospores (Zhao et al., 2000) to stimulate their germination and thereby blocking their mycelial growth by so doing preventing root infection from happening (Hawes et al., 2016). The interaction between root BCs and soil-borne microbes varies according to the plant species and the micro-organisms present. For example, root BCs from pea (Pisum sativum) are colonized by Agrobacterium tumefaciens whereas BCs from oat produce a mucilage which blocks and excludes bacteria from the BCs surface (Hawes et al., 2000). In addition, BCs were shown to synthesize and to secrete antimicrobial compounds (Driouich et al., 2013) such as phytoalexins, glycans/polymers and defense proteins that are able to fight against pathogens (Hawes et al., 2016). Arabinogalactan proteins (AGP) extracted from pea root cap and BCs prevent in vitro zoospores germination of A. euteiches. This could explain the reduced infection of the root cap that is observed compared to other RZs (Cannesan et al., 2012). Furthermore, the presence of extracellular DNA was reported within the mucilage surrounding the root BCs forming a Root Extracellular Trap (RET) by analogy with the Neutrophil Extracellular Trap (NET) in mammals (Driouich et al., 2013; Hawes et al., 2016; Tran T.M. et al., 2016). Additionally, it was demonstrated that BCs are also implicated in active induced defense strategies. Pathogens and/or elicitors cause an increase in the number of the BCs released into the rhizosphere as well as an enhanced secretion of metabolites by these cells (Curlango-Rivera et al., 2010; Cannesan et al., 2011). As a consequence of these studies, one can speculate that BCs activate defense mechanisms in greater intensity even before roots perceive MAMPS.
Interestingly, the plant model A. thaliana releases atypical BCs that do not separate individually but remain attached to each other and to the root cap. Due to this particular organization and mode of detachment, these cells are termed root border-like cells (BLCs). BLCs have recently been implicated in root defense but their functioning in planta is still unknown. However, Plancot et al., 2013 demonstrated that BLCs from A. thaliana are able to perceive MAMPs and activate defense responses by producing ROS as well as callose deposition. These findings suggest that BLCs are probably specifically involved in root defense in a similar manner to the root BCs.
The growing need for more sustainable and environmentally safe agricultural practices requires the development of new agronomic approaches. In this context, the use of natural plant defense activators (or PDAs) has emerged as an effective and eco-friendly approach to agriculture (Benhamou and Rey, 2012; Delaunois et al., 2014). Upon infection, plants can also induce through molecular mechanisms systemic acquire resistance (SAR) in response to biotic and abiotic stimuli. This acquired resistance allows for a faster and stronger induction of defense pathways in response to subsequent pathogens. The plant is therefore “semi-permanently activated” for any further interactions with pathogenic organisms. PDAs are either small compounds or polymers that precondition the plant against further or other diseases and/or pathogen attack. When applied to plants, PDAs mimics pathogen aggression and trigger defense responses similar to those induce upon infection. The efficacy of PDAs relies on their ability to stimulate the plant defense systems mainly. They have little to no direct effect on pathogens themselves and so unlike pesticides avoid generating selective pressures on plant pathogen populations. Although, some PDAs such as chitosan can have some direct effects on certain pathogens (El Hassni et al., 2004) it seems in general this is not commonly observed for most PDAs. Overall PDAs represent good substitutes for conventional pesticides in food crops and have contributed to the growing research area of environmentally friendly “plant care” industries. Many commercially available compounds, when applied as foliar sprays act as inducers of plant defense and their efficiency has been demonstrated in field trial studies (Pichyangkura and Chadchawan, 2015; Malerba and Cerana, 2016). A list of such molecules used in field crops can be found in the review written by Thakur and Sohal (2013). These examples reflect the great interest in PDAs for controlling plant diseases using foliar applications whereas relatively few studies have focused on root treatments. Two main reasons could explain this discrepancy: (1) the complexity and difficulty of PDAs applications in soil and (2) the inability to control root bioavailability toward PDAs.
PDAs have been shown to induce strong and rapid defense responses when applied to roots. Several authors report the enhanced resistance to pathogens after root treatment with PDAs. This enhanced resistance in treated plants can be associated with various cellular and molecular defense responses (Badri et al., 2008; Table 1). Roots responded to several elicitors with ROS production characterized by a rapid release of H2O2 observed in the apoplast of various plant species (Zambounis et al., 2012). Activation of several enzyme activities implicated in ROS production (Nars et al., 2013), cell wall reinforcement (Mandal et al., 2009, 2013) and the production of antimicrobial compounds (Francis et al., 2009) have been reported following elicitor application (Table 1). In addition, different metabolic profiles are observed depending on the molecules being tested (Badri et al., 2008). These differences might be explained by cell specific transport mechanism that is induced in relation to the applied PDAs (Badri et al., 2008). For example, SA differentially regulates potassium, calcium, sulfate, ammonium as well as Major Facilitator Superfamily (MFS) and Multi-Antimicrobial Extrusion protein (MATE) transporters (Badri et al., 2008). Whereas MeJA was shown to differentially regulate MATE, an H+ ATPase pump, a sugar transporter and a metal transporter (Badri et al., 2008). Thus, root treatment with PDAs result in enhanced resistance to pathogens via the SAR mechanisms (Mandal et al., 2009). Furthermore, some molecules act differently as compared to elicitors, these are named priming agents. Activation of defense mechanisms under pathogen free-conditions is energetically taxing and competes with normal plant growth and development processes (Martinez-Medina et al., 2016). Priming does not induce the direct activation of defense mechanisms but activates defense signal pathways in plant cells without affecting plant growth (Aranega-Bou et al., 2014). For example, inactive mitogen-activated protein kinases (MAPKs) are typical defense signaling molecules that accumulate during priming. These molecules will be deployed when the plant is exposed to a pathogen or elicitor stress exposure (Beckers et al., 2009; Conrath, 2011) leading to a faster defense response. Beta-aminobutyric acid (BABA) is a well-known priming agent that potentiates (i.e., primes) SA-dependent defense responses in leaves and in roots (Zimmerli et al., 2000, Table 1). Elicitors including priming agents can induce both plant SAR. Such treatments can provide good substitute for pesticides in agriculture because they induce defense mechanisms in both treated and non-treated organs (Mandal et al., 2009; Myresiotis et al., 2014). Moreover, it was recently demonstrated that SAR is transmissible through the root system from SAR-triggered plants to their neighboring plants when treated with benzothiadiazole (BTH) (Song et al., 2010; Cheol Song et al., 2016). These results provide novel insights that can help in the development of new strategies for enhancing root defense capacities and also effectively inducing resistance against plant pathogens in target crops.
TABLE 1. Elicitors (including signaling compounds, priming agents and PDAs) that have been shown to induce plant defense after root treatment on different plant species.
The use of PDAs is often controversial because of their variable efficiency as compared to pesticides which show a direct toxic action on the pathogenic organism. Although PDAs are effective in controlled laboratory conditions, efficacy in the field remains dependent on numerous factors (extrinsic and intrinsic). Variability can often be related directly to the specific PDAs used (Figure 2A). Chemical properties of the active compound are non-negligible parameters for efficacy. For example, biological activity of oligosaccharides like laminarin, a well-studied algal elicitor known to induce resistance, is highly dependent on their structures and degree of polymerization (Trouvelot et al., 2014). In addition, a chemical modification of sulphated laminarin, showed that the number of sulfate groups could influence its efficiency to induce plant defense responses (Menard, 2004, 2005; Gauthier et al., 2014). Indeed, the sulfate groups have been shown to protect the molecular core against enzymatic degradation (Menard, 2005). For an extensive review on the structure/activity relationships of carbohydrates see Trouvelot et al. (2014). It is also essential to consider the amount of product provided per plant, the time span for absorption and the application method used. The effect of the particular product used may be different if root uptake occurs from aqueous solutions or from the soil environment (Jakab et al., 2001). For example, induction of resistance to root-knot nematode in tomatoes was enhanced when SA was provided to plants as a soil drench rather than as root-dip (Molinari and Baser, 2010). Whereas the opposite occurred with Acibenzolar-S-methyl (ASM) treatments (Molinari and Baser, 2010; Table 1). The use of BABA for example as a soil drench is better tolerated by Arabidopsis without the deleterious effects of high concentrations used in foliar spray format (Jakab et al., 2001). The number of applications should also be considered in relation to the method of application (McGrann et al., 2017). When applied as a soil drench protective resistance lasts much longer as compared to a foliar spray (Francis et al., 2009). Similarly, when BION is applied as a soil drench it is a more efficient in its ability to reduce club-root development in brassica crops compared to applying it as a foliar spray (McGrann et al., 2017). PDAs efficiency (Figure 2B) is also dependent on the specific plant species being treated (Walters et al., 2013). In lettuce and cucumber, SA behaves as a phytotoxin by reducing root and shoot length (Pramanik et al., 2000) whereas in tomato, SA potentiates resistance (Molinari and Loffredo, 2006; Molinari and Baser, 2010). Environmental conditions can also affect plant vigor by reducing its capacity to trigger defense mechanisms and thereby impact PDAs bioavailabity. A case in point would be leaching of PDAs due to excessive rain (Figure 2C). Furthermore, soil properties and composition modulate the expression level of resistance of the plant and its response to PDAs treatment. For example, in tomato it was demonstrated that when SA was mixed with humic acid the induced resistance was more pronounced than when SA was provided alone (Molinari and Baser, 2010). The rhizosphere is highly enriched in microorganisms that can interact with PDAs in multiple ways. We can hypothesize that some of these microbes can degrade the PDAs via hydrolytic enzyme action resulting in a lower quantity that is bioavailable to the plant. However, to date, the role of microorganisms on the PDAs bioavailability remains unclear.
FIGURE 2. Overview of factors influencing plant defense stimulator (PDAs) efficiency in roots. (A) PDAs efficiency to trigger plant defense depends mainly on their chemical structure, on the application method and on treatment frequency. (B) Physiological state of the plant impact PDAs efficiency (Walters et al., 2013). PDAs effect can be different depending on species, plant genotype/variety, age of the plants and their vigor that depends on nutrition and agricultural practices. (C) In the environment, PDAs efficiency are dependent of physical and biological factors. For example, rain or irrigation can induce PDAs leaching. Soil properties and composition can improve PDAs bioavailability (e.g., humic acid) (Molinari and Loffredo, 2006; Molinari and Baser, 2010). Some microorganisms can degrade the PDAs via hydrolytic enzymes. When PDAs is absorbed by the plant, different root tissue can act as natural barrier reducing PDAs efficiency. As a consequence, receptor localization in the root is at the core of PDAs efficiency to trigger plant defense responses. However, it has to be noticed that once PDAs trigger plant responses, roots can release signal molecules such as salicylic acid (SA) (Cheol Song et al., 2016) to induce plant defense in neighboring plant and in non-treated organs such as leaves consisting in the systemic acquire resistance (SAR). : Plant defense activators
: Plant defense activators receptors
: salicylic acid
: Interaction of plant defense activator with microorganisms
One of the most important challenges to the use of PDAs is their effective assimilation into the host (Paris et al., 2016). It should not be forgotten that the developmental stage of the treated organ, i.e., the root, is also an essential parameter to consider. With age, the root cortex becomes dry, corky and impermeable thus reducing PDAs absorption. To be recognized by the host, a PDAs must first be recognized by a receptor. As for Flg22, these receptors should be located within the inner layer of the endodermis. However, different root tissues can act as natural barriers reducing PDAs efficiency. Once absorbed by root, the molecule can migrate via the apoplastic or symplastic pathways. The apoplastic pathway involves cell wall transport. Therefore, the cell wall itself can block natural diffusion of PDAs into the cell and between associated compartments. For example, the exodermis is a thick and suberized barrier, which varies in degree depending on the species and age of the plant concerned. These properties might contribute to reducing the apoplastic transport of water and solutes. In contrast, molecules can migrate in the root using the symplastic pathway that allows for direct transport through the cytoplasm cells via the presence of plasmodesmata. Then, molecules can thus migrate from the rhizodermis cells through the cortex into the endodermis. Cortical parenchyma thickness thus have an impact on PDAs efficiency by dispersing molecules and lowering their final concentrations. The endodermis, which is another natural selective barrier, forces molecules to cross via symplastic flow due to the presence of casparian strip. The casparian strip is characterized by cell walls containing suberin and sometimes lignin. Due to its cell wall composition, the casparian strip regulates the apoplastic pathways acting as a filter which blocks solutes diffusion between the cortex and the vascular tissues. As a consequence, molecules must cross root endodermal cells. Therefore, the endodermis and the exodermis constitute tight barriers preventing the natural diffusion of molecules. Consequently, receptor localization in the root is at the core of PDAs efficiency in triggering plant defense responses. Therefore, we hypothesize here that these structures might participate in the variable and limited efficacy of PDAs root applications (Figure 2C).
To date, root immunity is far from being fully understood and so many questions remain regarding mechanisms at the cellular and molecular levels. Interestingly, root defense response to elicitors and/or pathogen attacks exhibit tissue specificity. How such compartmentalization occurs in root defense as compared to leaves is still unclear. Is it due to distinct localization of signal receptors or only due to differences in the amplitude of responses in each tissue? One of the specificities of the root system is to release 100s and 1000s of living BCs in the surrounding environment. Due to their localization at the interface between root and soil, BCs are particularly important in root defense against pathogens. A. thaliana produces atypical BCs, so-called root border like-cells (BLCs) that remain attached to each other. Although the function of root BCs in root defense has been reported for different plant species, the exact function of root BLCs remains to be clearly determined. In A. thaliana, root BLCs were shown to perceive MAMPs and display defense mechanisms responses. These findings suggest that root BLCs are key elements in the root defense mechanism. Although BLCs production appear to be constitutively produced, no information is available regarding their formation and release upon biotic stress. Do they also produce specific defense molecules to form a root extracellular trap? Recent studies have confirmed that environmental signals can override the control of BCs production from pea roots. Such characteristic properties in BCs offers new and highly attractive prospects for the development of new defense strategies against pathogens. Thus, an exogenous treatment could increase the production of BCs and/or BLCs in order to cover and protect the elongation zone known to be the most sensitive to pathogens penetration. In this context, recent studies have demonstrated the potential of PDAs to trigger immunity in roots. Sometimes, such root treatments appear to be more efficient compared to foliar sprays. However, as compared to leaves, the efficiency of such elicitation in roots is dependent on multiple factors that influence the degree of induced resistance achieved, thus explaining why such treatment are still of limited use. Among these factors, soil properties associated with micro-organisms might undoubtedly impact PDAs bioavailability at the root level. Moreover, PDAs have to be recognized by receptors to trigger root defense. Although studies have reported strong activation of defense mechanisms after PDAs treatment, their receptor localization and mechanisms associated with the compartmentalized of defense in response to the elicitor remain unclear. Understanding the complex regulation of plant defense mechanisms and factors impacting PDAs efficiency is therefore of critical importance in order develop novel methods for disease prevention and prevention of disease spread.
The co-authors contribute to the writing of the review.
This work was supported by La Région de Normandie, la Structure Fédérative de Recherche “Normandie Végétale” (FED 4277), the University of Rouen Normandy.
The authors declare that the research was conducted in the absence of any commercial or financial relationships that could be construed as a potential conflict of interest.
Aranega-Bou, P., de la O Leyva, M., Finiti, I., García-Agustín, P., and González-Bosch, C. (2014). Priming of plant resistance by natural compounds. Hexanoic acid as a model. Front. Plant Sci. 5:488. doi: 10.3389/fpls.2014.00488
Attard, A., Gourgues, M., Callemeyn-Torre, N., and Keller, H. (2010). The immediate activation of defense responses in Arabidopsis roots is not sufficient to prevent Phytophthora parasitica infection. New Phytol. 187, 449–460. doi: 10.1111/j.1469-8137.2010.03272.x
Augustine, R., and Bisht, N. C. (2015). Biotic elicitors and mechanical damage modulate glucosinolate accumulation by co-ordinated interplay of glucosinolate biosynthesis regulators in polyploid Brassica juncea. Phytochemistry 117, 43–50. doi: 10.1016/j.phytochem.2015.05.015
Badri, D. V., Loyola-Vargas, V. M., Du, J., Stermitz, F. R., Broeckling, C. D., Iglesias-Andreu, L., et al. (2008). Transcriptome analysis of Arabidopsis roots treated with signaling compounds: a focus on signal transduction, metabolic regulation and secretion. New Phytol. 179, 209–223. doi: 10.1111/j.1469-8137.2008.02458.x
Balmer, D., de Papajewski, D. V., Planchamp, C., Glauser, G., and Mauch-Mani, B. (2013). Induced resistance in maize is based on organ-specific defence responses. Plant J. 74, 213–225. doi: 10.1111/tpj.12114
Balmer, D., and Mauch-Mani, B. (2013). More beneath the surface? Root versus shoot antifungal plant defenses. Front. Plant Sci. 4:256. doi: 10.3389/fpls.2013.00256
Beck, M., Wyrsch, I., Strutt, J., Wimalasekera, R., Webb, A., Boller, T., et al. (2014). Expression patterns of flagellin sensing 2 map to bacterial entry sites in plant shoots and roots. J. Exp. Bot. 65, 6487–6498. doi: 10.1093/jxb/eru366
Beckers, G. J. M., Jaskiewicz, M., Liu, Y., Underwood, W. R., He, S. Y., Zhang, S., et al. (2009). Mitogen-activated protein kinases 3 and 6 are required for full priming of stress responses in Arabidopsis thaliana. Plant Cell Online 21, 944–953. doi: 10.1105/tpc.108.062158
Beneduzi, A., Ambrosini, A., and Passaglia, L. M. P. (2012). Plant growth-promoting rhizobacteria (PGPR): their potential as antagonists and biocontrol agents. Genet. Mol. Biol. 35, 1044–1051. doi: 10.1590/S1415-47572012000600020
Benhamou, N., and Rey, P. (2012). Stimulateurs des défenses naturelles des plantes?: une nouvelle stratégie phytosanitaire dans un contexte d’écoproduction durable. II. Intérêt des SDN en protection des cultures. Phytoprotection 92, 24–35. doi: 10.7202/1013299ar
Bigeard, J., Colcombet, J., and Hirt, H. (2015). Signaling mechanisms in pattern-triggered immunity (PTI). Mol. Plant 8, 521–539. doi: 10.1016/j.molp.2014.12.022
Boller, T., and Felix, G. (2009). A renaissance of elicitors: perception of microbe-associated molecular patterns and danger signals by pattern-recognition receptors. Annu. Rev. Plant Biol. 60, 379–406. doi: 10.1146/annurev.arplant.57.032905.105346
Cai, M.-Z., Wang, F.-M., Li, R.-F., Zhang, S.-N., Wang, N., and Xu, G.-D. (2011). Response and tolerance of root border cells to aluminum toxicity in soybean seedlings. J. Inorg. Biochem. 105, 966–971. doi: 10.1016/j.jinorgbio.2011.04.004
Caldwell, D., Kim, B.-S., and Iyer-Pascuzzi, A. S. (2017). Ralstonia solanacearum differentially colonizes roots of resistant and susceptible tomato plants. Phytopathology 107, 528–536. doi: 10.1094/PHYTO-09-16-0353-R
Cannesan, M. A., Durand, C., Burel, C., Gangneux, C., Lerouge, P., Ishii, T., et al. (2012). Effect of arabinogalactan proteins from the root caps of pea and Brassica napus on Aphanomyces euteiches zoospore chemotaxis and germination. Plant Physiol. 159, 1658–1670. doi: 10.1104/pp.112.198507
Cannesan, M. A., Gangneux, C., Lanoue, A., Giron, D., Laval, K., Hawes, M., et al. (2011). Association between border cell responses and localized root infection by pathogenic Aphanomyces euteiches. Ann. Bot. 108, 459–469. doi: 10.1093/aob/mcr177
Cheol Song, G., Sim, H.-J., Kim, S.-G., and Ryu, C.-M. (2016). Root-mediated signal transmission of systemic acquired resistance against above-ground and below-ground pathogens. Ann. Bot. 118, 821–831. doi: 10.1093/aob/mcw152
Chinchilla, D. (2006). The Arabidopsis receptor kinase FLS2 binds flg22 and determines the specificity of flagellin perception. Plant Cell Online 18, 465–476. doi: 10.1105/tpc.105.036574
Choi, H. W., and Klessig, D. F. (2016). DAMPs, MAMPs, and NAMPs in plant innate immunity. BMC Plant Biol. 16:232. doi: 10.1186/s12870-016-0921-2
Conrath, U. (2011). Molecular aspects of defence priming. Trends Plant Sci. 16, 524–531. doi: 10.1016/j.tplants.2011.06.004
Curlango-Rivera, G., Duclos, D. V., Ebolo, J. J., and Hawes, M. C. (2010). Transient exposure of root tips to primary and secondary metabolites: impact on root growth and production of border cells. Plant Soil 332, 267–275. doi: 10.1007/s11104-010-0291-8
De Lorenzo, G., Ferrari, S., Cervone, F., and Okun, E. (2018). Extracellular DAMPs in plants and mammals: immunity, tissue damage and repair. Trends Immunol. doi: 10.1016/j.it.2018.09.006 [Epub ahead of print].
Delaunois, B., Farace, G., Jeandet, P., Clément, C., Baillieul, F., Dorey, S., et al. (2014). Elicitors as alternative strategy to pesticides in grapevine? Current knowledge on their mode of action from controlled conditions to vineyard. Environ. Sci. Pollut. Res. 21, 4837–4846. doi: 10.1007/s11356-013-1841-4
Dowd, C., Wilson, I. W., and McFadden, H. (2004). Gene expression profile changes in cotton root and hypocotyl tissues in response to infection with Fusarium oxysporum f. sp. vasinfectum. Mol. Plant Microbe Interact. 17, 654–667. doi: 10.1094/MPMI.2004.17.6.654
Driouich, A., Follet-Gueye, M.-L., Vicré-Gibouin, M., and Hawes, M. (2013). Root border cells and secretions as critical elements in plant host defense. Curr. Opin. Plant Biol. 16, 489–495. doi: 10.1016/j.pbi.2013.06.010
El Hassni, M., El Hadrami, A., Daayf, F., Barka, E. A., and El Hadrami, I. (2004). Chitosan, antifungal product against Fusarium oxysporum f. sp. albedinis and elicitor of defence reactions in date palm roots. Phytopathol. Mediterr. 43, 195–204.
Francis, M. I., Redondo, A., Burns, J. K., and Graham, J. H. (2009). Soil application of imidacloprid and related SAR-inducing compounds produces effective and persistent control of citrus canker. Eur. J. Plant Pathol. 124, 283–292. doi: 10.1007/s10658-008-9415-x
Fugate, K. K., de Oliveira, L. S., Ferrareze, J. P., Bolton, M. D., Deckard, E. L., and Finger, F. L. (2017). Jasmonic acid causes short- and long-term alterations to the transcriptome and the expression of defense genes in sugarbeet roots. Plant Gene 9, 50–63. doi: 10.1016/j.plgene.2016.12.006
Fugate, K. K., Ferrareze, J. P., Bolton, M. D., Deckard, E. L., and Campbell, L. G. (2012). Postharvest jasmonic acid treatment of sugarbeet roots reduces rot due to Botrytis cinerea, Penicillium claviforme, and Phoma betae. Postharvest Biol. Technol. 65, 1–4. doi: 10.1016/j.postharvbio.2011.10.005
Gauthier, A., Trouvelot, S., Kelloniemi, J., Frettinger, P., Wendehenne, D., Daire, X., et al. (2014). The sulfated laminarin triggers a stress transcriptome before priming the SA- and ROS-dependent defenses during grapevine’s induced resistance against Plasmopara viticola. PLoS One 9:e88145. doi: 10.1371/journal.pone.0088145
Goldberg, N. P., Hawes, M. C., and Stanghellini, M. E. (1989). Specific attraction to and infection of cotton root cap cells by zoospores of Pythium dissotocum. Can. J. Bot. 67, 1760–1767. doi: 10.1139/b89-223
Gómez-Gómez, L. (2004). Plant perception systems for pathogen recognition and defence. Mol. Immunol. 41, 1055–1062. doi: 10.1016/j.molimm.2004.06.008
Gómez-Gómez, L., and Boller, T. (2000). FLS2: an LRR receptor-like kinase involved in the perception of the bacterial elicitor flagellin in Arabidopsis. Mol. Cell 5, 1003–1011. doi: 10.1016/S1097-2765(00)80265-8
Góraj-Koniarska, J., Stochmal, A., Oleszek, W., Mołdoch, J., and Saniewski, M. (2015). Elicitation of anthocyanin production in roots of Kalanchoe blossfeldiana by methyl jasmonate. Acta Biol. Cracov. Bot. 57, 141–148. doi: 10.1515/abcsb-2015-0007
Gotté, M., Bénard, M., Kiefer-Meyer, M.-C., Jaber, R., Moore, J. P., Vicré-Gibouin, M., et al. (2016). Endoplasmic reticulum body–related gene expression in different root zones of Arabidopsis isolated by laser-assisted microdissection. Plant Genome 9. doi: 10.3835/plantgenome2015.08.0076
Gotté, M., Ghosh, R., Bernard, S., Nguema-Ona, E., Vicré-Gibouin, M., Hara-Nishimura, I., et al. (2015). Methyl jasmonate affects morphology, number and activity of endoplasmic reticulum bodies in Raphanus sativus root cells. Plant Cell Physiol. 56, 61–72. doi: 10.1093/pcp/pcu141
Gunawardena, U., and Hawes, M. C. (2002). Tissue specific localization of root infection by fungal pathogens: role of root border cells. Mol. Plant Microbe Interact. 15, 1128–1136. doi: 10.1094/MPMI.2002.15.11.1128
Gust, A. A., Biswas, R., Lenz, H. D., Rauhut, T., Ranf, S., Kemmerling, B., et al. (2007). Bacteria-derived peptidoglycans constitute pathogen-associated molecular patterns triggering innate immunity in Arabidopsis. J. Biol. Chem. 282, 32338–32348. doi: 10.1074/jbc.M704886200
Hacquard, S., Spaepen, S., Garrido-Oter, R., and Schulze-Lefert, P. (2017). Interplay between innate immunity and the plant microbiota. Annu. Rev. Phytopathol. 55, 565–589. doi: 10.1146/annurev-phyto-080516-035623
Hawes, M., Allen, C., Turgeon, B. G., Curlango-Rivera, G., Minh Tran, T., Huskey, D. A., et al. (2016). Root border cells and their role in plant defense. Annu. Rev. Phytopathol. 54, 143–161. doi: 10.1146/annurev-phyto-080615-100140
Hawes, M. C., Gunawardena, U., Miyasaka, S., and Zhao, X. (2000). The role of root border cells in plant defense. Trends Plant Sci. 5, 128–133. doi: 10.1016/S1360-1385(00)01556-9
Hawes, M. C., and Pueppke, S. G. (1987). Correlation between binding of Agrobacterium tumefaciens by root cap cells and susceptibility of plants to crown gall. Plant Cell Rep. 6, 287–290. doi: 10.1007/BF00272000
Henry, G., Thonart, P., and Ongena, M. (2012). PAMPs, MAMPs, DAMPs and others: an update on the diversity of plant immunity elicitors. Biotechnol. Agron. Soc. Environ. 16, 257–268.
Iven, T., König, S., Singh, S., Braus-Stromeyer, S. A., Bischoff, M., Tietze, L. F., et al. (2012). Transcriptional activation and production of tryptophan-derived secondary metabolites in Arabidopsis roots contributes to the defense against the fungal vascular pathogen Verticillium longisporum. Mol. Plant 5, 1389–1402. doi: 10.1093/mp/sss044
Jacobs, S., Zechmann, B., Molitor, A., Trujillo, M., Petutschnig, E., Lipka, V., et al. (2011). Broad-spectrum suppression of innate immunity is required for colonization of Arabidopsis roots by the fungus Piriformospora indica. Plant Physiol. 156, 726–740. doi: 10.1104/pp.111.176446
Jaiti, F., Verdeil, J. L., and El Hadrami, I. (2009). Effect of jasmonic acid on the induction of polyphenol oxidase and peroxidase activities in relation to date palm resistance against Fusarium oxysporum f. sp. albedinis. Physiol. Mol. Plant Pathol. 74, 84–90. doi: 10.1016/j.pmpp.2009.09.005
Jakab, G., Cottier, V., Toquin, V., Rigoli, G., Zimmerli, L., Métraux, J.-P., et al. (2001). β-Aminobutyric acid-induced resistance in plants. Eur. J. Plant Pathol. 107, 29–37. doi: 10.1023/A:1008730721037
Jones, J. D. G., and Dangl, J. L. (2006). The plant immune system. Nature 444, 323–329. doi: 10.1038/nature05286
Klarzynski, O., and Fritig, B. (2001). Stimulation des défenses naturelles des plantes. C. R. Acad. Sci. Ser. III Sci. 324, 953–963. doi: 10.1016/S0764-4469(01)01371-3
Koroney, A. S., Plasson, C., Pawlak, B., Sidikou, R., Driouich, A., Menu-Bouaouiche, L., et al. (2016). Root exudate of Solanum tuberosum is enriched in galactose-containing molecules and impacts the growth of Pectobacterium atrosepticum. Ann. Bot. 118, 797–808. doi: 10.1093/aob/mcw128
Lai, Z., and Mengiste, T. (2013). Genetic and cellular mechanisms regulating plant responses to necrotrophic pathogens. Curr. Opin. Plant Biol. 16, 505–512. doi: 10.1016/j.pbi.2013.06.014
Lyons, R., Stiller, J., Powell, J., Rusu, A., Manners, J. M., and Kazan, K. (2015). Fusarium oxysporum triggers tissue-specific transcriptional reprogramming in Arabidopsis thaliana. PLoS One 10:e0121902. doi: 10.1371/journal.pone.0121902
Malerba, M., and Cerana, R. (2016). Chitosan effects on plant systems. Int. J. Mol. Sci. 17:E996. doi: 10.3390/ijms17070996
Mandal, S. (2010). Induction of phenolics, lignin and key defense enzymes in eggplant (Solanum melongena L.) roots in response to elicitors. Afr. J. Biotechnol. 9, 8038–8047. doi: 10.5897/AJB10.984
Mandal, S., Kar, I., Mukherjee, A. K., and Acharya, P. (2013). Elicitor-induced defense responses in Solanum lycopersicum against Ralstonia solanacearum. Sci. World J. 2013, 1–9. doi: 10.1155/2013/561056
Mandal, S., Mallick, N., and Mitra, A. (2009). Salicylic acid-induced resistance to Fusarium oxysporum f. sp. lycopersici in tomato. Plant Physiol. Biochem. 47, 642–649. doi: 10.1016/j.plaphy.2009.03.001
Mandal, S., and Mitra, A. (2007). Reinforcement of cell wall in roots of Lycopersicon esculentum through induction of phenolic compounds and lignin by elicitors. Physiol. Mol. Plant Pathol. 71, 201–209. doi: 10.1016/j.pmpp.2008.02.003
Marcel, S., Sawers, R., Oakeley, E., Angliker, H., and Paszkowski, U. (2010). Tissue-adapted invasion strategies of the rice blast fungus Magnaporthe oryzae. Plant Cell 22, 3177–3187. doi: 10.1105/tpc.110.078048
Martínez-Ballesta, M., Moreno, D., and Carvajal, M. (2013). The physiological importance of glucosinolates on plant response to abiotic stress in Brassica. Int. J. Mol. Sci. 14, 11607–11625. doi: 10.3390/ijms140611607
Martinez-Medina, A., Flors, V., Heil, M., Mauch-Mani, B., Pieterse, C. M., Pozo, M. J., et al. (2016). Recognizing plant defense priming. Trends Plant Sci. 21, 818–822. doi: 10.1016/j.tplants.2016.07.009
Matsushima, R. (2002). An endoplasmic reticulum-derived structure that is induced under stress conditions in Arabidopsis. Plant Physiol. 130, 1807–1814. doi: 10.1104/pp.009464
McGrann, G. R. D., Yoxall, T., Paterson, L. J., Taylor, J. M. G., Birmpilis, I. G., Walters, D. R., et al. (2017). Control of light leaf spot and clubroot in brassica crops using defence elicitors. Eur. J. Plant Pathol. 148, 447–461. doi: 10.1007/s10658-016-1103-7
Menard, R. (2004). β-1,3 glucan sulfate, but not β-1,3 glucan, induces the salicylic acid signaling pathway in tobacco and Arabidopsis. Plant Cell Online 16, 3020–3032. doi: 10.1105/tpc.104.024968
Menard, R. (2005). Defense and resistance-inducing activities in tobacco of the sulfated β-1,3 glucan PS3 and its synergistic activities with the unsulfated molecule. Plant Cell Physiol. 46, 1964–1972. doi: 10.1093/pcp/pci212
Mendes, R., Garbeva, P., and Raaijmakers, J. M. (2013). The rhizosphere microbiome: significance of plant beneficial, plant pathogenic, and human pathogenic microorganisms. FEMS Microbiol. Rev. 37, 634–663. doi: 10.1111/1574-6976.12028
Millet, Y. A., Danna, C. H., Clay, N. K., Songnuan, W., Simon, M. D., Werck-Reichhart, D., et al. (2010). Innate immune responses activated in Arabidopsis roots by microbe-associated molecular patterns. Plant Cell 22, 973–990. doi: 10.1105/tpc.109.069658
Miyasaka, S. C., and Hawes, M. C. (2001). Possible role of root border cells in detection and avoidance of aluminum toxicity. Plant Physiol. 125, 1978–1987. doi: 10.1104/pp.125.4.1978
Molinari, S., and Baser, N. (2010). Induction of resistance to root-knot nematodes by SAR elicitors in tomato. Crop Prot. 29, 1354–1362. doi: 10.1016/j.cropro.2010.07.012
Molinari, S., and Loffredo, E. (2006). The role of salicylic acid in defense response of tomato to root-knot nematodes. Physiol. Mol. Plant Pathol. 68, 69–78. doi: 10.1016/j.pmpp.2006.07.001
Myresiotis, C. K., Vryzas, Z., and Papadopoulou-Mourkidou, E. (2014). Enhanced root uptake of acibenzolar-S-methyl (ASM) by tomato plants inoculated with selected Bacillus plant growth-promoting rhizobacteria (PGPR). Appl. Soil Ecol. 77, 26–33. doi: 10.1016/j.apsoil.2014.01.005
Nakano, R. T., Piślewska-Bednarek, M., Yamada, K., Edger, P. P., Miyahara, M., Kondo, M., et al. (2017). PYK10 myrosinase reveals a functional coordination between endoplasmic reticulum bodies and glucosinolates in Arabidopsis thaliana. Plant J. 89, 204–220. doi: 10.1111/tpj.13377
Nakano, R. T., Yamada, K., Bednarek, P., Nishimura, M., and Hara-Nishimura, I. (2014). ER bodies in plants of the Brassicales order: biogenesis and association with innate immunity. Front. Plant Sci. 5:73. doi: 10.3389/fpls.2014.00073
Nars, A., Rey, T., Lafitte, C., Vergnes, S., Amatya, S., Jacquet, C., et al. (2013). An experimental system to study responses of Medicago truncatula roots to chitin oligomers of high degree of polymerization and other microbial elicitors. Plant Cell Rep. 32, 489–502. doi: 10.1007/s00299-012-1380-3
Newman, M.-A., Sundelin, T., Nielsen, J. T., and Erbs, G. (2013). MAMP (microbe-associated molecular pattern) triggered immunity in plants. Front. Plant Sci. 4:139. doi: 10.3389/fpls.2013.00139
Okubara, P. A., and Paulitz, T. C. (2005). “Root defense responses to fungal pathogens: a molecular perspective,” in Root Physiology: from Gene to Function, eds H. Lambers and T. D. Colmer (Dordrecht: Springer), 215–226. doi: 10.1007/1-4020-4099-7_11
Oren, L., Ezrati, S., Cohen, D., and Sharon, A. (2003). Early events in the Fusarium verticillioides-maize interaction characterized by using a green fluorescent protein-expressing transgenic isolate. Appl. Environ. Microbiol. 69, 1695–1701. doi: 10.1128/AEM.69.3.1695-1701.2003
Papadopoulou, G. V., Maedicke, A., Grosser, K., van Dam, N. M., and Martínez-Medina, A. (2018). Defence signalling marker gene responses to hormonal elicitation differ between roots and shoots. AoB Plants 10:ly031. doi: 10.1093/aobpla/ply031
Paris, F., Krżyaniak, Y., Gauvrit, C., Jamois, F., Domergue, F., Joubès, J., et al. (2016). An ethoxylated surfactant enhances the penetration of the sulfated laminarin through leaf cuticle and stomata, leading to increased induced resistance against grapevine downy mildew. Physiol. Plant. 156, 338–350. doi: 10.1111/ppl.12394
Parra-Lobato, M. C., Fernandez-Garcia, N., Olmos, E., Alvarez-Tinaut, M. C., and Gómez-Jiménez, M. C. (2009). Methyl jasmonate-induced antioxidant defence in root apoplast from sunflower seedlings. Environ. Exp. Bot. 66, 9–17. doi: 10.1016/j.envexpbot.2009.01.002
Perrine-Walker, F. M., Prayitno, J., Rolfe, B. G., Weinman, J. J., and Hocart, C. H. (2007). Infection process and the interaction of rice roots with rhizobia. J. Exp. Bot. 58, 3343–3350. doi: 10.1093/jxb/erm181
Pertot, I., Fiamingo, F., and Elad, Y. (2009). Evaluation of disease control provided by the sar-inducer benzothiadiazole (bion) in strawberry. Acta Hortic. 807, 739–744. doi: 10.17660/ActaHortic.2009.807.111
Pichyangkura, R., and Chadchawan, S. (2015). Biostimulant activity of chitosan in horticulture. Sci. Hortic. 196, 49–65. doi: 10.1016/j.scienta.2015.09.031
Plancot, B., Santaella, C., Jaber, R., Kiefer-Meyer, M. C., Follet-Gueye, M.-L., Leprince, J., et al. (2013). Deciphering the responses of root border-like cells of Arabidopsis and flax to pathogen-derived elicitors. Plant Physiol. 163, 1584–1597. doi: 10.1104/pp.113.222356
Poncini, L., Wyrsch, I., Dénervaud Tendon, V., Vorley, T., Boller, T., Geldner, N., et al. (2017). In roots of Arabidopsis thaliana, the damage-associated molecular pattern AtPep1 is a stronger elicitor of immune signalling than flg22 or the chitin heptamer. PLoS One 12:e0185808. doi: 10.1371/journal.pone.0185808
Pramanik, M. H. R., Nagai, M., Asao, T., and Matsui, Y. (2000). Effects of temperature and photoperiod on phytotoxic root exudates of cucumber (Cucumis sativus) in hydroponic culture. J. Chem. Ecol. 26, 1953–1967. doi: 10.1023/A:1005509110317
Ribeiro, J. (2006). The contribution of extensin network formation to rapid, hydrogen peroxide-mediated increases in grapevine callus wall resistance to fungal lytic enzymes. J. Exp. Bot. 57, 2025–2035. doi: 10.1093/jxb/erj153
Robatzek, S., Chinchilla, D., and Boller, T. (2006). Ligand-induced endocytosis of the pattern recognition receptor FLS2 in Arabidopsis. Genes Dev. 20, 537–542. doi: 10.1101/gad.366506
Sesma, A., and Osbourn, A. E. (2004). The rice leaf blast pathogen undergoes developmental processes typical of root-infecting fungi. Nature 431, 582–586. doi: 10.1038/nature02880
Singh, B. K., Millard, P., Whiteley, A. S., and Murrell, J. C. (2004). Unravelling rhizosphere–microbial interactions: opportunities and limitations. Trends Microbiol. 12, 386–393. doi: 10.1016/j.tim.2004.06.008
Song, Y. Y., Zeng, R. S., Xu, J. F., Li, J., Shen, X., and Yihdego, W. G. (2010). Interplant communication of tomato plants through underground common mycorrhizal networks. PLoS One 5:e13324. doi: 10.1371/journal.pone.0013324
Spletzer, M. E., and Enyedi, A. J. (1999). Salicylic acid induces resistance to Alternaria solani in hydroponically grown tomato. Phytopathology 89, 722–727. doi: 10.1094/PHYTO.1999.89.9.722
Srivastava, P., George, S., Marois, J. J., Wright, D. L., and Walker, D. R. (2011). Saccharin-induced systemic acquired resistance against rust (Phakopsora pachyrhizi) infection in soybean: effects on growth and development. Crop Prot. 30, 726–732. doi: 10.1016/j.cropro.2011.02.023
Sukno, S. A., Garcia, V. M., Shaw, B. D., and Thon, M. R. (2008). Root infection and systemic colonization of maize by Colletotrichum graminicola. Appl. Environ. Microbiol. 74, 823–832. doi: 10.1128/AEM.01165-07
Teixeira, M. A., Wei, L., and Kaloshian, I. (2016). Root-knot nematodes induce pattern-triggered immunity in Arabidopsis thaliana roots. New Phytol. 211, 276–287. doi: 10.1111/nph.13893
Thakur, M., and Sohal, B. S. (2013). Role of elicitors in inducing resistance in plants against pathogen infection: a review. ISRN Biochem. 2013, 1–10. doi: 10.1155/2013/762412
Ton, J., and Mauch-Mani, B. (2004). β -amino-butyric acid-induced resistance against necrotrophic pathogens is based on ABA-dependent priming for callose. Plant J. 38, 119–130. doi: 10.1111/j.1365-313X.2004.02028.x
Torsvik, V., and Ovreas, L. (2002). Microbial diversity and function in soil: from genes to ecosystems. Curr. Opin. Microbiol. 5, 240–245. doi: 10.1016/S1369-5274(02)00324-7
Tran, T., Chen, S., and Wang, X. (2016). Root assays to study pattern-triggered immunity in plant-nematode interactions. Eur. J. Plant Pathol. 147, 955–961. doi: 10.1007/s10658-016-1053-0
Tran, T. M., MacIntyre, A., Hawes, M., and Allen, C. (2016). Escaping underground nets: extracellular DNases degrade plant extracellular traps and contribute to virulence of the plant pathogenic bacterium Ralstonia solanacearum. PLoS Pathog. 12:e1005686. doi: 10.1371/journal.ppat.1005686
Trouvelot, S., Héloir, M.-C., Poinssot, B., Gauthier, A., Paris, F., Guillier, C., et al. (2014). Carbohydrates in plant immunity and plant protection: roles and potential application as foliar sprays. Front. Plant Sci. 5:592. doi: 10.3389/fpls.2014.00592
Tytgat, T. O. G., Verhoeven, K. J. F., Jansen, J. J., Raaijmakers, C. E., Bakx-Schotman, T., McIntyre, L. M., et al. (2013). Plants know where it hurts: root and shoot jasmonic acid induction elicit differential responses in Brassica oleracea. PLoS One 8:e65502. doi: 10.1371/journal.pone.0065502
Vicedo, B., Flors, V., de la O Leyva, M., Finiti, I., Kravchuk, Z., Real, M. D., et al. (2009). Hexanoic acid-induced resistance against Botrytis cinerea in tomato plants. Mol. Plant Microbe Interact. 22, 1455–1465. doi: 10.1094/MPMI-22-11-1455
Walters, D. R., Ratsep, J., and Havis, N. D. (2013). Controlling crop diseases using induced resistance: challenges for the future. J. Exp. Bot. 64, 1263–1280. doi: 10.1093/jxb/ert026
Watt, M., Silk, W. K., and Passioura, J. B. (2006). Rates of root and organism growth, soil conditions, and temporal and spatial development of the rhizosphere. Ann. Bot. 97, 839–855. doi: 10.1093/aob/mcl028
Wen, F., VanEtten, H. D., Tsaprailis, G., and Hawes, M. C. (2006). Extracellular proteins in pea root tip and border cell exudates. Plant Physiol. 143, 773–783. doi: 10.1104/pp.106.091637
Wojtasik, W., Kulma, A., Kostyn, K., and Szopa, J. (2011). The changes in pectin metabolism in flax infected with Fusarium. Plant Physiol. Biochem. 49, 862–872. doi: 10.1016/j.plaphy.2011.03.002
Wyrsch, I., Domínguez-Ferreras, A., Geldner, N., and Boller, T. (2015). Tissue-specific FLAGELLIN-SENSING 2 (FLS2) expression in roots restores immune responses in Arabidopsis fls2 mutants. New Phytol. 206, 774–784. doi: 10.1111/nph.13280
Zambounis, A. G., Kalamaki, M. S., Tani, E. E., Paplomatas, E. J., and Tsaftaris, A. S. (2012). Expression analysis of defense-related genes in cotton (Gossypium hirsutum) after Fusarium oxysporum f. sp. vasinfectum infection and following chemical elicitation using a salicylic acid analog and methyl jasmonate. Plant Mol. Biol. Rep. 30, 225–234. doi: 10.1007/s11105-011-0335-0
Zhang, Y., Lubberstedt, T., and Xu, M. (2013). The genetic and molecular basis of plant resistance to pathogens. J. Genet. Genomics 40, 23–35. doi: 10.1016/j.jgg.2012.11.003
Zhao, X., Schmitt, M., and Hawes, M. C. (2000). Species-dependent effects of border cell and root tip exudates on nematode behavior. Phytopathology 90, 1239–1245. doi: 10.1094/PHYTO.2000.90.11.1239
Zhu, Y. J., Qiu, X., Moore, P. H., Borth, W., Hu, J., Ferreira, S., et al. (2003). Systemic acquired resistance induced by BTH in papaya. Physiol. Mol. Plant Pathol. 63, 237–248. doi: 10.1016/j.pmpp.2004.03.003
Zimmerli, L., Jakab, G., Metraux, J.-P., and Mauch-Mani, B. (2000). Potentiation of pathogen-specific defense mechanisms in Arabidopsis by beta -aminobutyric acid. Proc. Natl. Acad. Sci. U.S.A. 97, 12920–12925. doi: 10.1073/pnas.230416897
Keywords: elicitor, systemic acquired resistance, root immunity, root border cells, environment
Citation: Chuberre C, Plancot B, Driouich A, Moore JP, Bardor M, Gügi B and Vicré M (2018) Plant Immunity Is Compartmentalized and Specialized in Roots. Front. Plant Sci. 9:1692. doi: 10.3389/fpls.2018.01692
Received: 15 June 2018; Accepted: 31 October 2018;
Published: 28 November 2018.
Edited by:
Simone Ferrari, La Sapienza University of Rome, ItalyReviewed by:
Marc Libault, University of Oklahoma, United StatesCopyright © 2018 Chuberre, Plancot, Driouich, Moore, Bardor, Gügi and Vicré. This is an open-access article distributed under the terms of the Creative Commons Attribution License (CC BY). The use, distribution or reproduction in other forums is permitted, provided the original author(s) and the copyright owner(s) are credited and that the original publication in this journal is cited, in accordance with accepted academic practice. No use, distribution or reproduction is permitted which does not comply with these terms.
*Correspondence: Bruno Gügi, YnJ1bm8uZ3VnaUB1bml2LXJvdWVuLmZy Maïté Vicré, bWFpdGUudmljcmVAdW5pdi1yb3Vlbi5mcg==
†These authors have contributed equally to this work
Disclaimer: All claims expressed in this article are solely those of the authors and do not necessarily represent those of their affiliated organizations, or those of the publisher, the editors and the reviewers. Any product that may be evaluated in this article or claim that may be made by its manufacturer is not guaranteed or endorsed by the publisher.
Research integrity at Frontiers
Learn more about the work of our research integrity team to safeguard the quality of each article we publish.