- 1Enzyme Technology, Department of Bioengineering and Biomedicine, Technical University of Denmark, Lyngby, Denmark
- 2Enzyme and Protein Chemistry, Department of Bioengineering and Biomedicine, Technical University of Denmark, Lyngby, Denmark
Biosynthesis of starch is catalyzed by a cascade of enzymes. The activity of a large number of these enzymes depends on interaction with polymeric substrates via carbohydrate binding sites, which are situated outside of the catalytic site and its immediate surroundings including the substrate-binding crevice. Such secondary binding sites can belong to distinct starch binding domains (SBDs), classified as carbohydrate binding modules (CBMs), or be surface binding sites (SBSs) exposed on the surface of catalytic domains. Currently in the Carbohydrate-Active enZYmes (CAZy) database SBDs are found in 13 CBM families. Four of these families; CBM20, CBM45, CBM48, and CBM53 are represented in enzymes involved in starch biosynthesis, namely starch synthases, branching enzymes, isoamylases, glucan, water dikinases, and α-glucan phosphatases. A critical role of the SBD in activity has not been demonstrated for any of these enzymes. Among the well-characterized SBDs important for starch biosynthesis are three CBM53s of Arabidopsis thaliana starch synthase III, which have modest affinity. SBSs, which are overall less widespread than SBDs, have been reported in some branching enzymes, isoamylases, synthases, phosphatases, and phosphorylases active in starch biosynthesis. SBSs appear to exert roles similar to CBMs. SBSs, however, have also been shown to modulate specificity for example by discriminating the length of chains transferred by branching enzymes. Notably, the difference in rate of occurrence between SBDs and SBSs may be due to lack of awareness of SBSs. Thus, SBSs as opposed to CBMs are not recognized at the protein sequence level, which hampers their identification. Moreover, only a few SBSs in enzymes involved in starch biosynthesis have been functionally characterized, typically by structure-guided site-directed mutagenesis. The glucan phosphatase Like SEX4 2 from A. thaliana has two SBSs with weak affinity for β-cyclodextrin, amylose and amylopectin, which were indicated by mutational analysis to be more important than the active site for initial substrate recognition. The present review provides an update on occurrence of functional SBDs and SBSs in enzymes involved in starch biosynthesis.
Introduction
Starch is an insoluble polymer composed of two α-glucans: the branched amylopectin, containing α-1,4-, and α-1,6-linked glucose units; and amylose, an essentially linear α-1,4-glucan. During biosynthesis starch is deposited as supramolecular, semicrystalline granules [see (Vamadevan and Bertoft, 2015) for a recent review on starch]. Despite the chemical simplicity of the two polysaccharides, starch biosynthesis is a complicated process that involves several enzyme specificities from the initial precursor formation until completion of the starch granule: ADP-glucose pyrophosphorylase, starch synthase (soluble and granule bound), starch branching enzyme, starch debranching enzyme (isoamylase), and enzymes for phosphorylation and dephosphorylation. Most of the enzymes occur in more than one isoform (see (Pfister and Zeeman, 2016; Tetlow and Emes, 2017; Goren et al., 2018) for recent reviews on the starch structure and biosynthesis) showing the same activity, but having different substrate preferences, and catalytic efficiency. In some cases, the variations may be explained by how the enzymes interact with their substrates, which might be nature’s way of fine-tuning the intricate starch granule formation processes. In plantae experiments have shown that a few mutations in one of the enzymes involved in starch biosynthesis can affect the structure and functional properties of the produced polysaccharides. These mutations can be situated far from the active site and may lead to loss of carbohydrate binding ability. Some enzymes possess CBMs, i.e., non-catalytic domains with carbohydrate binding sites which are connected to catalytic modules, sometimes through polypeptide linkers. The CBMs are grouped into families in the Carbohydrate-Active enZYmes (CAZy) database based on the amino acid sequence (Boraston et al., 2004; Lombard et al., 2014). Currently starch binding domains (SBDs) are found in 13 CBM families. Four of these families (CBM20, CBM45, CBM48, and CBM53) are represented in enzymes involved in starch biosynthesis, namely starch synthases, branching enzymes, isoamylases, glucan, water dikinases, and α-glucan phosphatases (Table 1). Surface binding sites (SBSs), capable of interacting with carbohydrates and exposed on the surface of catalytic domains at a certain distance from the active site or on modules intimately associated with catalytic domains (Cuyvers et al., 2011; Cockburn and Svensson, 2013; Cockburn et al., 2014) have also been identified in above mentioned enzymes (Table 1). The present review focuses on enzymes in starch biosynthesis, which have been shown experimentally to possess functional CBMs (SBDs) or SBSs.
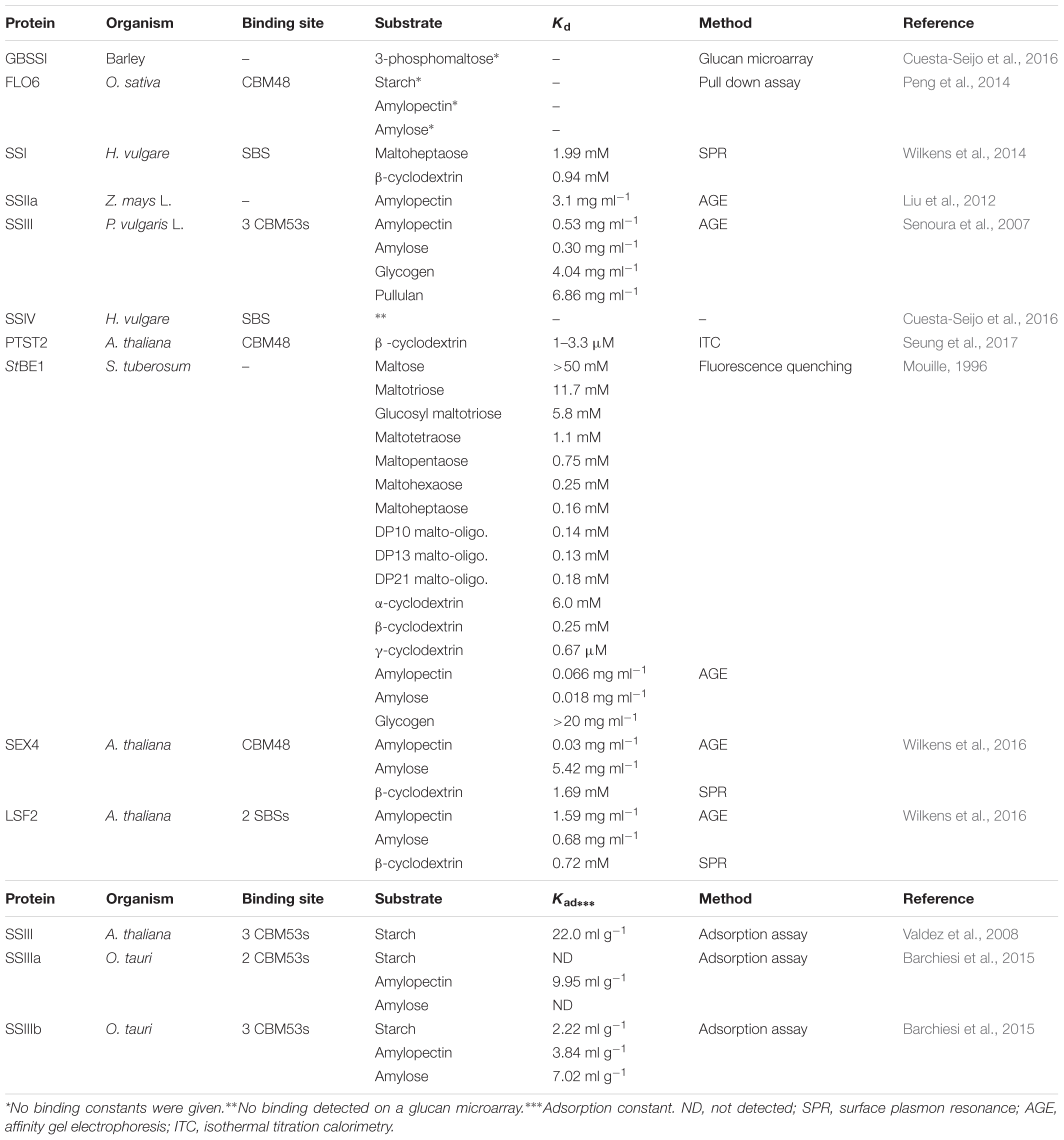
TABLE 1. Binding data for full-length enzymes and interacting proteins involved in starch biosynthesis.
Starch Synthases
Starch synthases (SSs; EC 2.4.1.21) catalyze transfer of glucose from the soluble precursor ADP-glucose to the non-reducing end of an α-1,4-glucan primer or growing chain. SSs belong to glycosyl transferase family 5 (GT5) and contain also a GT1 domain (Pfister and Zeeman, 2016). The GT1 domain is involved in interaction with other proteins (Seung et al., 2017). Five SSs exist: granule-bound SS (GBSS), solely responsible for biosynthesis of amylose (Tetlow and Emes, 2017), and four soluble SSs (SSI, SSII, SSIII, and SSIV) thought to be exclusively involved in amylopectin biosynthesis. In cereals the individual SSs have unique roles, each form predominantly synthesizing chains of different lengths. SSI specifically acts in synthesis of short chains in amylopectin (Delvallé et al., 2005), while SSII and SSIII have a major role in amylopectin synthesis producing chains of short to intermediate length (Zhang et al., 2005; Goren et al., 2018), and SSIV is involved in the initiation of the starch granule formation and control of the number of starch granules in the chloroplast (Roldán et al., 2007; Szydlowski et al., 2009; Crumpton-Taylor et al., 2013) (see Sections “Granule Bound SSs - SSIV”below). The different SSs use individual strategies for interacting with starch and related carbohydrates. Some have CBMs, while others have distinct SBSs. Yet other enzymes contain none of these structural features, but interact with specific proteins, which in turn interact with starch.
Granule Bound SSs
Plant
Two isoforms of GBSSs belonging to GT5 are found in cereals and have no identified CBM or SBS. Barley GBSSI did not bind to starches or polysaccharides sampled in a glucan microarray analysis; the only detected binding being to 3-phosphomaltose (Cuesta-Seijo et al., 2016). Recently, however, it was shown that the localization of GBSSI to starch granules and normal amylose synthesis depended on interaction with another protein, the so-called PROTEIN TARGETING TO STARCH (PTST) 1 that is a non-catalytic protein containing coiled-coils and a CBM48. PTST interacts with the C-terminal GT1 domain of GBSS (Seung et al., 2015). PTST1 was first identified in Arabidopsis, but appears to exist in all plant species (Lohmeier-Vogel et al., 2008; Seung et al., 2015). Two additional plastidial PTSTs (PTST2 and PTST3) were identified in Arabidopsis thaliana leaves. PTST2 is an ortholog to the CBM48-containing FLOURY ENDOSPERM6 (FLO6) from rice (Oryza sativa) endosperm that influences grain starch content, granule morphology, and starch physico-chemical properties. The CBM48 of FLO6 was shown to bind to starch as well as amylopectin and amylose. FLO6 also interacts with the rice debranching enzyme isoamylase 1 that does not bind starch directly (Peng et al., 2014). PTST2 and PTST3 are proposed to interact with SSIV in Arabidopsis leaves (Seung et al., 2017), see Section “SSIV” below.
Cyanobacterium sp. CLg1
Very recently, an SBS was identified in the crystal structure of the GT5 granule bound starch synthase from the Cyanobacterium sp. CLg1 (CLg1GBSS). CLg1GBSS crystallized as a trimer and on molecule B two planar electron densities corresponding to maltose was present out side the active site (Figure 1). At molecule A and C the putative SBS interacts with the His6 purification tag (Nielsen et al., 2018), which poses a steric hindrance for the maltose. However, a mutational analysis is needed to confirm the SBSs impact on CLgGBSS activity.
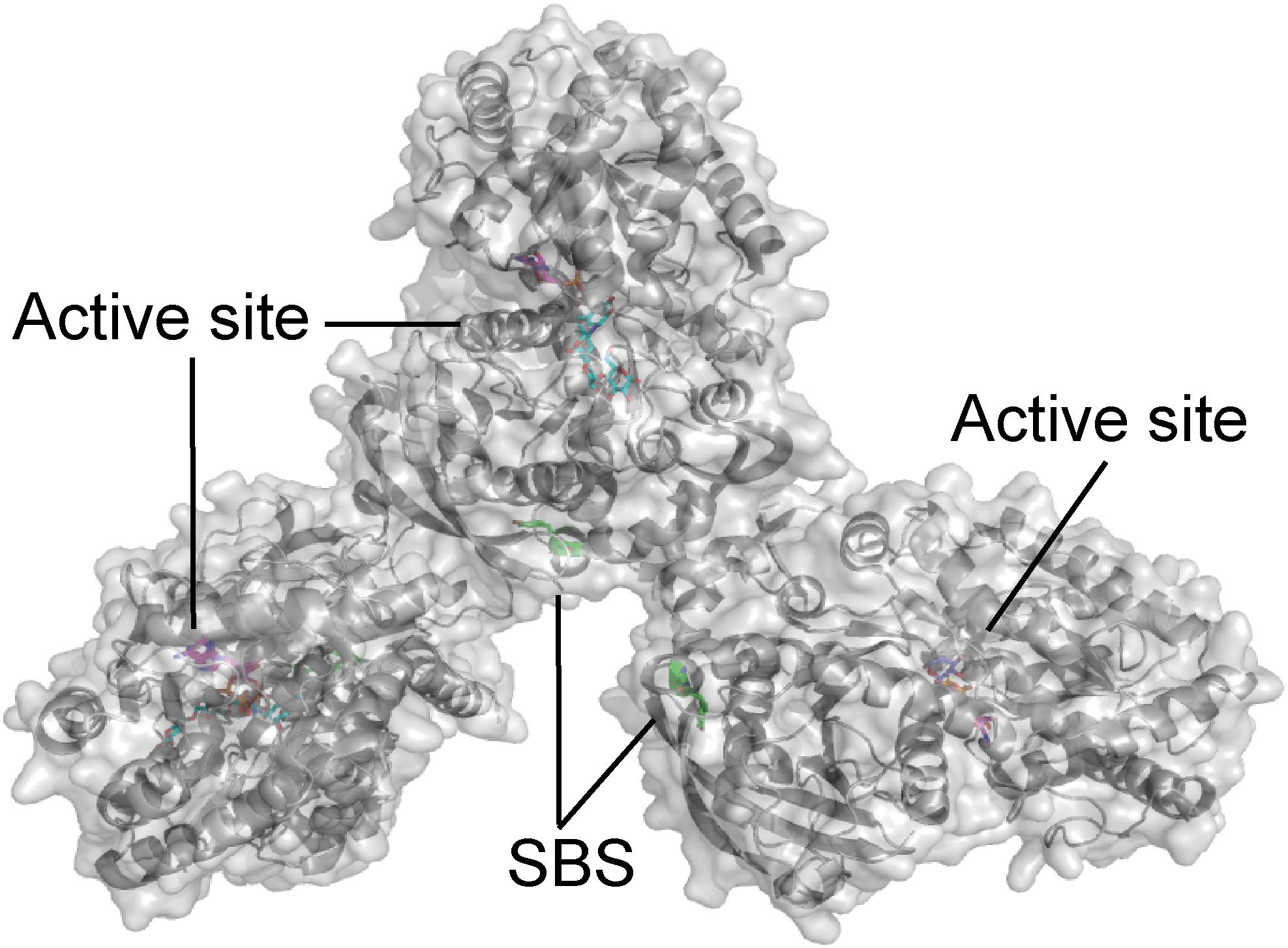
FIGURE 1. Cyanobacterium sp. CLg1 (CLg1GBSS) granule bound starch synthase trimer (gray) in complex with acarbose (cyan), ADP (pink) and glucose (purple), and the residues at the putative surface binding site shown as sticks (green) (PDB entry 6GNF).
Soluble SSs
SSI
The structure of barley SSI revealed a maltooligosaccharide-binding SBS situated 30 Å from the active site (Figure 2) and which was suggested based on mutational analysis to be involved in recognition of soluble starch and glycogen (Cuesta-Seijo et al., 2013). The SBS binds maltopentaose, -hexaose, -heptaose, and β-cyclodextrin (β-CD) as shown by surface plasmon resonance (SPR) analysis. However, Kd was only measurable for maltoheptaose and β-CD being 1.99 mM and 0.94 mM, respectively. An SBS mutant lost ability to bind β-CD and maltooligosaccharides, which indicated the SBS has higher affinity than the active site (Wilkens et al., 2014). Binding to recombinant wild type and truncated forms of SSI from maize (Zea mays L.) was analyzed by affinity gel electrophoresis (AGE) yielding Kd of 0.49 and 0.20 mg/ml for starch and amylopectin, respectively (Commuri and Keeling, 2001). Analysis of truncated forms and polypeptides (7–52 kDa) derived from wild type SSI by hydrolysis with trypsin indicated the N-terminal extension is neither required for catalysis nor for affinity and that a minimum size of 52 kDa (full-length is 64 kDa) was needed for catalysis and starch binding. Furthermore, removal either of approx. 50 amino acid residues from the C-terminus or a part of the N-terminal domain including a KSGGLGDV motif led to loss of activity and starch binding (Commuri and Keeling, 2001). The native SSI from maize binds amylopectin with a Kd of 2.5 mg/ml (Liu et al., 2012).
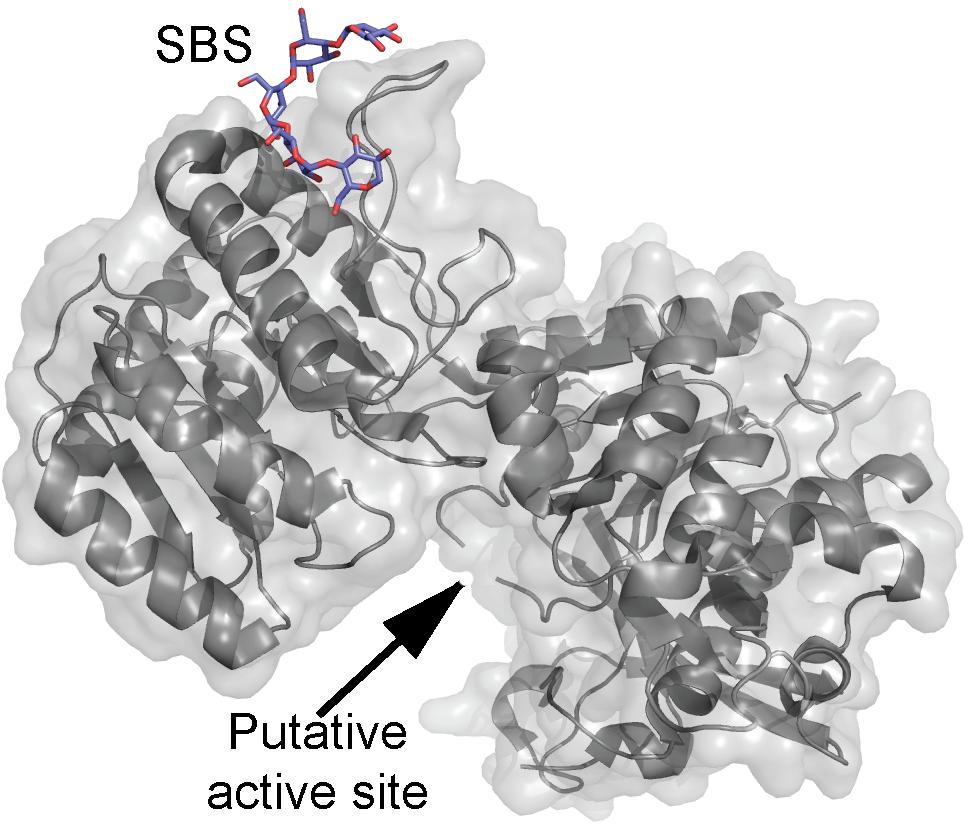
FIGURE 2. Barley soluble starch synthase I structure (gray) in complex with maltopentaose (purple) (PDB entry 4HLN).
SSII
SSII is thought to be important for the formation of chains with intermediate lengths (DP12–25), since SSII deficiency in numerous species results in increased frequency of short glucan chains (DP6–DP11) within amylopectin, decreased abundance of DP12 to DP25 chains, and in most instances an elevated amylose to amylopectin ratio (Zhang et al., 2008). Furthermore, SSIIa in maize plays a crucial role in trafficking SSI and starch branching enzyme IIb (SBEIIb) into the granule matrix (Liu et al., 2012). The sugary-2 mutation in maize (Zea mays L.), which causes major alterations to amylopectin architecture, is due to a catalytically inactive form of the endosperm-specific SSIIa having two amino acid substitutions (one outside the GT domains and one in the GT domains) and being unable to bind amylopectin. Thus, while wild type SSIIa has a Kd of 3.1 mg/ml for amylopectin no binding was observed of the sugary-2 mutant using AGE (Liu et al., 2012).
SSIII
SSIIIs contain one or more CBM53s and are the best studied SSs with regard to carbohydrate binding. In the CAZy database (Lombard et al., 2014), 133 protein sequences are classified as CBM53. Most of these are found in SSIIIs, which usually have three CBM53s in tandem and therefore the number of unique proteins containing CBM53 is quite low. Three archaeal Thermococci GH enzymes each with one CBM53 are not classified in CAZy. No structure is available of CBM53 (Lombard et al., 2014). Among the SSIIIs with characterized CBM53s are SSIII from A. thaliana (AtSSIII) (Valdez et al., 2008, 2011), two SSIIIs from Osterococcus tauri (OtSSIII-A and OtSSIII-B) (Barchiesi et al., 2015), and SSIII from kidney bean (Phaseolus vulgaris L.; PvSSIII).
AtSSIII has three N-terminal CBM53s in tandem, which preferentially bind to amylose, the first CBM53 being mainly responsible for this selective binding (Valdez et al., 2008, 2011). The second CBM53 has two binding sites, containing Y394 (binding site 1), and W366 (binding site 2), which act cooperatively with the first CBM53 in starch binding. Mutations in these sites affect kinetic parameters of AtSSIII toward a polysaccharide substrate (Wayllace et al., 2010; Valdez et al., 2011). Recently, in vitro experiments showed that SBDs of AtSSIII preferentially bind to cell wall polysaccharides over starch. Recombinantly produced AtSSIII comprising the three AtCBM53s thus binds to xylan and pectins with two-fold and to cellulose with 2.4-fold higher affinity than to starch (Grisolia et al., 2017). Amino acid residues at the second CBM53 were found to be important for the binding to plant cell wall polysaccharides, Y394 (binding site 1) being the most critical. Finally, transgenic plants overexpressing the three CBM53s in the cell wall are larger than wild type and have altered cell wall components (Grisolia et al., 2017).
The unicellular green alga, O. tauri, has three SSIII isoforms, OtSSIII-A, OtSSIII-B, and OtSSIII-C, which according to the CAZy database contain two, three, and no CBM53, respectively (Lombard et al., 2014). Phylogenetic analysis showed that OtSSIII-B is more closely related to higher plant SSIIIs, such as AtSSIII (Barchiesi et al., 2015). The CBM53s from OtSSIII-A and OtSSIII-B were recombinantly produced individually and in tandem and their ability to bind starch, amylose and amylopectin was evaluated. All individual OtCBM53s interacted at different levels with amylose and amylopectin in co-sedimentation assays, except for the first CBM53 from OtSSIII-A that lacked both residues equivalent to those important for binding in the second AtSSIII CBM53 (Barchiesi et al., 2015). The tandem CBM53s from OtSSIII-A were able to bind amylose, but not amylopectin. Furthermore, starch binding was only observed for the first CBM53 of OtSSIII-B and all three OtSSIII-B CBM53s together. The results from co-sedimentation was supported by an adsorption assay using starch, amylose, or amylopectin (Barchiesi et al., 2015).
The third CBM53 from barley (Hordeum vulgare) SSIII (HvSSIII) has been recombinantly produced and shown by glucan microarray analysis to bind to some starches, but not to any of the linear and branched oligosaccharides tested, while strong binding was observed to 3-phosphomaltose, which mimics the 3-phosphorylation of starch found in vivo (Cuesta-Seijo et al., 2016).
Recombinant full-length SSIII from kidney bean (Phaseoulus vulgaris L.) (PvSSIII), as well as its N-terminal and catalytic domains on their own, have been characterized with regard to enzymatic activity and binding to amylose and amylopectin (Senoura et al., 2007). The N-terminal region of PvSSIII is predicted to contain three CBM53s like other characterized SSIIIs. These N-terminal CBM53s were not essential for catalysis and had moderate effect on thermostability and pH stability. However, deletion of the CBM53s drastically decreased affinity and catalytic efficiency on glucan primers (Senoura et al., 2007). AGE indicated that N-terminal CBM53s have high affinity for amylose and amylopectin. Kd values for binding amylose, amylopectin, glycogen, and pullulan were similar for full-length PvSSIII and N-terminal CBM53s alone indicating the importance of CBM53s in polysaccharide binding. Kd was in the range from 0.30 to 6.88 mg/ml for the four polysaccharides (Senoura et al., 2007).
SSIV
Interestingly, barley SSIV did not bind to any of the starches and polysaccharides included in a glucan microarray (Cuesta-Seijo et al., 2016). However, the non-catalytic, CBM48-containing PTST2 and PTST3 proteins are proposed to interact with SSIV in Arabidopsis leaves, and play a critical role in starch granule initiation by delivering suitable glucan primers to SSIV. PTST2 was shown by isothermal titration calorimetry (ITC) analysis to interact with β-CD (Kd ranged from 1 to 3.3 μM), and the affinity of CBM48 alone with β-CD was very similar (Kd ranged from 1.7 to 4 μM). Injection of β-CD into an equimolar mixture of PTST2 and maltoheptaose resulted in similar heat changes as when PTST2 was analyzed alone, indicating that maltoheptaose and β-CD occupy different binding sites. On the other hand, when the experiment was repeated with maltodecaose together with PTST2, significant variations were observed, while the Kd was in the same range as for PTST2 alone. This suggested that the ability of maltodecaose to adopt a structure mimicking helical amylose chains is important for binding long maltooligosaccharides to CBM48 of PTST2 (Seung et al., 2017). Very recently a crystal structure was published of GT5 A. thaliana starch synthase IV (AtSSIV) in complex with the inhibitor acarbose, which showed an SBS (Figure 3) located in the same area as the SBS on the GT5 CLg1GBSS (Figure 1). AtSSIV crystallized as a dimer and the SBS was only occupied on molecule A and electron density was only present for a part of acarbose. Crystal contacts of molecule B blocked for glucan interactions (Nielsen et al., 2018). Unfortunately, a mutational analysis was not performed, so the role of the SBS remains enigimatic.
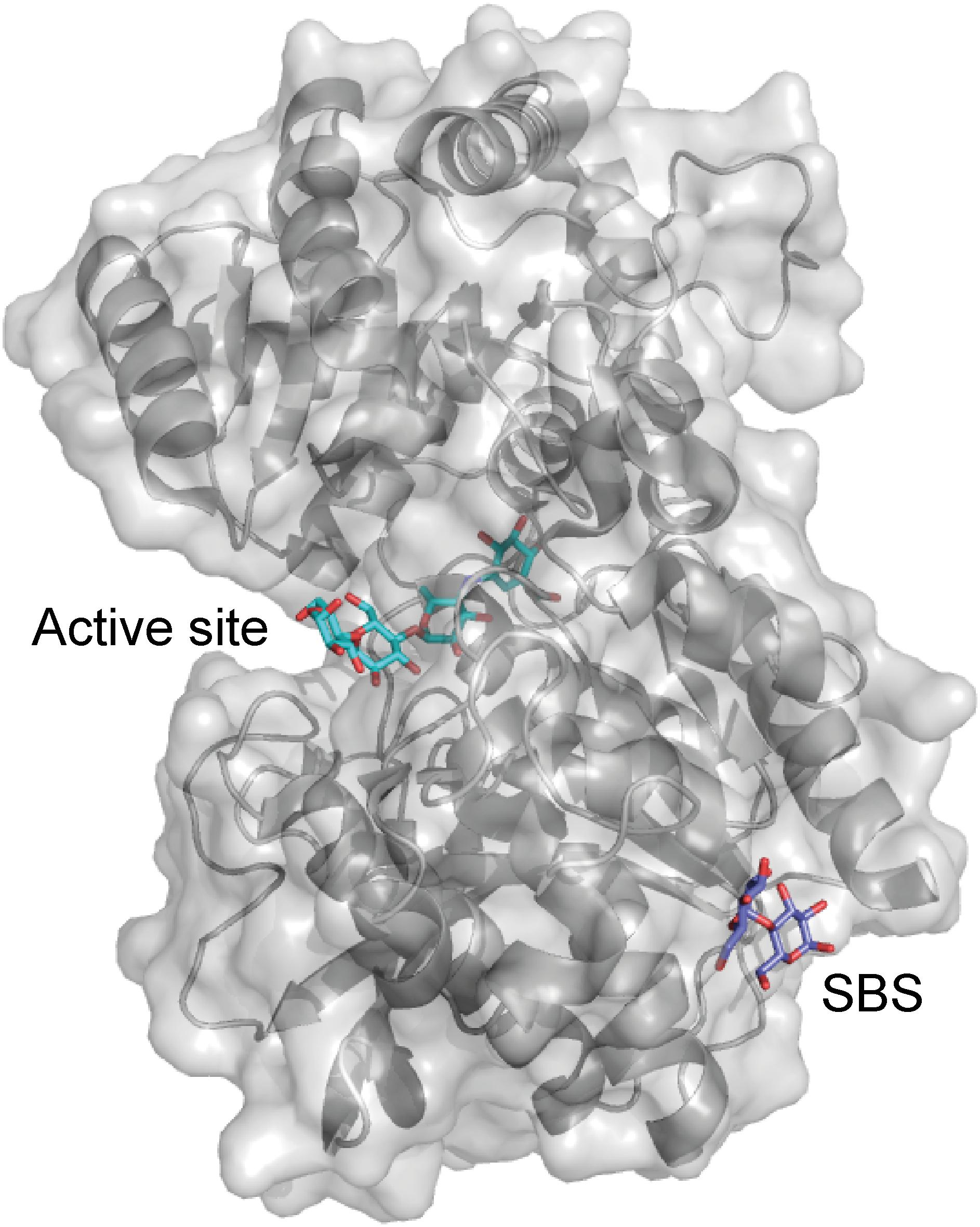
FIGURE 3. Arabidopsis thaliana starch synthase IV in complex with the inhibitor acarbose (cyan) and maltose (purple) (PDB entry 6GNE).
Starch Branching Enzymes
Starch branching enzymes (SBEs; EC 2.4.1.18) catalyze formation of α-1,6-glucosidic linkages creating branch points in amylopectin and are crucial in determining structural and physical properties of starch granules (see (Tetlow and Emes, 2014) for a review on SBEs and their role in starch biosynthesis). SBEs catalyze transglycosylation reactions involving cleavage of internal α-1,4-glucosidic linkages, and transfer of products from the substrate chain toward the non-reducing end to C-6 hydroxyl groups. Multiple forms of SBEs are required for starch biosynthesis in plants and algae, unlike in glycogen synthesizing prokaryotes and eukaryotes, which have a single BE. The SBE isoforms show wide variation in chain-length transfer pattern related to their glucan substrate preferences (Tetlow and Emes, 2014). Starch BEs belong to GH13 subfamilies 8 (eukaryotic BEs; GH13_8) and 9 (bacterial BEs; GH13_9) (Stam et al., 2006); glycogen BEs are also found in GH57 (Janeček et al., 2014). GH13 BEs according to CAZy are multimodular enzymes generally including an N-terminal CBM48 (Lombard et al., 2014). Three-dimensional structures of BEs revealed the presence of both CBM48 binding sites and SBSs.
Potato Tuber Starch BE1
A thorough analysis of StBE1 binding to a wide range of maltooligosaccharides by using tryptophan fluorescence quenching showed increasing affinity from maltose (Kd > 50 mM) to a maltooligosaccharide with degree of polymerization (DP) 13 (Kd of 0.13 mM) (Table 1). Notably, Kd was 0.18 mM for a maltodextrin of DP 21. It was demonstrated that maltooligosaccharides of DP below 20 were poor substrates. Binding of amylose and amylopectin yielded Kd of 0.018 and 0.066 mg ml-1, respectively, indicating that StBE1 has a substrate preference for unbranched chains. Ten-fold lower affinity for maltooligosaccharides was obtained by AGE in competition with amylose and amylopectin than by using tryptophan fluorescence quenching, which suggests the presence of a tryptophan-containing binding site different from the active site (Blennow et al., 1998).
AGE binding analysis of amylose and amylopectin to trypsin-digested StBE1 suggested that the C-terminal part is not important for starch binding (Blennow et al., 1998). This is surprising, because several SBSs as mentioned below are found in this region of other characterized BE1s.
Cyanobacterial BE1
Crystal structures of a BE from Cyanothece sp. ATCC 51142 (CyBE) in complex with maltohexaose and maltoheptaose were published recently (Hayashi et al., 2017). Unlike most cyanobacteria, Cyanothece sp. ATCC 51142 produces an amylopectin-like polysaccharide designated as cyanobacterial starch (Hayashi et al., 2017). Glycogen producing cyanobacteria usually have one GH13_9 BE and one GH57 BE. By contrast Cyanothece sp. ATCC 51142 contains three GH13_9 BEs and one GH57 BE (Suzuki et al., 2013; Suzuki and Suzuki, 2016). The three GH13_9 BE isoforms have been characterized and CyBE1 and CyBE2 transferred short glucans (DP 6–7), while CyBE3 transfers short as well as long glucan chains (DP 30) (Suzuki et al., 2015). The chain length preferences of CyBE1, CyBE2 and the SBE2b isoform of rice (O. sativa L.) were similar, while the CyBE3 specificity was similar to that of rice BE1 (Suzuki et al., 2015).
The crystal structure of CyBE1 with maltoheptaose at the active site revealed seven additional binding sites (Figure 4A; Hayashi et al., 2017). The architecture of CyBE1 was unprecedented among BEs and consists of domain N, CBM48, and the catalytic domain common for all GH13s known as domain A, and domain C (Figure 4A). Two binding sites were situated on CBM48 and the domain C contained three SBSs (Figure 4A). The functional role of these five binding sites was not investigated, but mutation of two SBSs on domain A (A1 and A2) (Figure 4A) resulted in up to 50% loss of activity with unchanged chain length profile for A1 mutants. By contrast A2 mutants showed changes in the relative proportions of the products and up to 90% decrease in activity. On the basis of these results, the maltoheptaose orientation at A1 and A2, as well as the A1 and A2 locations, the authors suggested that A1 functions as entrance for the α-glucan chain to the acceptor binding site where the new branch is transferred to the chain coming in via A1. A2 functions as an exit for the chain coming in via A1 and is also suggested to be responsible for discriminating the length of the incoming chains (Hayashi et al., 2017).
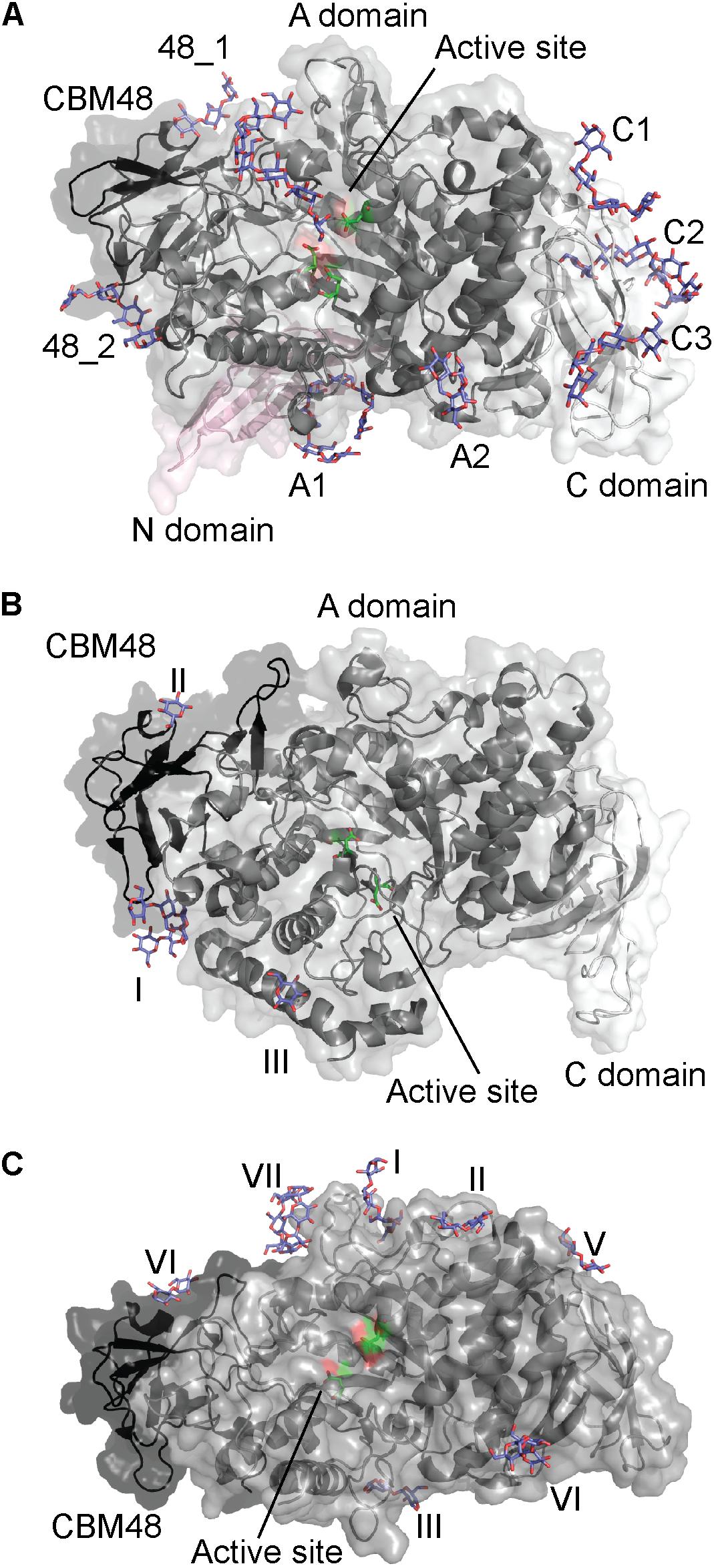
FIGURE 4. Starch branching enzymes from (A) Cyanothece sp. ATCC 51142 in complex with maltoheptaose (PDB entry 5GQX), (B) O. sativa in complex with maltopentaose (PDB entry 3VU2) and (C) E. coli in complex with maltoheptaose and α-cyclodextrin (PDB entries 4LPC and 5E6Y). The active site residues are shown as green sticks and all glucans in purple.
Rice BE1
Excluding the N-domain, GH13_9 BE1 from rice (O. sativa; OsBE1) has a similar domain architecture to CyBE1 (Figure 4B; Hayashi et al., 2017). The OsBE1 complex structure with maltopentaose reveals two binding sites on the CBM48 (Figure 4B), which are also occupied in CyBE1 (Chaen et al., 2012; Hayashi et al., 2017). In addition, an SBS was observed on domain A (Figure 4B). A large number of residues interacting with maltopentaoses are conserved (Chaen et al., 2012), suggesting that this SBS has a functional role. However, mutational experiments are needed to describe the various functional roles.
Escherichia coli BE1
GH13_9 BE1 from Escherichia coli (EcBE1) resembles CyBE1 in domain architecture, but does not contain the N-domain (Figure 4C; Hayashi et al., 2017). EcBE1 has been crystallized in complex with α-, β-, and γ-cyclodextrins, maltohexaose and maltoheptaose, but not all ligands are seen to bind to all binding sites in EcBE1. Further, EcBE1 crystallized as a tetramer and the binding sites are not occupied on all four EcBE1 monomers (Figure 4C). Of the seven EcBE1binding sites, binding site I is at a distance of approximately 18 Å from and the closest to the active site (Feng et al., 2015, 2016).
A binding site corresponding to CBM48_1 of CyBE1 was observed for EcBE1 (binding site IV), while the remaining 6 sites are SBSs some of which (Figure 4C) are corresponding to A1, A2 and C1 on CyBE1 (Feng et al., 2015; Hayashi et al., 2017). In addition, binding site I, occupied by linear maltooligosaccharides (Feng et al., 2015), and binding site VII, only occupied by α- and γ-CDs (Feng et al., 2016), were only seen in EcBE1. For linear maltooligosaccharides to bind at binding site VII they would have to adopt a curved conformation similar to the CDs. Notably, CDs were only observed at binding sites IV–VI, which suggests that binding sites I–III are unable to accommodate the curved ligands (Feng et al., 2016). Binding sites I and II (Figure 4C) apparently accommodate maltotetraose and maltose, respectively, but their orientation suggests that they together represent a maltoheptaose molecule. Binding sites III and VI are located close to where glucan chains typically exit GH13 enzymes. Mutations in binding sites I, II, VI resulted in up to 84, 24, and 86% reduced activity, respectively (Feng et al., 2015), which clearly demonstrates their importance for the function of EcBE1. Mutation of binding site VII led to loss of up to 92% activity (Feng et al., 2016).
EcBE1, similarly to potato (Solanum tuberosum) tuber starch BE1 (StBE1) described above (Blennow et al., 1998), transfers maltohexaose and larger maltooligosaccharides to polymeric substrates (Binderup et al., 2000). The EcBE1 active site is unoccupied in all published complex structures (Feng et al., 2015, 2016), and considered to have poor affinity for shorter linear and circular maltooligosaccharides. This probably prevents EcBE1 from transferring short branches onto relatively short polymers. If this is true, the SBSs and the CBM48 binding site seem to have distinct roles. Binding sites I, II, and IV (on CBM48) could bind the glucan chain entering the active site, and III and VI could bind the glucan chain exiting the active site (Feng et al., 2015).
Starch Debranching Enzymes
The starch debranching enzymes (DBEs) hydrolyze α-1,6-glycosidic linkages at branch points in amylopectin and oligosaccharides derived thereof. DBEs play a role during amylopectin biosynthesis and degradation. Two types of starch DBEs are found in plants, the isoamylase-type (EC 3.2.1.68) and the pullulanase-type (EC 3.2.1.41). While at least three isoforms exist of the isoamylase-type, the pullulanase-type occurs as a single form, known as limit dextrinase. Both belong to GH13 but are categorized into different subfamilies with distinct specificities; isoamylases are found in GH13 subfamily 11 (GH13_11), and pullulanase-type DBEs are found in GH13 subfamilies 12–14 (GH13_12–14) with the plant pullulanases being found solely in GH13 subfamily 13 (Møller et al., 2016). Isoamylases were hypothesized to trim misplaced branches in amylopectin, which otherwise will prevent adjacent linear chains from associating and crystallizing during the biosynthesis (Myers et al., 2000; Nakamura, 2002; Jeon et al., 2010; Goren et al., 2018). The function of pullulanase-type DBE during starch synthesis is less understood (Li et al., 2017). However, besides during germination, substantial pullulanase activity has been detected in developing rice and maize endosperms (Nakamura, 1996; Beatty et al., 1999), and the presence of mRNA for barley limit dextrinase was found in this stage of the plant lifecycle (Burton et al., 1999). A recent study has shown that variations in the gene encoding the pullulanase type DBE in Sorghum bicolor result in starch with better digestability (Gilding et al., 2013).
Plant DBEs are multimodular possessing at least a CBM48 in addition to the catalytic domain A and the C-domain typical of GH13. In most CBM48s from GH13_11–14 DBEs, residues predicted to constitute a canonical SBS are not fully conserved. Carbohydrate binding to CBM48 has not been demonstrated experimentally for isoamylase- and pullulanase-type DBEs (Malle et al., 2006; Mikami et al., 2006; Gourlay et al., 2009; Vester-Christensen et al., 2010; Lammerts van Bueren et al., 2011; Møller et al., 2015), but CBM48s from these enzymes possess a conserved tryptophan corresponding to Trp563 in SBS2 of a closely related CBM20 in Aspergillus niger glucoamylase, suggesting that plant DBE CBM48s may be intermediates between CBM20 and CBM48 (Janeček et al., 2011; Møller et al., 2016).
Rice Isoamylase
Rice (O. sativa) isoamylase 1 (OsISA1) does not bind starch directly, but it can interact both in vivo and in vitro with the CBM48-containing FLO6 protein (see also Section “Plant”). Hence, FLO6 might assist binding of OsISA1 to starch (Peng et al., 2014). Recently, a point mutation in the barley gene Fra corresponding to FLO6 was shown to cause fractured starch granules (Saito et al., 2018). These CBM48 containing proteins could be a way of the isoamylases to overcome the lack of a functional SBD.
Chlamydomonas reinhardtii Isoamylase
The green alga Chlamydomonas reinhardtii is a model for studying starch synthesis, and two C. reinhardtii isoamylases have been shown to be important for the starch synthesis (Mouille, 1996; Dauvillee et al., 2001). C. reinhardtii isoamylase 1 consists of the GH13 catalytic domain, a CBM48, and a characteristic GH13 C-terminal domain. The structure of the GH13 maltoheptaose-complexed C. reinhardtii isoamylase 1 showed carbohydrates occupying an SBS situated at the reducing-end binding area of the active site as well as a second SBS at the interface of the catalytic and C-terminal domains (Figure 5). However, the possible function of these SBSs has not been investigated (Sim et al., 2014).
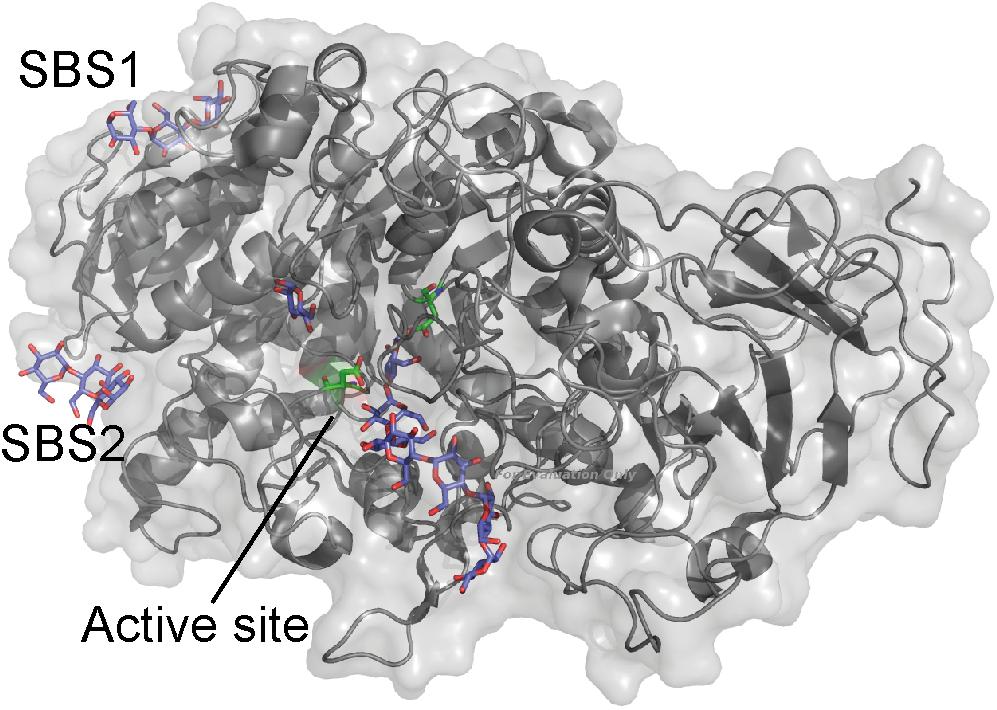
FIGURE 5. C. reinhardtii isoamylase A (gray) in complex with maltopentaose (purple) (PDB entry 4OKD). The active site residues are shown as sticks (green).
α-Glucan, Water Dikinases and α-Glucan Phosphatases
Starch phosphorylation can stimulate and hence perhaps be essential for starch biosynthesis (Hejazi et al., 2014; Skeffington et al., 2014). However, much remains to be learned about the exact metabolic role of starch phosphorylation (Mahlow et al., 2016; Xu et al., 2017). Two enzyme classes, α-glucan, water dikinases (EC 2.7.9.4) and α-glucan phosphatases (EC 3.1.3.48) are responsible for starch phosphorylation and dephosphorylation, respectively (Zeeman et al., 2010). The α-glucan, water dikinase 1 (GWD1) and GWD3 phosphorylate starch at the C6 and C3 position, respectively, and the two phosphatases, Starch Excess 4 (SEX4) and Like SEX4 2 (LSF2) dephosphorylates starch at the C6 and C3 position, respectively (Hejazi et al., 2010; Santelia et al., 2011).
GWD1
GWD1 from S. tuberosum (StGWD1) has a C-terminal catalytic domain and two CBM45s: CBM45-1 located at the N-terminal next to the chloroplast transit peptide and CBM45-2 at the center of the sequence, and large areas of the sequence were unmapped (Glaring et al., 2011). Despite the plethora of sequence data available since 2011, a new search against the Conserved Domains Database (Marchler-Bauer et al., 2017) did not reveal additional domains in the unmapped regions.
Each of the StGWD1 CBM45-1 and CBM45-2 was produced recombinantly, but only CBM45-2 was stable in solution and only at pH 8. SPR and ITC showed low affinity of CBM45-2 for the starch model β-CD (SPR: Kd = 0.38 mM; ITC: Kd = 0.68 mM). Such low affinity may be a prerequisite for a dynamic interaction of GWD1 with the starch granule facilitating a necessary tight control of the starch metabolism (Glaring et al., 2011). StGWD1 CBM45-1 was prepared by trypsin hydrolysis of the full-length enzyme and showed relatively weak affinity, yielding Kd of 7.2 mg ml-1 for starch granules in an adsorption assay and Kd of 1.2 mg ml-1 for soluble starch by using AGE (Mikkelsen et al., 2006). Mutations in the binding site of CBM45-1 of full-length StGWD1 resulted in complete loss of binding for these starch substrates demonstrating its pivotal role in enzyme function (Mikkelsen et al., 2006).
GWD3
GWD3 from A. thaliana (AtGWD3) has a single CBM20 appended at the N-terminus and a C-terminal GWD catalytic domain, while the function of the ∼700 amino acid residues long segment in between these two domains is unknown (Janeček et al., 2011). As for GWD1, search against the Conserved Domains Database (Marchler-Bauer et al., 2017) failed to identify any additional domains. Most CBM20s have two binding sites (Janeček et al., 2011), but presumably only binding site 1 is functional in AtGWD3 CBM20, which lacks residues typically for binding site 2 (Christiansen et al., 2009a).
Compared to CBM20s from amylolytic enzymes having Kd for β-CD in the μM-range recombinant AtGWD3 CBM20 showed unusually low affinity for α-, β-, and γ-CDs (Kd of 0.22–0.84 mM) as determined by SPR (Christiansen et al., 2009a). As for CBM45-2 of StGWD1, this may allow dynamic binding of AtGWD3 CBM20 facilitating GWD3 regulation (Christiansen et al., 2009b). The binding of α-, β-, and γ-CD by AtGWD3 CBM20 depended on pH – e.g., Kd for γ-CD was 0.84 and 5.56 mM at pH 5.5 and pH 9, respectively. However, for β-CD the observed affinity increased from Kd of 1.09 mM at pH 6 to 0.53 mM at pH 9, and this pH dependence was suggested to be related to the plants physiological needs as the binding would be stronger during the day when stromal pH increases (Christiansen et al., 2009a).
Starch Excess 4
The crystal structure of A. thaliana SEX4 contains a single maltoheptaose molecule that spans the extended binding pocket at the interface of the catalytic dual-specificity phosphatase (DSP) and CBM48 domains (Figure 6A; Meekins et al., 2014). Kd of A. thaliana SEX4 for amylose and amylopectin was determined by AGE to be 5.42 and 0.03 mg ml-1, respectively, in agreement with the preference of SEX4 for double helical structures in amylopectin. SPR analysis gave Kd for β-CD of 1.69 mM and a stoichiometry of 2.89, indicating three β-CD binding sites on SEX4, although this remains to be confirmed by structural analysis (Wilkens et al., 2016). Mutation of residues responsible for binding in both domains resulted in 10–80% loss of activity toward para-nitrophenyl phosphate (Meekins et al., 2014). Furthermore, the CBM48 mutant lost 95% and a DSP mutant 76% of the amylopectin binding affinity showing that the CBM48 plays a critical role in the function of SEX4 (Wilkens et al., 2016).
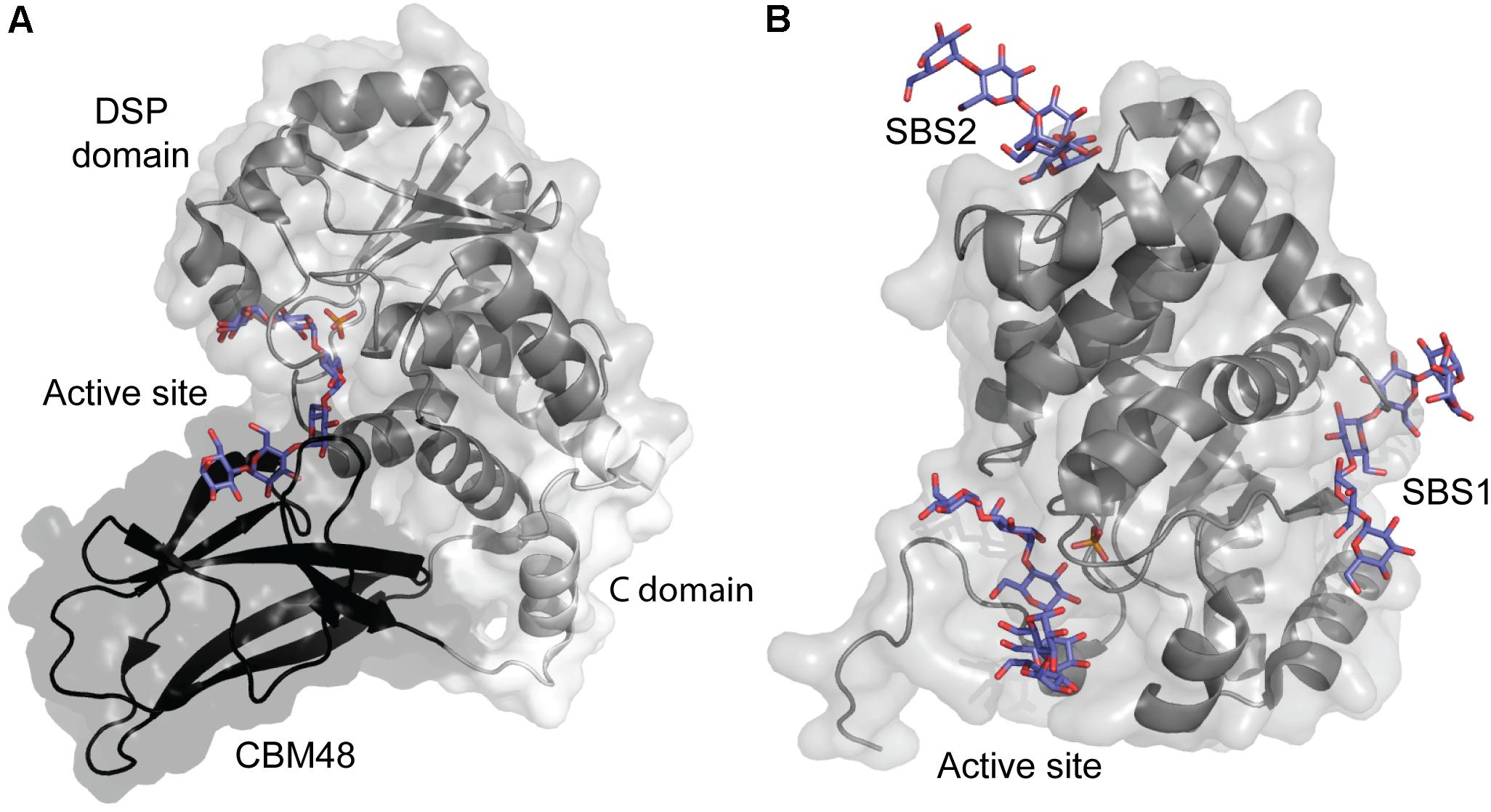
FIGURE 6. (A) A. thaliana Starch Excess 4 in complex with maltoheptaose (purple) and phosphate in the active site (orange) (PDB entry 4PYH). (B) A. thaliana Like SEX4 2 (gray) in complex with maltohexaose (purple) and phosphate in the active site (orange) (PDB entry 4KYR).
Like SEX4 2
Three distinct maltohexaose binding sites are seen in a crystal structure of A. thaliana Like SEX4 2 (LSF2) (Figure 6B). Of these, two SBSs, SBS1 and SBS2, are situated > 20 Å away from the active site which accommodates the third maltohexose molecule (Meekins et al., 2013). Using AGE A. thaliana LSF2 was shown to have Kd of 0.68 and 1.59 mg ml-1 for amylose and amylopectin, respectively, emphasizing its preference for the single chain helical conformation in amylose (Wilkens et al., 2016). LSF2 specifically hydrolyses C3 phosphate ester groups in starch (Santelia et al., 2011), which are hypothesized to disrupt the double helical conformation in amylopectin (Blennow and Engelsen, 2010). The higher affinity of LSF2 for amylose thus mimics its natural substrate preference (Wilkens et al., 2016). Unexpectedly, despite the long distance to the active site, SBS1 and SBS2 mutations resulted in 50 and 88% loss of activity for para-nitrophenyl phosphate, respectively (Meekins et al., 2013). Remarkably, however, molecular dynamics simulation showed that binding at the two SBSs affected the active site thus supporting the role of SBSs in activity. Mutation at all three binding sites reduced affinity for amylose below a measurable level. Still, comparison of the mobility of SBSs mutants in AGE pointed to their involvement in starch binding. As SBS2 seemed to have higher affinity for amylose than SBS1, it was suggested to be responsible for the initial starch recognition (Wilkens et al., 2016).
Conclusion and Looking Ahead
As the presence of either CBMs or SBSs in several cases has been crucial for correct starch biosynthesis, the recent focus on non-catalytic sites can potentially open new promising routes to obtain useful modified starches.
Current insights on CBM53s from SSIIIs and their interaction with starch may be useful for manipulating catalytic efficiency of SSIII by altering the active site structure without modifying the catalytic module. Determination of the structure of a CBM53 would take the knowledge to the next level and improve understanding of the protein–carbohydrate interactions, maybe to deduce a mechanism for how the different CBM53s can alter activity of SSIII (Wayllace et al., 2010).
SBSs in BE1s appear to define the length of transferred branch chains (Blennow et al., 1998; Feng et al., 2015; Hayashi et al., 2017), which could potentially constitute a target for rational protein engineering. Recently, the non-catalytic proteins PTST2 and FLO6 gained attention as targets for obtaining modified starches. The PTST gene has been demonstrated to be essential for amylose synthesis in A. thaliana leaves and could be useful in obtaining amylose-free starches, which find extensive applications in food and non-food industrial products (Santelia and Zeeman, 2011; Seung et al., 2015). CBM48 of PTST2 (FLO6) was suggested as a potential target for biotechnological modification of starch, particularly for modifying granule size. A ptst2 A. thaliana mutant had larger starch granules, but with similar morphology to wild type granules, while much smaller granules were observed in the ptst2 overexpression lines (Seung et al., 2017). Furthermore, a knock-out of FLO6 in rice was shown to affect starch synthesis (Peng et al., 2014).
Notably, these various examples evidently demonstrate that properties of starch can be modified by altering the enzyme–substrate interaction during starch biosynthesis. Yet, the impact of especially SBSs on the function of the starch biosynthetic enzymes is still to be further explored. However, the challenge is that they are not easily recognized based on bioinformatics analysis, like the carbohydrate binding sites of CBMs.
Author Contributions
MM, CW, and BS conceptualized the manuscript, which was written by CW and MM with invaluable input from BS.
Funding
This work was supported by Independent Research Fund Denmark | Natural Sciences (FNU) by grants to the projects ‘Discovery and Characterization of Carbohydrate SBS in Polysaccharide Converting Enzymes’ (Grant no. 09-027151) and ‘Roles and Structural Determinants of Low-affinity Carbohydrate-Protein Interactions’ (Grant No. 6108-00476B).
Conflict of Interest Statement
The authors declare that the research was conducted in the absence of any commercial or financial relationships that could be construed as a potential conflict of interest.
Acknowledgments
Andreas Blennow was thanked for discussions on α-glucan, water dikinases, and α-glucan phosphatases in starch biosynthesis.
References
Barchiesi, J., Hedin, N., Gomez-Casati, D. F., Ballicora, M. A., and Busi, M. V. (2015). Functional demonstrations of starch binding domains present in Ostreococcus tauri starch synthases isoforms. BMC Res. Notes 8:613. doi: 10.1186/s13104-015-1598-6
Beatty, M. K., Rahman, A., Cao, H., Woodman, W., Lee, M., Myers, A. M., et al. (1999). Purification and molecular genetic characterization of ZPU1, a pullulanase-type starch-debranching enzyme from maize. Plant Physiol. 119, 255–266. doi: 10.1104/pp.119.1.255
Binderup, K., Mikkelsen, R., and Preiss, J. (2000). Limited proteolysis of branching enzyme from Escherichia coli. Arch. Biochem. Biophys. 377, 366–371. doi: 10.1006/abbi.2000.1815
Blennow, A., and Engelsen, S. B. (2010). Helix-breaking news: fighting crystalline starch energy deposits in the cell. Trends Plant Sci. 15, 236–240. doi: 10.1016/j.tplants.2010.01.009
Blennow, A., Viksø-Nielsen, A., and Morell, M. K. (1998). α-glucan binding of potato-tuber starch-branching enzyme I as determined by tryptophan fluorescence quenching, affinity electrophoresis and steady-state kinetics. Eur. J. Biochem. 252, 331–338. doi: 10.1046/j.1432-1327.1998.2520331.x
Boraston, A. B., Bolam, D. N., Gilbert, H. J., and Davies, G. J. (2004). Carbohydrate-binding modules: fine-tuning polysaccharide recognition. Biochem. J. 382, 769–781. doi: 10.1042/BJ20040892
Burton, R. A., Zhang, X. Q., Hrmova, M., and Fincher, G. B. (1999). A single limit dextrinase gene is expressed both in the developing endosperm and in germinated grains of barley. Plant Physiol. 119, 859–871. doi: 10.1104/pp.119.3.859
Chaen, K., Noguchi, J., Omori, T., Kakuta, Y., and Kimura, M. (2012). Crystal structure of the rice branching enzyme I (BEI) in complex with maltopentaose. Biochem. Biophys. Res. Commun. 424, 508–511. doi: 10.1016/j.bbrc.2012.06.145
Christiansen, C., Abou Hachem, M., Glaring, M. A., Viksø-Nielsen, A., Sigurskjold, B. W., Svensson, B., et al. (2009a). A CBM20 low-affinity starch-binding domain from glucan, water dikinase. FEBS Lett. 583, 1159–1163. doi: 10.1016/j.febslet.2009.02.045
Christiansen, C., Abou Hachem, M., Janecek, S., Viksø-Nielsen, A., Blennow, A., and Svensson, B. (2009b). The carbohydrate-binding module family 20 - diversity, structure, and function. FEBS J. 276, 5006–5029. doi: 10.1111/j.1742-4658.2009.07221.x
Cockburn, D., and Svensson, B. (2013). “Surface binding sites in carbohydrate active enzymes: an emerging picture of structural and functional diversity,” in Carbohydrate Chemistry, Vol. 39, eds P. Rauter and T. Lindhorst (Cambrigde: The Royal Society of Chemistry), 204–221. doi: 10.1039/9781849737173-00204
Cockburn, D., Wilkens, C., Ruzanski, C., Andersen, S., Willum Nielsen, J., Smith, A. M., et al. (2014). Analysis of surface binding sites (SBSs) in carbohydrate active enzymes with focus on glycoside hydrolase families 13 and 77 – a mini-review. Biologia 69, 705–712. doi: 10.2478/s11756-014-0373-9
Commuri, P. D., and Keeling, P. L. (2001). Chain-length specificities of maize starch synthase I enzyme: studies of glucan affinity and catalytic properties. Plant J. 25, 475–486. doi: 10.1046/j.1365-313x.2001.00955.x
Crumpton-Taylor, M., Pike, M., Lu, K. J., Hylton, C. M., Feil, R., Eicke, S., et al. (2013). Starch synthase 4 is essential for coordination of starch granule formation with chloroplast division during Arabidopsis leaf expansion. New Phytol. 200, 1064–1075. doi: 10.1111/nph.12455
Cuesta-Seijo, J. A., Nielsen, M. M., Marri, L., Tanaka, H., Beeren, S. R., and Palcic, M. M. (2013). Structure of starch synthase I from barley: insight into regulatory mechanisms of starch synthase activity. Acta Crystallogr. Sect. D Biol. Crystallogr. 69, 1013–1025. doi: 10.1107/S090744491300440X
Cuesta-Seijo, J. A., Nielsen, M. M., Ruzanski, C., Krucewicz, K., Beeren, S. R., Rydhal, M. G., et al. (2016). In vitro biochemical characterization of all barley endosperm starch synthases. Front. Plant Sci. 6:1265. doi: 10.3389/fpls.2015.01265
Cuyvers, S., Dornez, E., Delcour, J. A., and Courtin, C. M. (2011). Occurrence and functional significance of secondary carbohydrate binding sites in glycoside hydrolases. Crit. Rev. Biotechnol. 31, 93–107. doi: 10.3109/07388551.2011.561537
Dauvillee, D., Colleoni, C., Mouille, G., Morell, M. K., D’Hulst, C., Wattebled, F., et al. (2001). Biochemical characterization of wild-type and mutant isoamylases of Chlamydomonas reinhardtii supports a function of the multimeric enzyme organization in amylopectin maturation. Plant Physiol. 125, 1723–1731. doi: 10.1104/pp.125.4.1723
Delvallé, D., Dumez, S., Wattebled, F., Roldán, I., Planchot, V., Berbezy, P., et al. (2005). Soluble starch synthase I: a major determinant for the synthesis of amylopectin in Arabidopsis thaliana leaves. Plant J. 43, 398–412. doi: 10.1111/j.1365-313X.2005.02462.x
Feng, L., Fawaz, R., Hovde, S., Gilbert, L., Chiou, J., and Geiger, J. H. (2015). Crystal structures of Escherichia coli branching enzyme in complex with linear oligosaccharides. Biochemistry 54, 6207–6218. doi: 10.1021/acs.biochem.5b00228
Feng, L., Fawaz, R., Hovde, S., Sheng, F., Nosrati, M., and Geiger, J. H. (2016). Crystal structures of Escherichia coli branching enzyme in complex with cyclodextrins. Acta Crystallogr. Sect. D Struct. Biol. 72, 641–647. doi: 10.1107/S2059798316003272
Gilding, E. K., Frčre, C. H., Cruickshank, A., Rada, A. K., Prentis, P. J., Mudge, A. M., et al. (2013). Allelic variation at a single gene increases food value in a drought-tolerant staple cereal. Nat. Commun. 4:1483. doi: 10.1038/ncomms2450
Glaring, M. A., Baumann, M. J., Abou Hachem, M., Nakai, H., Nakai, N., Santelia, D., et al. (2011). Starch-binding domains in the CBM45 family – low-affinity domains from glucan, water dikinase and α-amylase involved in plastidial starch metabolism. FEBS J. 278, 1175–1185. doi: 10.1111/j.1742-4658.2011.08043.x
Goren, A., Ashlock, D., and Tetlow, I. J. (2018). Starch formation inside plastids of higher plants. Protoplasma 255, 1855–1876. doi: 10.1007/s00709-018-1259-4
Gourlay, L. J., Santi, I., Pezzicoli, A., Grandi, G., Soriani, M., and Bolognesi, M. (2009). Group B streptococcus pullulanase crystal structures in the context of a novel strategy for vaccine development. J. Bacteriol. 191, 3544–3552. doi: 10.1128/JB.01755-08
Grisolia, M. J., Peralta, D. A., Valdez, H. A., Barchiesi, J., Gomez-Casati, D. F., and Busi, M. V. (2017). The targeting of starch binding domains from starch synthase III to the cell wall alters cell wall composition and properties. Plant Mol. Biol. 93, 121–135. doi: 10.1007/s11103-016-0551-y
Hayashi, M., Suzuki, R., Colleoni, C., Ball, S. G., Fujita, N., and Suzuki, E. (2017). Bound substrate in the structure of cyanobacterial branching enzyme supports a new mechanistic model. J. Biol. Chem. 292, 5465–5475. doi: 10.1074/jbc.M116.755629
Hejazi, M., Fettke, J., Kötting, O., Zeeman, S. C., and Steup, M. (2010). The Laforin-like dual-specificity phosphatase SEX4 from Arabidopsis hydrolyzes both C6- and C3-phosphate esters introduced by starch-related dikinases and thereby affects phase transition of alpha-glucans. Plant Physiol. 152, 711–722. doi: 10.1104/pp.109.149914
Hejazi, M., Mahlow, S., and Fettke, J. (2014). The glucan phosphorylation mediated by α-glucan, water dikinase (GWD) is also essential in the light phase for a functional transitory starch turn-over. Plant Signal. Behav. 9, 10–13. doi: 10.4161/psb.28892
Janeček,Š, Svensson, B., and MacGregor, E. A. (2011). Structural and evolutionary aspects of two families of non-catalytic domains present in starch and glycogen binding proteins from microbes, plants and animals. Enzyme Microb. Technol. 49, 429–440. doi: 10.1016/j.enzmictec.2011.07.002
Janeček,Š, Svensson, B., and MacGregor, E. A. (2014). α-Amylase: an enzyme specificity found in various families of glycoside hydrolases. Cell. Mol. Life Sci. 71, 1149–1170. doi: 10.1007/s00018-013-1388-z
Jeon, J.-S., Ryoo, N., Hahn, T.-R., Walia, H., and Nakamura, Y. (2010). Starch biosynthesis in cereal endosperm. Plant Physiol. Biochem. 48, 383–392. doi: 10.1016/j.plaphy.2010.03.006
Lammerts van Bueren, A., Ficko-Blean, E., Pluvinage, B., Hehemann, J.-H., Higgins, M. A., Deng, L., et al. (2011). The conformation and function of a multimodular glycogen-degrading pneumococcal virulence factor. Structure 19, 640–651. doi: 10.1016/j.str.2011.03.001
Li, C., Powell, P. O., and Gilbert, R. G. (2017). Recent progress toward understanding the role of starch biosynthetic enzymes in the cereal endosperm. Amylase 1, 59–74. doi: 10.1515/amylase-2017-0006
Liu, F., Romanova, N., Lee, E. A., Ahmed, R., Evans, M., Gilbert, E. P., et al. (2012). Glucan affinity of starch synthase IIa determines binding of starch synthase I and starch-branching enzyme IIb to starch granules. Biochem. J. 448, 373–387. doi: 10.1042/BJ20120573
Lohmeier-Vogel, E. M., Kerk, D., Nimick, M., Wrobel, S., Vickerman, L., Muench, D. G., et al. (2008). Arabidopsis At5g39790 encodes a chloroplast-localized, carbohydrate- binding, coiled-coil domain-containing putative scaffold protein. BMC Plant Biol. 8:120. doi: 10.1186/1471-2229-8-120
Lombard, V., Golaconda Ramulu, H., Drula, E., Coutinho, P. M., and Henrissat, B. (2014). The carbohydrate-active enzymes database (CAZy) in 2013. Nucleic Acids Res. 42, D490–D495. doi: 10.1093/nar/gkt1178
Mahlow, S., Orzechowski, S., and Fettke, J. (2016). Starch phosphorylation: insights and perspectives. Cell. Mol. Life Sci. 73, 2753–2764. doi: 10.1007/s00018-016-2248-4
Malle, D., Itoh, T., Hashimoto, W., Murata, K., Utsumi, S., and Mikami, B. (2006). Overexpression, purification and preliminary X-ray analysis of pullulanase from Bacillus subtilis strain 168. Acta Crystallogr. Sect. F Struct. Biol. Cryst. Commun. 62, 381–384. doi: 10.1107/S1744309106007901
Marchler-Bauer, A., Bo, Y., Han, L., He, J., Lanczycki, C. J., Lu, S., et al. (2017). CDD/SPARCLE: Functional classification of proteins via subfamily domain architectures. Nucleic Acids Res. 45, D200–D203. doi: 10.1093/nar/gkw1129
Meekins, D. A., Guo, H.-F., Husodo, S., Paasch, B. C., Bridges, T. M., Santelia, D., et al. (2013). Structure of the Arabidopsis glucan phosphatase LIKE SEX FOUR2 reveals a unique mechanism for starch dephosphorylation. Plant Cell 25, 2302–3214. doi: 10.1105/tpc.113.112706
Meekins, D. A., Raththagala, M., Husodo, S., White, C. J., Guo, H.-F., Kötting, O., et al. (2014). Phosphoglucan-bound structure of starch phosphatase Starch Excess4 reveals the mechanism for C6 specificity. Proc. Natl. Acad. Sci. U.S.A. 111, 7272–7277. doi: 10.1073/pnas.1400757111
Mikami, B., Iwamoto, H., Malle, D., Yoon, H.-J., Demirkan-Sarikaya, E., Mezaki, Y., et al. (2006). Crystal structure of pullulanase: evidence for parallel binding of oligosaccharides in the active site. J. Mol. Biol. 359, 690–707. doi: 10.1016/j.jmb.2006.03.058
Mikkelsen, R., Suszkiewicz, K., and Blennow, A. (2006). A novel type carbohydrate-binding module identified in α-glucan, water dikinases is specific for regulated plastidial starch metabolism. Biochemistry 45, 4674–4682. doi: 10.1021/bi051712a
Møller, M. S., Henriksen, A., and Svensson, B. (2016). Structure and function of α-glucan debranching enzymes. Cell. Mol. Life Sci. 73, 2619–2641. doi: 10.1007/s00018-016-2241-y
Møller, M. S., Windahl, M. S., Sim, L., Bøjstrup, M., Abou Hachem, M., Hindsgaul, O., et al. (2015) Oligosaccharide and substrate binding in the starch debranching enzyme barley limit dextrinase. J. Mol. Biol. 427, 1263–1277. doi: 10.1016/j.jmb.2014.12.019
Mouille, G. (1996). Preamylopectin Processing: a Mandatory Step for starch biosynthesis in plants. Plant Cell 8, 1353–1366. doi: 10.1105/tpc.8.8.1353
Myers, A. M., Morell, M. K., James, M. G., and Ball, S. G. (2000). Update on biochemistry recent progress toward understanding biosynthesis of the amylopectin crystal. Plant Physiol. 122, 989–997. doi: 10.1104/pp.122.4.989
Nakamura, Y. (1996). Some properties of starch debranching enzymes and their possible role in amylopectin biosynthesis. Plant Sci. 121, 1–18. doi: 10.1016/S0168-9452(96)04504-9
Nakamura, Y. (2002). Towards a better understanding of the metabolic system for amylopectin biosynthesis in plants: rice endosperm as a model tissue. Plant Cell Physiol. 43, 718–725. doi: 10.1093/pcp/pcf091
Nielsen, M. M., Ruzanski, C., Krucewicz, K., Striebeck, A., Cenci, U., Ball, S. G., et al. (2018). Crystal structures of the catalytic domain of Arabidopsis thaliana starch synthase IV, of granule bound starch synthase from CLg1 and of granule bound starch synthase I of Cyanophora paradoxa illustrate substrate recognition in starch synthases. Front. Plant Sci. 9:1138. doi: 10.3389/fpls.2018.01138
Peng, C., Wang, Y., Liu, F., Ren, Y., Zhou, K., Lv, J., et al. (2014). FLOURY ENDOSPERM6 encodes a CBM48 domain-containing protein involved in compound granule formation and starch synthesis in rice endosperm. Plant J. 77, 917–930. doi: 10.1111/tpj.12444
Pfister, B., and Zeeman, S. C. (2016). Formation of starch in plant cells. Cell. Mol. Life Sci. 73, 2781–2807. doi: 10.1007/s00018-016-2250-x
Roldán, I., Wattebled, F., Mercedes Lucas, M., Delvallé, D., Planchot, V., Jiménez, S., et al. (2007). The phenotype of soluble starch synthase IV defective mutants of Arabidopsis thaliana suggests a novel function of elongation enzymes in the control of starch granule formation. Plant J. 49, 492–504. doi: 10.1111/j.1365-313X.2006.02968.x
Saito, M., Tanaka, T., Sato, K., Vrinten, P., and Nakamura, T. (2018). A single nucleotide polymorphism in the “Fra” gene results in fractured starch granules in barley. Theor. Appl. Genet. 131, 353–364. doi: 10.1007/s00122-017-3006-1
Santelia, D., Kötting, O., Seung, D., Schubert, M., Thalmann, M., Bischof, S., et al. (2011). The phosphoglucan phosphatase Like Sex Four2 dephosphorylates starch at the C3-position in Arabidopsis. Plant Cell 23, 4096–4111. doi: 10.1105/tpc.111.092155
Santelia, D., and Zeeman, S. C. (2011). Progress in Arabidopsis starch research and potential biotechnological applications. Curr. Opin. Biotechnol. 22, 271–280. doi: 10.1016/j.copbio.2010.11.014
Senoura, T., Asao, A., Takashima, Y., Isono, N., Hamada, S., Ito, H., et al. (2007). Enzymatic characterization of starch synthase III from kidney bean (Phaseolus vulgaris L.). FEBS J. 274, 4550–4560. doi: 10.1111/j.1742-4658.2007.05984.x
Seung, D., Boudet, J., Monroe, J., Schreier, T. B., David, L. C., Abt, M., et al. (2017). Homologs of PROTEIN TARGETING TO STARCH control starch granule initiation in Arabidopsis leaves. Plant Cell 29, 1657–1677. doi: 10.1105/tpc.17.00222
Seung, D., Soyk, S., Coiro, M., Maier, B. A., Eicke, S., and Zeeman, S. C. (2015). Protein targeting to starch is required for localising granule-bound starch synthase to starch granules and for normal amylose synthesis in Arabidopsis. PLoS Biol. 13:e1002080. doi: 10.1371/journal.pbio.1002080
Sim, L., Beeren, S. R., Findinier, J., Dauvillée, D., Ball, S., Henriksen, A., et al. (2014). Crystal structure of the Chlamydomonas starch debranching enzyme isoamylase ISA1 reveals insights into the mechanism of branch trimming and complex assembly. J. Biol. Chem. 289, 22991–23003. doi: 10.1074/jbc.M114.565044
Skeffington, A. W., Graf, A., Duxbury, Z., Gruissem, W., and Smith, A. M. (2014). Glucan, water dikinase exerts little control over starch degradation in Arabidopsis leaves at night. Plant Physiol. 165, 866–879. doi: 10.1104/pp.114.237016
Stam, M. R., Danchin, E. G. J., Rancurel, C., Coutinho, P. M., and Henrissat, B. (2006). Dividing the large glycoside hydrolase family 13 into subfamilies: towards improved functional annotations of α-amylase-related proteins. Protein Eng. Des. Sel. 19, 555–562. doi: 10.1093/protein/gzl044
Suzuki, E., Onoda, M., Colleoni, C., Ball, S., Fujita, N., and Nakamura, Y. (2013). Physicochemical variation of cyanobacterial starch, the insoluble α-glucans in cyanobacteria. Plant Cell Physiol. 54, 465–473. doi: 10.1093/pcp/pcs190
Suzuki, E., and Suzuki, R. (2016). Distribution of glucan-branching enzymes among prokaryotes. Cell. Mol. Life Sci. 73, 2643–2660. doi: 10.1007/s00018-016-2243-9
Suzuki, R., Koide, K., Hayashi, M., Suzuki, T., Sawada, T., Ohdan, T., et al. (2015). Functional characterization of three (GH13) branching enzymes involved in cyanobacterial starch biosynthesis from Cyanobacterium sp. NBRC 102756. Biochim. Biophys. Acta Proteins Proteomics 1854, 476–484. doi: 10.1016/j.bbapap.2015.02.012
Szydlowski, N., Ragel, P., Raynaud, S., Lucas, M. M., Roldan, I., Montero, M., et al. (2009). Starch granule initiation in Arabidopsis requires the presence of either class IV or class III starch synthases. Plant Cell 21, 2443–2457. doi: 10.1105/tpc.109.066522
Tetlow, I., and Emes, M. (2017). Starch biosynthesis in the developing endosperms of grasses and cereals. Agronomy 7:81. doi: 10.3390/agronomy7040081
Tetlow, I. J., and Emes, M. J. (2014). A review of starch-branching enzymes and their role in amylopectin biosynthesis. IUBMB Life 66, 546–558. doi: 10.1002/iub.1297
Valdez, H. A., Busi, M. V., Wayllace, N. Z., Parisi, G., Ugalde, R. A., and Gomez-Casati, D. F. (2008). Role of the N-terminal starch-binding domains in the kinetic properties of starch synthase III from Arabidopsis thaliana. Biochemistry 47, 3026–3032. doi: 10.1021/bi702418h
Valdez, H. A., Peralta, D. A., Wayllace, N. Z., Grisolía, M. J., Gomez-Casati, D. F., and Busi, M. V. (2011). Preferential binding of SBD from Arabidopsis thaliana SSIII to polysaccharides: study of amino acid residues involved. Starch Staerke 63, 451–460. doi: 10.1002/star.201000111
Vamadevan, V., and Bertoft, E. (2015). Structure-function relationships of starch components. Starch Stärke 67, 55–68. doi: 10.1002/star.201400188
Vester-Christensen, M. B., Abou Hachem, M., Svensson, B., and Henriksen, A. (2010). Crystal structure of an essential enzyme in seed starch degradation: barley limit dextrinase in complex with cyclodextrins. J. Mol. Biol. 403, 739–750. doi: 10.1016/j.jmb.2010.09.031
Wayllace, N. Z., Valdez, H. A., Ugalde, R. A., Busi, M. V., and Gomez-Casati, D. F. (2010). The starch-binding capacity of the noncatalytic SBD2 region and the interaction between the N- and C-terminal domains are involved in the modulation of the activity of starch synthase III from Arabidopsis thaliana. FEBS J. 277, 428–440. doi: 10.1111/j.1742-4658.2009.07495.x
Wilkens, C., Auger, K. D., Anderson, N. T., Meekins, D. A., Raththagala, M., Abou Hachem, M., et al. (2016). Plant α-glucan phosphatases SEX4 and LSF2 display different affinity for amylopectin and amylose. FEBS Lett. 590, 118–128. doi: 10.1002/1873-3468.12027
Wilkens, C., Cuesta-Seijo, J. A., Palcic, M., and Svensson, B. (2014). Selectivity of the surface binding site (SBS) on barley starch synthase I. Biologia 69, 1118–1121. doi: 10.2478/s11756-014-0418-0
Xu, X., Dees, D., Dechesne, A., Huang, X. F., Visser, R. G. F., and Trindade, L. M. (2017). Starch phosphorylation plays an important role in starch biosynthesis. Carbohydr. Polym. 157, 1628–1637. doi: 10.1016/j.carbpol.2016.11.043
Zeeman, S. C., Kossmann, J., and Smith, A. M. (2010). Starch: its metabolism, evolution, and biotechnological modification in plants. Annu. Rev. Plant Biol. 61, 209–234. doi: 10.1146/annurev-arplant-042809-112301
Zhang, X., Myers, A. M., and James, M. G. (2005). Mutations affecting starch synthase III in Arabidopsis alter leaf starch structure and increase the rate of starch synthesis. Plant Physiol. 138, 663–674. doi: 10.1104/pp.105.060319
Keywords: carbohydrate binding module, surface binding site, starch synthesis, protein-carbohydrate interaction, starch binding domain, glycoside hydrolase, glycosyl transferase
Citation: Wilkens C, Svensson B and Møller MS (2018) Functional Roles of Starch Binding Domains and Surface Binding Sites in Enzymes Involved in Starch Biosynthesis. Front. Plant Sci. 9:1652. doi: 10.3389/fpls.2018.01652
Received: 26 June 2018; Accepted: 24 October 2018;
Published: 13 November 2018.
Edited by:
Andreas Blennow, University of Copenhagen, DenmarkReviewed by:
Yan Lu, Western Michigan University, United StatesAleksander Riise Hansen, University of Copenhagen, Denmark
Copyright © 2018 Wilkens, Svensson and Møller. This is an open-access article distributed under the terms of the Creative Commons Attribution License (CC BY). The use, distribution or reproduction in other forums is permitted, provided the original author(s) and the copyright owner(s) are credited and that the original publication in this journal is cited, in accordance with accepted academic practice. No use, distribution or reproduction is permitted which does not comply with these terms.
*Correspondence: Marie Sofie Møller, msm@bio.dtu.dk