- 1School of Life and Environmental Sciences, Plant Breeding Institute, Sydney Institute of Agriculture, The University of Sydney, Narrabri, NSW, Australia
- 2Agriculture Victoria, AgriBio, Centre for AgriBioscience, Melbourne, VIC, Australia
- 3School of Applied Systems Biology, La Trobe University, Melbourne, VIC, Australia
- 4School of Life and Environmental Sciences, Plant Breeding Institute, Sydney Institute of Agriculture, The University of Sydney, Cobbitty, NSW, Australia
Rising global temperatures cause substantial yield losses in many wheat growing environments. Emmer wheat (Triticum dicoccon Schrank), one of the first wheat species domesticated, carries significant variation for tolerance to abiotic stresses. This study identified new genetic variability for high-temperature tolerance in hexaploid progeny derived from crosses with emmer wheat. Eight hexaploid and 11 tetraploid parents were recombined in 43 backcross combinations using the hexaploid as the recurrent parent. A total of 537 emmer-based hexaploid lines were developed by producing approximately 10 doubled haploids on hexaploid like BC1F1 progeny and subsequent selection for hexaploid morphology. These materials and 17 commercial cultivars and hexaploid recurrent parents were evaluated under two times of sowing in the field, in 2014–2016. The materials were genotyped using a 90K SNP platform and these data were used to estimate the contribution of emmer wheat to the progeny. Significant phenotypic and genetic variation for key agronomical traits including grain yield, TKW and screenings was observed. Many of the emmer derived lines showed improved performance under heat stress (delayed sowing) compared with parents and commercial cultivars. Emmer derived lines were the highest yielding material in both sowing dates. The emmer wheat parent contributed between 1 and 44% of the genome of the derived lines. Emmer derived lines with superior kernel weight and yield generally had a greater genetic contribution from the emmer parent compared to those with lower trait values. The study showed that new genetic variation for key traits such as yield, kernel weight and screenings can be introduced to hexaploid wheat from emmer wheat. These genetic resources should be explored more systematically to stabilize grain yield and quality in a changing climate.
Introduction
Wheat (Triticum aestivum) is a major cereal crop and important for human nutrition worldwide (Fischer et al., 2014; Arzani and Ashraf, 2017). High temperature stress is common in most wheat growing regions of the world, affecting crop productivity, yield stability, and quality (Teixeira et al., 2013; Zhao et al., 2017). Wheat grain characteristics, number, size, and quality, are impacted by heat stress (Lobell et al., 2012; Branlard et al., 2015) and the identification of wheat genotypes with stable yield and quality across a range of environments are important breeding objectives (Dupont et al., 2006; Malik et al., 2013).
Heat tolerance is complex and is controlled by many genes (Richards et al., 2007; Rebetzke et al., 2008; Petrarulo et al., 2009). A number of agronomical, morphological and physiological traits have been associated with heat tolerance of wheat, which include pollen stability and grain set (Lopes and Reynolds, 2012; Mondal et al., 2013; Pinto et al., 2017; Thistlethwaite, 2017). High grain growth rates and larger grain weight have also been linked with improved performance under heat stress (Singha et al., 2006; Dias and Lidon, 2009; Zhang et al., 2014). For breeding programs to be effective, screening should be high-throughput and traits should be easy to measure and highly heritable (Edmeades et al., 2001). Various methods of field-based screening have been evaluated including different sowing dates to study yield and related traits (Mondal et al., 2013; Devasirvatham et al., 2016). Cooper and Podlich (1999) stated that breeding for a target environment is generally effective when selection is made under representative environmental conditions. They concluded that field-based screening should closely represent the most probable conditions experienced by farmers.
Plant breeding has led to genetic erosion due to uniformity requirements and the need to increase productivity (Bharadwaj, 2016). Gradual addition of new genetic variation is needed to sustain the crop improvement process and further enhancements in tolerance to environmental stresses (Dwivedi et al., 2016). The systematic evaluation of genetic resources can define biodiversity patterns, which will facilitate the characterization of allelic variation for yield in stressful environments (Ceccarelli, 2011; Ceccarelli et al., 2013; Bharadwaj, 2016; Dwivedi et al., 2016). However, the addition of diverse allelic variation to applied breeding is problematic (Rasmusson and Phillips, 1997). Thus, to meet short-term breeding objectives; elite germplasm that is pre-adapted to the target environment is used instead of exotic germplasm that requires extensive pre-breeding (Sharma et al., 2013).
For wheat, exotic genetic resources often exhibit better adaptation in stressful climatic conditions (Trethowan and Mujeeb-Kazi, 2008) and contain more diverse genes for stress tolerance (Reynolds et al., 2007; van Ginkel and Ogbonnaya, 2007). These resources include wheat ancestors that can be used to improve the sustainability of wheat production and quality (Nevo, 2014; Arzani and Ashraf, 2017). Emmer wheat (Triticum dicoccon Schrank), which is one of the earliest domesticated wheat species and is closely related to modern wheat (Troccoli and Codianni, 2005; Nevo, 2014), has exhibited tolerance to both drought and heat stress (Zaharieva et al., 2010; Nevo, 2014). Moreover, diversity for abiotic and biotic stresses in this tetraploid species can be transferred to commercial wheat cultivars (Xie and Nevo, 2008; Hassan et al., 2016). Introduction of new diversity from all three wheat genomes in emmer-based synthetic wheat produced promising results under stress compared to modern durum wheat synthetic derivatives (Dreisigacker et al., 2008; Trethowan and Mujeeb-Kazi, 2008), especially for drought and high-temperature tolerance (Zaharieva et al., 2010). However, the introduction of emmer-based genetic diversity for heat tolerance in hexaploid wheat has not been fully explored.
This study evaluated a large population of hexaploid wheat genotypes developed through recombination with diverse emmer wheat. These accessions included materials previously identified as tolerant to abiotic stresses (Zaharieva et al., 2010). Genetic diversity for high-temperature tolerance was explored by evaluating the material in the field under managed conditions. It was expected that allelic diversity from emmer wheat would contribute to improved high-temperature stress tolerance in hexaploid wheat.
Materials and Methods
Plant Material and Environment
Diverse emmer wheat (T. dicoccon Schrank), including accessions identified as stress tolerant by Zaharieva et al. (2010), were crossed and backcrossed with hexaploid bread wheat to transfer A and B genome genetic variation into modern hexaploid wheat. Eight hexaploid and 11 tetraploid parents were recombined in 43 backcross combinations using the hexaploid as the recurrent parent. The pentaploid (AABBD) F1 was backcrossed to the hexaploid parent and hexaploid progeny selected based on plant morphology. Doubled haploids were then produced from each hexaploid BC1F1 plant to produce an average of 10 homozygous lines per plant. A population of approximately 537 doubled haploid genotypes was developed (see Supplementary Table 1 for details) and subsequently evaluated for heat stress, along with their recurrent parents and commercial check cultivars. The parental materials and their pedigrees are reported in Table 1. The genotype Waxwing∗2/Kiritati and its progeny were not included in the DNA study as DNA of the parent was not available.
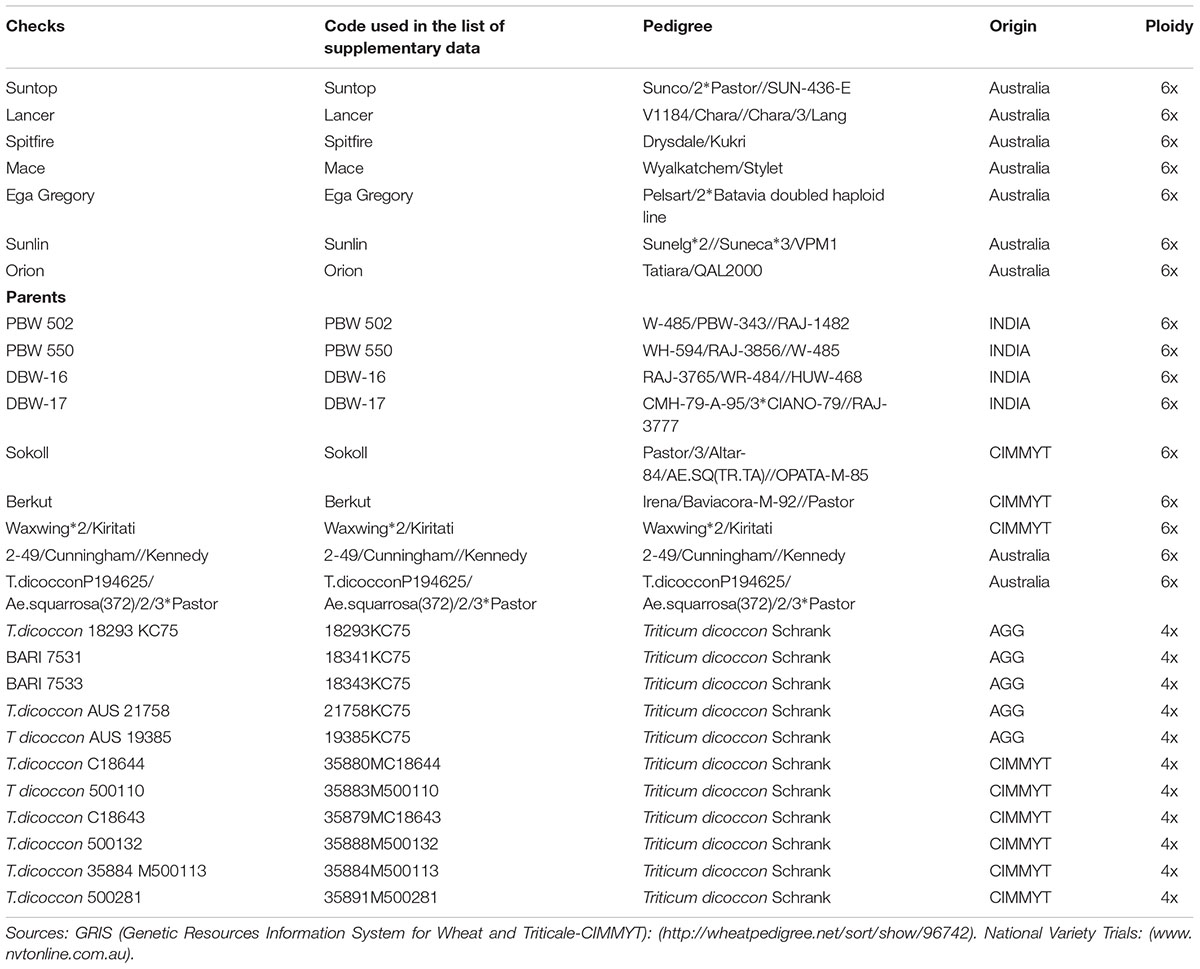
TABLE 1. List of commercial cultivars and parents used to develop emmer-based derivatives evaluated in field studies during 2014–2016.
Experimental Design
Field experiments were sown at the IA Watson Grains Research Centre, The University of Sydney, Narrabri, NSW (30° 20′S 149° 45′E) during the cropping seasons 2014–2016. Experiments were arranged in randomized complete block designs with two replications. Genotypes were sown in plots comprising six rows spaced 33 cm apart and 6 m length, which was reduced to 4 m for harvest. Two experiments were sown adjacent to each other, each year. Experiment 1 (E1, Environment 1) was sown at the optimal sowing date for the region in mid-May. Experiment 2 (E2, Environment 2) was sown 8 weeks after the optimal sowing date.
A set of 200 wheat genotypes from the population were sown in 2014 and a different set of 196 wheat genotypes were sown in 2015. The commercial check cultivars were the same in both years. All genotypes from 2014 to 2015 combined were evaluated in 2016, representing 543 genotypes, including commercial checks, recurrent parents and additional materials. Experiments received supplementary irrigation as required to reduce the confounding effects of drought; particularly in late sown conditions. The experiments were fertilized and managed according to standard industry practices for the region.
Germplasm Characterization
A common set of traits including days to flowering and physiological maturity, percentage of screenings, thousand kernel weight (TKW) and grain yield was assessed each year following Pask et al. (2012). The grain yield/plot was measured in g/plot and later converted to tons ha-1 for all further analyzes. For TKW, five hundred grains were counted using a CONTADOR seed counter (Pfeuffer GmbH, Flugplatzstraβe 70. D-97318 Kitizingen, Germany), then weighed (g) and the result multiplied by 2 to determine TKW. Care was taken to avoid broken grains. Percentage screenings were determined as the amount of split, small sized and shriveled grains in each plot sample (approximately 450–500 g) using an Agtator sieve shaker (Graintec Scientific, QLD, Australia) with 2.0 mm diameter sieves. Screenings were estimated after 40 shakes (standardized for wheat) using the formula; Screenings (%) = (weight of screenings)/(weight of sample) × 100.
Genetic Analysis
DNA of all genotypes was extracted following the CTAB method proposed by Doyle and Doyle (1990). Five leaves per plot (about 0.5–0.6 g) were collected from random plants of the middle rows of plots during tillering and placed in a 15 mL centrifuge tube containing silica gel to extract the moisture. Tubes containing leaf samples were then kept at room temperature for 7 days to dry the samples for DNA extraction. The material was then genotyped by AgriBio, La Trobe University, VIC, Australia using the Infinium iSelect SNP 90K SNP Assay (Cavanagh et al., 2013; Wang et al., 2014) following the protocol prescribed by the manufacturer. In total 35,267 polymorphic SNP markers were used for further analysis (see Supplementary Data).
Statistical Analysis
The best linear unbiased estimate (BLUE) of each wheat genotype was calculated using ASReml-R (Gilmour et al., 2009). The phenotypic data was combined across all 3 years within each sowing date and BLUEs calculated for each genotype in each environment. Genotypes and environments were considered fixed terms and ranges/rows within replicates within environments as random terms in the model. GGE bi-plots of the relationships between genotypes and environments were constructed on phenotypic data using the meta-analysis procedure from GenStat version 16 (Payne et al., 2011). GGE bi-plots were produced based on screenings, TKW and grain yield to estimate genotypic stability across both environments. Cluster analysis was performed using the multivariate analysis procedure from GenStat on 90K SNPs data to form similarity matrix and dendrograms were constructed on the basis of genetic distances.
Results
Temperature Fluctuations in the Field
Anthesis occurred during the first 2 weeks of September in E1, whereas anthesis was delayed by an average of 4 weeks in E2. The maximum temperature between anthesis and physiological maturity in 2014 was 35°C in E1 and 40.8°C in E2. The respective E1/E2 maximum temperatures in 2015 and 2016 were 35.4/35.6°C and 29.9/36.7°C. High temperature was more pronounced in the later stages of grain filling in all environments (Supplementary Figures 1–3).
Trait Expression and Stability
The GGE bi-plot analysis accounted for 100% of the total variation of all traits examined in both environments. There was a significant environmental effect and three typical examples of the responses of the recurrent parent (circled in red) and its derivatives for screenings, TKW and grain yield are presented in Figures 1–3. The responses of all remaining cross combinations are presented in Supplementary Figures 4–8. A dendrogram constructed using DNA diversity (part D in each figure) indicated that many genotypes were closely related to the recurrent parent. This is likely an artifact of the line development process as material was first selected visually for hexaploid type before under-going haploidization and subsequent chromosome doubling. This process skewed the population toward the more stable recurrent parent genotype. The impact of emmer introgression on each trait is presented below.
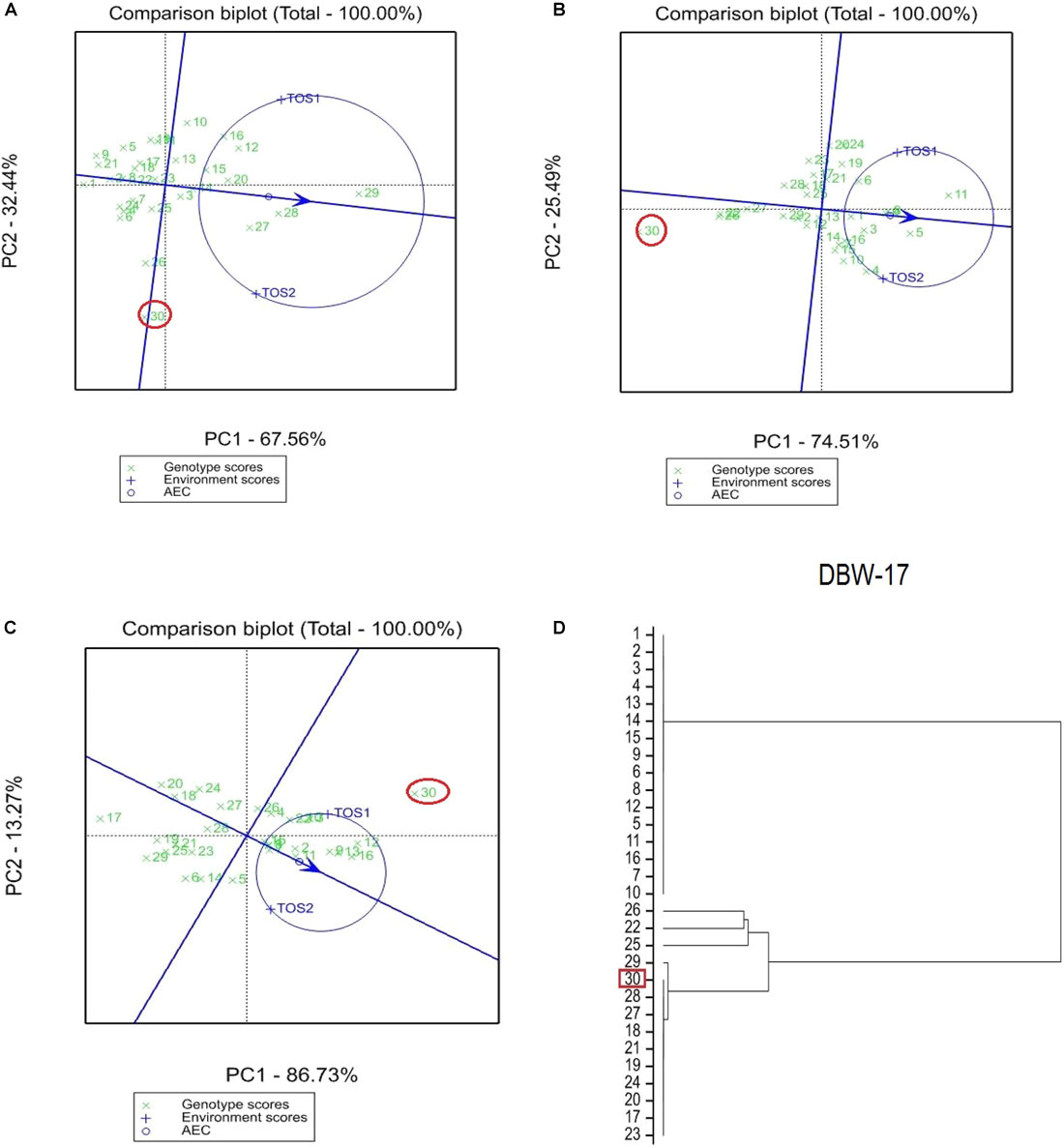
FIGURE 1. Comparison of recurrent parent DBW-17 (encircled red) and progenies based on mean performance and stability across the two environments (E1, optimal sowing; E2, delayed sowing – heat stressed) for (A) percentage screenings, (B) thousand kernel weight (TKW), and (C) grain yield. A dendrogram constructed using DNA diversity is given in part (D).
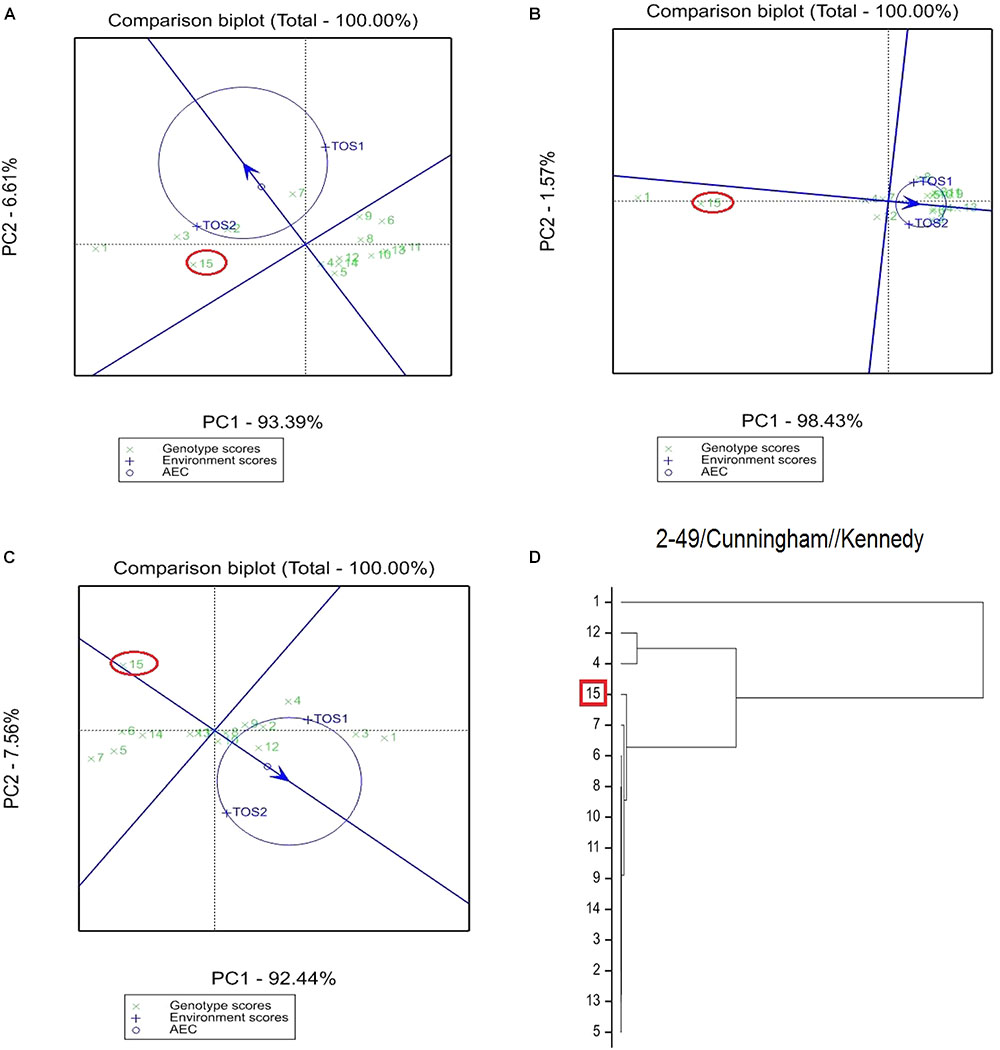
FIGURE 2. Comparison of recurrent parent 2-49/Cunningham//Kennedy (encircled red) and progenies based on mean performance and stability across the two environments (E1, optimal sowing; E2, delayed sowing – heat stressed) for (A) percentage screenings, (B) TKW, and (C) grain yield. A dendrogram constructed using DNA diversity is given in part (D).
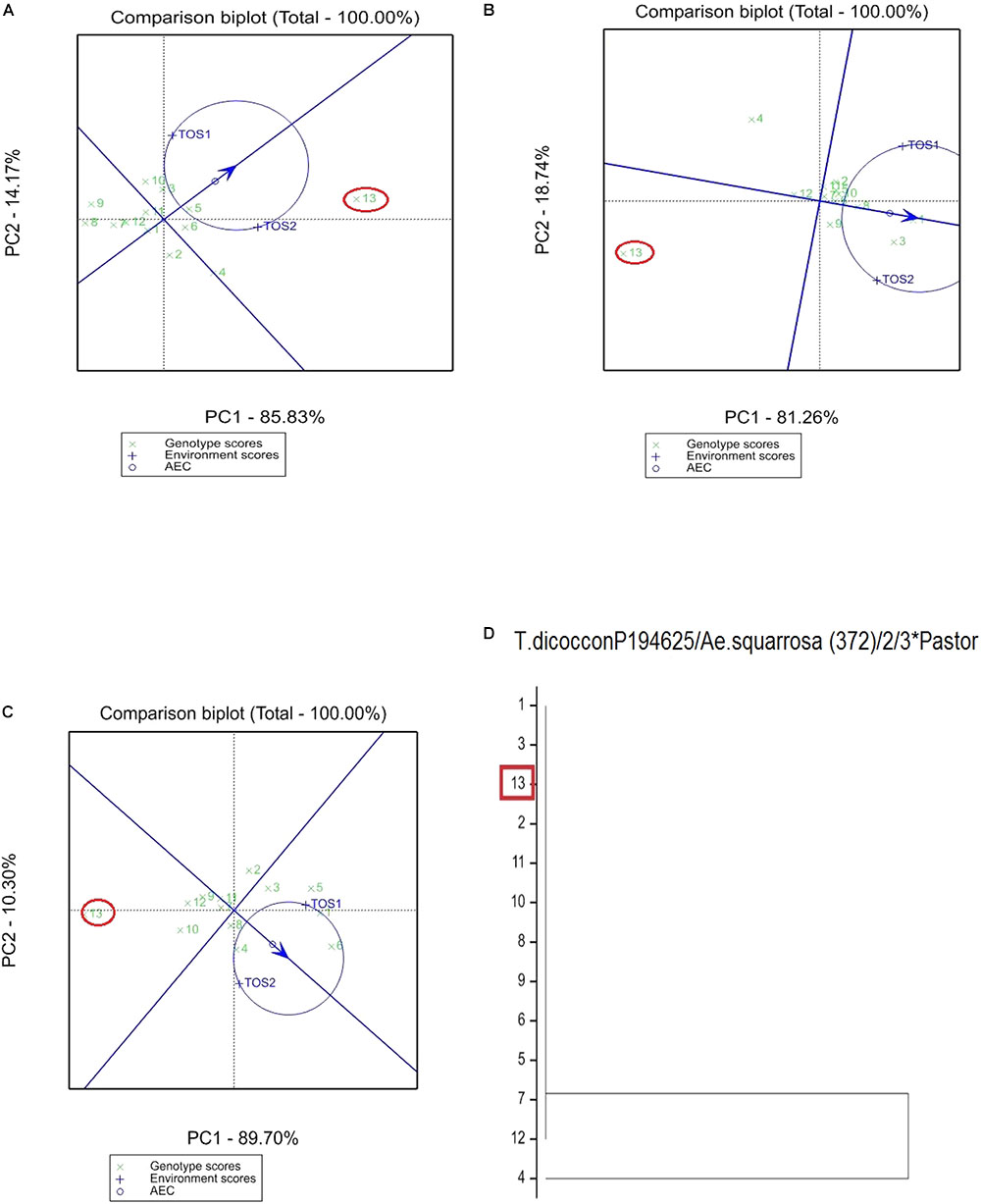
FIGURE 3. Comparison of recurrent parent T.dicocconP194625/Ae.squarrosa (372)/2/3∗Pastor (encircled red) and progenies based on mean performance and stability across the two environments (E1, optimal sowing; E2, delayed sowing – heat stressed) for (A) screenings, (B) TKW, and (C) grain yield. A dendrogram constructed using DNA diversity is given in part (D).
Screenings
The derived materials generally had higher screenings than the recurrent parent with the exception of the parents T.dicocconP194625/Ae.squarrosa (372)/2/3∗Pastor and 2-49/Cunningham//Kennedy (Figures 2A, 3A). Nevertheless, while the majority of progeny from other crosses had high screenings, there were some with lower screenings than the parents in crosses to PBW 502, DBW-16, and PBW 550 (Table 2). Interestingly, DBW-17 progeny had higher screenings in E1, but reduced screenings when sown late indicating possible tolerance to higher temperatures among some progeny (Figure 1A).
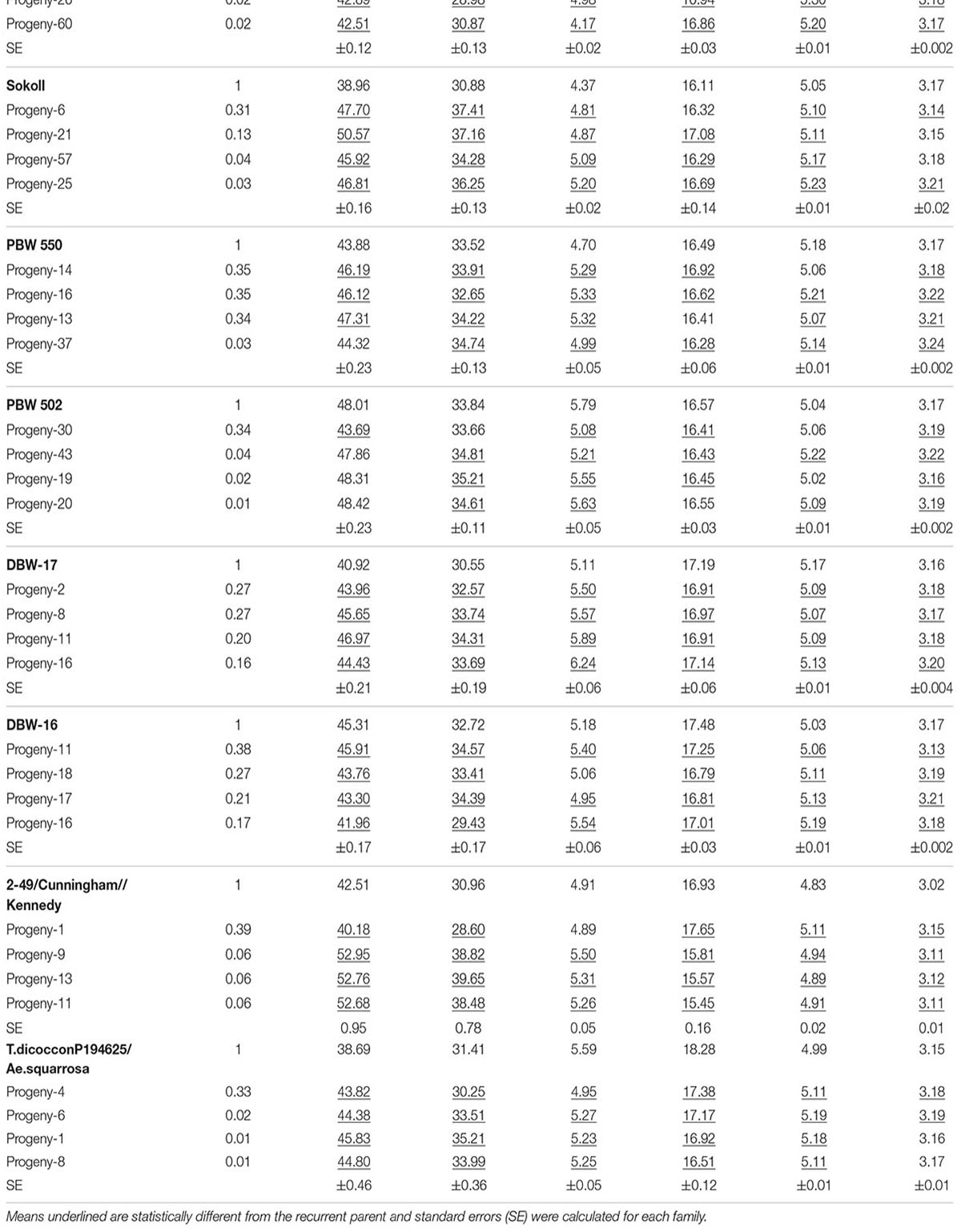
TABLE 2. Emmer derived progeny with superior phenotypic performance compared to their respective recurrent parents and the coefficient of parentage contributed by the emmer parent (COEP) determined by DNA analysis for the two environments (E1, optimal sowing; E2, delayed sowing – heat stressed).
Kernel Weight
The derived progeny significantly varied for TKW on the basis of recurrent parent. Crosses to DBW-17 (Figure 1A), 2-49/Cunningham//Kennedy (Figure 2B), T.dicocconP194625/Ae.squarrosa (372)/2/3∗Pastor (Figure 3B) and Sokoll produced many progeny with higher TKW than the recurrent parent (Table 2). Some crosses, such as those made to PBW 502, DBW-16, and PBW 550, produced progeny with generally similar TKW, although some with significantly lower TKW were observed. All progeny derived from Berkut had lower TKW than the recurrent parent. The recurrent parent 2-49/Cunningham//Kennedy derived line #9 and #13 had greater TKW and lower screening percentages under normal and stressed environments (Table 2).
Grain Yield
The grain yield of derived progeny varied significantly with the recurrent parent used. Higher yielding progeny were generally observed in E1 compared with E2. High yielding progeny compared to the recurrent hexaploid parent in E1 were observed for crosses to 2-49/Cunningham//Kennedy (Figure 2C), T.dicoc-conP194625/Ae.squarrosa (372)/2/3∗PASTOR (Figure 3C), PBW 502 and Sokoll. Some progeny were high yielding in both environments, including Sokoll and T.dicocconP194625/Ae.squarrosa (372)/2/3∗Pastor. Progeny higher yielding than the recurrent parent in E2 only were observed in crosses to DBW-16 and DBW-17 (Figure 1C and Table 2), indicating possible tolerance to higher temperatures. Berkut progeny #26 produced greater yield (5.29 t ha-1) under optimal sowing than recurrent hexaploid parent and check cultivars, whereas the PBW 550 derived line #37 produced greater yield (3.24 t ha-1) under heat stress.
Emmer Genetic Contribution and Trait Expression
The range of genetic variation contributed by emmer wheat, determined as the difference between individual progeny and the recurrent parent, varied from 0.01 to 0.44 (Table 2). The greatest emmer wheat contribution was observed for the Berkut progeny #45.
The emmer derived lines in the top and bottom group for each trait in each environment and the emmer parent genetic contribution are given in Table 3. Emmer derived lines in the top 10% based on yield under optimal sowing had an average 11% contribution from the emmer parent compared with only 6% contributed in the bottom 10% (Table 3). Similar trends were observed under heat stress (delayed sowing) where the top 10% of progeny had an emmer contribution of 14% compared with 5% in the bottom group. Grain weight in the top 10% of progeny in both optimal and delayed sowing conditions also had a higher emmer contribution (up to 11%) than the bottom group (up to 6%) (Table 3). However, screenings showed a different emmer contribution depending on sowing date. Under optimal sowing, progeny with lower screenings had a smaller contribution (4%) from the emmer parent compared with 10% in the bottom grouping. This was reversed in late sowing where those with lower screenings had a higher comparative emmer contribution (11 vs. 4%) in the bottom group.
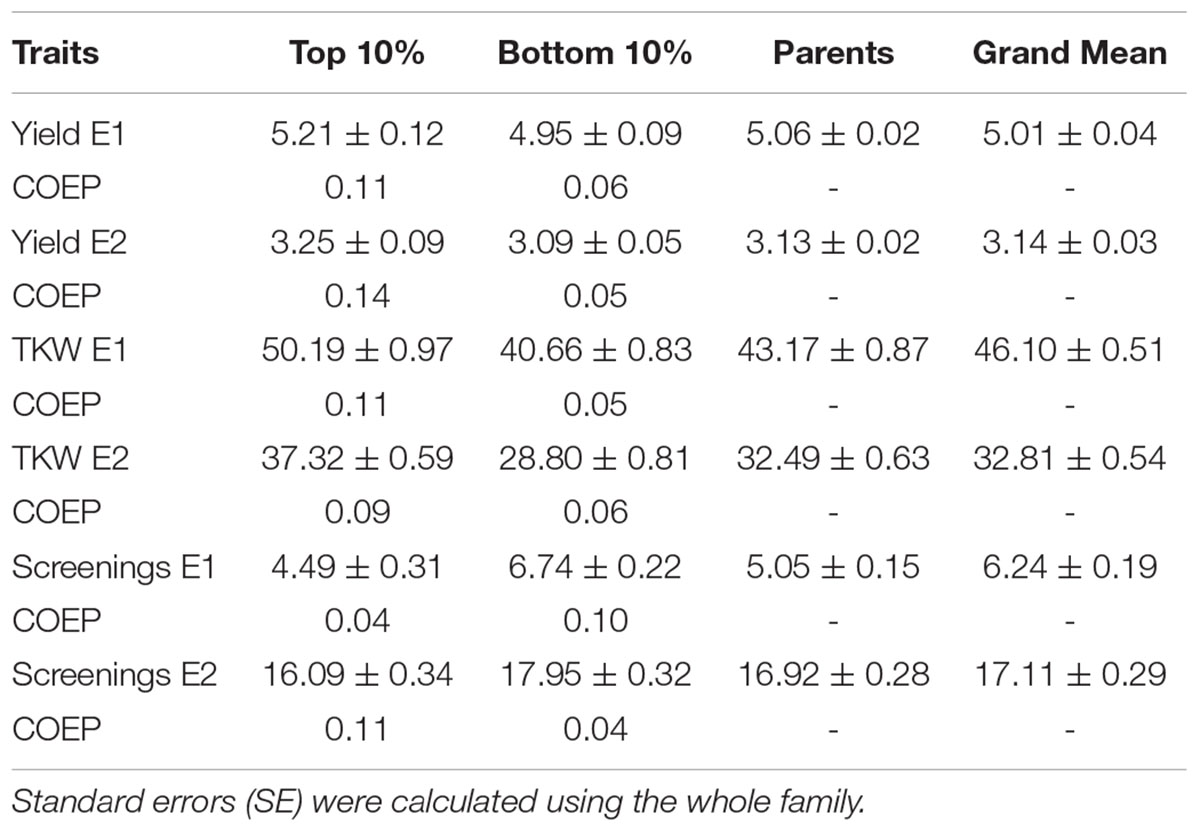
TABLE 3. Means ± SE of the highest and lowest performing progeny and their coefficient of parentage contributed by the emmer parent (COEP) determined by DNA analysis for the two environments (E1, optimal sowing; E2, delayed sowing – heat stressed) for yield, thousand kernel weight (TKW) and percentage screenings compared with the recurrent parents.
Discussion
This study showed that new genetic variation for key traits such as yield, kernel weight, and screenings can be introduced to hexaploid wheat from emmer wheat (Zaharieva et al., 2010; Chandrasekhar et al., 2017). This variation was expressed in both optimally sown and late sown materials, although trait expression under each condition did vary by genotype with some genotypes showing enhanced expression under both conditions. The hexaploid genotypes used as recurrent parents were selected because of their high yield potential and superior performance under late sowing (data not shown); the aim was to find different diversity to that already accumulated in high-yielding and heat tolerant hexaploid wheat genotypes. Interestingly, the theoretical expected 25% contribution of emmer wheat in the single backcross derived progeny was not observed. Instead, this varied between 1 and 44% and was a direct consequence of visual selection for hexaploid appearance and agronomic type, and the subsequent double haploidy process. This also helped to explain the large number of progenies closely related to the recurrent parent obtained from each cross combination. Nevertheless, progeny with <5% deviance from the recurrent parent based on their DNA profiles did produce significantly positive differences in trait values, particularly grain weight and grain yield. It appeared that the larger observed grain weight in the emmer progeny was offset by an increase in smaller grains or screenings as screenings were generally higher in all populations or derived families. It is likely that basal and distal florets failed to fill properly under increased temperature stress in the delayed sowing while those in the center of the spike produced much larger seed.
One of the aims of this research was to identify new sources of allelic variation for high-temperature tolerance as others have reported that useful variation exists in the wild tetraploid gene pool (Wang et al., 2005; Trethowan and Mujeeb-Kazi, 2008; Nevo, 2014). Later sowing increased post-anthesis heat stress, particularly in 2014 and 2015 and this reduced grain yield, lowered TKW and increased screenings. Similar responses to delayed sowing have been observed by others (Tewolde et al., 2006; Mondal et al., 2013). Given the large numbers of lines evaluated, sowing date treatments were not replicated within years; sowing dates were instead replicated across years, thus allowing large numbers of lines to be evaluated with replication in each environment each year. The observed reduction in grain yield under heat stress is most likely associated with reduced starch accumulation, which reduced grain size and weight (Farooq et al., 2011). However, as many of the progeny derived from a range of crosses maintained their grain weight and produced superior yield under heat stress, it can be concluded that useful variation was introduced from emmer wheat.
Thousand kernel weight was positively associated with yield and generally had a higher heritability than yield in earlier studies, making it an ideal target for indirect selection (Cossani and Reynolds, 2015; Pinto et al., 2017). The progeny of two recurrent parents, 2-49/Cunningham//Kennedy and T.dicocconP194625/Ae.squarrosa, had consistently higher kernel weight than their recurrent parents under heat stress. Unlike the progeny of other recurrent parents with equally higher kernel weight, they produced relatively low screenings, indicating an ability to fill all grains within the spike under stress. These materials express genuine high-temperature tolerance. Earlier studies, including that by Chandrasekhar et al. (2017), found that high TKW was linked to high-temperature tolerance; however, as screenings were not presented in that earlier work it is difficult to determine if this tolerance was equivalent.
Author Contributions
SU designed the experiments, collected the data, analyzed the results, and wrote this article. HB and TM as associated research supervisors facilitated for planning and conducting the research. HD and SH helped in genetic analysis. RJT assisted in phenotyping. RT as a primary research supervisor aided in material development, and strategy advice. All the authors read and approved the final manuscript.
Funding
This research was provided by the Grains Research and Development Corporation (US00057, US00059, US00080 and US00081) and Generation Challenge Program heat.
Conflict of Interest Statement
The authors declare that the research was conducted in the absence of any commercial or financial relationships that could be construed as a potential conflict of interest.
Acknowledgments
SU is grateful to The University of Sydney, International Postgraduate Research Scholarship (IPRS) Scheme and the Australian Government International Research Training Program for his scholarship.
Supplementary Material
The Supplementary Material for this article can be found online at: https://www.frontiersin.org/articles/10.3389/fpls.2018.01529/full#supplementary-material
References
Arzani, A., and Ashraf, M. (2017). Cultivated ancient wheats (Triticum spp.): a potential source of health-beneficial food products. Compr. Rev. Food Sci. Food Saf. 16, 477–488. doi: 10.1111/1541-4337.12262
Bharadwaj, D. N. (2016). “Sustainable agriculture and plant breeding,” in Advances in Plant Breeding Strategies: Agronomic, Abiotic and Biotic Stress Traits, eds J. M. Al-Khayri, S. Mohan Jain, and D. V. Johnson (Berlin: Springer), 3–34.
Branlard, G., Lesage, V. S., Bancel, E., Martre, P., Méleard, B., and Rhazi, L. (2015). “Coping with wheat quality in a changing environment: proteomics evidence for stress caused by environmental changes,” in Advances in Wheat Genetics: From Genome to Field, eds Y. Ogihara, S. Takumi, and H. Handa (Berlin: Springer), 255–264.
Cavanagh, C. R., Chao, S., Wang, S., Huang, B. E., Stephen, S., Kiani, S., et al. (2013). Genome-wide comparative diversity uncovers multiple targets of selection for improvement in hexaploid wheat landraces and cultivars. Proc. Natl. Acad. Sci. U.S.A. 110, 8057–8062. doi: 10.1073/pnas.1217133110
Ceccarelli, S. (2011). “Landraces: importance and use in breeding and environmentally friendly agronomic systems,” in Agrobiodiversity Conservation Securing the Diversity of Crop Wild Relatives and Landraces, ed. N. Maxted (Wallingford: CAB Interenational), 103.
Ceccarelli, S., Galie, A., and Grando, S. (2013). “Participatory breeding for climate change-related traits,” in Genomics and Breeding for Climate-Resilient Crops, ed. C. Kole (Berlin: Springer), 331–376.
Chandrasekhar, K., Nashef, K., and Ben-David, R. (2017). Agronomic and genetic characterization of wild emmer wheat (Triticum turgidum subsp. dicoccoides) introgression lines in a bread wheat genetic background. Genet. Resour. Crop Evol. 64, 1917–1926. doi: 10.1007/s10722-016-0481-1
Cooper, M., and Podlich, D. (1999). “Genotype × environment interactions, selection response and heterosis,” in Genetics and Exploitation of Heterosis in Crops, eds J. G. Coors and S. Pandey (Madison, WI: American Society of Agronomy), 81–92.
Cossani, C. M., and Reynolds, M. P. (2015). Heat stress adaptation in elite lines derived from synthetic hexaploid wheat. Crop Sci. 55, 2719–2735. doi: 10.2135/cropsci2015.02.0092
Devasirvatham, V., Tan, D. K., and Trethowan, R. M. (2016). “Breeding strategies for enhanced plant tolerance to heat stress,” in Advances in Plant Breeding Strategies: Agronomic, Abiotic and Biotic Stress Traits, eds J. Al-Khayri, S. Jain, and D. Johnson (Berlin: Springer), 447–469.
Dias, A., and Lidon, F. (2009). Evaluation of grain filling rate and duration in bread and durum wheat, under heat stress after anthesis. J. Agron. Crop Sci. 195, 137–147. doi: 10.1111/j.1439-037X.2008.00347.x
Dreisigacker, S., Kishii, M., Lage, J., and Warburton, M. (2008). Use of synthetic hexaploid wheat to increase diversity for CIMMYT bread wheat improvement. Crop Pasture Sci. 59, 413–420. doi: 10.1071/AR07225
Dupont, F. M., Hurkman, W. J., Vensel, W. H., Tanaka, C., Kothari, K. M., Chung, O. K., et al. (2006). Protein accumulation and composition in wheat grains: effects of mineral nutrients and high temperature. Eur. J. Agron. 25, 96–107. doi: 10.1016/j.eja.2006.04.003
Dwivedi, S. L., Ceccarelli, S., Blair, M. W., Upadhyaya, H. D., Are, A. K., and Ortiz, R. (2016). Landrace germplasm for improving yield and abiotic stress adaptation. Trends Plant Sci. 21, 31–42. doi: 10.1016/j.tplants.2015.10.012
Edmeades, G., Cooper, M., Lafitte, R., Zinselmeier, C., Ribaut, J., Habben, J., et al. (2001). Abiotic stresses and staple crops. Crop Science: Progress and Prospects. Papers Presented at the Third International Crop Science Congress, (Hamburg: CABI Publishing), 137–154.
Farooq, M., Bramley, H., Palta, J. A., and Siddique, K. H. (2011). Heat stress in wheat during reproductive and grain-filling phases. Crit. Rev. Plant Sci. 30, 491–507. doi: 10.1080/07352689.2011.615687
Fischer, R., Byerlee, D., and Edmeades, G. (2014). Crop Yields and Global Food Security: Will Yield Increase Continue to Feed the World? Canberra: Australian Centre for International Agricultural Research.
Gilmour, A., Gogel, B., Cullis, B., and Thompson, R. (2009). ASReml User Guide Release 3.0. Hemel Hempstead: VSN International Ltd.
Hassan, M. I., Mohamed, E. A., El-rawy, M. A., and Amein, K. A. (2016). Evaluating interspecific wheat hybrids based on heat and drought stress tolerance. J. Crop Sci. Biotechnol. 19, 85–98. doi: 10.1007/s12892-015-0085-x
Lobell, D. B., Sibley, A., and Ortiz-Monasterio, J. I. (2012). Extreme heat effects on wheat senescence in India. Nat. Clim. Chang. 2, 186–189. doi: 10.1038/nclimate1356
Lopes, M. S., and Reynolds, M. P. (2012). Stay-green in spring wheat can be determined by spectral reflectance measurements (normalized difference vegetation index) independently from phenology. J. Exp. Bot. 63, 3789–3798. doi: 10.1093/jxb/ers071
Malik, A. H., Kuktaite, R., and Johansson, E. (2013). Combined effect of genetic and environmental factors on the accumulation of proteins in the wheat grain and their relationship to bread-making quality. J. Cereal Sci. 57, 170–174. doi: 10.1016/j.jcs.2012.09.017
Mondal, S., Singh, R., Crossa, J., Huerta-Espino, J., Sharma, I., Chatrath, R., et al. (2013). Earliness in wheat: a key to adaptation under terminal and continual high temperature stress in South Asia. Field Crops Res. 151, 19–26. doi: 10.1016/j.fcr.2013.06.015
Nevo, E. (2014). Evolution of wild emmer wheat and crop improvement. J. Syst. Evol. 52, 673–696. doi: 10.1111/jse.12124
Pask, A., Pietragalla, J., Mullan, D., and Reynolds, M. (2012). Physiological Breeding II: A Field Guide to Wheat Phenotyping. Mexico: CIMMYT.
Payne, R., Murray, D., Harding, S., Baird, D., and Soutar, D. (2011). An Introduction to GENSTAT for Windows, 14th Edn. Hemel Hempstead: VSN International.
Petrarulo, M., Marone, D., De Vita, P., Sillero, J., Ferragonio, P., Giovanniello, V., et al. (2009). “Mapping QTLs for root morphological traits in durum wheat,” in International Symposium “Root Research and Applications, (Vienna:RootRap).
Pinto, R. S., Molero, G., and Reynolds, M. P. (2017). Identification of heat tolerant wheat lines showing genetic variation in leaf respiration and other physiological traits. Euphytica 213:76. doi: 10.1007/s10681-017-1858-8
Rasmusson, D., and Phillips, R. (1997). Plant breeding progress and genetic diversity from de novo variation and elevated epistasis. Crop Sci. 37, 303–310. doi: 10.2135/cropsci1997.0011183X003700020001x
Rebetzke, G., Condon, A. G., Farquhar, G., Appels, R., and Richards, R. (2008). Quantitative trait loci for carbon isotope discrimination are repeatable across environments and wheat mapping populations. Theor. Appl. Genet. 118, 123–137. doi: 10.1007/s00122-008-0882-4
Reynolds, M., Dreccer, F., and Trethowan, R. (2007). Drought-adaptive traits derived from wheat wild relatives and landraces. J. Exp. Bot. 58, 177–186. doi: 10.1093/jxb/erl250
Richards, R., Watt, M., and Rebetzke, G. (2007). Physiological traits and cereal germplasm for sustainable agricultural systems. Euphytica 154, 409–425. doi: 10.1007/s10681-006-9286-1
Sharma, S., Upadhyaya, H. D., Varshney, R. K., and Gowda, C. (2013). Pre-breeding for diversification of primary gene pool and genetic enhancement of grain legumes. Front. Plant Sci. 4:309. doi: 10.3389/fpls.2013.00309
Singha, P., Bhowmick, J., and Chaudhuri, B. (2006). Effect of temperature on yield and yield components of fourteen wheat (Triticum aestivum L.) genotypes. Environ. Ecol. 24:550.
Teixeira, E. I., Fischer, G., van Velthuizen, H., Walter, C., and Ewert, F. (2013). Global hot-spots of heat stress on agricultural crops due to climate change. Agricult. Forest Meteorol. 170, 206–215. doi: 10.1016/j.agrformet.2011.09.002
Tewolde, H., Fernandez, C., and Erickson, C. (2006). Wheat cultivars adapted to post-heading high temperature stress. J. Agron. Crop Sci. 192, 111–120. doi: 10.1111/j.1439-037X.2006.00189.x
Thistlethwaite, R. J. (2017). Identification of Genetic Variation in Heat Stress, Genotype Screening for and Mechanisms of Tolerance in Wheat. Ph.D. dessertation, The University of Sydney, Sydney.
Trethowan, R., and Mujeeb-Kazi, A. (2008). Novel germplasm resources for improving environmental stress tolerance of hexaploid wheat. Crop Sci. 48, 1255–1265. doi: 10.2135/cropsci2007.08.0477
Troccoli, A., and Codianni, P. (2005). Appropriate seeding rate for einkorn, emmer, and spelt grown under rainfed condition in southern Italy. Eur. J. Agron. 22, 293–300. doi: 10.1016/j.eja.2004.04.003
van Ginkel, M., and Ogbonnaya, F. (2007). Novel genetic diversity from synthetic wheats in breeding cultivars for changing production conditions. Field Crops Res. 104, 86–94. doi: 10.1016/j.fcr.2007.02.005
Wang, H., Liu, D., Yan, Z., Wei, Y., and Zheng, Y. (2005). Cytological characteristics of F∼ 2 hybrids between Triticum aestivum L. and T. durum Desf. with reference to wheat breeding. J. Appl. Genet. 46, 365–369.
Wang, S., Wong, D., Forrest, K., Allen, A., Chao, S., Huang, B. E., et al. (2014). Characterization of polyploid wheat genomic diversity using a high-density 90 000 single nucleotide polymorphism array. Plant Biotechnol. J. 12, 787–796. doi: 10.1111/pbi.12183
Xie, W., and Nevo, E. (2008). Wild emmer: genetic resources, gene mapping and potential for wheat improvement. Euphytica 164, 603–614. doi: 10.1038/srep10763
Zaharieva, M., Ayana, N. G., Hakimi, A. A., Misra, S. C., and Monneveux, P. (2010). Cultivated emmer wheat (Triticum dicoccon Schrank), an old crop with promising future: a review. Genet. Resour. Crop Evol. 57, 937–962. doi: 10.1007/s10722-010-9572-6
Zhang, B., Li, W., Chang, X., Li, R., and Jing, R. (2014). Effects of favorable alleles for water-soluble carbohydrates at grain filling on grain weight under drought and heat stresses in wheat. PLoS One 9:e102917. doi: 10.1371/journal.pone.0102917
Keywords: emmer wheat, genetic diversity, genotyping, hexaploid wheat, agronomic traits, heat tolerance
Citation: Ullah S, Bramley H, Daetwyler H, He S, Mahmood T, Thistlethwaite R and Trethowan R (2018) Genetic Contribution of Emmer Wheat (Triticum dicoccon Schrank) to Heat Tolerance of Bread Wheat. Front. Plant Sci. 9:1529. doi: 10.3389/fpls.2018.01529
Received: 16 August 2018; Accepted: 28 September 2018;
Published: 20 November 2018.
Edited by:
Jacqueline Batley, University of Western Australia, AustraliaReviewed by:
Ahmad Arzani, Isfahan University of Technology, IranShun Sakuma, Tottori University, Japan
Copyright © 2018 Ullah, Bramley, Daetwyler, He, Mahmood, Thistlethwaite and Trethowan. This is an open-access article distributed under the terms of the Creative Commons Attribution License (CC BY). The use, distribution or reproduction in other forums is permitted, provided the original author(s) and the copyright owner(s) are credited and that the original publication in this journal is cited, in accordance with accepted academic practice. No use, distribution or reproduction is permitted which does not comply with these terms.
*Correspondence: Smi Ullah, c21pLnVsbGFoQHN5ZG5leS5lZHUuYXU=