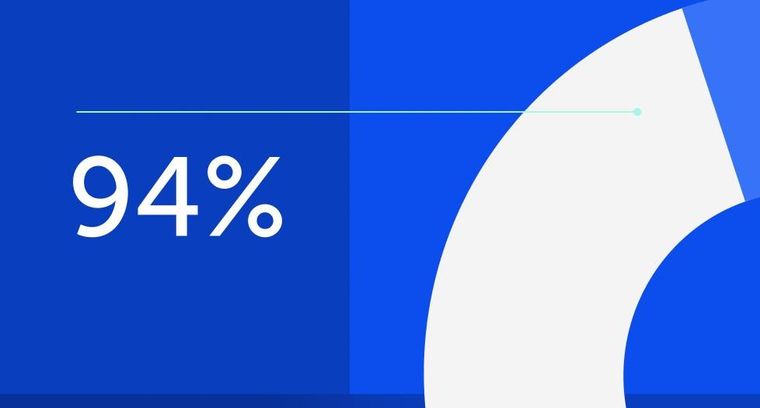
94% of researchers rate our articles as excellent or good
Learn more about the work of our research integrity team to safeguard the quality of each article we publish.
Find out more
ORIGINAL RESEARCH article
Front. Plant Sci., 18 September 2018
Sec. Plant Physiology
Volume 9 - 2018 | https://doi.org/10.3389/fpls.2018.01370
This article is part of the Research TopicFruit Ripening: From Present Knowledge to Future DevelopmentView all 13 articles
Light signaling and plant hormones, particularly ethylene and auxins, have been identified as important regulators of carotenoid biosynthesis during tomato fruit ripening. However, whether and how the light and hormonal signaling cascades crosstalk to control this metabolic route remain poorly elucidated. Here, the potential involvement of ethylene and auxins in the light-mediated regulation of tomato fruit carotenogenesis was investigated by comparing the impacts of light treatments and the light-hyperresponsive high pigment-2 (hp2) mutation on both carotenoid synthesis and hormonal signaling. Under either light or dark conditions, the overaccumulation of carotenoids in hp2 ripening fruits was associated with disturbed ethylene production, increased expression of genes encoding master regulators of ripening and higher ethylene sensitivity and signaling output. The increased ethylene sensitivity observed in hp2 fruits was associated with the differential expression of genes encoding ethylene receptors and downstream signaling transduction elements, including the downregulation of the transcription factor ETHYLENE RESPONSE FACTOR.E4, a repressor of carotenoid synthesis. Accordingly, treatments with exogenous ethylene promoted carotenoid biosynthetic genes more intensively in hp2 than in wild-type fruits. Moreover, the loss of HP2 function drastically altered auxin signaling in tomato fruits, resulting in higher activation of the auxin-responsive promoter DR5, severe down-regulation of AUXIN/INDOLE-3-ACETIC ACID (Aux/IAA) genes and altered accumulation of AUXIN RESPONSE FACTOR (ARF) transcripts. Both tomato ARF2 paralogues (Sl-ARF2a and SlARF2b) were up-regulated in hp2 fruits, which agrees with the promotive roles played by these ARFs in tomato fruit ripening and carotenoid biosynthesis. Among the genes differentially expressed in hp2 fruits, the additive effect of light treatment and loss of HP2 function was particularly evident for those encoding carotenoid biosynthetic enzymes, ethylene-related transcription factors, Aux/IAAs and ARFs. Altogether, the data uncover the involvement of ethylene and auxin as part of the light signaling cascades controlling tomato fruit metabolism and provide a new link between light signaling, plant hormone sensitivity and carotenoid metabolism in ripening fruits.
Light plays a dual role during plant development, providing energy for photosynthesis and information for adjusting plant growth, development and reproduction. Processes as diverse as seed germination, seedling de-etiolation, phototropism, flowering, fruit pigmentation and entrainment of circadian rhythms are intrinsically regulated by light stimuli (Azari et al., 2010a; Llorente et al., 2016a). In tomato, a model crop for fleshy fruits, multiple photomorphogenic mutants have been identified over the years, greatly facilitating the deciphering of the relevance of light signaling in fruit biology and quality traits (Levin et al., 2006; Azari et al., 2010b). Among these genotypes, the tomato high pigment (hp) mutants hp1 and hp2 have been instrumental in illustrating the positive role of light signaling in fruit metabolism and nutritional composition. These mutants are characterized by their exaggerated light responsiveness, over-accumulation of chlorophyll and chloroplasts in leaves and immature fruits as well as intense red fruit pigmentation (Mustilli et al., 1999; Levin et al., 2003, 2006). Compared to their WT counterparts, hp ripe fruits display higher levels of health-promoting substances, including carotenoids, flavonoids, tocopherol (vitamin E) and ascorbic acid (vitamin C) (Yen et al., 1997; Liu et al., 2004; Kolotilin et al., 2007). Fruit carotenogenesis is particularly up-regulated in hp mutants, which agrees with the positive influence of light on isoprenoid metabolism in both fruit and vegetative tissues (Piringer and Heinze, 1954; Alba et al., 2000; Schofield and Paliyath, 2005).
Genetic analysis of hp1 and hp2 alleles revealed mutations in tomato homologs of the nuclear proteins UV-DAMAGED DNA BINDING PROTEIN1 (DDB1) and DEETIOLATED1 (DET1), respectively, two negative regulators of light signal transduction (Mustilli et al., 1999; Schroeder et al., 2002; Levin et al., 2003; Lieberman et al., 2004; Liu et al., 2004). Confirming these findings, silencing of Sl-DDB1/HP1 or Sl-DET1/HP2 greatly promotes plastid biogenesis and carotenoid accumulation in fruit tissues (Davuluri et al., 2004, 2005; Wang et al., 2008). Besides Sl-DDB1/HP1 and Sl-DET1/HP2, other components of the light signaling cascade have also been implicated in controlling tomato fruit metabolism, including the E3 ubiquitin-ligases CULLIN4 (CUL4) and CONSTITUTIVELY PHOTOMORPHOGENIC 1 (COP1), as well as the transcription factors ELONGATED HYPOCOTYL 5 (HY5) and PHYTOCHROME-INTERACTING FACTORs (PIFs) (Liu et al., 2004; Davuluri et al., 2005; Wang et al., 2008; Llorente et al., 2016b). Constitutive silencing of tomato CUL4, COP1 or PIF1a generates fruits with increased carotenoid levels (Liu et al., 2004; Wang et al., 2008; Llorente et al., 2016b), whereas the opposite phenotype is caused by the suppression of the light-signaling effector HY5 (Liu et al., 2014). Significant alterations in carotenoid biosynthesis have also been observed in ripening fruits of transgenic plants with fruit-specific silencing of phytochrome (PHY)-encoding genes (Bianchetti et al., 2018), as well as in cryptochrome1a (CRY1a)-deficient mutants and CRY1a-overexpressing lines (Liu et al., 2018).
Virtually all fruit metabolic processes influenced by light are also strictly controlled by an integrated, multi-hormonal signaling network (Giovannoni, 2004; Karlova et al., 2014; Liu M. et al., 2015). Compelling data implicate ethylene as a primary regulator of multiple ripening-related physiological, biochemical, and molecular processes (Barry and Giovannoni, 2007; Pech et al., 2012). Accordingly, disturbed ethylene biosynthesis, perception or signal transduction directly impact fruit ripening initiation and progression (Liu M. et al., 2015). Without undermining the role of ethylene, auxins have also been shown to interfere with fruit ripening and carotenoid accumulation, as revealed by the delayed ripening phenotype and the down-regulation in carotenoid biosynthesis observed in IAA-treated tomato fruits (Su et al., 2015).
Although light signaling and plant hormones, such as ethylene and auxins, are essential regulators of tomato fruit ripening and carotenogenesis, whether and how the light and hormonal signaling cascades crosstalk to control these metabolic processes remains poorly elucidated. Here, the potential involvement of ethylene and auxins in the light-mediated regulation of tomato fruit ripening and carotenogenesis was investigated by comparing the impact of light and dark treatments, isolated or combined with the loss of Sl-DET1/HP2 function, on both carotenoid synthesis and hormonal signaling.
Wild-type (WT) Solanum lycopersicum L. (cv. Micro-Tom), a near-isogenic line (NIL) harboring the mutation high pigment-2 (hp2), and transgenic plants carrying the synthetic auxin-responsive (DR5) or ethylene-responsive (EBS) promoters fused to the reporter gene uid (encoding a β-glucuronidase, GUS) were obtained from the tomato mutant collection maintained at ESALQ, University of São Paulo (USP), Brazil (Carvalho et al., 2011). Crosses and successive screening were performed to generate the double mutants hp2-DR5::GUS and hp2-EBS::GUS. Plants were grown in 6-L rectangular pots containing a 1:1 mixture of commercial substrate (Plantmax HT, Eucatex, São Paulo, Brazil) and expanded vermiculite, supplemented with 1 g L-1 of NPK 10:10:10, 4 g L-1 of dolomite limestone (MgCO3 + CaCO3) and 2 g L-1 thermophosphate (Yoorin Master®, Yoorin Fertilizantes, Brazil) in greenhouse under automatic irrigation at an average mean temperature of 25°C, 11.5 h/13 h (winter/summer) photoperiod and approximately 250–350 μmol m-2 s-1 PAR irradiance.
Fruits at mature green (MG) stage were harvested about 30 days after anthesis (dpa) and transferred to continuous white light or maintained under absolute darkness (D) until reaching distinct ripening stages. White light was delivered at around 50 μmol m-2 s-1 and supplied by an array of SMD5050 Samsung LEDs mounted in a temperature-controlled growth chamber maintained at 25 ± 1°C and air relative humidity at 80 ± 5%. Top and bottom illumination were applied to homogenize the light environment surrounding the fruits. Fruits were placed into a 0.5-L sealed transparent vessel and continuously flushed with ethylene-free, humidified air (1 L min-1) to avoid accumulation of ET inside the containers. Samples from light- or dark-incubated fruits were harvested under white light or dim green light, respectively. Harvesting was performed at the same daytime to avoid possible fluctuations in the parameters due to circadian rhythm. Pericarp samples (without seeds, columella, placental tissues and locule walls) were harvested as soon as the fruits had reached the following ripening stages: MG (displaying jelly placental tissues, 2 days after harvesting), Bk (breaker, showing the first external yellow color signals) and Bk1, Bk3, Bk6, and Bk12, corresponding 1, 3, 6, and 12 days after Bk, respectively. Fruits at distinct treatments achieved each ripening stage at a different number of days of treatment. Four biological samples composed of at least five fruits each were harvested at each sampling time. Ethylene emission analysis and quantitative in vitro GUS activity assays were performed immediately after harvesting. For all other analyses, samples were frozen in liquid N2, powdered and stored at -80°C until use.
Fruits harvested at the MG stage were submitted to ethylene or auxin treatment at 25°C in the presence of white light (50 μmol m-2 s-1). For the ethylene treatment, fruits were kept inside transparent sealed tubes in the presence of 50 ppm of ethylene, whereas control fruits were maintained in ethylene-free air. For the auxin treatment, fruits were injected with a buffer solution containing 10 mM 2-(N-morpholino) ethanesulfonic acid (MES) pH 5.6, 3% sorbitol (w/v) and 100 μM of indole-3-acetic acid (IAA) whereas control fruits were treated with buffer without IAA (Su et al., 2015). After 6 h treatment, fruit pericarp samples were collected before snap freezing in nitrogen.
Chlorophyll extraction and quantification were carried out as described in Bianchetti et al. (2018). Carotenoids (namely lycopene, β-carotene and lutein) were extracted and analyzed by high-pressure liquid chromatography (HPLC) with photodiode array detector (PDA). Carotenoid extraction was performed precisely as described by Bianchetti et al. (2018). Chromatography was carried out on an Agilent Technologies series 1100 HPLC system on a normal-phase column Phenomenex (Luna C18; 250 × 4.6 mm; 5 μm particle diameter) with a flow rate of 1 mL min-1 at 25°C. The mobile phase was a gradient of ethyl acetate (A) and acetonitrile:water 9:1 (v/v) (B): 0–4 min: 20% A; 4–30 min: 20–65% A; 30–35 min: 65% A; 35–40 min: 65–20% A. Eluted compounds were detected between 340 and 700 nm and quantified at 450 nm. The endogenous metabolite concentration was obtained by comparing the peak areas of the chromatograms with commercial standards.
Fruit surface color was assessed with a using Konica Minolta CR-400 colorimeter, using the D65 illuminant and the L∗, a∗, b∗ space, and the data were processed to obtain hue and chroma values. Three measures were taken at the equator of each fruit and average values were calculated. The hue angle (in degrees) was calculated according to the following equations: hue = tan-1 (b∗/a∗) if a > 0 and 180 + tan-1 (b∗/a∗) if a < 0 (Ecarnot et al., 2013).
Antioxidant activity was measured using the method of Trolox equivalent antioxidant capacity (TEAC). Frozen pericarp samples (approximately 200 mg FW) ground in liquid nitrogen were homogenized with 1 mL of 100 mM sodium acetate buffer (pH 5) and shaken for 30 min at 4°C. After centrifugation (4°C, 5000 g, 10 min), the supernatant was discarded, 0.5 mL of hexane was added to the pellet, and the suspension was kept shaking for 30 min at 4°C. After centrifugation (4°C, 5000 g, 10 min), the supernatant was collected, and the same process was repeated twice. The lipophilic antioxidant extract was concentrated and suspended in 150 μL of hexane. Absorbance was read at 734 nm after 2 h of incubation under darkness. The activity of the extract was determined by the deactivation of 2,2′-azino-bis(3-ethylbenzothiazoline-6-sulfonic acid (ABTS+) compared to a standard curve of 6-hydroxy-2,5,7,8-tetramethylchroman-2-carboxylic acid (Trolox).
Endogenous levels of indole acetic acid (IAA) were determined by gas chromatography-tandem mass spectrometry-selecting ion monitoring (GC-MS-SIM) as described by Santana-Vieira et al. (2016). Frozen pericarp samples (approximately 100 mg FW) were ground in liquid nitrogen and homogenized with 1 mL of isopropanol:acetic acid (95:5, v/v). Precisely 0.5 μg [13C6]-IAA (Cambridge Isotopes, Inc.) was added to each sample as internal standards. Samples were incubated at 4°C for approximately 2 h. After centrifugation (4°C, 16.000 g, 20 min), the supernatant was collected, and 100 μL of ultrapure water and 500 μl of ethyl acetate were added. After centrifugation (4°C, 16.000 g, 5 min) the supernatant was collected, and this step was repeated. The extract was completely vacuum dried and suspended in 50 μL methanol followed by a 30-min derivatization step at room temperature using 40 μL (trimethylsilyl)diazomethane.
The analysis was performed with a gas chromatograph coupled to a mass spectrometer (model GCMS-QP2010 SE, Shimadzu) in selective ion monitoring mode. One microliter of each sample was automatically injected (model AOC-20i, Shimadzu) on splitless mode, using helium as the carrier gas at a flow rate of 4.5 mL min-1 through a fused-silica capillary column (30 m, 0.25 mm ID, 0.50-μm-thick internal film) DB-5 MS stationary phase in the following program: 2 min at 100°C, followed by gradients of 10°C min-1 to 140°C, 25°C min-1 to 160°C, 35°C min-1 to 250°C, 20°C min-1 to 270°C and 30°C min-1 to 300°C. The injector temperature was 250°C, and the following MS operating parameters were used: ionization voltage, 70 eV (electron impact ionization); ion source temperature, 230°C; and interface temperature, 260°C. Ions with a mass ratio/charge (m/z) of 130 and 189 (corresponding to endogenous IAA) and 136 and 195 (corresponding to [13C6]-IAA) were monitored. Endogenous concentrations were calculated based on extracted chromatograms at m/z 130 and 136.
Ethylene emission was analyzed by gas chromatography with a flame-ionization detector (GC-FID) as described in Melo et al. (2016). Intact tomato fruits (typically 4 individuals) were enclosed in a sealed transparent tube for 1 h under specific experimental conditions. After incubation, 9-mL gas samples were collected from tubes and injected into a glass vial headspace previously flushed with ethylene-free air (1 L min-1) for 1 min. At least three 1-mL aliquots of each sample were injected in a headspace coupled to a Trace GC Ultra gas chromatography (Thermo Electron) fitted with a flame ionization detector (GC-FID) using an RT-alumina Plot column (Restek Corporation). Nitrogen was used as the carrier gas at a flow rate of 3 mL min-1, and commercial standard mixtures of ethylene were used for the calibration curves. Column, injector and detector temperatures were 34, 250, and 250°C, respectively.
ACC was extracted and subsequently quantified as described by Bulens et al. (2011). Frozen pericarp samples (approximately 1 g FW) were ground in liquid nitrogen and homogenized with 4 mL of a 5% (w/v) sulfosalicylic acid aqueous solution. Extracts were shaken for 30 min at 4°C at 180 rpm in the dark. The supernatant was collected after centrifugation at 4°C, 5000 g, for 10 min. The reactions were performed by adding 1.4 mL of extract to a reaction mixture composed of 0.4 mL of 10 mM HgCl2 and 0.2 mL of a 2:1 (v/v) solution of NaOCl 5%:NaOH 6 M. The final product of this reaction, ethylene, was measured by GC-FID as described above.
ACO extraction and activity assay were performed according to Bulens et al. (2011). Frozen pericarp samples (approximately 100 mg FW) were ground in liquid nitrogen and homogenized with extraction buffer composed of 300 mM Tris-HCl (pH 8.0), 15 mg mL-1 insoluble polyvinylpolypyrrolidone (PVPP), 10% (v/v) glycerol and 30 mM ascorbic acid. After centrifugation (4°C, 20000 g, 20 min), 200 μL of the supernatant was added to 1.8 mL of reaction medium composed of 100 mM Tris-HCl (pH 8.0), 10% (v/v) glycerol, 30 mM ascorbic acid, 100 μM FeSO4, 50 mM NaHCO3, 5 mM DTT and 2 mM ACC. ACO activity was estimated by measuring the ability of the extract to convert exogenous ACC to ethylene after incubation at 30°C for 60 min. The ethylene formed during the reactions was measured by GC-FID as described above.
GUS activity was assayed according to Melo et al. (2016). Frozen pericarp samples (approximately 500 mg FW) were ground in liquid nitrogen and homogenized in 1 mL extraction buffer composed of 50 mM Hepes-KOH (pH 7.0), 5 mM DTT and 0.5% (w/v) PVP. After centrifugation (4°C, 20.000 g, 20 min), 200 μL aliquots of the supernatant were mixed with 200 μL of an assay buffer composed of 50 mM HEPES-KOH (pH 7.0), 5 mM DTT, 10 mM EDTA and 2 mM 4-methylumbelliferyl-β-D-glucuronide (MUG) and incubated at 37°C for 30 min. Subsequently, aliquots of 100 μL were taken from each tube and the reactions were stopped with 2.9 mL of 0.2 M Na2CO3 (pH 9.5). Fluorescence was measured using 365 nm excitation and 460 nm emission wavelength (5 nm bandwidth) by using a spectrofluorometer (LS55, Perkin Elmer). The same instrument settings were maintained throughout the experiments.
Promoter sequences were retrieved from Sol Genomics Network1 and analyzed using PlantPAN 2.0 platform2 (Chow et al., 2016) to identify the regulatory motifs. Fragments of 3 kb upstream from the initial codon ATG were analyzed for the presence of PBE-box (CACATG), G-box (CACGTG), CA-hybrid (GACGTA) and CG-hybrid (GACGTG) motifs, which are recognized by HY5 and/or PIFs (Martínez-García et al., 2000; Song et al., 2008).
Total RNA extraction was performed using ReliaPrepTM RNA Tissue Miniprep System (Promega) according to manufacturer’s instructions for fibrous tissues. Total RNA and integrity of samples were determined using spectrophotometer and 1% (w/v) agarose gel. Only RNA samples with 260/280 and 260/230 ratio values within 1.8–2.2 were used for the subsequent steps. Approximately 1 μg of total RNA was treated with DNase (DNase I Amplification Grade, Thermo Fisher Scientific) for 30 min at room temperature and complementary DNA (cDNA) was synthesized using SuperScript® IV Reverse Transcriptase kit (Thermo Fisher Scientific) according to manufacturer’s instructions. Only cDNA samples free of DNA contamination were used in the subsequent steps.
Quantitative reverse-transcriptase PCR (qPCR) reactions were performed using the StepOnePlusTM Real-Time PCR System (Applied Biosystems) using 10 μl mix reaction composed of 5 μL Power SYBR green 2X (Thermo Fisher Scientific), 2 μL cDNA sample and 200 nM of forward and 200 nM of reverse primer. The amplification program consisted of 10 min initial step at 95°C, followed by 40 cycles with 15 s 95°C, 30 s 55/60°C and 30 s 72°C. Melting curve was analyzed to detect unspecific amplifications and primer dimerization. The primer sequences used in this study are listed in Supplementary Table 1. Fluorescence data were analyzed using LingReg PCR software, and expression values were normalized against mean values of two references genes: Sl-EXPRESSED and Sl-CAC, which have been already successfully used to normalize data from fruit development and ripening experiments (Expósito-Rodríguez et al., 2008; Bianchetti et al., 2018).
Two-way analysis of variance (ANOVA) was performed to determine effects of genotype, light treatment and their interactions, using JMP statistical software package (14th edition)3. One-way ANOVA with Tukey’s test or Student’s t-test was used to discriminate means of samples within and between genotypes, respectively. Comparisons with P < 0.05 were considered statistically significant. Carotenoid-related data were also compared via principal component analysis (PCA) using JMP statistical software package.
The impacts of Sl-DET1/HP2 knockout or knockdown on tomato fruit carotenogenesis have been exclusively investigated in fruits ripening on-the-vine under greenhouse conditions (Davuluri et al., 2004; Kolotilin et al., 2007; Azari et al., 2010a; Enfissi et al., 2010; Sestari et al., 2014). However, after reaching the MG stage, tomato fruits are also able to ripen off-the-vine (i.e., isolated from the plant), a frequent commercial practice in harvesting tomato fruit for human consumption (Sorrequieta et al., 2013).
Here, we demonstrated that the loss of Sl-DET1/HP2 function promotes carotenogenesis even when tomato ripening occurs separated from the plant under either light or absolute dark conditions (Figure 1 and Supplementary Figure 1). Two-way ANOVA showed that both the hp2 mutation and the light treatment had a significant (P < 0.05) effect on carotenoid biosynthesis and accumulation (Supplementary Table 2). In both light- and dark-treated fruits, lutein and β-carotene levels were significantly higher in hp2 than in the WT at virtually all sampling stages (Figure 1B). Moreover, lycopene levels of dark-treated hp2 fruits were higher than the WT at the final stages of ripening (i.e., Bk6 and Bk12). In agreement, the genes encoding key carotenoid biosynthesis-related enzymes such as geranylgeranyl diphosphate synthase (GGPS), phytoene synthase 1 (PSY1) and phytoene desaturase (PDS) were strongly up-regulated during the climacteric phase (i.e., Bk to Bk6) in both light- and dark-treated hp2 fruits compared with WT counterparts (Figure 1C and Supplementary Figure 1). Overall, Sl-GGPS, Sl-PSY1 and Sl-PDS transcripts were less abundant in fruits maintained under dark than under light conditions, and this dark-induced reduction in mRNA levels was less marked in the hp2 mutant compared to the WT (Figure 1C). Genes encoding the chloroplast- and chromoplast-specific β-lycopene cyclases (LYCβ and CYCβ, respectively) were also up-regulated in hp2 fruits compared to the WT, particularly when ripening occurred under light conditions. Among the carotenoid biosynthesis-related genes differently expressed in hp2 fruits, the additive effect of light treatment and loss of Sl-DET1/HP2 function was particularly observed at the Bk, Bk1 and Bk12 stages (Supplementary Figure 1). Interestingly, lycopene levels were slightly higher in hp2 fruits ripened in the dark than in light-treated ones (Figure 1B), which is very likely due to the accumulation of this carotenoid because the opposite pattern was observed for the transcript levels of Sl-PSY1 and Sl-PDS, i.e., higher mRNA levels in the light than in the dark conditions (Figure 1C).
FIGURE 1. Loss of Sl-DET1/HP2 function promotes tomato fruit carotenoid biosynthesis and antioxidant capacity in both dark- and light-ripened fruits. Wild-type (WT) and high pigment-2 (hp2) fruits harvested at mature green (MG) stage were left to ripen under constant light (L) or dark (D) conditions. Pericarp samples were harvested at MG (2 days after the beginning of treatment), breaker (Bk), Bk1 (1 day after Bk), Bk3, Bk6, and Bk12 stages. (A) Schematic representation of carotenoid biosynthetic pathway in tomato. Intermediate reactions are omitted. (B) Lutein, β-carotene and lycopene content in pericarp tissues. (C) Relative mRNA levels of carotenoid biosynthesis genes. Mean relative expression was normalized against wild-type (WT) samples at mature green (MG) stage under dark conditions. (D) Trolox equivalent antioxidant capacity (TEAC) content in lipophilic extracts. Data are means (±SE) of at least three biological replicates. Different letters indicate statistically significant differences (Tukey’s test, p < 0.05) within each genotype. Asterisks indicate statistically significant differences (Student’s t-test, p < 0.05) between genotypes. MEP, Methylerythritol 4-phosphate; GGDP, Geranylgeranyl diphosphate; GGPS, GGDP synthase; PSY, Phytoene synthase; PDS, Phytoene desaturase; LCYβ, Chloroplast-specific β-lycopene cyclase; CYCβ, Chromoplast-specific β-lycopene cyclase.
In line with the increment in carotenoid content observed in hp2 fruits, lipophilic extracts obtained from either dark- or light-incubated fruits of the mutant exhibited higher values of antioxidant capacity than the WT counterparts, a response intensified under light conditions (Figure 1D). The influence of the hp2 mutation on lycopene, β-carotene and antioxidant capacity was moderated by the light treatment, as indicated by a significant genotype x light treatment interaction (P < 0.0001, Supplementary Table 2). Moreover, when PCA was performed with carotenoid data, the model explained 62.2% of the data variance for these conditions, displaying hp2 samples separated from WT independently of the developmental stage or light condition, and a strong positive correlation between the changes in mRNA levels of genes encoding carotenoid biosynthetic genes with the fruit carotenoid composition and antioxidant capacity was confirmed (Supplementary Figure 2).
At MG, hp2 fruits displayed a distinctive dark-green coloration, increased chlorophyll levels and higher color saturation (chroma, which is indicative of color intensity) compared to the WT (Supplementary Figure 3). In line with the higher content of pigments in hp2 than in WT fruits, an overall trend of higher values of fruit color intensity was observed in the mutant fruits during ripening (Bk to Bk12) regardless of the light conditions (Supplementary Figure 3).
As dark-incubated hp2 fruits showed carotenoid levels and lipophilic antioxidant capacity higher than dark- or even light-treated WT fruits, this mutation seems to represent a valid strategy to promote fruit nutritional quality even when the light stimulus is not present during fruit ripening.
To investigate whether the loss of Sl-DET1/HP2 function impacts tomato fruit ripening initiation and progression, we first monitored the ripening-associated changes in fruit color in both the hp2 and WT genotypes (Figure 2A). Hue angle values revealed that light-incubated fruits acquired the distinctive red coloration faster and more intensively than those kept under complete darkness. Moreover, the ripening-associated fruit color transition occurred slightly faster in hp2 than in WT fruits, particularly under dark conditions (Figure 2A).
FIGURE 2. Light signaling influences tomato fruit ripening. Treatment details as described in Figure 1. (A) Ripening-related changes in fruit color (Hue angle). (B) Transcript abundance of ripening regulator genes in dark- and light ripened fruits. Mean relative expression was normalized against wild-type (WT) samples at mature green (MG) stage under dark conditions. Data are means (±SE) of at least three biological replicates. Different letters indicate statistically significant differences (Tukey’s test, p < 0.05) within each genotype. Asterisks indicate statistically significant differences (Student’s t-test, p < 0.05) between genotypes. hp2, high pigment-2; Bk, Breaker; RIN, ripening inhibitor; NOR, non-ripening; FUL1, fruitfull1; AP2a, apetala2a; TAGL1, tomato agamous-like1.
In line with these results, mRNA levels of genes encoding the master regulators of ripening RIPENING INHIBITOR (RIN), NON-RIPENING (NOR), FRUITFULL1 (FUL1), APETALA2a (AP2a) and TOMATO AGAMOUS-LIKE1 (TAGL1) were significantly higher in hp2 than in WT fruits ripening either under light or dark conditions (Figure 2B). Overall, the impact of the loss of Sl-DET1/HP2 function on the transcript abundance of these ripening-associated genes was influenced by the light treatment, as indicated by a significant genotype x light treatment interaction (P < 0.05, Supplementary Table 2). Therefore, a positive correlation was observed between the up-regulation of the master regulators of ripening and the carotenoid overaccumulation observed in hp2 ripening fruits. The promotive impact of the loss of Sl-DET1/HP2 function on the expression of master regulators of ripening may also be linked to the slightly faster fruit color transition observed in the mutant compared to the WT under dark conditions (Figure 2A). Accordingly, HY5- and/or PIF-binding motifs were identified in the promoter regions of all five master regulators of ripening genes analyzed (Supplementary Figure 4).
To gain insight into the potential influence of light treatment and the loss of Sl-DET1/HP2 function on fruit ethylene metabolism, we next monitored ethylene emission, 1-aminocyclopropane-1-carboxylic acid (ACC) content, ACC oxidase (ACO) activity and transcript abundance of ethylene biosynthetic genes in WT and hp2 ripening fruits. In both genotypes and light conditions, the highest values of ethylene emission were detected from Bk to Bk3 (Figure 3A). Also, ACC accumulated at the end of the ripening (Bk12) in all conditions analyzed, which was associated with a drastic reduction in ACO activity from BK stage onward (Figure 3A). Compared to the WT, hp2 fruits exhibited significantly lower ethylene emission rates, ACC content and ACO activity regardless of the light treatment. In both genotypes, climacteric ethylene emission was significantly lower under light than under dark conditions (Figure 3A).
FIGURE 3. Light-hypersensitivity represses ethylene metabolism in ripening tomato fruits. Treatment details as described in Figure 1. (A) Ethylene emission, 1-aminocyclopropane-1-carboxylic acid (ACC) content, in vitro ACC oxidase (ACO) activity. (B) Relative mRNA levels of tomato genes encoding ACO. (C) Relative mRNA levels of tomato genes encoding ACC synthase (ACS). Mean relative expression was normalized against wild-type (WT) samples at mature green (MG) stage under dark conditions. Data are means (±SE) of at least three biological replicates. Different letters indicate statistically significant differences (Tukey’s test, p < 0.05) within each genotype. Asterisks indicate statistically significant differences (Student’s t-test, p < 0.05) between genotypes. hp2, high pigment-2; Bk, Breaker.
To investigate whether these light-induced alterations in ethylene emission were associated with changes in the transcriptional profile of ethylene biosynthetic genes, the mRNA levels of all ACS- and ACO-encoding genes responsible for the climacteric ethylene burst in ripening tomato fruits were profiled. Overall, the influence of light exposure or the hp2 mutation on the transcript abundance of these genes was highly variable, greatly varying depending on the gene analyzed or the ripening stage (Figures 3B,C). Therefore, no clear correlation was observed between the transcriptional regulation of tomato ACS- and ACO-encoding genes (Figures 3B,C) and the reduced ethylene biosynthesis (Figure 3A) observed in light compared to the dark treatment or in hp2 compared to the WT genotype. Together, these findings indicate that light exposure and the hp2 mutation, either combined or isolated, can cause an overall down-regulation in tomato ethylene biosynthesis, which is associated with complex changes in the transcriptional profile of ACS and ACO genes.
Based on these findings, we further investigated whether light hypersensitivity alters ethylene signaling in ripening tomato fruits. First, the ethylene signaling output was determined by monitoring the activity of the reporter protein GUS expressed under the control of the EBS ethylene-responsive promoter in EBS::GUS and hp2-EBS::GUS genotypes. Whether under light or dark conditions, the highest GUS activity values in both genotypes coincided with the climacteric burst of ethylene production (Figure 4A). However, the loss of Sl-DET1/HP2 function resulted in higher EBS promoter activation, and this phenomenon was clearly intensified by the presence of light (Figure 4A).
FIGURE 4. Loss of Sl-DET1/HP2 function promotes ethylene tissue sensitivity and signaling output. Treatment details as described in Figure 1. (A) In vitro GUS activity assayed in wild-type (WT) and high pigment-2 (hp2) fruits carrying the ethylene-responsive promoter EBS fused to the GUS reporter protein (EBS::GUS and hp2-EBS::GUS). (B) Heatmap representation of the differences in relative mRNA levels of ethylene perception and signaling-related genes between the WT and hp2 fruits ripened under light or dark conditions. (C) Heatmap representation of the differences in relative mRNA levels of ethylene perception and signaling-related genes between light and dark samples of hp2 fruits at each sampling time. The relative transcript values are presented in Supplementary Figure 5. (D) Relative mRNA levels of tomato genes encoding carotenoid biosynthetic enzymes in WT and hp2 fruits treated with 50 ppm ethylene for 6 h. Data are means (±SE) of at least three biological replicates. Different letters indicate statistically significant differences (Tukey’s test, p < 0.05) within each genotype (in A) or among all data (in C). In A, asterisks indicate statistically significant differences (Student’s t-test, p < 0.05) between genotypes. MG, mature green; Bk, Breaker; ETR, ethylene response; EIN, ethylene insensitive; EIL, ethylene insensitive 3-like; ERF, ethylene response factor; GGPS, geranylgeranyl diphosphate synthase; PSY, phytoene synthase; PDS, phytoene desaturase; LCYβ, chloroplast-specific β-lycopene cyclase; CYCβ, chromoplast-specific β-lycopene cyclase.
The altered ethylene signaling output observed in hp2 fruits was associated with marked differences in the transcript abundance of genes involved in ethylene perception and signaling (Figure 4B and Supplementary Figure 5). ETHYLENE RESPONSE 3 (Sl-ETR3), one of the tomato ethylene receptor genes most highly expressed during ripening initiation (Liu M. et al., 2015), was strongly up-regulated in hp2 compared to the WT regardless of the light conditions. To a certain extent, a similar trend was also observed for some other ETR genes, including Sl-ETR4, Sl-ETR5 and Sl-ETR6.
The mRNA levels of ETHYLENE INSENSITIVE 2 (Sl-EIN2), which encodes a key component in the ethylene signaling cascade, was differentially affected by the hp2 mutation depending on the light conditions, being more greatly expressed in hp2 than in WT fruits in the dark and displaying the opposite pattern under light conditions (Figure 4B and Supplementary Figure 5). Transcript levels of both primary (ETHYLENE INSENSITIVE 3-LIKE, EIL) and secondary (ETHYLENE RESPONSE FACTOR, ERF) ethylene-related transcription factors were also altered in hp2 fruits compared to the WT. Both Sl-EIL2 and Sl-EIL3 were more abundantly expressed in hp2 than in the WT fruits whereas the opposite was observed for the Sl-ERF.E4, which encodes a repressor of tomato carotenogenesis (Lee et al., 2012). The additive effect of light treatment and loss of Sl-DET1/HP2 function was particularly observed for Sl-ETR3, Sl-ETR5, and Sl-EIN2 (Figure 4C).
All tomato ethylene receptor and signaling-related genes analyzed, except Sl-ETR3 and Sl-EIN2, displayed HY5 and PIF-binding motifs within their 3-kb promoter sequences (Supplementary Figure 6). Interestingly, Sl-EIN2 was the only ethylene-related gene that was differentially affected by the loss of Sl-DET1/HP2 function depending on the light conditions (Figure 4B).
To further investigate the relationship between ethylene responsiveness and carotenoid biosynthesis, carotenoid biosynthetic genes were profiled in both WT and hp2 fruits at MG stage exposed to a short-term (6h) treatment with exogenous ethylene (Figure 4D). All genes profiled, except for Sl-GGPS, were significantly up-regulated in WT fruits, thereby validating the efficacy of the ethylene treatment and confirming the positive influence of this hormone on the transcriptional regulation of the carotenoid pathway in tomato fruits. Comparatively, the ethylene-induced up-regulation of genes such as Sl-GGPS, Sl-PDS, and particularly Sl-LYCβ and Sl-CYCβ, was significantly more pronounced in hp2 than in the WT fruits, which corroborates the hypothesis that the increased responsiveness of hp2 fruits to ethylene may be associated with the overaccumulation of carotenoids in this mutant.
In concert with ethylene, auxin is also part of the regulatory network controlling tomato fruit ripening and carotenoid synthesis (Su et al., 2015). To evaluate whether the carotenoid overaccumulation and altered ethylene signaling observed in hp2 fruits are associated with changes in auxin levels and signaling, we next compared the endogenous IAA content, DR5 promoter activation and transcriptional profile of genes encoding auxin-related signaling elements in WT and hp2 ripening fruits.
Endogenous IAA levels were remarkably similar in WT and hp2 ripening fruits (Figure 5A). In contrast, the activity of the reporter protein GUS expressed under the control of the auxin-responsive DR5 promoter was considerably higher in either light or dark-incubated fruits of hp2-DR5::GUS compared to the DR5::GUS (Figure 5B). In both genotypes, a progressive reduction in auxin signaling output, as indicated by the DR5 promoter activation, was observed during fruit ripening. Auxin signaling output remained higher in hp2-DR5::GUS than in the DR5::GUS fruits from MG to Bk6 and from MG to Bk stage in dark- and light-incubated fruits, respectively.
FIGURE 5. Light-hypersensitivity promotes auxin sensitivity and signaling without altering endogenous IAA levels. Treatment details as described in Figure 1. (A) Endogenous indole-3-acetic acid (IAA) levels in wild-type (WT) and high pigment-2 (hp2) fruits. (B) In vitro GUS activity assayed in WT and hp2 fruits carrying the auxin-responsive promoter DR5 fused to the GUS reporter protein (DR5::GUS and hp2-DR5::GUS). (C) Heatmap representation of the differences in mRNA levels of auxin signaling genes between the WT and hp2 fruits ripened under light or dark conditions. (D) Heatmap representing differences in mRNA levels of auxin signaling genes between light and dark samples of hp2 fruits at each sampling time. The relative transcript values are presented in Supplementary Figures 7, 8. (E) Relative mRNA levels of auxin-responsive genes in WT and hp2 fruits treated with 100 μM IAA for 6 h. Data are means (±SE) of at least three biological replicates. Different letters indicate statistically significant differences (Tukey’s test, p < 0.05) within each genotype (in A,B) or among all data (in E). In (A,B), asterisks indicate statistically significant differences (Student’s t-test, p < 0.05) between genotypes. MG, mature green; Bk, Breaker; Aux/IAA, auxin/indole-3-acetic acid; ARF, auxin response factor.
As the higher auxin signaling output detected in hp2 fruits were not associated with marked differences in endogenous IAA content between the genotypes (Figures 5A,B), it seems plausible to suggest that hp2 fruits display increased sensitivity to this hormone compared to the WT. Corroborating these findings, the hp2 mutation was found to trigger marked changes in the transcriptional profile of genes encoding auxin-associated signaling proteins such as Aux/IAA and ARFs (Figure 5C).
Among the five Aux/IAA tomato genes closely associated with fruit ripening – i.e., Sl-IAA3, Sl-IAA4, Sl-IAA9, Sl-IAA15 and Sl-IAA27 (Audran-Delalande et al., 2012) – a dramatic reduction in Sl-IAA3, Sl-IAA4, Sl-IAA9 and Sl-IAA27 mRNA levels in hp2 compared to WT fruits was observed (Figure 5C and Supplementary Figure 7). Sl-IAA15 mRNA levels were also reduced in hp2 compared to the WT at certain ripening stages. Therefore, regardless of the light conditions, an overall down-regulation of Sl-IAA genes was observed in hp2 fruits compared to the WT. The repressor role of light in the expression of these Aux/IAA genes was supported by the additive effect of light treatment and loss of Sl-DET1/HP2 function on the mRNA levels of all Aux/IAA genes analyzed (Figure 5D).
The marked impact of loss of Sl-DET1/HP2 function on auxin signaling output and Aux/IAA mRNA levels, prompted us to investigate whether changes in light signaling cause significant alterations in the transcript abundance of seven ARF genes highly expressed in ripening tomato fruits, i.e., Sl-ARF2a, Sl-ARF2b, Sl-ARF3, Sl-ARF4, Sl-ARF5, Sl-ARF8a and Sl-ARF8b. Data showed that transcript levels of Sl-ARF2a and Sl-ARF2b, which are considered key convergence points of auxin and ethylene signaling and important promoters of tomato fruit ripening and carotenoid biosynthesis (Hao et al., 2015; Breitel et al., 2016), were higher in hp2 than in WT fruits (Figure 5C and Supplementary Figure 8). Similarly, mRNA levels of Sl-ARF8b, a known activator of auxin-dependent gene transcription (Kumar et al., 2014), were considerably higher in hp2 than in WT fruits. Conversely, transcript abundance of Sl-ARF3, a well-established repressor of auxin-dependent gene transcription (Zouine et al., 2014), was dramatically reduced in hp2 than in WT fruits regardless of the light treatment (Figure 5C). An overall reduction in Sl-ARF8a mRNA levels was also detected in hp2 fruits compared to the WT, particularly under dark conditions. In contrast, the impacts of the loss of Sl-DET1/HP2 function on Sl-ARF4 and Sl-ARF5 mRNA levels were considerably more variable as these genes were either up- or down-regulated in hp2 compared to the WT depending on the ripening stage considered (Supplementary Table 2, i.e., non-significant influence of the genotype and the genotype × light treatment interaction). The combined effect of light exposure and the hp2 mutation was clearly observed for all tomato ARF genes analyzed (Figure 5D). In summary, among all light-triggered alterations in the transcriptional profile of Sl-ARF genes, Sl-ARF3 mRNA levels were down-regulated in response to both light exposure and the loss of Sl-DET1/HP2 function, with the opposite being observed for Sl-ARF2a, Sl-ARF2b, and Sl-ARF8b.
Finally, the relationship between light and auxin responsiveness in hp2 was also investigated by comparing the impacts of auxin treatment on the transcript abundance of the auxin-responsive genes Sl-GH3, Sl-IAA4, Sl-IAA9 and Sl-IAA27 (Figure 5E). Although Sl-GH3 was clearly up-regulated in both WT and hp2 fruits, the auxin-triggered accumulation of transcripts of this gene was significantly higher in the mutant, which further indicates increased auxin sensitivity in hp2 compared to WT fruits. Auxin treatment promoted Sl-IAA4, Sl-IAA9 and Sl-IAA27 transcript accumulation in WT fruits but failed to alter the expression of these genes in hp2 fruits (Figure 5E). These results are in line with the detection of lower Sl-IAA mRNA levels in the hp2 compared to the WT, although both genotypes displayed similar endogenous IAA levels throughout the ripening phase (Figures 5A,C).
Assumptions that light-hormonal crosstalk may be involved in controlling tomato fruit ripening and carotenoid metabolism have been formulated for a long time in the literature (Lieberman, 1979; Yang and Hoffman, 1984), while unequivocal genetic or physiological evidence supporting this hypothesis remained lacking. As a major regulator of numerous ripening-associated processes, ethylene was one of the first hormones investigated as part of the regulatory mechanisms behind the light-dependent regulation of fruit carotenoid biosynthesis (Alba et al., 2000).
In vegetative tissues, ethylene biosynthesis is highly regulated by light quality, intensity and duration. Overall, light perception via photoreceptors, such as PHYs and CRYs, inhibits ethylene emission (Corbineau et al., 1995; Vandenbussche et al., 2003; Pierik, 2004; Giliberto et al., 2005; Melo et al., 2016), ACC accumulation (Jiao et al., 1987; Melo et al., 2016), ACO activity (Melo et al., 2016) and ACS transcript levels (Khanna et al., 2007). Our data revealed that the negative influence of light on ethylene metabolism typically found in vegetative tissues is also observed in ripening tomato fruits as indicated by the light-triggered reduction in ACC content, ACO activity and ethylene emission, a response that was further intensified in fruits of the light-hyperresponsive hp2 mutant. The main ACS and ACO family members expressed during ripening were either up- or down-regulated in response to light exposure or the loss of Sl-DET1/HP2 function, suggesting that light-dependent down-regulation of the ethylene climacteric burst in tomato is linked to complex alterations in the transcript abundance of its biosynthetic genes. These findings contrast with the observation that PHY-mediated light perception in plant vegetative tissues is frequently associated with the modulation of ethylene biosynthesis via conspicuous changes in the ACS transcription (Rodrigues et al., 2014), as illustrated by the several 100-fold enhancements in AtACS4 transcript abundance detected in Arabidopsis seedlings overexpressing AtPIF5 (Khanna et al., 2007).
Ethylene biosynthesis in tomato fruits is tightly regulated by master regulators of ripening, stimulated by Sl-RIN, Sl-NOR, Sl-FUL, and Sl-TAGL1 (Itkin et al., 2009; Liu M. et al., 2015) and repressed by Sl-AP2a (Karlova et al., 2011). The up-regulation of all these ripening master regulators in hp2 ripening fruits entails a complex interaction between the light signaling cascade and the regulatory cascade controlling ripening. Sl-AP2a acts as a negative regulator of tomato climacteric ethylene synthesis via a negative feedback loop (Karlova et al., 2011); therefore, the reduced ethylene production detected in hp2 fruits may be associated with the up-regulation of Sl-AP2a in this mutant (Figure 6). In contrast, all ripening master regulators analyzed are well-established promoters of fruit carotenoid biosynthesis (Itkin et al., 2009; Martel et al., 2011; Liu L. et al., 2015); hence, their up-regulation in hp2 ripening fruits is consistent with the over-accumulation of carotenoids in the mutant.
FIGURE 6. Proposed model for light-hormonal interaction controlling tomato fruit carotenoid biosynthesis. Light signaling promotes master regulators of ripening, which positively regulate carotenoid biosynthetic genes. Light also down-regulates ethylene metabolism and emission and reduces the expression of ETHYLENE RESPONSE FACTOR E4 (Sl-ERF.E4), a key repressor of tomato fruit carotenogenesis. Moreover, light represses AUXIN/INDOLE-3-ACETIC ACID (Sl-IAAs) genes and up-regulates both AUXIN RESPONSE FACTOR 2 paralogues (SlARF2a/b), consequently promoting most components of the tomato carotenoid biosynthetic route. Additionally, light signaling up-regulates genes encoding ethylene receptor (ETRs) and intermediate components in ethylene signaling cascade (EILs). The arrow- and bar-headed lines indicate stimulatory and inhibitory effects, respectively. The lines terminated by a circle describe more complex interactions. RIN, ripening inhibitor; NOR, non-ripening; FUL, fruitfull; AP2a, apetala2a, TAGL1, tomato agamous-like1; ETR, ethylene response; EIL, ethylene insensitive 3-like.
Besides altering ethylene biosynthesis, the loss of Sl-DET1/HP2 function also impacted tomato fruit responsiveness to ethylene, a response associated with marked changes in ethylene receptors (ETRs) and downstream signaling transduction elements (EIN, EILs, ERFs). The receptor signaling model states that ETRs, including those involved in tomato ripening (i.e., Sl-ETR3 and Sl-ETR4), are negative regulators of ethylene responses (Kevany et al., 2007; Kamiyoshihara et al., 2012); consequently, reductions in the abundance of receptors promote tissue ethylene sensitivity (Tieman et al., 2000). However, information about the temporal fluctuations in ETR transcripts and protein levels during tomato ripening is controversial. Opposite temporal patterns between the mRNA and protein levels of Sl-ETR3 and Sl-ETR4 have been observed during tomato ripening, as the protein and transcript abundance of these receptors peak at the immature and ripening stages, respectively (Kevany et al., 2007). However, no significant alterations in ethylene receptor protein abundance were observed during tomato fruit ripening in a subsequent study (Kamiyoshihara et al., 2012). Therefore, on the one hand, the apparent contradiction between the up-regulation of ETR transcripts and the increased ethylene sensitivity detected in hp2 fruits may be explained by the inverse pattern in ethylene receptor mRNA and protein levels already observed in tomato fruits (Kevany et al., 2007). On the other hand, if the hp2-triggered up-regulation of Sl-ETR expression results in increased receptor protein abundance, the increased ethylene sensitivity observed in the fruits of these mutants may be associated with a more complex alteration in ethylene perception and signaling cascade.
Acting downstream to ETR receptors, EIN2 plays a significant role in ethylene signaling by stabilizing EIN3/EIL transcription factors, which in turn will activate the transcription of multiple ethylene-responsive genes, including secondary transcription factors (i.e., ERFs) (Alonso, 1999). Many of these downstream signaling transduction elements are involved in photomorphogenic responses, sometimes acting as integrators of light and ethylene signaling during vegetative plant development (Zhong et al., 2009). In tomato, Sl-EIN2, Sl-EIL or Sl-ERF.E4 suppression disturbed fruit ripening and, consequently, altered carotenoid metabolism (Tieman et al., 2001; Fu et al., 2005; Lee et al., 2012). As these genes were differentially expressed in hp2 fruits compared to the WT, it seems that disturbances in light signaling can affect multiple steps in the ethylene transduction cascade, which may contribute to explain the altered ethylene responsiveness detected in this mutant. In this context, it is also worth mentioning that Sl-ERF.E4 mRNA levels were severely reduced in hp2 fruits and this ERF has been proposed as a major repressor of carotenoid synthesis in tomato, as revealed by the over-accumulation of this class of isoprenoid in fruits of Sl-ERF.E4-knockdown transgenic lines (Lee et al., 2012). Therefore, it seems that the increased ethylene responsiveness of hp2 fruits may compensate for its reduced ethylene biosynthesis, which is supported by the comparatively higher expression of carotenoid biosynthetic genes in the mutant when both WT and hp2 fruits were supplemented with the same concentration of ethylene.
Tomato fruit carotenogenesis is undeniably regulated by ethylene-related signaling components, but other plant hormones have also been increasingly implicated in controlling this metabolic pathway (Kumar et al., 2014; Liu L. et al., 2015). Auxins, for instance, have been demonstrated to counteract the promotive influence of ethylene on tomato fruit ripening and carotenogenesis (Pirrello et al., 2012; Su et al., 2015). Here, we provide several lines of evidence indicating that the loss of Sl-DET1/HP2 function promotes auxin responsiveness in fruit tissues via changes in the transcript abundance of auxin signaling-related genes. The increased activation of DR5 promoter in hp2 fruits was not associated with significant differences in the endogenous IAA levels between the mutant and WT genotypes but instead was accompanied by the down-regulation of the Sl-IAA genes most greatly expressed in tomato fruits (i.e., Sl-IAA3, Sl-IAA4, Sl-IAA9, and Sl-IAA27).
Accordingly, functional characterization studies have revealed that the down-regulation of Sl-IAA3, Sl-IAA9 or Sl-IAA27 disturbs auxin responsiveness in tomato plants. Whereas Sl-IAA3 knockdown resulted in lower auxin sensitivity, Sl-IAA9- or Sl-IAA27-silenced lines exhibited increased auxin responsiveness (Wang et al., 2005; Chaabouni et al., 2009; Bassa et al., 2012). Therefore, the progressive reduction in DR5 promoter activity observed from the MG to Bk12 stages in both dark- and light-incubated fruits may be linked to the gradual increment in transcripts of the repressor of auxin responsiveness Sl-IAA3 (Chaabouni et al., 2009), and the progressive reduction in transcripts of Sl-IAA9 and Sl-IAA27, two positive regulators of tissue responsivity to auxins (Wang et al., 2005; Bassa et al., 2012). Among these tomato Aux/IAA genes, Sl-IAA3 has been suggested to represent a crossroad of auxin and ethylene signaling in tomato, being highly regulated by both these hormones. Recent findings also indicate that Sl-IAA3 mediates the interplay between light and ethylene signaling, since dark- and light-grown Sl-IAA3-knockdown tomato seedlings exhibited marked differences in ethylene sensitivity (Chaabouni et al., 2009) and this tomato Aux/IAA gene was particularly up-regulated in ripening fruits of PHY-deficient tomato plants (Bianchetti et al., 2017). Therefore, it seems tempting to speculate that the light-dependent down-regulation of Sl-IAA3 may be associated with the increased responsivity to ethylene observed in hp2 fruits.
Aux/IAA proteins are known to inhibit the activity of ARF, and ARFs can either act as transcriptional repressors or activators of auxin-responsive genes (Zouine et al., 2014). Hence, changes in ARF abundance also significantly impact plant tissue responsiveness to auxins (Sagar et al., 2013; Zouine et al., 2014; Hao et al., 2015). Accordingly, the increased auxin responsiveness observed in hp2 fruits was associated with a marked down- and up-regulation of Sl-ARF3 and Sl-ARF8b, respectively, as the former is a repressor of auxin-dependent gene transcription whereas the latter is an activator of auxin responses. In both cases, the impact of the hp2 mutation was intensified by light exposure, which suggests that the light-dependent transcriptional regulation of these two ARFs may be associated with the increased auxin responsiveness observed in the hp2 fruits.
The up-regulation of Sl-ARF2a and Sl-ARF2b caused by the loss of Sl-DET1/HP2 function is also consistent with the proposed role suggested for these two ARFs on tomato fruit ripening and carotenogenesis (Hao et al., 2015; Breitel et al., 2016). Both Sl-ARF2 paralogs are known to cooperate in promoting the expression of master controllers of ripening, such as Sl-RIN and Sl-NOR, stimulating ethylene biosynthesis and signaling and inducing carotenoid biosynthesis (Hao et al., 2015; Breitel et al., 2016). Therefore, the up-regulation of both Sl-ARF2a and Sl-ARF2b genes observed in light-incubated hp2 fruits agrees with the higher expression of genes encoding master controllers of ripening and carotenoid biosynthetic enzymes detected in this light-hyperresponsive mutant.
Here, we put forward the hypothesis that light-triggered changes in auxin and ethylene responsiveness and signaling are associated with the overaccumulation of carotenoids in hp2 fruits. In the proposed working model of light-hormonal crosstalk controlling tomato carotenogenesis (Figure 6), the positive and negative influence of light on ethylene biosynthesis and signaling, respectively, are supported by both genetic (i.e., hp2 mutation versus WT genotype) and physiological evidence (i.e., light versus dark treatment). The assumption that light modulates auxin signaling is corroborated by the marked down-regulation of Aux/IAA tomato genes and altered ARF expression profile in hp2 fruits compared to the WT. The two ARF genes most closely associated with tomato fruit ripening and carotenogenesis (i.e., Sl-ARF2a and Sl-ARF2b) and the genes encoding the master regulators of ripening (e.g., Sl-RIN, Sl-NOR, Sl-FUL1, Sl-AP2a) were up-regulated, whereas Sl-ERF.E4, a repressor of tomato fruit carotenogenesis, was repressed in hp2 fruits compared to WT counterparts. All these changes in the central ripening-related regulatory modules are consistent with the increased transcript abundance of carotenoid biosynthetic genes (e.g., Sl-GGPS, Sl-PSY1, Sl-PDS, Sl-LYCβ and Sl-LYCβ) and the over-accumulation of carotenoids typically observed in the hp2 mutant.
Although DET1 has long been identified as a major repressor of light signaling in plants (Chory et al., 1989), the molecular mechanisms responsible for its action on photomorphogenesis remain not yet fully characterized. However, accumulating evidence indicates that DET1 may interfere with multiple steps of the light signaling cascades. In Arabidopsis, DET1 interacts with DDB1 and COP10 to form the CDD complex, which physically associates with CUL4, giving rise to an E3 ligase that promotes the proteolytic degradation of photomorphogenesis-promoting factors, including HY5 (Yanagawa et al., 2004). DET1 has also been shown to positively and negatively regulate the accumulation of PIF and DELLA proteins, respectively (Dong et al., 2014; Li et al., 2015), which partially explains how DET1 represses Arabidopsis photomorphogenesis in darkness. Data also implicates DET1 action in chromatin remodeling (Benvenuto et al., 2002) and as a transcriptional co-repressor of key regulators of the circadian clock genes (Lau et al., 2011). Therefore, multiple mechanisms may be involved in the Sl-DET1/HP2-mediated regulation of ethylene and auxin pathways in ripening tomato fruits, including its influence on balancing HY5 and PIF protein abundance, possible global alterations in gene expression via chromatin remodeling, and its potential action as a transcriptional co-regulator. Hence, future work is needed to characterize the precise molecular mechanisms behind the Sl-DET1/HP2-mediated regulation of tomato fruit hormonal balance and physiology.
Although the exact mechanisms behind the light-triggered alterations in fruit hormone responsiveness are not yet clear, the data obtained in this study provide clear evidence that an intricate crosstalk between light, ethylene and auxin signaling may be involved in controlling tomato fruit carotenogenesis. Therefore, these findings open up a window of opportunity for further improvement in tomato fruit nutritional content through the combined manipulation of auxin, ethylene and light signaling-related genes.
LF, LP, MR, and AC designed the experiments. AC, RB, FA, and EP conducted the experiments and analyzed the results. AC, RB, and LF prepared the manuscript.
This work was supported by the CNPq (Conselho Nacional de Desenvolvimento Científico e Tecnológico, Grant No. 442045/2014-0) and the FAPESP (Fundação de Amparo à Pesquisa do Estado de São Paulo, Grant Nos. 2013/18056-2 and 2016/04924-0).
The authors declare that the research was conducted in the absence of any commercial or financial relationships that could be construed as a potential conflict of interest.
The Supplementary Material for this article can be found online at: https://www.frontiersin.org/articles/10.3389/fpls.2018.01370/full#supplementary-material
Alba, R., Cordonnier-Pratt, M.-M., and Pratt, L. H. (2000). Fruit-localized phytochromes regulate lycopene accumulation independently of ethylene production in tomato. Plant Physiol. 123, 363–370. doi: 10.1104/pp.123.1.363
Alonso, J. M. (1999). EIN2, a bifunctional transducer of ethylene and stress responses in Arabidopsis. Science 284, 2148–2152. doi: 10.1126/science.284.5423.2148
Audran-Delalande, C., Bassa, C., Mila, I., Regad, F., Zouine, M., and Bouzayen, M. (2012). Genome-wide identification, functional analysis and expression profiling of the Aux/IAA gene family in tomato. Plant Cell Physiol. 53, 659–672. doi: 10.1093/pcp/pcs022
Azari, R., Reuveni, M., Evenor, D., Nahon, S., Shlomo, H., Chen, L., et al. (2010a). Overexpression of UV-Damaged DNA binding protein 1 links plant development and phytonutrient accumulation in high pigment-1 tomato. J. Exp. Bot. 61, 3627–3637. doi: 10.1093/jxb/erq176
Azari, R., Tadmor, Y., Meir, A., Reuveni, M., Evenor, D., Nahon, S., et al. (2010b). Light signaling genes and their manipulation towards modulation of phytonutrient content in tomato fruits. Biotechnol. Adv. 28, 108–118. doi: 10.1016/j.biotechadv.2009.10.003
Barry, C. S., and Giovannoni, J. J. (2007). Ethylene and fruit ripening. J. Plant Growth Regul. 26, 143–159. doi: 10.1007/s00344-007-9002-y
Bassa, C., Mila, I., Bouzayen, M., and Audran-Delalande, C. (2012). Phenotypes associated with down-regulation of Sl-IAA27 support functional diversity among Aux/IAA family members in tomato. Plant Cell Physiol. 53, 1583–1595. doi: 10.1093/pcp/pcs101
Benvenuto, G., Formiggini, F., Laflamme, P., Malakhov, M., and Bowler, C. (2002). The photomorphogenesis regulator DET1 binds the amino-terminal tail of histone H2B in a nucleosome context. Curr. Biol. 12, 1529–1534. doi: 10.1016/S0960-9822(02)01105-3
Bianchetti, R. E., Cruz, A. B., Oliveira, B. S., Demarco, D., Purgatto, E., Peres, L. E. P., et al. (2017). Phytochromobilin deficiency impairs sugar metabolism through the regulation of cytokinin and auxin signaling in tomato fruits. Sci. Rep. 7:7822. doi: 10.1038/s41598-017-08448-2
Bianchetti, R. E., Lira, B. S., Moneiro, S. S., DeMarco, D., Purgatto, E., Rossi, M., et al. (2018). Fruit-localized phytochromes regulate plastid biogenesis, starch synthesis and carotenoid metabolism in tomato. J. Exp. Bot. 69, 3573–3586. doi: 10.1093/jxb/ery145
Breitel, D. A., Chappell-Maor, L., Meir, S., Panizel, I., Puig, C. P., Hao, Y., et al. (2016). AUXIN RESPONSE FACTOR 2 intersects hormonal signals in the regulation of tomato fruit ripening. PLoS Genet. 12:e1005903. doi: 10.1371/journal.pgen.1005903
Bulens, I., Van de Poel, B., Hertog, M. L., De Proft, M. P., Geeraerd, A. H., and Nicolaï, B. M. (2011). Protocol: an updated integrated methodology for analysis of metabolites and enzyme activities of ethylene biosynthesis. Plant Methods 7:17. doi: 10.1186/1746-4811-7-17
Carvalho, R. F., Campos, M. L., Pino, L. E., Crestana, S. L., Zsögön, A., Lima, J. E., et al. (2011). Convergence of developmental mutants into a single tomato model system: “Micro-Tom” as an effective toolkit for plant development research. Plant Methods 7:18. doi: 10.1186/1746-4811-7-18
Chaabouni, S., Jones, B., Delalande, C., Wang, H., Li, Z., Mila, I., et al. (2009). Sl-IAA3, a tomato Aux/IAA at the crossroads of auxin and ethylene signalling involved in differential growth. J. Exp. Bot. 60, 1349–1362. doi: 10.1093/jxb/erp009
Chory, J., Peto, C., Feinbaum, R., Pratt, L., and Ausubel, F. (1989). Arabidopsis thaliana mutant that develops as a light-grown plant in the absence of light. Cell 58, 991–999. doi: 10.1016/0092-8674(89)90950-1
Chow, C. N., Zheng, H. Q., Wu, N. Y., Chien, C. H., Huang, H., Da Lee, T. Y., et al. (2016). PlantPAN 2.0: an update of plant promoter analysis navigator for reconstructing transcriptional regulatory networks in plants. Nucleic Acids Res. 44, D1154–D1164. doi: 10.1093/nar/gkv1035
Corbineau, F., Rudnicki, R. M., Goszczynska, D. M., and Come, D. (1995). The effect of light quality on ethylene production in leaves of oat seedlings (Avena sativa L.). Environ. Exp. Bot. 35, 227–233. doi: 10.1016/0098-8472(94)00052-7
Davuluri, G. R., van Tuinen, A., Fraser, P. D., Manfredonia, A., Newman, R., Burgess, D., et al. (2005). Fruit-specific RNAi-mediated suppression of DET1 enhances carotenoid and flavonoid content in tomatoes. Nat. Biotechnol. 23, 890–895. doi: 10.1038/nbt1108
Davuluri, G. R., Van Tuinen, A., Mustilli, A. C., Manfredonia, A., Newman, R., Burgess, D., et al. (2004). Manipulation of DET1 expression in tomato results in photomorphogenic phenotypes caused by post-transcriptional gene silencing. Plant J. 40, 344–354. doi: 10.1111/j.1365-313X.2004.02218.x
Dong, J., Tang, D., Gao, Z., Yu, R., Li, K., He, H., et al. (2014). Arabidopsis DE-ETIOLATED1 represses photomorphogenesis by positively regulating phytochrome-interacting factors in the dark. Plant Cell 26, 3630–3645. doi: 10.1105/tpc.114.130666
Ecarnot, M., Baogonekczyk, P., Tessarotto, L., and Chervin, C. (2013). Rapid phenotyping of the tomato fruit model, Micro-Tom, withaportable VIS-NIR spectrometer. Plant Physiol. Biochem. 70, 159–163. doi: 10.1016/j.plaphy.2013.05.019
Enfissi, E. M., Barneche, F., Ahmed, I., Lichtlé, C., Gerrish, C., McQuinn, R. P., et al. (2010). Integrative transcript and metabolite analysis of nutritionally enhanced DE-ETIOLATED1 downregulated tomato fruit. Plant Cell 22, 1190–1215. doi: 10.1105/tpc.110.073866
Expósito-Rodríguez, M., Borges, A. A., Borges-Pérez, A., and Pérez, J. A. (2008). Selection of internal control genes for quantitative real-time RT-PCR studies during tomato development process. BMC Plant Biol. 8:131. doi: 10.1186/1471-2229-8-131
Fu, D. Q., Zhu, B. Z., Zhu, H. L., Jiang, W.-B., and Luo, Y.-B. (2005). Virus-induced gene silencing in tomato fruit. Plant J. 43, 299–308. doi: 10.1111/j.1365-313X.2005.02441.x
Giliberto, L., Perrotta, G., Pallara, P., Weller, J. L., Fraser, P. D., Bramley, P. M., et al. (2005). Manipulation of the blue light photoreceptor cryptochrome 2 in tomato affects vegetative development, flowering time, and fruit antioxidant content. Plant Physiol. 137, 199–208. doi: 10.1104/pp.104.051987
Giovannoni, J. J. (2004). Genetic regulation of fruit development and ripening. Plant Cell 16, 170–181. doi: 10.1105/tpc.019158
Hao, Y., Hu, G., Breitel, D., Liu, M., Mila, I., Frasse, P., et al. (2015). Auxin Response Factor SlARF2 is an essential component of the regulatory mechanism controlling fruit ripening in tomato. PLoS Genet. 11:e1005649. doi: 10.1371/journal.pgen.1005649
Itkin, M., Seybold, H., Breitel, D., Rogachev, I., Meir, S., and Aharoni, A. (2009). TOMATO AGAMOUS-LIKE 1 is a component of the fruit ripening regulatory network. Plant J. 60, 1081–1095. doi: 10.1111/j.1365-313X.2009.04064.x
Jiao, X. Z., Yip, W. K., and Yang, S. F. (1987). The effect of light and phytochrome on 1-aminocyclopropane-1-carboxylic acid metabolism in etiolated wheat seedling leaves. Plant Physiol. 85, 643–647. doi: 10.1104/pp.85.3.643
Kamiyoshihara, Y., Tieman, D. M., Huber, D. J., and Klee, H. J. (2012). Ligand-induced alterations in the phosphorylation state of ethylene receptors in tomato fruit. Plant Physiol. 160, 488–497. doi: 10.1104/pp.112.202820
Karlova, R., Chapman, N., David, K., Angenent, G. C., Seymour, G. B., and De Maagd, R. A. (2014). Transcriptional control of fleshy fruit development and ripening. J. Exp. Bot. 65, 4527–4541. doi: 10.1093/jxb/eru316
Karlova, R., Rosin, F. M., Busscher-Lange, J., Parapunova, V., Do, P. T., Fernie, A. R., et al. (2011). Transcriptome and metabolite profiling show that APETALA2a is a major regulator of tomato fruit ripening. Plant Cell 23, 923–941. doi: 10.1105/tpc.110.081273
Kevany, B. M., Tieman, D. M., Taylor, M. G., Cin, V. D., and Klee, H. J. (2007). Ethylene receptor degradation controls the timing of ripening in tomato fruit. Plant J. 51, 458–467. doi: 10.1111/j.1365-313X.2007.03170
Khanna, R., Shen, Y., Marion, C. M., Tsuchisaka, A., Theologis, A., Schafer, E., et al. (2007). The Basic Helix-Loop-Helix Transcription Factor PIF5 acts on ethylene biosynthesis and phytochrome signaling by distinct mechanisms. Plant Cell 19, 3915–3929. doi: 10.1105/tpc.107.051508
Kolotilin, I., Koltai, H., Tadmor, Y., Bar-Or, C., Reuveni, M., Meir, A., et al. (2007). Transcriptional profiling of high pigment-2dg tomato mutant links early fruit plastid biogenesis with its overproduction of phytonutrients. Plant Physiol. 145, 389–401. doi: 10.1104/pp.107.102962
Kumar, R., Khurana, A., and Sharma, A. K. (2014). Role of plant hormones and their interplay in development and ripening of fleshy fruits. J. Exp. Bot. 65, 4561–4575. doi: 10.1093/jxb/eru277
Lau, O. S., Huang, X., Charron, J.-B., Lee, J.-H., Li, G., and Deng Xing, W. (2011). Interaction of Arabidopsis DET1 with CCA1 and LHY in mediating transcriptional repression in the plant circadian clock. Mol. Cell 43, 703–712. doi: 10.1016/j.molcel.2011.07.013
Lee, J. M., Joung, J. G., McQuinn, R., Chung, M. Y., Fei, Z., Tieman, D., et al. (2012). Combined transcriptome, genetic diversity and metabolite profiling in tomato fruit reveals that the ethylene response factor SlERF6 plays an important role in ripening and carotenoid accumulation. Plant J. 70, 191–204. doi: 10.1111/j.1365-313X.2011.04863.x
Levin, I., de Vos, C., Tadmor, Y., Bovy, A., Lieberman, M., Oren-Shamir, M., et al. (2006). High pigment tomato mutants—more than just lycopene (a review). Isr. J. Plant Sci. 54, 179–190. doi: 10.1560/IJPS_54_3_179
Levin, I., Frankel, P., Gilboa, N., Tanny, S., and Lalazar, A. (2003). The tomato dark green mutation is a novel allele of the tomato homolog of the deetiolated1 gene. Theor. Appl. Genet. 106, 454–460. doi: 10.1007/s00122-002-1080-4
Li, K., Gao, Z., He, H., Terzaghi, W., Fan, L.-M., Deng Xing, W., et al. (2015). Arabidopsis DET1 represses photomorphogenesis in part by negatively regulating della protein abundance in darkness. Mol. Plant 8, 622–630. doi: 10.1016/j.molp.2014.12.017
Lieberman, M. (1979). Biosynthesis and action of ethylene. Annu. Rev. Plant Physiol. 30, 533–591. doi: 10.1146/annurev.pp.30.060179.002533
Lieberman, M., Segev, O., Gilboa, N., Lalazar, A., and Levin, I. (2004). The tomato homolog of the gene encoding UV-damaged DNA binding protein 1 (DDB1) underlined as the gene that causes the high pigment-1 mutant phenotype. Theor. Appl. Genet. 108, 1574–1581. doi: 10.1007/s00122-004-1584-1
Liu, C. C., Ahammed, G. J., Wang, G. T., Xu, C. J., Chen, K. S., Zhou, Y. H., et al. (2018). Tomato CRY1a plays a critical role in the regulation of phytohormone homeostasis, plant development, and carotenoid metabolism in fruits. Plant Cell Environ. 41, 354–366. doi: 10.1111/pce.13092
Liu, L., Shao, Z., Zhang, M., and Wang, Q. (2015). Regulation of carotenoid metabolism in tomato. Mol. Plant 8, 28–39. doi: 10.1016/j.molp.2014.11.006
Liu, M., Pirrello, J., Chervin, C., Roustan, J.-P., and Bouzayen, M. (2015). Ethylene control of fruit ripening: revisiting the complex network of transcriptional regulation. Plant Physiol. 169, 2380–2390. doi: 10.1104/pp.15.01361
Liu, N., Wu, S., Houten, J., Van Wang, Y., Ding, B., Fei, Z., et al. (2014). Down-regulation of AUXIN RESPONSE FACTORS 6 and 8 by microRNA 167 leads to floral development defects and female sterility in tomato. J. Exp. Bot. 65, 2507–2520. doi: 10.1093/jxb/eru141
Liu, Y., Roof, S., Ye, Z., Barry, C., Van Tuinent, A., Vrebalov, J., et al. (2004). Manipulation of light signal transduction as a means of modifying fruit nutritional quality in tomato. Proc. Natl. Acad. Sci. U.S.A. 101, 9897–9902. doi: 10.1073/pnas.0400935101
Llorente, B., D’Andrea, L., and Rodríguez-Concepción, M. (2016a). Evolutionary recycling of light signaling components in fleshy fruits: new insights on the role of pigments to monitor ripening. Front. Plant Sci. 7:263. doi: 10.3389/fpls.2016.00263
Llorente, B., D’Andrea, L., Ruiz-Sola, M. A., Botterweg, E., Pulido, P., Andilla, J., et al. (2016b). Tomato fruit carotenoid biosynthesis is adjusted to actual ripening progression by a light-dependent mechanism. Plant J. 85, 107–119. doi: 10.1111/tpj.13094
Martel, C., Vrebalov, J., Tafelmeyer, P., and Giovannoni, J. J. (2011). The tomato MADS-Box transcription factor RIPENING INHIBITOR interacts with promoters involved in numerous ripening processes in a colorless nonripening-dependent manner. Plant Physiol. 157, 1568–1579. doi: 10.1104/pp.111.181107
Martínez-García, J. F., Huq, E., and Quail, P. H. (2000). Direct targeting of light signals to a promoter element-bound transcription factor. Science 288, 859–863. doi: 10.1126/science.288.5467.859
Melo, N. K. G., Bianchetti, R. E., Lira, B. S., Oliveira, P. M. R., Zuccarelli, R., Dias, D. L. O., et al. (2016). Nitric oxide, ethylene, and auxin cross talk mediates greening and plastid development in deetiolating tomato seedlings. Plant Physiol. 170, 2278–2294. doi: 10.1104/pp.16.00023
Mustilli, A. C., Fenzi, F., Ciliento, R., Alfano, F., and Bowler, C. (1999). Phenotype of the tomato high pigment-2 mutant is caused by a mutation in the tomato homolog of DEETIOLATED1. Plant Cell 11, 145–157. doi: 10.1105/tpc.11.2.145
Pech, J. C., Purgatto, E., Bouzayen, M., and Latché, A. (2012). Ethylene and fruit ripening. Annu. Plant Rev. 44, 275–304. doi: 10.1002/9781118223086.ch11
Pierik, R. (2004). Interactions between ethylene and gibberellins in phytochrome-mediated shade avoidance responses in tobacco. Plant Physiol. 136, 2928–2936. doi: 10.1104/pp.104.045120
Piringer, A. A., and Heinze, P. H. (1954). Effect of light on the formation of a pigment in the tomato fruit cuticle. Plant Physiol. 29, 467–472. doi: 10.1104/pp.29.5.467
Pirrello, J., Prasad, N., Zhang, W., Chen, K., Mila, I., Zouine, M., et al. (2012). Functional analysis and binding affinity of tomato ethylene response factors provide insight on the molecular bases of plant differential responses to ethylene. BMC Plant Biol. 12:190. doi: 10.1186/1471-2229-12-190
Rodrigues, M. A., Bianchetti, R. E., and Freschi, L. (2014). Shedding light on ethylene metabolism in higher plants. Front. Plant Sci. 5:665. doi: 10.3389/fpls.2014.00665
Sagar, M., Chervin, C., Mila, I., Hao, Y., Roustan, J.-P., Benichou, M., et al. (2013). SlARF4, an auxin response factor involved in the control of sugar metabolism during tomato fruit development. Plant Physiol. 161, 1362–1374. doi: 10.1104/pp.113.213843
Santana-Vieira, D. D., Freschi, L., Almeida, L. A., Moraes, D. H., Neves, D. M., Santos, L. M., et al. (2016). Survival strategies of citrus rootstocks subjected to drought. Sci. Rep. 6, 1–12. doi: 10.1038/srep38775
Schofield, A., and Paliyath, G. (2005). Modulation of carotenoid biosynthesis during tomato fruit ripening through phytochrome regulation of phytoene synthase activity. Plant Physiol. Biochem. 43, 1052–1060. doi: 10.1016/j.plaphy.2005.10.006
Schroeder, D. F., Gahrtz, M., Maxwell, B. B., Cook, R. K., Kan, J. M., Alonso, J. M., et al. (2002). De-Etiolated 1 and damaged DNA binding protein 1 interact to regulate Arabidopsis photomorphogenesis. Curr. Biol. 12, 1462–1472. doi: 10.1016/S0960-9822(02)01106-5
Sestari, I., Zsögön, A., Rehder, G. G., de Lira Teixeira, L., Hassimotto, N. M. A., Purgatto, E., et al. (2014). Near-isogenic lines enhancing ascorbic acid, anthocyanin and carotenoid content in tomato (Solanum lycopersicum L. cv Micro-Tom) as a tool to produce nutrient-rich fruits. Sci. Hortic. 175, 111–120. doi: 10.1016/j.scienta.2014.06.010
Song, Y. H., Yoo, C. M., Hong, A. P., Kim, S. H., Jeong, H. J., Shin, S. Y., et al. (2008). DNA-binding study identifies C-box and hybrid C/G-box or C/A-box motifs as high-affinity binding sites for STF1 and LONG HYPOCOTYL5 proteins. Plant Physiol. 146, 1862–1877. doi: 10.1104/pp.107.113217
Sorrequieta, A., Abriata, L. A., Boggio, S. B., and Valle, E. M. (2013). Off-the-vine ripening of tomato fruit causes alteration in the primary metabolite composition. Metabolites 3, 967–978. doi: 10.3390/metabo3040967
Su, L., Diretto, G., Purgatto, E., Danoun, S., Zouine, M., Li, Z., et al. (2015). Carotenoid accumulation during tomato fruit ripening is modulated by the auxin-ethylene balance. BMC Plant Biol. 15:114. doi: 10.1186/s12870-015-0495-4
Tieman, D. M., Ciardi, J. A., Taylor, M. G., and Klee, H. J. (2001). Members of the tomato LeEIL (EIN3-like) gene family are functionally redundant and regulate ethylene responses throughout plant development. Plant J. 26, 47–58. doi: 10.1046/j.1365-313X.2001.01006.x
Tieman, D. M., Taylor, M. G., Ciardi, J. A., and Klee, H. J. (2000). The tomato ethylene receptors NR and LeETR4 are negative regulators of ethylene response and exhibit functional compensation within a multigene family. Proc. Natl. Acad. Sci. U.S.A. 97, 5663–5668. doi: 10.1073/pnas.090550597
Vandenbussche, F., Vriezen, W. H., Smalle, J., Laarhoven, L. J. J., Harren, F. J. M., and Van Der Straeten, D. (2003). Ethylene and auxin control the Arabidopsis response to decreased light intensity. Plant Physiol. 133, 517–527. doi: 10.1104/pp.103.022665
Wang, H., Jones, B., Li, Z., Frasse, P., Delalande, C., Regad, F., et al. (2005). The tomato Aux/IAA transcription factor IAA9 is involved in fruit development and leaf morphogenesis. Plant Cell 17, 2676–2692. doi: 10.1105/tpc.105.033415
Wang, S., Liu, J., Feng, Y., Niu, X., Giovannoni, J., and Liu, Y. (2008). Altered plastid levels and potential for improved fruit nutrient content by downregulation of the tomato DDB1-interacting protein CUL4. Plant J. 55, 89–103. doi: 10.1111/j.1365-313X.2008.03489.x
Yanagawa, Y., Sullivan, J. A., Komatsu, S., Gusmaroli, G., Suzuki, G., Yin, J., et al. (2004). Arabidopsis COP10 forms a complex with DDB1 and DET1 in vivo and enhances the activity of ubiquitin conjugating enzymes. Genes Dev. 18, 2172–2181. doi: 10.1101/gad.1229504
Yang, S. F., and Hoffman, N. E. (1984). Ethylene biosynthesis and its regulation in higher plants. Annu. Rev. Plant Physiol. 35, 155–189. doi: 10.1146/annurev.pp.35.060184.001103
Yen, H. C., Shelton, B. A., Howard, L. R., Lee, S., Vrebalov, J., and Giovannoni, J. J. (1997). The tomato high-pigment (hp) locus maps to chromosome 2 and influences plastome copy number and fruit quality. Theor. Appl. Genet. 95, 1069–1079. doi: 10.1007/s001220050664
Zhong, S., Zhao, M., Shi, T., Shi, H., An, F., Zhao, Q., et al. (2009). EIN3/EIL1 cooperate with PIF1 to prevent photo-oxidation and to promote greening of Arabidopsis seedlings. Proc. Natl. Acad. Sci. U.S.A. 106, 21431–21436. doi: 10.1073/pnas.0907670106
Keywords: fruit ripening, auxin, ethylene, photomorhogenesis, tomato, climacteric, high pigment mutant, light–dark
Citation: Cruz AB, Bianchetti RE, Alves FRR, Purgatto E, Peres LEP, Rossi M and Freschi L (2018) Light, Ethylene and Auxin Signaling Interaction Regulates Carotenoid Biosynthesis During Tomato Fruit Ripening. Front. Plant Sci. 9:1370. doi: 10.3389/fpls.2018.01370
Received: 30 June 2018; Accepted: 29 August 2018;
Published: 18 September 2018.
Edited by:
Anna N. Stepanova, North Carolina State University, United StatesCopyright © 2018 Cruz, Bianchetti, Alves, Purgatto, Peres, Rossi and Freschi. This is an open-access article distributed under the terms of the Creative Commons Attribution License (CC BY). The use, distribution or reproduction in other forums is permitted, provided the original author(s) and the copyright owner(s) are credited and that the original publication in this journal is cited, in accordance with accepted academic practice. No use, distribution or reproduction is permitted which does not comply with these terms.
*Correspondence: Luciano Freschi, ZnJlc2NoaUB1c3AuYnI=
Disclaimer: All claims expressed in this article are solely those of the authors and do not necessarily represent those of their affiliated organizations, or those of the publisher, the editors and the reviewers. Any product that may be evaluated in this article or claim that may be made by its manufacturer is not guaranteed or endorsed by the publisher.
Research integrity at Frontiers
Learn more about the work of our research integrity team to safeguard the quality of each article we publish.