- Laboratory of Rice Biology in Henan Province, Collaborative Innovation Center of Henan Grain Crops, College of Agronomy, Henan Agricultural University, Zhengzhou, China
Nitrogen (N) is a major essential nutrient for plant growth, and rice is an important food crop globally. Although ammonium (NH4+) is the main N source for rice, nitrate (NO3-) is also absorbed and utilized. Rice responds to NO3- supply by changing root morphology. However, the mechanisms of rice root growth and formation under NO3- supply are unclear. Nitric oxide (NO) and auxin are important regulators of root growth and development under NO3- supply. How the interactions between NO and auxin in regulating root growth in response to NO3- are unknown. In this study, the levels of indole-3-acetic acid (IAA) and NO in roots, and the responses of lateral roots (LRs) and seminal roots (SRs) to NH4+ and NO3-, were investigated using wild-type (WT) rice, as well as osnia2 and ospin1b mutants. NO3- supply promoted LR formation and SR elongation. The effects of NO donor and NO inhibitor/scavenger supply on NO levels and the root morphology of WT and nia2 mutants under NH4+ or NO3- suggest that NO3--induced NO is generated by the nitrate reductase (NR) pathway rather than the NO synthase (NOS)-like pathway. IAA levels, [3H] IAA transport, and PIN gene expression in roots were enhanced under NO3- relative to NH4+ supply. These results suggest that NO3- regulates auxin transport in roots. Application of SNP under NH4+ supply, or of cPTIO under NO3- supply, resulted in auxin levels in roots similar to those under NO3- and NH4+ supply, respectively. Compared to WT, the roots of the ospin1b mutant had lower auxin levels, fewer LRs, and shorter SRs. Thus, NO affects root growth by regulating auxin transport in response to NO3-. Overall, our findings suggest that NO3- influences LR formation and SR elongation by regulating auxin transport via a mechanism involving NO.
Introduction
Nitrogen (N) is a major essential nutrient for plant growth (Stitt, 1999). Ammonium (NH4+) and nitrate (NO3-) are the major sources of N for plants (Kronzucker et al., 2000). Rice (Oryza sativa L.) is a major staple food globally. NH4+ is the main form of N in paddy soil (Wang et al., 1993). Rice roots are exposed to both NH4+ and NO3-, and are efficient at acquiring NO3- by nitrification in the rhizosphere (Kirk and Kronzucker, 2005; Duan et al., 2007). It has been predicted that 40% of the total N acquired by rice roots grown under flooded conditions is NO3- (Kronzucker et al., 2000; Kirk and Kronzucker, 2005). However, most previous studies on root growth and formation in response to NO3- have focused on upland plants such as Arabidopsis and maize, and so further work is needed.
Plants have various mechanisms to adapt to NO3- supply, such as plasticity of root growth (Patterson et al., 2016; Shahzad and Amtmann, 2017; Sun C. et al., 2017). Localized NO3- supply stimulates the initiation and/or elongation of lateral roots (LRs) (Drew and Saker, 1975; Zhang and Forde, 1998; Friml et al., 2003). In Arabidopsis, the local stimulation of LR growth is caused by NO3- functioning as a signal rather than as a nutrient (Zhang and Forde, 1998). Nitrate transporters, transcription factors, and micro-RNAs regulate root growth and formation in response to NO3- (Remans et al., 2006; Vidal et al., 2010; Trevisan et al., 2011, 2012; Zhao et al., 2012, 2013; Alvarez et al., 2014; Yan et al., 2014; Huang et al., 2015). For example, NRT1.1, which encodes an NO3- transporter, reportedly regulates the stimulatory effects of NO3- on LR growth and development (Zhang and Forde, 1998; Zhang et al., 1999; Remans et al., 2006). AtNRT2.1 is involved in the response of roots to low NO3- supply, mainly in LR formation (Little et al., 2005; Remans et al., 2006). Knockdown of OsNAR2.1, a partner protein of the high-affinity nitrate transporter, inhibits LR formation in response to nitrate (Huang et al., 2015). NO3- regulates root growth by posttranscriptional regulation of the NRT1.1/NPF6.3 (Bouguyon et al., 2016). NPF7.3/NRT1.5, a nitrate transporter, is involved in LR formation in Arabidopsis (Zheng et al., 2016). miR444a plays key roles in nitrate-dependent LR elongation and nitrate accumulation by downregulating the expression of ANR1-like genes in the NO3- signalling pathway in rice (Yan et al., 2014). miR393/AFB3, an NO3--responsive module, regulates LR density in response to external and internal N concentrations in Arabidopsis (Vidal et al., 2010; Vidal et al., 2013). The transcript levels of four ANR1-like genes, OsMADS25, OsMADS27, OsMADS57 and OsMADS61, as well as TGA1/TGA4 and CPC, are influenced by NO3- supply and regulate root growth and formation (Yu et al., 2014; Canales et al., 2017; Sun et al., 2018). However, how plants sense external nitrate and the signal transduction system that influences root system development are remain unclear.
In addition to environmental conditions, the root growth of plant is regulated by plant hormones, such as auxin. Most auxin is synthesized in aboveground tissues by YUCCA family genes (Stepanova et al., 2011; Zhao, 2012) and is transported by auxin carriers, such as AUX1/LAX family (auxin-influx carriers), and ABCB/PGP and PIN family (auxin-efflux carriers) (Friml, 2003; Friml et al., 2003; Blakeslee et al., 2005; Zazimalova et al., 2010; Peret et al., 2012; Bhosale et al., 2018; Giri et al., 2018). Auxin plays a key role in root growth in response to NO3- (Zhang et al., 1999; Zhang and Mi, 2005; Krouk et al., 2010). Localized NO3- supply does not stimulate LR elongation in axr4, an auxin-insensitive mutant, which suggests that NO3- regulates LR growth via auxin signaling pathways (Zhang et al., 1999). The NO3- and auxin signaling pathways are linked by their effect on auxin transport through AtNRT1.1 (Krouk et al., 2010). Liu et al. (2010) suggested that in LRs, NO3--fed compartments have lower auxin levels than NO3--free compartments, and localized NO3- supply inhibits auxin transport from shoot to root in maize. Knockdown of OsNAR2.1 decreases LR formation by inhibiting auxin transport from shoots to roots (Huang et al., 2015). However, the roles of auxin transport in regulating LR growth under NO3- supply are more complex.
Nitric oxide (NO), as a signaling molecule, is involved in the growth and formation of the root system under NO3- supply (Manoli et al., 2014; Trevisan et al., 2014; Sun et al., 2015; Kan et al., 2016). NO synthase-like (NOS-like) and nitrate reductase (NR) are the two key NO production pathways in plants. The NOS of plant has not been identified (Crawford, 2006; Moreau et al., 2008, 2010; Gas et al., 2009; Gupta et al., 2011), although studies that have used inhibitors of the animal NOS enzyme have demonstrated the involvement of the L-arginine pathway in the production of NO (Zhao et al., 2007). Moreau et al. (2008) suggested that Arabidopsis AtNOS1 does not possess NOS activity, as it is a GTPase, and renamed it NO-associated enzyme (AtNOA1). Despite the lack of clarity on the role of AtNOS, the roots of noa1 mutants (formerly Atnos1) have lower NO levels than WT (Guo and Crawford, 2005; Schlicht et al., 2013). In plants, the NR pathway mediates NO generation, and the nitrate concentration in roots influences the production of NO by regulating NR activity (Yamasaki et al., 1999; Meyer et al., 2005; Yamasaki, 2005). The levels of nitrate and nitrite are important determinants of NR-induced NO generation (Vanin et al., 2004). NO is a nitrate-related signal generated by the NR pathway that regulates root growth and formation (Zhao et al., 2007; Manoli et al., 2014; Trevisan et al., 2014; Sun et al., 2015). However, the mechanism by which NO regulates the root system architecture requires further investigation.
The interactions between NO and auxin in regulating root growth are closely linked (Correa-Aragunde et al., 2004; Fernández-Marcos et al., 2011; Jin et al., 2011; Chen and Kao, 2012; Sun H. et al., 2017). Application of SNP (a NO donor) and IAA/IBA (exogenous auxin) increased the lateral root (LR) formation. This effect of SNP and IBA were significantly inhibited by cPTIO (a NO scavenger)(Jin et al., 2011; Chen and Kao, 2012; Sun H. et al., 2017), suggesting that NO maybe act downstream of auxin in regulation of LR development. However, the interaction between NO and auxin in regulating root elongation is different from affecting LR formation. NO inhibited the elongation of roots by decreasing acropetal auxin transport in Arabidopsis and rice (Fernández-Marcos et al., 2011; Sun H. et al., 2017), suggesting that the interactions between auxin and NO in regulating root growth are complex and unclear.
Rice, an important food crop globally, is an ideal model for studying plant root growth because of its small genome size and availability of its complete genome sequence and well-characterized related mutants (Feng et al., 2002; Sasaki et al., 2002). In this study, we evaluated LR formation and the length of seminal roots (SRs) of rice and measured auxin concentrations, DR5::GUS activity, [3H] indole-3-acetic acid (IAA) transport, and NO levels under NH4+ and NO3- supply. The results suggest that NO influences rice root growth by regulating auxin transport in response to NO3-.
Materials and Methods
Plant Materials
The Nipponbare and Dongjin (DJ) ecotype of rice were used in this study. osnia2-1 and osnia2-2 mutant lines (Sun et al., 2016) and ospin1b-1 and ospin1b-2 mutant lines (Sun H. et al., 2017) with the japonica cv. Dongjin ecotype were also used.
Plant Growth
Rice seedlings were grown at day/night temperatures of 30°C/18°C under natural light in a greenhouse. Seven-days-old seedlings of uniform size and vigor were transplanted into holes in a lid placed over the top of pots (four holes per lid and three seedlings per hole). Nutrient solutions ranging from one fourth (2 days), one third (2 days), and a half (2 days) to full strength (1 day) were applied for 1 week, followed by full-strength nutrient solution for 1 week. The chemical composition of International Rice Research Institute (IRRI) nutrient solution was (mM): 2.5 (NH4)2SO4 and/or Ca(NO3)2, 0.3 KH2PO4, 0.35 K2SO4, 1.0 CaCl2, 1.0 MgSO4⋅7H2O, 0.5 Na2SiO3; and (μM) 9.0 MnCl2, 0.39 (NH4)6Mo7O24, 20.0 H3BO3, 0.77 ZnSO4, and 0.32 CuSO4 (pH 5.5).
The treatments applied were as follows: 100 nM indole-3-acetic acid (IAA), auxin transport inhibitor 300 nM N-1-naphthylphthalamic acid (NPA), 10 μM sodium nitroprusside (SNP), 25 μM Tu (tungstate), 100 μM [2-(4-carboxyphenyl)-4,4,5,5-tetramethylimidazoline-1-oxyl-3-oxide] (cPTIO), and 100 μM L-NAME (NG-nitro-L-arginine methyl ester) (Sun H. et al., 2017).
Root System Architecture
The previous experiments (Sun et al., 2014) and the preliminary experiments suggested that the elongation of root (seminal root and adventitious root) and the lateral root (LR) number of seminal root/adventitious root were increased under NO3- relative to NH4+. The seminal root here is the first and longest root formation from embryo and functions mainly during the early stages of rice. Therefore, SRs and the numbers of LRs on SRs were used to evaluate the effects of NH4+ and NO3- on the root system. The length of SR was measured with a ruler. LRs were enumerated visually.
To visualize the formation of LR primordia, pDR5::GUS, a specific reporter that contains seven repeats of a synthetic auxin response element and reflects in vivo auxin levels (Ulmasov et al., 1997), were transformed into rice plants. After staining roots in β-glucuronidase (GUS) buffer for 2 h, LR primordia were enumerated using a stereomicroscope (Olympus SZX16) according to Sun H. et al. (2017). All experiments included eight replicates.
Determination of Total N Concentration
The shoots and roots were separated from rice plants, and heated at 105°C for 30 min to kill the enzyme activities, followed by desiccation at 70°C for 48 h to a constant weight. The desiccated samples were ground into powder, and about 0.05 g of the powder was digested using 5 mL of 98% H2SO4 and about 1 mL of 30% H2O2 at 270°C for 30 min. The digested liquid was diluted to 100 mL with distilled water after cooling. The total N concentration of rice plants was analyzed using the Kjeldahl method. A 5 mL aliquot from the 100 mL digested liquid was determined by a colorimetric continuous flow analysis (Autoanalyzer 3; Bran+Luebbe, Germany) (Li et al., 2008). All experiments included eight replicates.
Determination of IAA Levels
Indole-3-acetic acid levels of roots were determined as described previously (Lu et al., 2009). Fresh samples (0.5 g) were frozen in liquid N2. IAA levels were analyzed by high-performance liquid chromatography (HPLC).
To assess auxin distribution, rice plants were transformed with the pDR5::GUS constructs using Agrobacterium tumefaciens (strain EHA105). The roots were subjected to GUS staining. Stained plant tissues were photographed using a stereomicroscope (Olympus SZX16) equipped with a color CCD camera. All experiments included eight replicates.
[3H] IAA-Transport
Shoot-to-root auxin transport in rice plants was assayed according to Song et al. (2013). [3H]IAA polar transport was assayed in root samples under NH4+ and NO3- supply. The [3H]IAA solution contained 0.5 μM [3H]IAA (20 Ci mmol -1) in 2% dimethyl sulfoxide (DMSO), 25 mM MES (pH 5.2), and 0.25% agar.
Shoot to root auxin transport in intact plants was monitored as follows. [3H]IAA solution (20 μL) was applied to the cut surface after rice shoots were removed at 2 cm above the junction of shoot and root. After an 18 h (overnight) incubation in darkness, two root segments, namely all the lateral root (LR) region and the root tip (RT), were weighed and incubated in 4 mL of scintillation solution. [3H]IAA radioactivity was detected using a multipurpose scintillation counter (LS6500; Beckman-Coulter, Fullerton, CA, United States).
The assay for acropetal (3–6 cm from the root tip) and basipetal (0–3 cm from the root tip) auxin transport was performed. [3H]IAA solution (3 μL) was applied to the root tip placed horizontally on a plastic film. After incubation in a humid, dark environment for 18 h (overnight), root segments were cut into two parts: (1) the distal 1 cm from the root tip and (2) the remaining 2 cm. [3H]IAA radioactivity was measured in the 2 cm long segments. All experiments included five replicates.
Cortical Cell Length Analysis
Cortical cell length was analyzed as described by Jia et al. (2008). Cortical cells were visualized under a microscope (Olympus SZX16) equipped with a color CCD camera. The average cortical cell length of the maturation zone of SRs was determined using a mixture of 40–60 cortical cells at about 6 cortical cell layers (on per longitudinal section) with eight replicates in the maturation zone.
pCYCB1;1::GUS Construct
The pCYCB1;1::GUS fusion construct was generated as described by Colón-Carmona et al. (1999), and transformed into rice plants. Plants were stained for GUS activity in the root tips (RTs) for 2 h at 37°C. The RTs were subjected to histochemical GUS staining and photographed using a microscope (Olympus SZX16) equipped with a color CCD camera. All experiments included eight replicates.
Measurement of NO Levels in Roots
Nitric oxide was imaged by staining with 4-amino-5-methylamino-2′7′-difluorofluorescein diacetate (DAF-FM DA) under an epifluorescence microscope. The roots were soaked with 10 μM DAF-FM DA in 20 mM HEPES-NaOH buffer (pH 7.5) for 30 min in the dark. The roots were washed three times in fresh buffer and immediately visualized with a stereomicroscope (Olympus SZX16; excitation 488 nm, emission 495–575 nm) equipped with a color CCD camera. Green fluorescence intensity was quantified as described by Guo and Crawford (2005) using Photoshop software (Adobe Systems, San Jose, CA, United States). All experiments included eight replicates.
Measurement of Nitrate Reductase (NR) Activity in Roots
Nitrate reductase activity in rice roots was analyzed by Ogawa et al. (1999). The assay mixture contained 25 mM K3PO4 buffer (pH 7.5), 10 mM KNO3, 0.2 mM NADH, 5 mM NaHCO3, and 5 μL extract in a final volume of 0.5 mL. The assays were conducted at 30°C for 15 min. The reaction was terminated by adding 50 μL of 0.5 M Zn(CH3COO)2, and excess NADH was oxidized by adding 50 μL of 0.15 mM phenazine methosulphate. The mixture was centrifuged at 10,000 × g for 5 min. The NO2- level was quantified by combining 500 μL supernatant with 250 μ Lof 1% sulfanilamide prepared in 1.5 N HCl and 250 μL of 0.02% N-(1-naphthyl)ethylene-diamine dihydrochloride, and the absorbance at 540 nm was read using a spectrophotometer. All experiments included five replicates.
Quantitative Reverse Transcription-Polymerase Chain Reaction
Total RNA was isolated from the roots of rice plants under NH4+ or NO3- supply for 14 days. The RNA extraction, reverse transcription, and quantitative reverse transcription-polymerase chain reaction (qRT-PCR) methods were as described by Jia et al. (2011). All experiments with three replicates. The primer sets for PINs, YUCCAs, NOA, NIA1, NIA2, and CYCB1;1 are listed in Supplementary Tables 1–3.
Data Analysis
Data were pooled to calculate means and standard errors (SEs) and subjected to one-way analysis of variance (ANOVA), followed by a Ryan–Eynot–Gabriel–Welch F-test at P < 0.05 to determine the statistical significance of differences between treatments. All statistical evaluations were conducted using SPSS (version 11.0) statistical software (SPSS Inc., Chicago, IL, United States). All experiments included three independent biological replicates.
Results
NO3- Regulates LR Formation and SR Elongation
Compared to under NH4+ supply, the number of LRs and SR length were increased by 28 and 20%, respectively, under NO3- supply (Figure 1). However, the total N concentration in shoots and roots were decreased by about 20% under NO3- relative to under NH4+ supply. These results suggest that the root growth and total N concentration of rice plants are regulated by NO3- (Supplementary Figure 1).
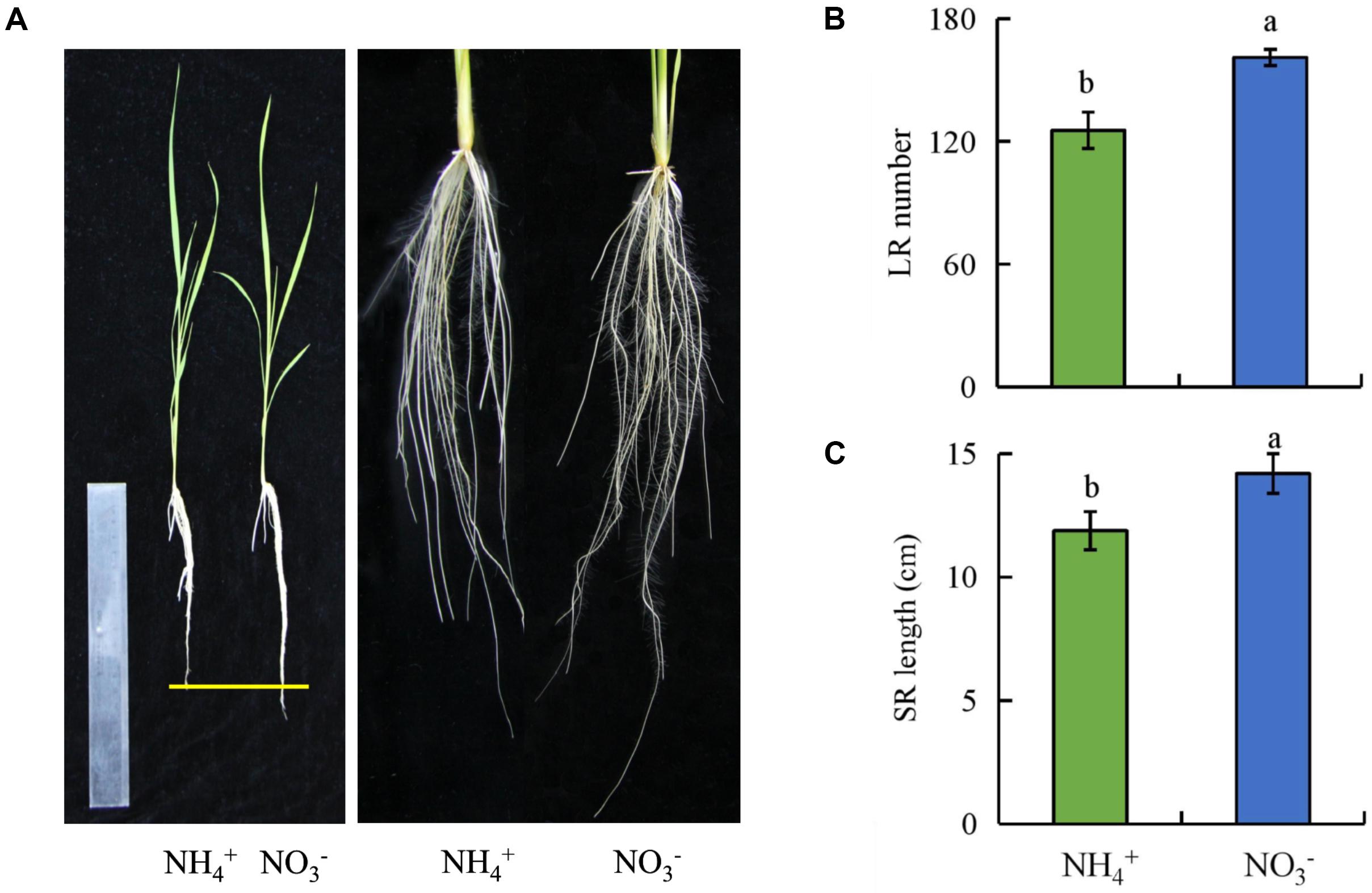
FIGURE 1. The lateral root (LR) number and seminal root (SR) length in wild-type (Nipponbare) rice seedlings. Seedlings were grown in hydroponic media containing NH4+ and NO3- for 14 days. (A), The morphology of the rice plants; (B), LR number; (C), SR length. Data are means ± SE and bars with different letters indicate significant difference at P < 0.05 tested with ANOVA.
NO Is Generated by the NR Pathway and Is Involved in LR Formation and SR Elongation Under NO3- Supply
To determine whether NO regulates LR formation and SR elongation under NO3- supply, we analyzed NO-associated green fluorescence in SRs (LR region and RT) (Figures 2A,B). Compared to NH4+, NO-associated green fluorescence signals in RTs and the LR regions were stronger under NO3- supply, which suggests that production of NO in roots is induced by NO3-.
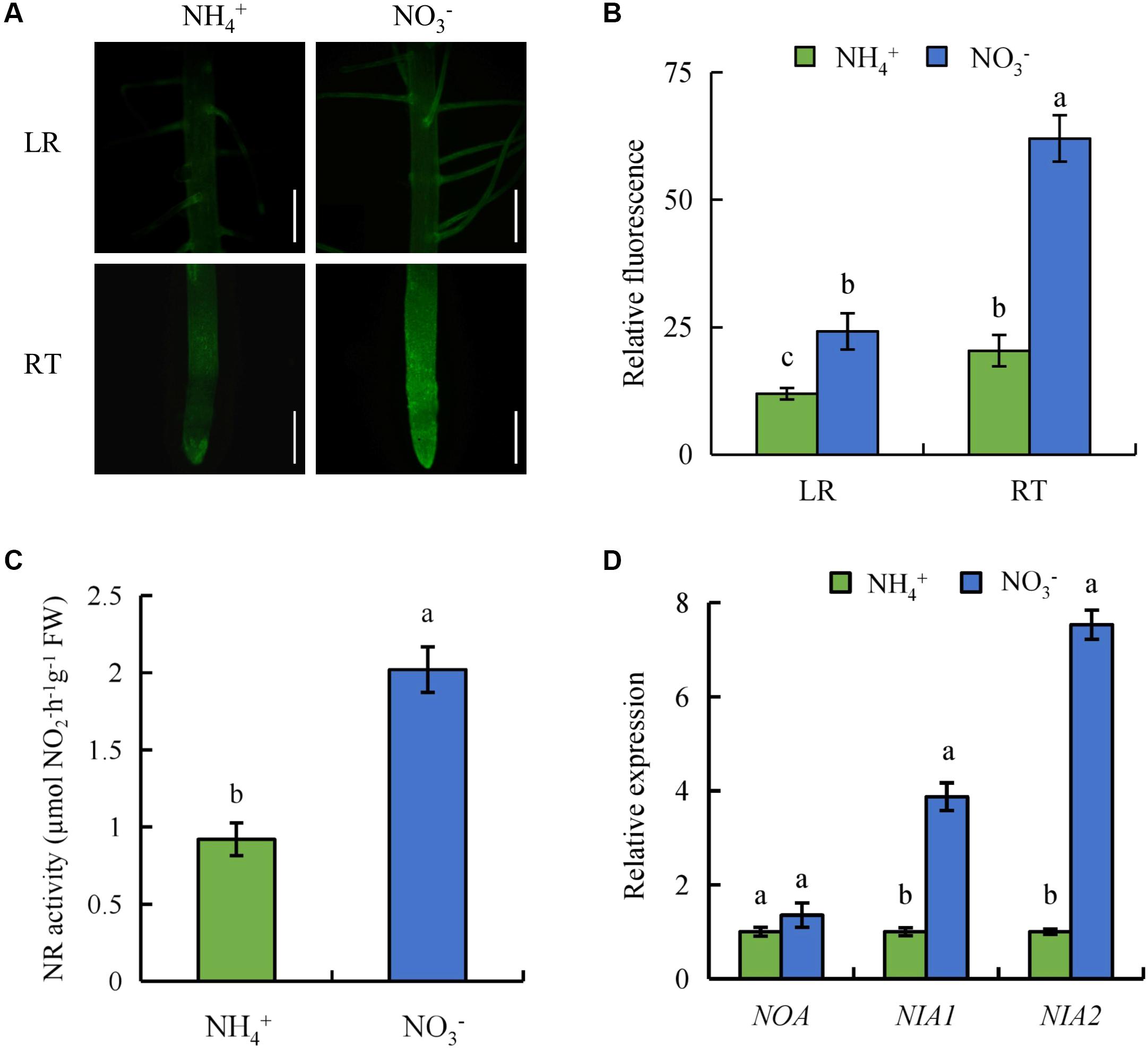
FIGURE 2. Accumulation of nitric oxide (NO), relative nitrate reductase (NR) activity, and qRT-PCR analysis of NO-associated (NOA), NIA1, NIA2 genes in wild-type (Nipponbare) rice seedlings. Seedlings were grown in hydroponic medium containing NH4+ and NO3- for 14 days. (A,B), Photographs of NO production shown as green fluorescence in the lateral root (LR) and root tip (RT) (A); and NO production expressed as fluorescence intensity relative to the roots (B); (C), Relative nitrate reductase (NR) activity in the roots; (D), Relative expression of NOA, NIA1 and NIA2 in roots. Bar = 1 mm. Data are means ± SE and bars with different letters indicate significant difference at P < 0.05 tested with ANOVA.
We examined the functions of an NO donor (SNP) and NO scavenger (cPTIO) in root elongation and LR formation under NO3- supply. Application of SNP under NH4+ supply significantly increased the NO-associated green fluorescence signal in SRs, the number of LRs, and the SR length to levels similar to those under NO3- supply (Figure 3). However, the number of LRs, and the SR length did not respond to SNP under NO3- supply (Supplementary Figure 2). Treatment with cPTIO under NO3- supply markedly decreased the NO-associated green fluorescence signal, the number of LRs, and the SR length (Figure 3). Thus, NO production in rice roots is enhanced by NO3- and is involved in LR formation and SR elongation.
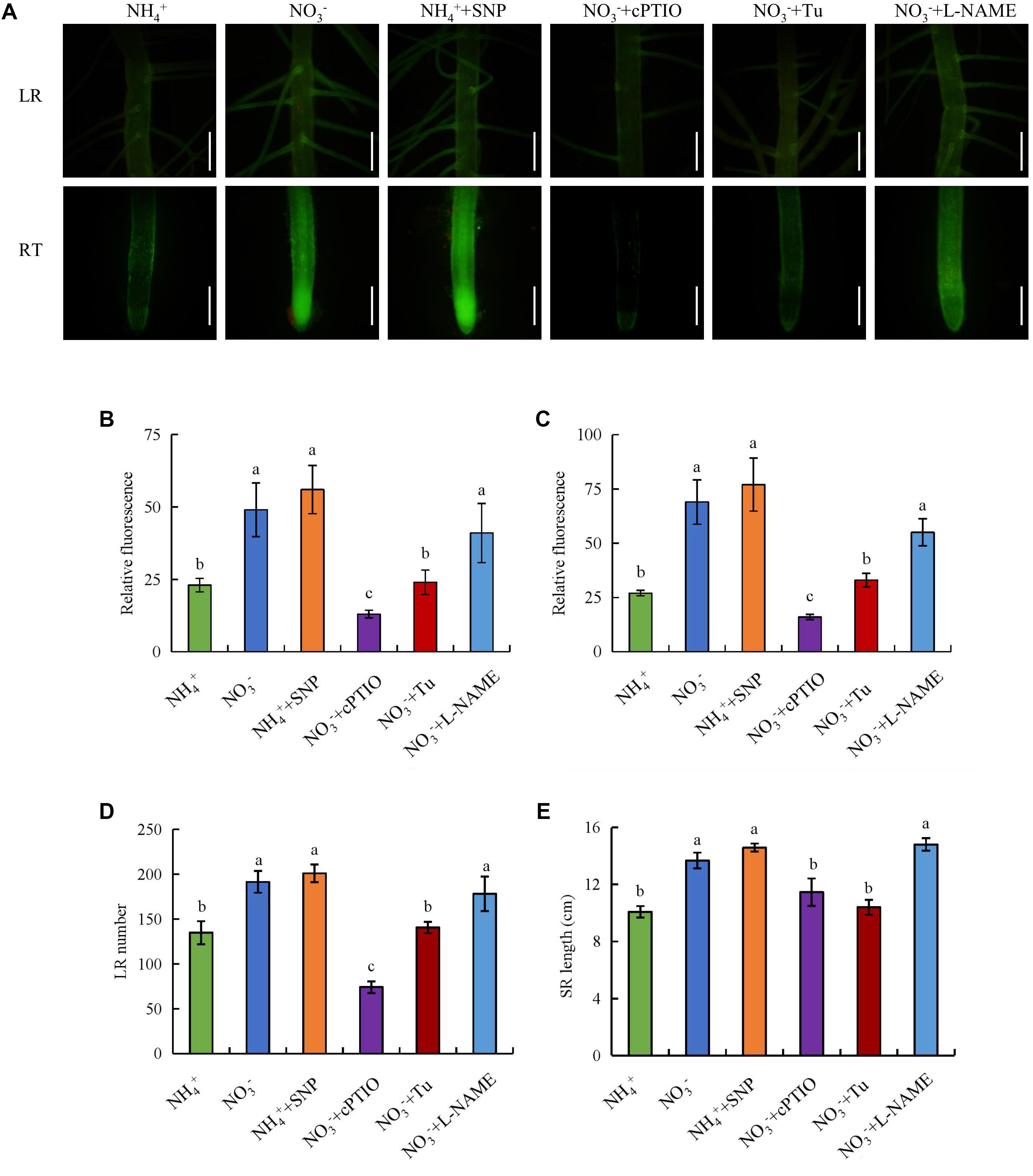
FIGURE 3. Accumulation of nitric oxide (NO) and root morphology in wild-type (Nipponbare). Seedlings were grown in hydroponic medium containing NH4+ and NO3- in addition to SNP (10 μM), cPTIO (100 μM), Tu (25 μM) and L-NAME (100 μM) for 14 days. (A–C), Photographs of NO production shown as green fluorescence in the lateral root (LR) and root tip (RT) (A), and NO production expressed as fluorescence intensity relative to lateral root (B) and root tip (C); (D), Lateral root (LR) number; (E), Seminal root (SR) length. Bar = 1 mm. Data are means ± SE and bars with different letters indicate significant difference at P < 0.05 tested with ANOVA.
Nitrate reductase activity in rice roots was assessed under NH4+ and NO3- supply. NR activity increased by 119% in roots under NO3- supply relative to NH4+ supply (Figure 2C). The expression of NIA2 was significantly higher under NO3- supply than under NH4+ supply. However, compared with NIA2, the expression of NIA1 had less differences between NH4+ and NO3-. The transcript level of NO-associated (NOA) (a homolog of NOA1 in Arabidopsis) in roots was similar under NH4+ supply and NO3- supply (Figure 2D). These results suggest that NO generation is enhanced by NR rather than the NOS-like pathway under NO3- supply.
Application of the NR inhibitor Tu (25 μM) decreased the NO-associated green fluorescence signal, the number of LRs, and the SR length under NO3-. However, treatment of rice plants with the NOS inhibitor L-NAME (100 μM) under NO3- supply did not influence any of the parameters (Figure 3). These results confirm that NO is generated by NR rather than NOS-like under NO3- supply.
The osnia2-1 and osnia2-2 mutant lines have reduced NR activity (Sun et al., 2016). All parameters of both nia2 mutant lines were similar to those of WT plants under NH4+ supply, but significantly lower under NO3- supply (Figure 4). Application of SNP to nia2 mutants under NO3- supply increased the number of LRs and the SR length to levels similar to those in the WT (Supplementary Figure 3). Moreover, treatment of WT with Tu decreased the number of LRs and SR length to levels similar to those in the nia2 mutants (Supplementary Figure 3), confirming that NO is produced via the NIA2-dependent NR pathway under NO3- supply.
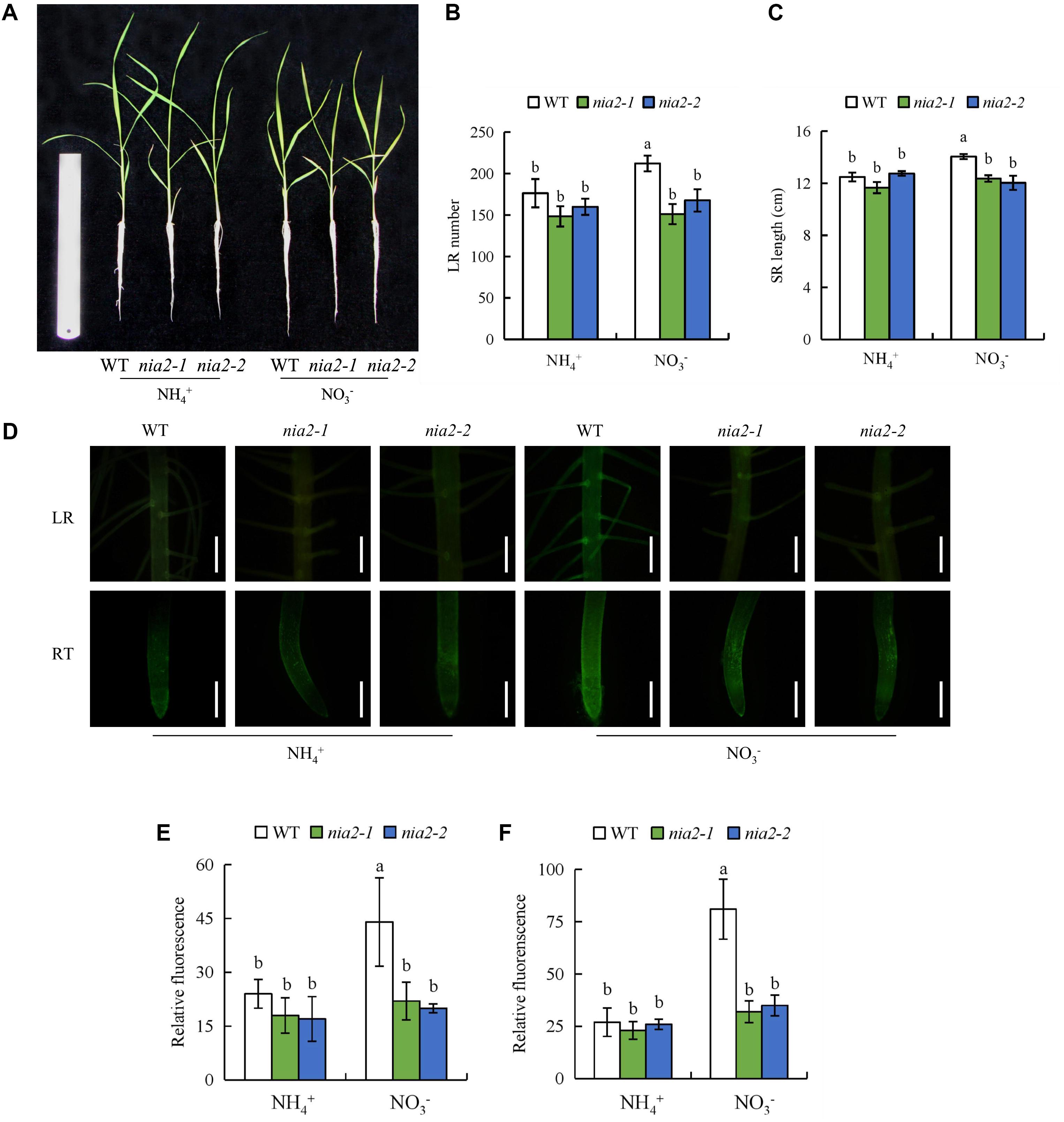
FIGURE 4. Root morphology and accumulation of nitric oxide (NO) in nia2 mutants and wild-type (DJ) the rice seedlings. Seedlings were grown in hydroponic medium containing NH4+ and NO3- for 14 days. (A), The morphology of the rice plants; (B), LR number; (C), SR length; (D), Photographs of NO production shown as green fluorescence in the lateral root (LR) and root tip (RT); (E-F), NO production expressed as fluorescence intensity relative to lateral root (E) and root tip (F). Bar = 1 mm. Data are means ± SE and bars with different letters indicate significant difference at P < 0.05 tested with ANOVA.
Auxin Levels in Roots Are Regulated by NO3-
We measured endogenous IAA concentrations in the LR region and RT. The endogenous IAA concentrations were 75 and 91% higher in the LR region and RT, respectively, under NO3- relative to NH4+ (Figure 5A). We investigated the effects of NH4+ and NO3- on auxin status in rice with transgenic plants transformed with the pDR5::GUS constructs. DR5::GUS activity was more widely distributed in the LR region and RT under NO3- relative to NH4+ supply (Figure 5C). This was consistent with the IAA concentration results. [3H] IAA transport from shoots to roots was significantly higher in roots under NO3- relative to NH4+ supply. Basipetal transport and acropetal transport of [3H] IAA were higher under NO3- relative to NH4+ supply (Figures 5B,D). Therefore, polar auxin transport was increased under NO3- supply.
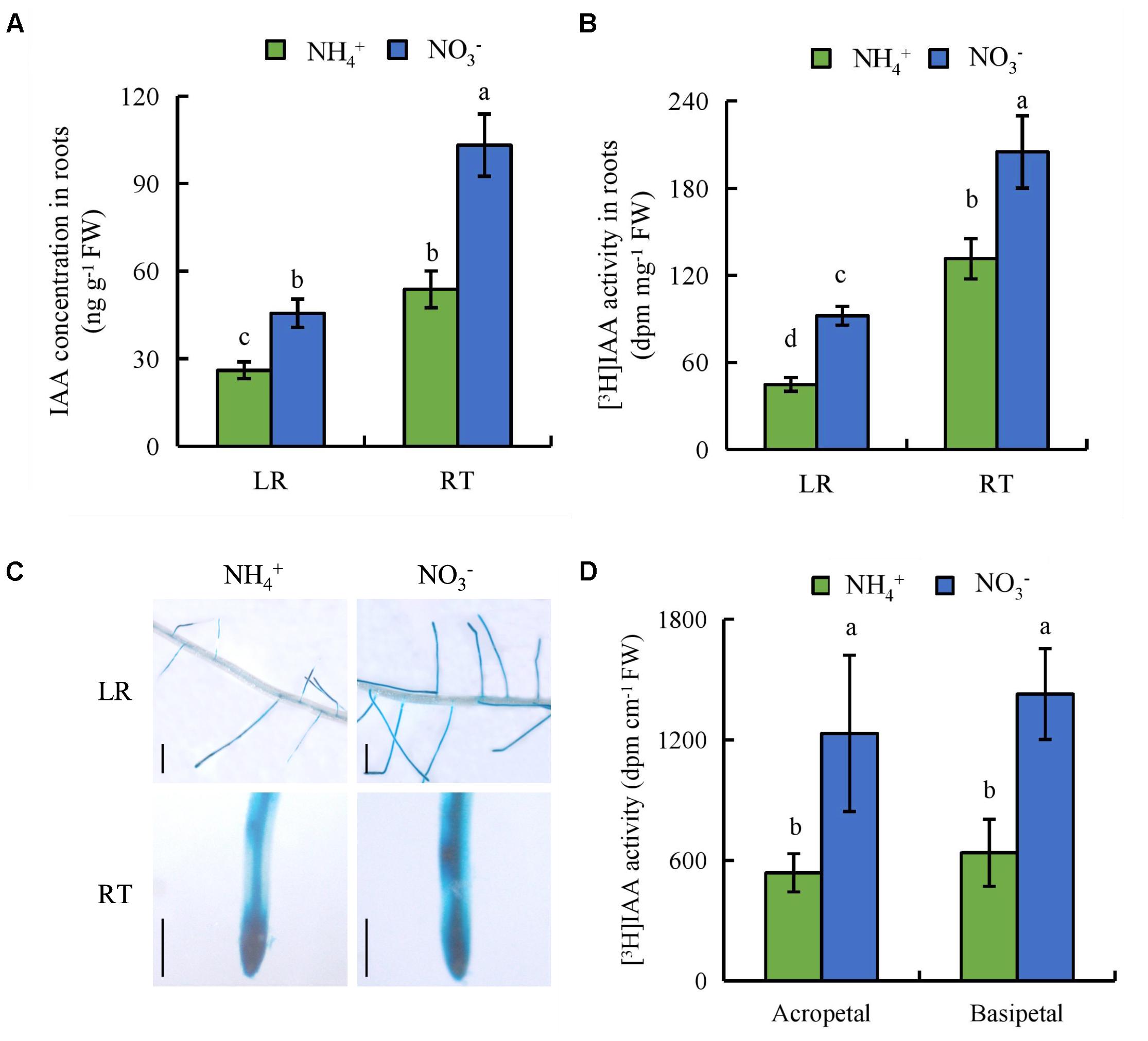
FIGURE 5. IAA concentration, [3H]IAA transport and histochemical localization of DR5::GUS activity in rice seedlings. Rice seedlings were grown in hydroponic media containing NH4+ and NO3- for 14 days. (A), IAA concentration in roots; (B,D) [3H] IAA activity in the root tip (RT) and lateral root zone (LR); (C), DR5::GUS, a specific reporter that contains seven repeats of a highly active synthetic auxin response element and can reflect the in vivo auxin level. Roots were stained for GUS activity for 2 h at 37°C. Bar = 1 mm. Data are means ± SE and bars with different letters indicate significant difference at P < 0.05 tested with ANOVA.
Auxin Is Involved in SR Elongation and LR Formation
We examined the number of LRs and the SR length after application of IAA and NPA (Figure 6). Application of IAA (100 nM) under NH4+ supply increased DR5::GUS expression in roots, the number of LRs, and the SR length to levels similar to those under NO3- supply. The effects of application of IAA (100 nM) on DR5::GUS expression in roots and root morphology was of lesser magnitude under NO3- supply. Treatment with NPA (300 nM) under NO3- supply markedly decreased the DR5::GUS expression level in roots, the number of LRs, and SR length to levels similar to those under NH4+ supply. The effects of application of NPA (300 nM) on DR5::GUS expression in roots and root morphology was of lesser magnitude under NH4+ supply (Figure 6). These results suggest that SR elongation and LR formation are regulated by auxin transport under NO3- supply.
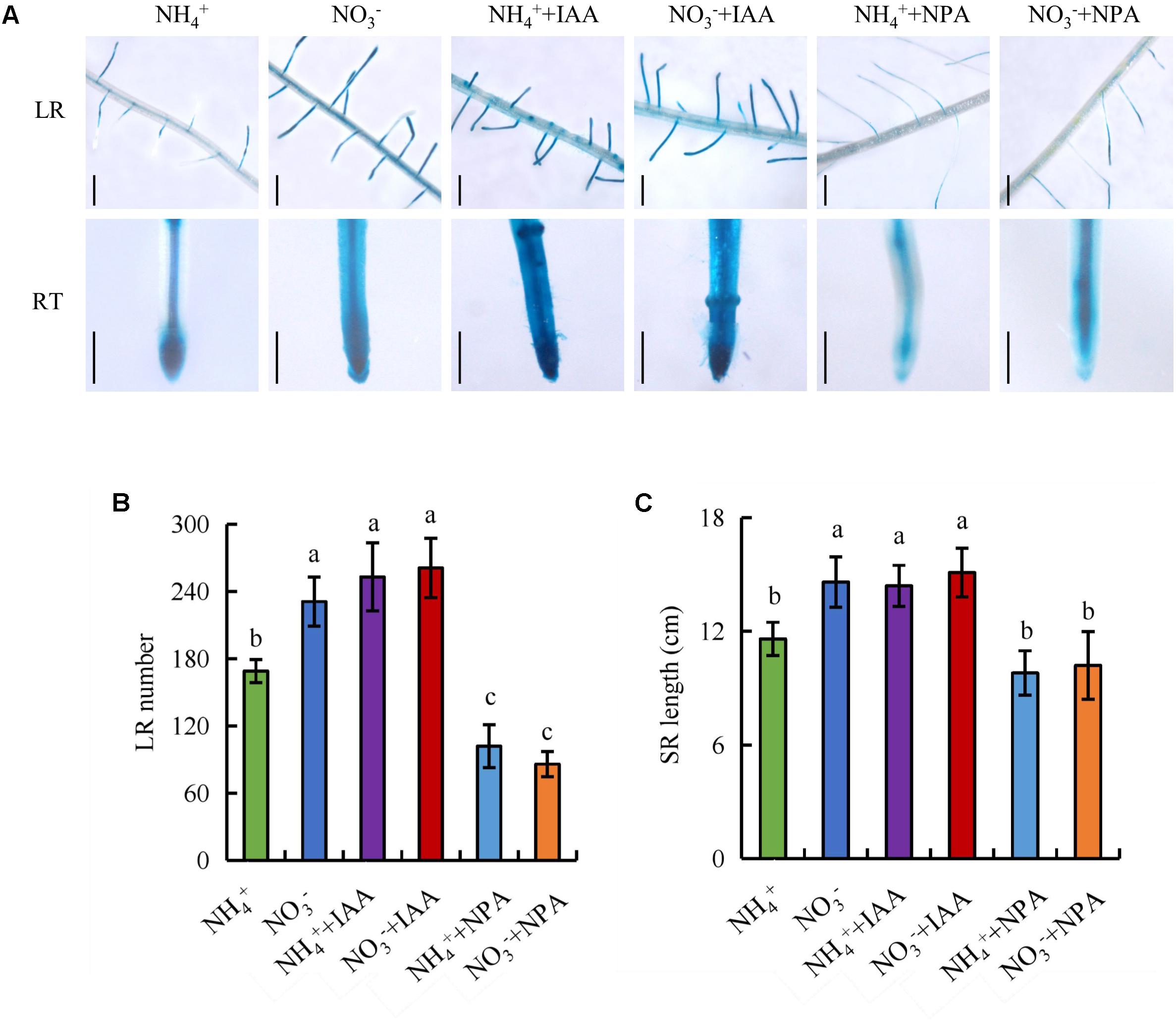
FIGURE 6. DR5::GUS activity and root morphology in wild-type (DJ). Seedlings were grown in hydroponic medium containing NH4+ and NO3- in addition to IAA (100 nM) and NPA (300 nM) for 14 days. (A), DR5::GUS activity in the lateral root zone (LR) and root tip (RT); (B), Lateral root (LR) number; (C), Seminal root (SR) length. Bar = 1 mm. Data are means ± SE and bars with different letters indicate significant difference at P < 0.05 tested with ANOVA.
Expression of OsPIN Family Genes and Root Morphology of Ospin1b Mutants
We analyzed the expression of the PIN1-10 auxin transport genes in roots (Figure 7). Compared to under NH4+ supply, the expression levels of PIN genes in roots were upregulated under NO3- supply (Figure 7). The expression level of OsPIN1b is the highest of the nine OsPIN genes in rice root (Wang et al., 2009; Sun H. et al., 2017). Therefore, OsPIN1b was used as a target gene in subsequent analyses.
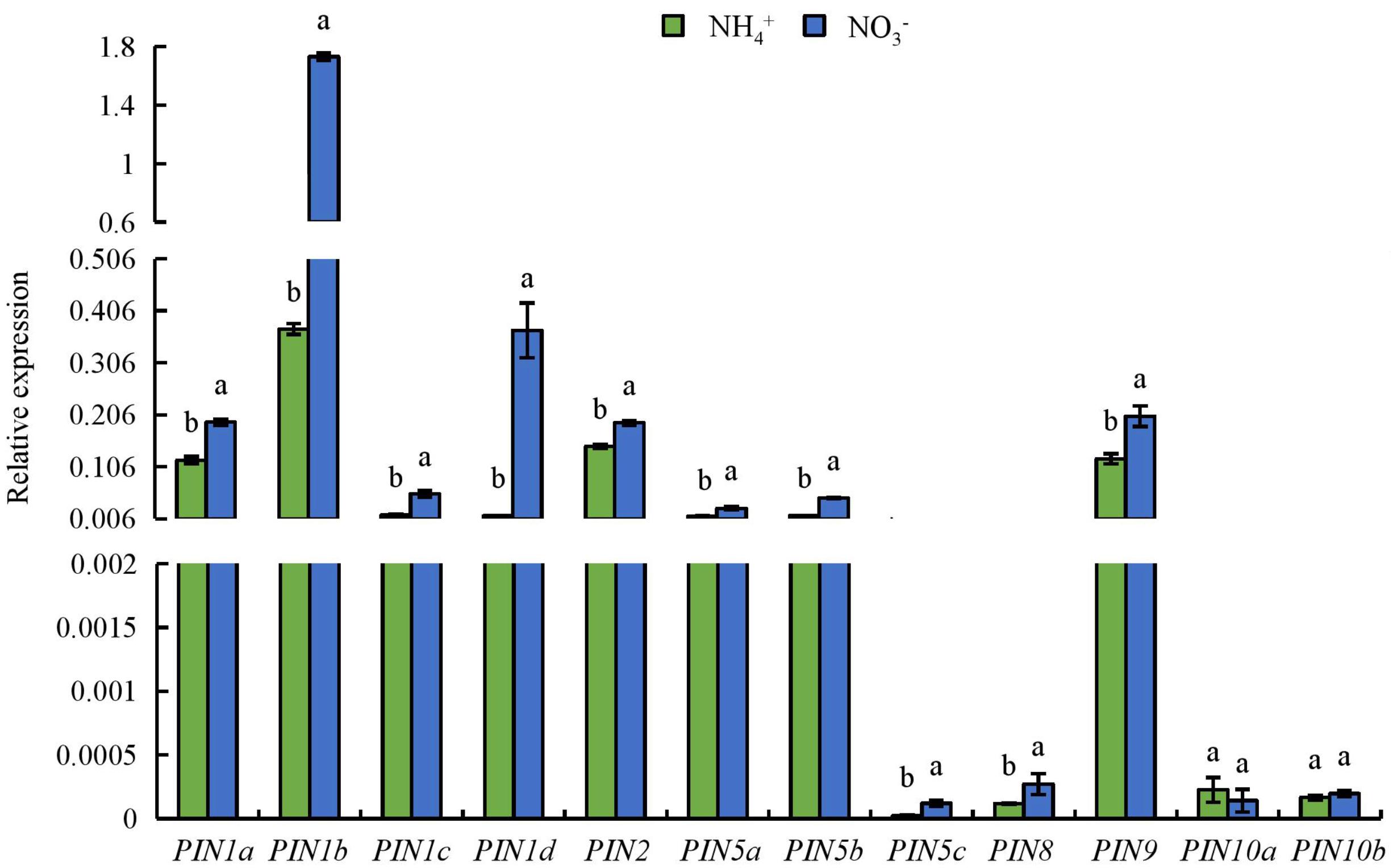
FIGURE 7. qRT-PCR analysis of PIN family genes in rice seedlings. Seedlings were grown in hydroponic medium containing NH4+ and NO3- for 14 days. Relative mRNA levels were normalized for individual gene relative to OsACT. Data are means ± SE and bars with different letters in the same gene indicate significant difference at P < 0.05 tested with ANOVA.
The ospin1b-1 and ospin1b-2 mutant lines have reduced auxin levels in LRs and the RT (Sun H. et al., 2017). The IAA concentration in roots of the ospin1b-1 mutant did not differ between NH4+ and NO3- supply (Figures 8B,C). The number of LRs and the SR length of the ospin1b mutant did not respond to NH4+ or NO3-. Compared to WT plants, the number of LRs and the SR length of the two ospin1b mutants were reduced under both NH4+ and NO3- supply (Figures 8D,E). These findings confirm that LR formation and SR elongation are regulated by auxin polar transport under NO3- supply.
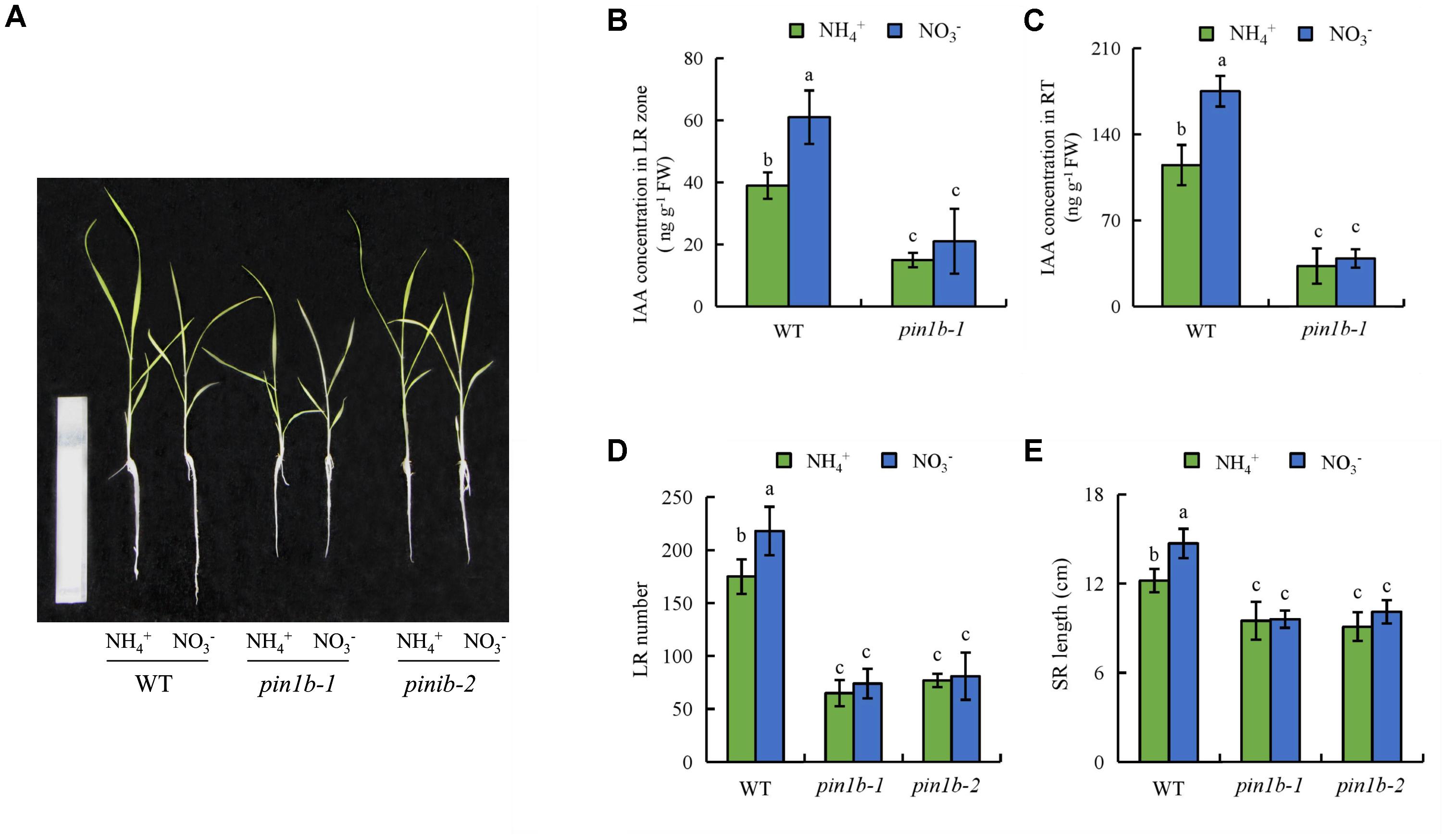
FIGURE 8. Root morphology and IAA concentration in pin1b mutants and wild-type (DJ) the rice seedlings. Seedlings were grown in hydroponic medium containing NH4+ and NO3- for 14 days. (A), The morphology of the rice plants; (B,C), IAA concentration in the lateral root region (B) and root tip (C); (D), Lateral root (LR) number; (E), Seminal root (SR) length. Bar = 1 mm. Data are means ± SE and bars with different letters indicate significant difference at P < 0.05 tested with ANOVA.
NO Regulates Auxin Transport Under NO3- Supply
Both NO and auxin are involved in regulation of root growth in response to NO3- supply, so we investigated the effects of their interaction. Application of SNP under NH4+ supply increased DR5::GUS activity and [3H] IAA activity in roots to levels similar to those under NO3- supply. Moreover, treatment with cPTIO under NO3- supply decreased DR5::GUS expression and [3H] IAA activity in roots to levels similar to those under NH4+ supply (Figures 9A,B). However, application of IAA to roots did not affect the levels of NO in LR and RT under NH4+ condition (Supplementary Figure 6).These results suggest that NO regulates auxin transport under NO3- supply. The expression of YUCCA1-8 in the first leaf had no differences under NH4+ with or without SNP (Supplementary Figure 4A). However, compared with NH4+, application of SNP up-regulated the levels of OsPIN1b and OsPIN1d gene expression (Supplementary Figure 4B).
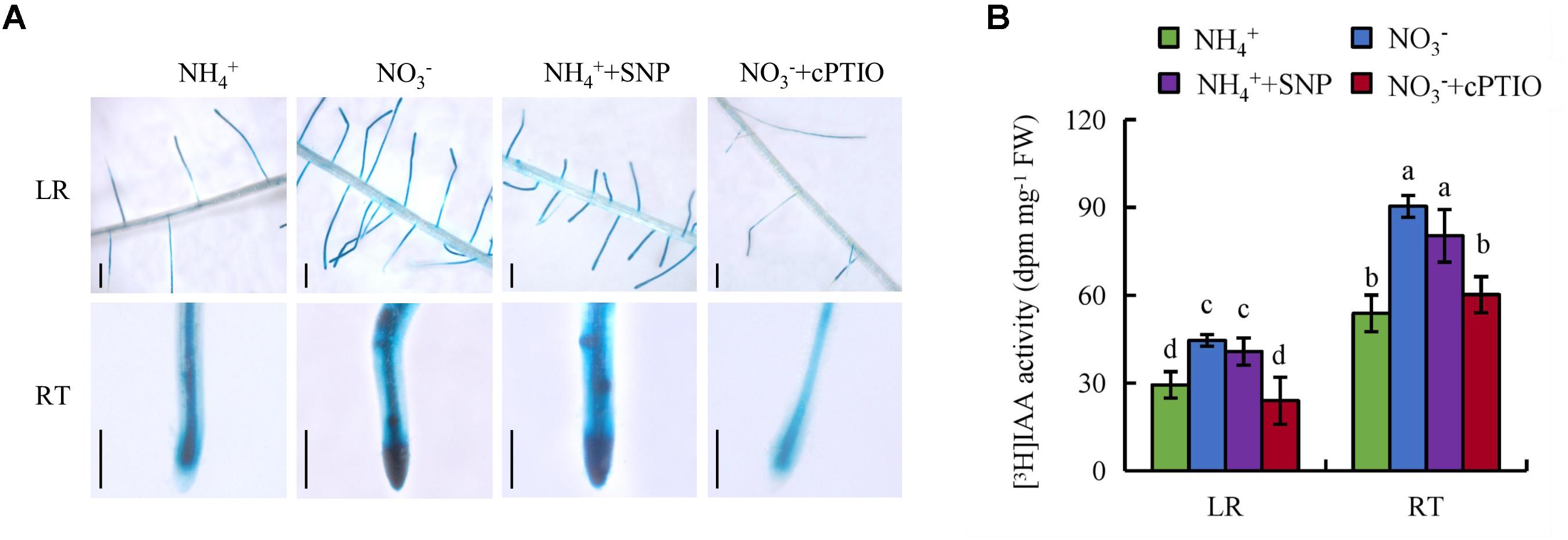
FIGURE 9. Histochemical localization of DR5::GUS activity and [3H]IAA transport in wild-type (DJ). Seedlings were grown in a hydroponic media containing NH4+ and NO3- in addition to SNP (10 μM) and cPTIO (80 μM) for 14 days. (A), DR5::GUS, a specific reporter that contains seven repeats of a highly active synthetic auxin response element and can reflect the in vivo auxin level. Roots were stained for GUS activity for 2 h at 37°C; (B), [3H] IAA activity in root tip (RT) and lateral root zone (LR). Data are means ± SE and bars with different letters indicate significant difference at P < 0.05 tested with ANOVA.
To determine the effects of duration of NO exposure for auxin buildup and root architecture change. The levels of DR5::GUS, LR number and SR length were examined over 16 days under NH4+ with or without SNP supply (Supplementary Figure 5). The results showed that the levels of DR5::GUS in LR region and RT were increased from 2 and 1 days, respectively, under SNP supply relative to application of NH4+ alone (Supplementary Figures 5A,B). Compared with sole NH4+ supply, the LR number and SR length were increased from 10 days under SNP treatment (Supplementary Figures 5C,D).
LR Primordia Formation and Root Meristem Activity Under NO3- Supply
To determine the mechanism by which NO3- regulates LR formation and SR elongation, we enumerated LR primordia, determined the lengths of epidermal cells in the maturity zone, and assayed CYCB1;1::GUS activity in the RT (Figure 10). The number of LR primordia increased by 61% under NO3- relative to NH4+ supply, which suggests that LR formation is dependent on LR primordia (Figures 10A–D,K). The lengths of epidermal cells did not differ between NH4+ and NO3- supply (Figures 10E–H,M), which suggests that the promotion of root elongation by NO3- was not due to changes in cell elongation. We used transgenic plants expressing the pCYCB1;1::GUS construct to assess the cyclic activity of cells in the root meristem. CYCB1;1::GUS activity and CYCB1;1 expression in the root meristem were increased under NO3- relative to NH4+ supply (Figures 10I,J,L). Therefore, NO3- affected LR formation by increasing LR primordia formation and promoted root elongation mainly by increasing root meristem activity rather than the elongation of epidermal cells in the maturity zone.
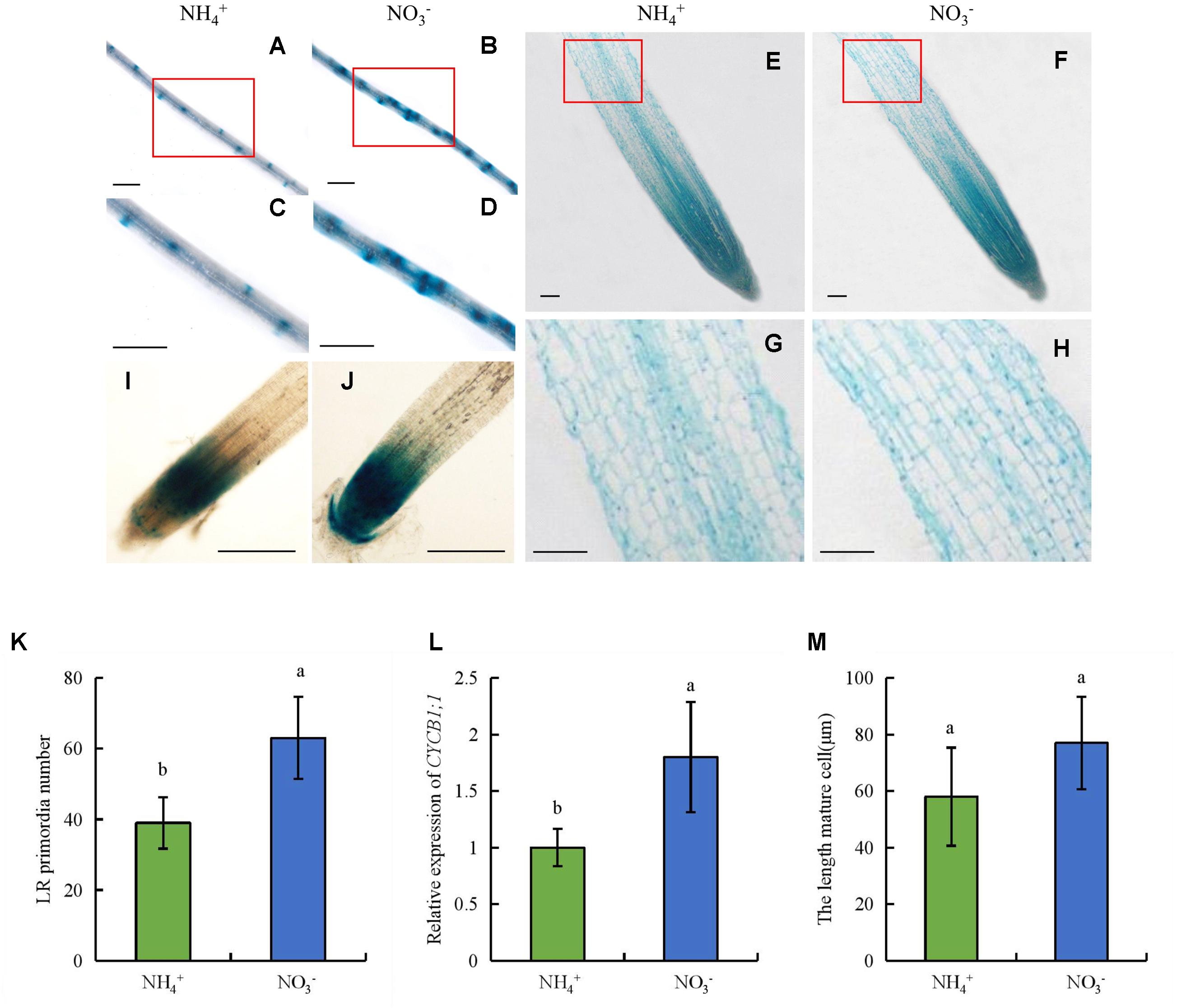
FIGURE 10. LR primordia and epidermal cell lengths in the maturity zone, expression levels of pCYCB1;1::GUS and OsCYCB1;1 in the meristem zone. Seedlings were grown in hydroponic media containing NH4+ and NO3- for 14 days. (A–D,K), Lateral root primordia. Bar = 1 mm; (E–H,M), Epidermal cell length of seminal root. Bar = 100 μm; (I,J), Cell cycle activity of the root meristem of seminal root, as monitored by the pCYCB1;1::GUS reporter. Bar = 500 μm; (L), The expression of CYCB1;1 gene. Data are means ± SE and bars with different letters indicate significant difference at P < 0.05 tested with ANOVA.
Discussion
The regulation of root elongation and formation in response to NO3- supply is important for the growth of plants. Two examples of the plasticity of root growth and development are promotion of root elongation and LR growth under NO3- supply. In upland species such as maize and Arabidopsis, the root length is increased under NO3- supply (Liu et al., 2013; Manoli et al., 2014) and localized NO3- supply stimulates LR elongation (Zhang and Forde, 1998; Friml et al., 2003). In rice, localized NO3- supply may stimulate LR elongation relative to no NO3- supply (Wang et al., 2002). In this study, compared to NH4+, NO3- supply increased the SR length and stimulated the formation of LRs (Figure 1), which suggests that the functions of NO3- in regulating rice root growth and development are similar in maize and Arabidopsis.
Several lines of studies suggested that NO had two strategies in plants response to NO3- supply. Firstly, NO as a signaling molecule functions in the regulation of root growth and formation in plants under NO3- condition (Manoli et al., 2014; Sun et al., 2015). Manoli et al. (2014) suggested that the NO3--induced increase in root length is dependent on the NO signaling pathway. Sun et al. (2015) showed that NO is induced by partial nitrate nutrition (PNN) and is involved in LR formation in rice. Secondly, NO enhanced N uptake by increasing the expression of N transport genes under PNN (Sun et al., 2015). In this study, application of SNP increased the SR length and LR number under NH4+. Treatment with cPTIO under NO3- supply decreased the SR length and the number of LR (Figure 3), These results confirm that NO is involved in LR formation and SR elongation in the presence of NO3-. The concentrations of total N in rice plants were decreased under NO3- relative to NH4+ (Supplementary Figure 1), suggesting NH4+ is the main N source for rice. NO was induced by PNN condition and NO could enhance the N uptake in rice (Sun et al., 2015). In this study, NO production was induced by NO3- maybe a strategy for rice plants to obtain more N.
NOS-like and NR pathways participated in NO production in plants (Wilson et al., 2008). In Arabidopsis, the gene of AtNOS1 did not regulate NOS activity, therefore, it was renamed NO-associated enzyme (NOA1) (Moreau et al., 2008). The NO levels were significantly decrease in the root of noa1 mutant (formerly Atnos1) relative to WT plants (Guo and Crawford, 2005). Besides NOA1-dependent pathway, NIA1 was involved in NR-regulated NO production in plants (Bright et al., 2006; Zhao et al., 2009). NIA2 expression is higher than that of NIA1 (Fan et al., 2007; Sun et al., 2015). Sun et al. (2015) reported that the NO generated by NIA2-dependent NR increases LR formation in rice. In this study, NR activity and NIA2 expression were significantly higher under NO3- supply relative to NH4+ supply. Moreover, the regulation of SR elongation and LR formation by NO3- was inhibited by Tu (NR inhibitor) but not by L-NAME (NOS inhibitor) (Figures 2D, 3), which suggests that NO regulated root growth and formation under NO3- supply main via the NR pathway. The changes in the root morphology and NO-associated green fluorescence signal of nia2 mutants were little affected by NO3- (Figure 4). This suggests that NO is produced by the NR pathway rather than the NOS-like pathway and is involved in regulation of root growth under NO3- supply.
Auxin distribution in the LR region is regulated by auxin transport, and auxin controls LR initiation and elongation in response to NO3- supply (Grieneisen et al., 2007; Vanneste and Friml, 2009; Krouk et al., 2010; Song et al., 2013). Application of a low concentration of NO3- affects LR growth by regulating auxin transport (Krouk et al., 2010). Liu et al. (2010) reported that local application of NO3- reduces acropetal and basipetal transport compared to N-free treatment, and decreases auxin distribution in the LR region to a level more suitable for LR elongation in maize. Song et al. (2011, 2013) found that auxin synthesis and auxin transport from shoot to root are higher under (PNN treatment relative to application of NH4+ alone in a high-NO3--response rice cultivar. The polarity of auxin transport is determined by the asymmetric localisation of the AUX1 and PIN auxin influx and efflux facilitators (Kramer, 2004). PIN proteins are the main auxin efflux carriers in plants (Friml et al., 2003; Wisniewska et al., 2006). Song et al. (2013) reported that PIN5b expression is upregulated under PNN relative to NH4+ supply. In Arabidopsis, PIN2 expression is upregulated in roots under NO3- supply compared to NH4+ (Liu et al., 2013). In this study, the auxin levels in LR and RT were higher under NO3- relative to NH4+ supply (Figures 5B,D), which suggests that the auxin distribution in roots is regulated by NO3- supply. [3H] IAA transport and PIN family gene expression were increased under NO3- relative to NH4+ supply (Figures 5B,D, 7), which suggests that PIN genes are involved in auxin transport under NO3- supply.
NO and auxin help regulate root growth and formation (Jin et al., 2011; Chen and Kao, 2012). NO acts downstream of auxin in regulating lateral root formation (Chen et al., 2010; Jin et al., 2011; Cao et al., 2017) and affects root elongation by regulating polar auxin transport (Fernández-Marcos et al., 2011). In rice, NO functions downstream of auxin in regulating LR formation but inhibits elongation of root by decreasing auxin levels in root tips under Fe deficiency (Sun H. et al., 2017). However, Manoli et al. (2016) found that the NO-mediated root apex responses to NO3- are regulated by auxin in maize. These results suggest that the interactions between auxin and NO in regulating root growth are complex. In this study, application of SNP under NH4+ supply increased the auxin levels in roots, and treatment with cPTIO under NO3- supply decreased the auxin levels in the roots (Figure 9). Thus, NO is involved in NO3--regulated auxin transport in roots. However, treatment with IAA did not affect the level of NO in roots under NH4+ supply, consistent with the previous report by Sun H. et al. (2017). These results suggested that NO maybe act upstream of auxin in regulating root growth and formation. The expression of PIN1b and PIN1d in roots were up-regulated under SNP supply relative to application of NH4+ alone. However, the expression of YUCCAs in the first leaf had no changes between NH4+ and NH4+ in addition to SNP (Supplementary Figure 4), suggesting that NO increased auxin levels in root mainly by regulating auxin transport but not auxin synthesis. Compared to WT, roots of the pin1b mutant had lower auxin levels, fewer LRs, and shorter SRs (Figure 8). Moreover, the root morphology of the pin1b mutant had less changes between NH4+ and NO3- (Figures 8D,E). Therefore, NO3- affects root growth by regulating root auxin transport via a mechanism involving NO. And these results suggest that the interactions between auxin and NO in regulating root growth in response to NO3- supply are different from Fe deficiency.
Lateral root formation is dependent on LR primordia initiation under NO3- supply (Song et al., 2013; Sun et al., 2015). In this study, the number of LR primordia was higher under NO3- supply compared to NH4+. Root length depends on two basal formation processes: cell division in the RT meristem and the length of root cells in the maturity zone (Scheres et al., 2002). The activity of meristematic cells in the root meristem affects root elongation (Blilou et al., 2005). NO3- supply increases root meristem activity by regulating the expression of CYCB1;1 in Arabidopsis (Liu et al., 2013). In this study, NO3- supply increased pCYCB1;1::GUS construct and CYCB1;1 expression levels in the RT but did not affect the length of mature cells (Figure 10). These findings suggest that SR elongation is regulated by increasing cell division in the root meristem zone under NO3- relative to NH4+ supply.
Conclusion
In conclusion, NO is generated mainly by the NR pathway and induces LR formation and SR elongation by regulating auxin transport in the presence of NO3-. NO3- influences LR formation by increasing the number of LR primordia, and root elongation by increasing root meristem activity.
Author Contributions
HS and FF performed the experiments and wrote the paper, JL analyzed the data, QZ designed the experiment.
Funding
This work was funded by the National Nature Science Foundation of China (Grant No. 31601821).
Conflict of Interest Statement
The authors declare that the research was conducted in the absence of any commercial or financial relationships that could be construed as a potential conflict of interest.
Supplementary Material
The Supplementary Material for this article can be found online at: https://www.frontiersin.org/articles/10.3389/fpls.2018.00659/full#supplementary-material
References
Alvarez, J. M., Riveras, E., Vidal, E. A., Gras, D. E., Contreras-López, O., Tamayo, K. P., et al. (2014). Systems approach identifies TGA1 and TGA4 transcription factors as important regulatory components of the nitrate response of Arabidopsis thaliana roots. Plant J. 80, 1–13. doi: 10.1111/tpj.12618
Bhosale, R., Giri, J., Pandey, B. K., Giehl, R. F. H., Hartmann, A., Traini, R., et al. (2018). A mechanistic framework for auxin dependent Arabidopsis root hair elongation in response to low external phosphate. Nat. Commun. 9:1409. doi: 10.1038/s41467-018-03851-3
Blakeslee, J., Peer, W., and Murphy, A. (2005). Auxin transport. Curr. Opin. Plant Biol. 8, 494–500. doi: 10.1016/j.pbi.2005.07.014
Blilou, I., Xu, J., Wildwater, M., Willemsen, V., Paponov, I., Friml, J., et al. (2005). The PIN auxin efflux facilitator network controls growth and patterning in Arabidopsis roots. Nature 433, 39–44. doi: 10.1038/nature03184
Bouguyon, E., Perrine-Walker, F., Pervent, M., Rochette, J., Cuesta, C., Benkova, E., et al. (2016). Nitrate controls root development through posttranscriptional regulation of the NRT1.1/NPF6.3 transporter/sensor. Plant Physiol. 172, 1237–1248. doi: 10.1104/pp.16.01047
Bright, J., Desikan, R., Hancock, J., Weir, S., and Neill, S. (2006). ABA-induced NO generation and stomatal closure in Arabidopsis are dependent on H2O2 synthesis. Plant J. 45, 113–122. doi: 10.1111/j.1365-313X.2005.02615.x
Canales, J., Contreras-López, O., Álvarez, J. M., and Gutiérrez, R. A. (2017). Nitrate induction of root hair density is mediated by TGA1/TGA4 and CPC transcription factors in Arabidopsis thaliana. Plant J. 92, 305–316. doi: 10.1111/tpj.13656
Cao, Z., Duan, X., Yao, P., Cui, W., Cheng, D., Zhang, J., et al. (2017). Hydrogen gas is involved in auxin-induced lateral root formation by modulating nitric oxide synthesis. Int. J. Mol. Sci. 18:E2084. doi: 10.3390/ijms18102084
Chen, W., Yang, J., Qin, C., Jin, C., Mo, J., Ye, T., et al. (2010). Nitric oxide acts downstream of auxin to trigger root ferric-chelate reductase activity in response to iron deficiency in Arabidopsis. Plant Physiol. 154, 810–819. doi: 10.1104/pp.110.161109
Chen, Y., and Kao, C. (2012). Calcium is involved in nitric oxide- and auxin-induced lateral root formation in rice. Protoplasma 249, 187–195. doi: 10.1007/s00709-011-0277-2
Colón-Carmona, A., You, R., Haimovitch-Gal, T., and Doerner, P. (1999). Spatio-temporal analysis of mitotic activity with a labile cyclin-GUS fusion protein. Plant J. 20, 503–508. doi: 10.1046/j.1365-313x.1999.00620.x
Correa-Aragunde, N., Graziano, M., and Lamattina, L. (2004). Nitric oxide plays a central role in determining lateral root development in tomato. Planta 218, 900–905. doi: 10.1007/s00425-003-1172-7
Crawford, N. M. (2006). Mechanisms for nitric oxide synthesis in plants. J. Exp. Bot. 57, 471–478. doi: 10.1093/jxb/erj050
Drew, M., and Saker, L. (1975). Nutrient Supply and the Growth of the Seminal Root System in Barley II. Localized, compensatory increases in lateral root growth and rates of nitrate uptake when nitrate supply is restricted to only part of the root system. J. Exp. Bot. 26, 79–90. doi: 10.1038/srep18192
Duan, Y., Zhang, Y., Ye, L., Fan, X., Xu, G., and Shen, Q. (2007). Responses of rice cultivars with different nitrogen use efficiency to partial nitrate nutrition. Ann. Bot. 99, 1153–1160. doi: 10.1093/aob/mcm051
Fan, X., Jia, L., Li, Y., Smith, S. J., Miller, A. J., and Shen, Q. (2007). Comparing nitrate storage and remobilization in two rice cultivars that differ in their nitrogen use efficiency. J. Exp. Bot. 58, 1729–1740. doi: 10.1093/jxb/erm033
Feng, Q., Zhang, Y., Ha, P., Wang, S., Fu, G., Huang, Y., et al. (2002). Sequence and analysis of rice chromosome 4. Nature 420, 316–320. doi: 10.1038/nature01183
Fernández-Marcos, M., Sanz, L., Lewis, D. R., Muday, G. K., and Lorenzo, O. (2011). Nitric oxide causes root apical meristem defects and growth inhibition while reducing PIN-FORMED 1 (PIN1)-dependent acropetal auxin transport. Proc. Natl. Acad. Sci. U.S.A. 108, 18506–18511. doi: 10.1073/pnas.1108644108
Friml, J. (2003). Auxin transport-shaping the plant. Curr. Opin. Plant Biol. 6, 7–12. doi: 10.1016/S1369526602000031
Friml, J., Vieten, A., Sauer, M., Weijers, D., Schwarz, H., Hamann, T., et al. (2003). Efflux-dependent auxin gradients establish the apical–basal axis of Arabidopsis. Nature 426, 147–153. doi: 10.1038/nature02085
Gas, E., Flores-Pérez, U., Sauret-Güeto, S., and Rodríguez-Concepción, M. (2009). Hunting for plant nitric oxide synthase provides new evidence of a central role for plastids in nitric oxide metabolism. Plant Cell 21, 18–23. doi: 10.1105/tpc.108.065243
Giri, J., Bhosale, R., Huang, G., Pandey, B., Parker, H., Zappala, S., et al. (2018). The rice auxin influx carrier OsAUX1 facilitates root hair elongation in response to low external phosphate. Nat. Commun. 9:1408. doi: 10.1038/s41467-018-03850-4
Grieneisen, V., Xu, J., Marée, A., Hogeweg, P., and Scheres, B. (2007). Auxin transport is sufficient to generate a maximum and gradient guiding root growth. Nature 449, 1008–1013. doi: 10.1038/nature06215
Guo, F., and Crawford, N. (2005). Arabidopsis nitric oxide synthase1 is targeted to mitochondria and protects against oxidative damage and dark-induced senescence. Plant Cell 17, 3436–3450. doi: 10.1105/tpc.105.037770
Gupta, K. J., Fernie, A. R., Kaiser, W. M., and van Dongen, J. T. (2011). On the origins of nitric oxide. Trends Plant Sci. 16, 160–168. doi: 10.1016/j.tplants.2010.11.007
Huang, S., Chen, S., Liang, Z., Zhang, C., Yan, M., Chen, J., et al. (2015). Knockdown of the partner protein OsNAR2.1 for high-affinity nitrate transport represses lateral root formation in a nitrate-dependent manner. Sci. Rep. 5:18192. doi: 10.1038/srep18192
Jia, H., Ren, H., Gu, M., Zhao, J., Sun, S., Zhang, X., et al. (2011). The phosphate transporter gene OsPht1;8 is involved in phosphate homeostasis in rice. Plant Physiol. 156, 1164–1175. doi: 10.1104/pp.111.175240
Jia, L., Zhang, B., Mao, C., Li, J., Wu, Y., Wu, P., et al. (2008). OsCYTINV1 for alkaline/neutral invertase is involved in root cell development and reproductivity in rice (Oryza sativa L.). Planta 228, 51–59. doi: 10.1007/s00425-008-0718-0
Jin, C., Du, S., Shamsi, I., Luo, B., and Lin, X. (2011). NO synthase-generated NO acts downstream of auxin in regulating Fe-deficiency-induced root branching that enhances Fe-deficiency tolerance in tomato plants. J. Exp. Bot. 62, 3875–3884. doi: 10.1093/jxb/err078
Kan, Q., Wu, W., Yu, W., Zhang, J., Xu, J., Rengel, Z., et al. (2016). Nitrate reductase-mediated NO production enhances Cd accumulation in Panax notoginseng roots by affecting root cell wall properties. J. Plant Physiol. 193, 64–70. doi: 10.1016/j.jplph.2016.01.017
Kirk, G. J., and Kronzucker, H. J. (2005). The potential for nitrification and nitrate uptake in the rhizosphere of wetland plants: a modelling study. Ann. Bot. 96, 639–646. doi: 10.1093/aob/mci216
Kramer, E. M. (2004). PIN and AUX/LAX proteins: their role in auxin accumulation. Trends Plant Sci. 9, 578–582. doi: 10.1016/j.tplants.2004.10.010
Kronzucker, H. J., Glass, A. D. M., Siddiqi, M. Y., and Kirk, G. J. D. (2000). Comparative kinetic analysis of ammonium and nitrate acquisition by tropical lowland rice: implications for rice cultivation and yield potential. New Phytol. 145, 471–476. doi: 10.1046/j.1469-8137.2000.00606.x
Krouk, G., Lacombe, B., Bielach, A., Perrine-Walker, F., Malinska, K., Mounier, E., et al. (2010). Nitrate-regulated auxin transport by NRT1.1 defines a mechanism for nutrient sensing in plants. Dev. Cell 18, 927–937. doi: 10.1016/j.devcel.2010.05.008
Li, Y., Fan, X., and Shen, Q. (2008). The relationship between rhizosphere nitrification and nitrogen-use efficiency in rice plants. Plant Cell Environ. 31, 73–85. doi: 10.1111/j.1365-3040.2007.01737.x
Little, D. Y., Rao, H., Oliva, S., Daniel-Vedele, F., Krapp, A., and Malamy, J. E. (2005). The putative high-affinity nitrate transporter NRT2.1 represses lateral root initiation in response to nutritional cues. Proc. Natl. Acad. Sci. U.S.A. 102, 13693–13698. doi: 10.1073/pnas.0504219102
Liu, J., An, X., Cheng, L., Chen, F., Bao, J., Yuan, L., et al. (2010). Auxin transport in maize roots in response to localized nitrate supply. Ann. Bot. 106, 1019–1026. doi: 10.1093/aob/mcq202
Liu, Y., Lai, N., Gao, K., Chen, F., Yuan, L., and Mi, G. (2013). Ammonium inhibits primary root growth by reducing the length of meristem and elongation zone and decreasing elemental expansion rate in the root apex in Arabidopsis thaliana. PLoS One 8:e61031. doi: 10.1371/journal.pone.0061031
Lu, Y., Xu, Y., Shen, Q., and Dong, C. (2009). Effects of different nitrogen forms on the growth and cytokinin content in xylem sap of tomato (Lycopersicon esculentum Mill.) seedlings. Plant Soil. 315, 67–77. doi: 10.1007/s11104-008-9733-y
Manoli, A., Begheldo, M., Genre, A., Lanfranco, L., Trevisan, S., and Quaggiotti, S. (2014). NO homeostasis is a key regulator of early nitrate perception and root elongation in maize. J. Exp. Bot. 65, 185–200. doi: 10.1093/jxb/ert358
Manoli, A., Trevisan, S., Voigt, B., Yokawa, K., Baluška, F., and Quaggiotti, S. (2016). Nitric oxide-mediated maize root apex responses to nitrate are regulated by auxin and strigolactones. Front. Plant Sci. 6:1269. doi: 10.3389/fpls.2015.01269
Meyer, C., Lea, U., Provan, F., Kaiser, W., and Lillo, C. (2005). Is nitrate reductase a major player in the plant NO (nitric oxide) game? Photosynth. Res. 83, 181–189. doi: 10.1007/s11120-004-3548-3
Moreau, M., Lee, G. I., Wang, Y., Crane, B., and Klessig, D. (2008). At NOS/A1 is a function al Arabidopsis thaliana cGTPase and not a nitric oxide synthase. J. Biol. Chem. 283, 32957–32967. doi: 10.1093/jxb/ert358
Moreau, M., Lindermayr, C., Durner, J., and Klessig, D. (2010). NO synthesis and signaling in plants-where do we stand? Physiol. Plant. 138, 372–383. doi: 10.1111/j.1399-3054.2009.01308.x
Ogawa, T., Fukuoka, H., Yano, H., and Ohkawa, Y. (1999). Relationships between nitrite reductase activity and genotype-dependent callus growth in rice cell cultures. Plant Cell Rep. 18, 576–581. doi: 10.1007/s002990050625
Patterson, K., Walters, L., Cooper, A., Olvera, J., Rosas, M., Rasmusson, A., et al. (2016). Nitrate-regulated glutaredoxins control Arabidopsis primary root growth. Plant Physiol. 170, 989–999. doi: 10.1104/pp.15.01776
Peret, B., Swarup, K., Ferguson, A., Seth, M., Yang, Y., Dhondt, S., et al. (2012). AUX/LAX genes encode a family of auxin influx transporters that perform distinct functions during Arabidopsis development. Plant Cell 24, 2874–2885. doi: 10.1105/tpc.112.097766
Remans, T., Nacry, P., Pervent, M., Filleur, S., Diatloff, E., Mounier, E., et al. (2006). The Arabidopsis NRT1.1 transporter participates in the signaling pathway triggering root colonization of nitrate-rich patches. Proc. Natl. Acad. Sci. U.S.A. 103, 19206–19211. doi: 10.1073/pnas.0605275103
Sasaki, T., Matsumoto, T., and Yamamoto, K. (2002). The genome sequence and structure of rice chromosome 1. Nature 420, 312–316. doi: 10.1038/nature01184
Scheres, B., Benfey, P., and Dolan, L. (2002). Root devepopment. Arabidopsis Book 1:e0101. doi: 10.1199/tab.0101
Schlicht, M., Müller, J. L., Burbach, C., Volkmann, D., and Baluska, F. (2013). Indole-3-butyric acid induces lateral root formation via peroxisome-derived indole-3-acetic acid and nitric oxide. New Phytol. 200, 473–482. doi: 10.1111/nph.12377
Shahzad, Z., and Amtmann, A. (2017). Food for thought: how nutrients regulate root system architecture. Curr. Opin. Plant Biol. 39, 80–87. doi: 10.1016/j.pbi.2017.06.008
Song, W., Makeen, K., Wang, D., et al. (2011). Nitrate supply affects root growth differentially in two rice cultivars differing in nitrogen use efficiency. Plant Soil 343, 357–368. doi: 10.1007/s11104-011-0723-0
Song, W., Sun, H., Li, J., Gong, X., Huang, S., Zhu, X., et al. (2013). Auxin distribution is differentially affected by nitrate in roots of two rice cultivars differing in responsiveness to nitrogen. Ann. Bot. 112, 1383–1393. doi: 10.1093/aob/mct212
Stepanova, A., Yun, J., Robles, L., Novak, O., He, W., Guo, H., et al. (2011). The Arabidopsis YUCCA1 flavin monooxygenase functions in the indole-3-pyruvic acid branch of auxin biosynthesis. Plant Cell 23, 3961–3973. doi: 10.1105/tpc.111.088047
Stitt, M. (1999). Nitrate regulation of metabolism and growth. Curr. Opin. Plant Biol. 2, 178–186. doi: 10.1109/TMAG.2004.830229
Sun, C., Yu, J., and Hu, D. (2017). Nitrate: a crucial signal during lateral roots development. Front. Plant Sci. 8:485. doi: 10.3389/fpls.2017.00485
Sun, C. H., Yu, J. Q., Wen, L. Z., Guo, Y. H., Sun, X., Hao, Y. J., et al. (2018). Chrysanthemum MADS-box transcription factor CmANR1 modulates lateral root development via homo-/heterodimerization to influence auxin accumulation in Arabidopsis. Plant Sci. 266, 27–36. doi: 10.1016/j.plantsci
Sun, H., Bi, Y., Tao, J., Huang, S., Hou, M., Xue, R., et al. (2016). Strigolactones are required for nitric oxide to induce root elongation in response to nitrogen and phosphate deficiencies in rice. Plant Cell Environ. 39, 1473–1484. doi: 10.1111/pce.12709
Sun, H., Fan, F., Liu, J., and Zhao, Q. (2017). The interaction between auxin and nitric oxide regulates root growth in response to iron deficiency in rice. Front. Plant Sci. 8:2169. doi: 10.3389/fpls.2017.02169
Sun, H., Li, J., Song, W., Tao, J., Huang, S., Chen, S., et al. (2015). Nitric oxide generated by nitrate reductase increases nitrogen uptake capacity by inducing lateral root formation and inorganic nitrogen uptake under partial nitrate nutrition in rice. J. Exp. Bot. 66, 2449–2459. doi: 10.1093/jxb/erv030
Sun, H., Tao, J., Liu, S., Huang, S., Chen, S., Xie, X., et al. (2014). Strigolactones are involved in phosphate- and nitrate-deficiency-induced root development and auxin transport in rice. J. Exp. Bot. 65, 6735–6746. doi: 10.1093/jxb/eru029
Trevisan, S., Begheldo, M., Nonis, A., and Quaggiotti, S. (2012). The miRNA-mediated post-transcriptional regulation of maize response to nitrate. Plant Signal. Behav. 7, 822–826. doi: 10.4161/psb.20462
Trevisan, S., Manoli, A., Begheldo, M., Nonis, A., Enna, M., Vaccaro, S., et al. (2011). Transcriptome analysis reveals coordinated spatiotemporal regulation of hemoglobin and nitrate reductase in response to nitrate in maize roots. New Phytol. 192, 338–352. doi: 10.1111/j.1469-8137.2011.03822.x
Trevisan, S., Manoli, A., and Quaqqiotti, S. (2014). NO signaling is a key componet of root growth response to nitrate in Zea mays L. Plant Signal. Behav. 9:e28290. doi: 10.4161/psb.28290
Ulmasov, T., Murfett, J., Hagen, G., and Guilfoyle, T. (1997). Aux/IAAproteins repress expression of reporter genes containing natural and highly active synthetic auxin response elements. Plant Cell 9, 1963–1971. doi: 10.1016/j.cell.2010.09.027
Vanin, A., Svistunenko, D., Minkoyan, V., Serezhenkov, V., Fryer, M., Baker, N., et al. (2004). Endogenous superoxide production and the nitrite/nitrate ratio control the concentration of bioavailable free nitric oxide in leaves. J. Biol. Chem. 279, 24100–24107. doi: 10.1074/jbc.M312601200
Vanneste, S., and Friml, J. (2009). Auxin: a trigger for change in plant development. Cell 136, 1005–1016. doi: 10.1016/j.cell.2009.03.001
Vidal, E., Araus, V., Lu, C., Parry, G., Green, P., Coruzzi, G., et al. (2010). Nitrate-responsive miR393/AFB3 regulatory module controls root system architecture in Arabidopsis thaliana. Proc. Natl. Acad. Sci. U.S.A. 107, 4477–4482. doi: 10.1073/pnas.0909571107
Vidal, E. A., Moyano, T. C., Riveras, E., Contreras-Lopez, O., and Gutierrez, R. A. (2013). Systems approaches map regulatory networks downstream of the auxin receptor AFB3 in the nitrate response of Arabidopsis thaliana roots. Proc. Natl. Acad. Sci. U.S.A. 110, 12840–12845. doi: 10.1073/pnas.1310937110
Wang, J. R., Hu, H., Wang, G. H., Li, J., Chen, J. Y., and Wu, P. (2009). Expression of PIN genes in rice (Oryza sativa L.): tissue specificity and regulation by hormones. Mol. Plant 2, 823–831. doi: 10.1093/mp/ssp023
Wang, M., Siddeqi, M., Ruth, T., and Glass, A. (1993). Ammonium uptake by rice roots. I. Kinetics of 13NH4+ influx across the plasmalemma. Plant Physiol. 103, 1259–1267. doi: 10.1104/pp.103.4.1259
Wang, X., Wu, P., Xia, M., Wu, Z., Chen, Q., and Liu, F. (2002). Identification of genes enriched in rice roots of the local nitrate treatment and their expression patterns in split-root treatment. Gene 297, 93–102. doi: 10.1016/S0378-1119(02)00870-3
Wilson, I., Neill, S., and Hancock, J. (2008). Nitric oxide synthesis and signalling in plants. Plant Cell Environ. 31, 622–631. doi: 10.1111/j.1365-3040.2007.01761.x
Wisniewska, J., Xu, J., Seifertova, D., Brewer, P., Ruzicka, K., Blilou, I., et al. (2006). Polar PIN localization directs auxin flow in plants. Science 312, 883–883. doi: 10.1126/science.1121356
Yamasaki, H. (2005). The NO world for plants: achieving balance in an open system. Plant Cell Environ. 28, 78–84. doi: 10.1111/j.1365-3040.2005.01297.x
Yamasaki, H., Sakihama, Y., and Takahashi, S. (1999). An alternative pathway for nitric oxide production in plants: new features of an old enzyme. Trends Plant Sci. 4, 128–129. doi: 10.1016/S1360-1385(99)01393-X
Yan, Y., Wang, H., Hamera, S., Chen, X., and Fang, R. (2014). miR444a has multiple functions in rice nitrate-signaling pathway. Plant J. 78, 44–55. doi: 10.1111/tpj.12446
Yu, C., Su, S., Xu, Y., Zhao, Y., Yan, A., Huang, L., et al. (2014). The effects of fluctuations in the nutrient supply on the expression of five members of the AGL17 clade of MADS-box genes in rice. PLoS One 9:e105597. doi: 10.1371/journal.pone.0105597
Zazimalova, E., Murphy, A., Yang, H., Hoyerova, K., and Hosek, P. (2010). Auxin transporters-why so many? Cold Spring Harb. Perspect. Biol. 2:a001552. doi: 10.1101/cshperspect.a001552
Zhang, F., and Mi, G. (2005). Auxin transport from shoot to root is involved in the response of lateral root growth to localized supply of nitrate in maize. Plant Sci. 169, 894–900. doi: 10.1016/j.plantsci.2005.06.007
Zhang, H., and Forde, B. (1998). An Arabidopsis MADS box gene that controls nutrient-induced changes in root architecture. Science 279, 407–409. doi: 10.1126/science.279.5349.407
Zhang, H., Jennings, A., Barlow, P., and Forde, B. (1999). Dual pathways for regulation of root branching by nitrate. Proc. Natl. Acad. Sci. U.S.A. 96, 6529–6534. doi: 10.1073/pnas.96.11.6529
Zhao, M., Tai, H., Sun, S., Zhang, F., Xu, Y., and Li, W. (2012). Cloning and characterization of maize miRNAs involved in responses to nitrogen deficiency. PLoS One 7:e29669. doi: 10.1371/journal.pone.0029669
Zhao, M., Tian, Q., and Zhang, W. (2007). Nitric oxide synthase-dependent nitric oxide production is associated with salt tolerance in Arabidopsis. Plant Physiol. 144, 206–217. doi: 10.1104/pp.107.096842
Zhao, M. G., Chen, L., Zhang, L. L., and Zhang, W. H. (2009). Nitric reductase-dependent nitric oxide production is involved in cold acclimation and freezing tolerance in Arabidopsis. Plant Physiol. 151, 755–767. doi: 10.1104/pp.109.140996
Zhao, Y. (2012). Auxin biosynthesis: a simple two-step pathway converts tryptophan to indole-3-acetic acid in plants. Mol. Plant 5, 334–338. doi: 10.1093/mp/ssr104
Zhao, Y., Xu, Z., Mo, Q., Zou, C., Li, W., Xu, Y., et al. (2013). Combined small RNA and degradome sequencing reveals novel miRNAs and their targets in response to low nitrate availability in maize. Ann. Bot. 112, 633–642. doi: 10.1093/aob/mct133
Keywords: auxin, nitrate (NO3-), nitric oxide (NO), rice, root
Citation: Sun H, Feng F, Liu J and Zhao Q (2018) Nitric Oxide Affects Rice Root Growth by Regulating Auxin Transport Under Nitrate Supply. Front. Plant Sci. 9:659. doi: 10.3389/fpls.2018.00659
Received: 10 January 2018; Accepted: 30 April 2018;
Published: 23 May 2018.
Edited by:
Raul Antonio Sperotto, University of Taquari Valley, BrazilReviewed by:
Shu-Jen Wang, National Taiwan University, TaiwanJitender Giri, National Institute of Plant Genome Research (NIPGR), India
Copyright © 2018 Sun, Feng, Liu and Zhao. This is an open-access article distributed under the terms of the Creative Commons Attribution License (CC BY). The use, distribution or reproduction in other forums is permitted, provided the original author(s) and the copyright owner are credited and that the original publication in this journal is cited, in accordance with accepted academic practice. No use, distribution or reproduction is permitted which does not comply with these terms.
*Correspondence: Huwei Sun, c3VuaHV3ZWkxOTQzMUAxNjMuY29t Quanzhi Zhao, cXp6aGFvaEAxMjYuY29t
†These authors have contributed equally to this work.