- 1College of Biological Science and Biotechnology, Shenyang Agricultural University, Shenyang, China
- 2Department of Medicine, HE University School of Clinical Medicine, Shenyang, China
- 3Vegetable Research Institute of Liaoning Academy of Agricultural Sciences, Shenyang, China
Water stress has a major influence on plant growth, development, and productivity. However, the cross-talk networks involved in drought tolerance are not well understood. Arabidopsis PCaP2 is a plasma membrane-associated Ca2+-binding protein. In this study, we employ qRT-PCR and β-glucuronidase (GUS) histochemical staining to demonstrate that PCaP2 expression was strongly induced in roots, cotyledons, true leaves, lateral roots, and whole plants under water deficit conditions. Compared with the wild type (WT) plants, PCaP2-overexpressing (PCaP2-OE) plants displayed enhanced water deficit tolerance in terms of seed germination, seedling growth, and plant survival status. On the contrary, PCaP2 mutation and reduction via PCaP2-RNAi rendered plants more sensitive to water deficit. Furthermore, PCaP2-RNAi and pcap2 seedlings showed shorter root hairs and lower relative water content compared to WT under normal conditions and these phenotypes were exacerbated under water deficit. Additionally, the expression of PCaP2 was strongly induced by exogenous abscisic acid (ABA) and salicylic acid (SA) treatments. PCaP2-OE plants showed insensitive to exogenous ABA and SA treatments, in contrast to the susceptible phenotypes of pcap2 and PCaP2-RNAi. It is well-known that SNF1-related kinase 2s (SnRK2s) and pathogenesis-related (PRs) are major factors that influence plant drought tolerance by ABA- and SA-mediated pathways, respectively. Interestingly, PCaP2 positively regulated the expression of drought-inducible genes (RD29A, KIN1, and KIN2), ABA-mediated drought responsive genes (SnRK2.2, -2.3, -2.6, ABF1, -2, -3, -4), and SA-mediated drought responsive genes (PR1, -2, -5) under water deficit, ABA, or SA treatments. Taken together, our results showed that PCaP2 plays an important and positive role in Arabidopsis water deficit tolerance by involving in response to both ABA and SA signals and regulating root hair growth. This study provides novel insights into the underlying cross-talk mechanisms of plants in response to water deficit stress.
Introduction
Water deficit is one of the most acute abiotic stresses affecting plant growth and the economic yield of crop plants. It leads to alterations in various cellular processes in plants, for example, gene expression, photosynthesis, protein synthesis, carbon partitioning, lipid metabolism, and osmotic homeostasis (Hua et al., 2012; Jarzyniak and Jasiński, 2014; Fleta-Soriano and Munné-Bosch, 2016). Phytohormones, such as abscisic acid (ABA), salicylic acid (SA), gibberellin (GA), indole-3-acetic acid (IAA), and jasmonic acid (JA) function as central factors that link and reprogram these complex cellular processes (Hua et al., 2012).
In response to water deficit, ABA, a well-known stress phytohormone, is rapidly induced, leading to the expression of stress-responsive genes and the activation of plants’ cellular physiological adaptation to water stress (Fujii and Zhu, 2009; Cutler et al., 2010; Weiner et al., 2010). In the ABA signaling pathway, SNF1-related kinase 2s (SnRK2s) are central regulators that mediate ABA-responsive transcription factors and genes to activate ABA-mediated physiological processes (Yoshida et al., 2002; Boudsocq et al., 2004; Fujita et al., 2009; Umezawa, 2009; Vlad et al., 2009; Raghavendra et al., 2010; Kulik et al., 2011; Ambrosone et al., 2015).
Among the 10 SnRK2s in Arabidopsis, SnRK2.2, SnRK2.3, and SnRK2.6 function as central regulators in response to ABA and drought. Genetic analysis has shown that the Arabidopsis triple mutant snrk2.2/snrk2.3/snrk2.6 exhibits greatly reduced water deficit tolerance and is extremely insensitive to ABA (Fujii and Zhu, 2009; Fujita et al., 2009). The triple mutant is strongly impaired in ABA- and drought-responsive genes expression under water stress (Fujita et al., 2009). The phenotype of the triple mutant indicates that these three SnRK2s are partially redundant, although all of them are crucial for plants’ response to water stress and ABA, as well as ABA-mediated seed germination and dormancy. Seed dormancy, germination, and seedling growth of snrk2.2/snrk2.3 mutants are greatly insensitive to exogenous ABA. In contrast, snrk2.6 shows a significant increase in leaf water loss after ABA treatment (Yoshida et al., 2002; Fujii et al., 2007; Nakashima et al., 2009). This could be because SnRK2.6/ OST1 are important for stomatal movements, as they phosphorylate anion (SLAC1) and cation (KAT1) channels, which might be required for ABA-dependent stomatal closing in response to water deficit (Pilot et al., 2001; Geiger et al., 2009, 2010; Lee et al., 2009; Sato et al., 2009). In addition, guard cells (GCs) display transcriptional memory in a daily dehydration stress and watered recovery cycle. SnRK2.2, SnRK2.3, and SnRK2.6 have distinguishable roles in the process: SnRK2.2 and SnRK2.3 are more important for implementing guard cell stress memory, while SnRK2.6 is more important for overall stomatal control in the subsequent dehydration response (Virlouvet and Fromm, 2015). It has been found that SnRK2.2, -2.3, and 2.6 are regulated by nitric oxide (NO), phosphatidic acid (PA), and Ca2+ changes (Boudsocq et al., 2004; Fujii and Zhu, 2009; Kulik et al., 2011), suggesting SnRK2s may be regulated by complex pathways during water stress; however, such mechanisms remain largely unknown.
The phytohormone SA plays an important role in various plant developmental processes and responses to abiotic and biotic stress (Raskin, 1992; Bandurska and Stroiński, 2005; Khan et al., 2012a,b, 2013). Water deficit induces increased endogenous SA levels in various plants (Munne-Bosch and Penuelas, 2003; Miura and Tada, 2014). Exogenous treatment with SA modulates plant drought resistance through multiple pathways such as oxidative stress (Alam et al., 2013), stomatal conductance (Hao et al., 2010; Khokon et al., 2011; Habibi, 2012), antioxidant defense system (Hayat et al., 2008; Saruhan et al., 2012), and NO production (Hao et al., 2010; Khokon et al., 2011). Additionally, some SA-responsive genes are involved in plant response to water deficit, such as GST1, GST2, GR, and MDHAR in Triticum aestivum (Kang et al., 2013) and MPK3, MPK4, MPK6, PR1, PR2, and PR5 in Arabidopsis thaliana (Ichimura et al., 2000; Ahlfors et al., 2004; Gudesblat et al., 2007; Liu P. et al., 2013; Liu W.X. et al., 2013). Some Arabidopsis mutants that accumulate endogenous SA (adr1, acd6, cpr5 myb96-1d, and siz1) show both SA-mediated disease resistance and water deficit tolerance (Miura et al., 2013). One genetic analysis reports that Arabidopsis seedlings overexpressing PR1, PR2, or PR5 are drought tolerant (Liu W.X. et al., 2013). In addition, PR1, -2, and -5 genes are widely used as marker genes for SA-mediated drought tolerance in plants. For example, both SA-accumulating mutants (cpr5 and acd6) and overexpression of transcription factor Di19 in Arabidopsis improve drought tolerance via SA-induced expression of PR1, -2, -5 genes (Liu P. et al., 2013; Liu W.X. et al., 2013). Interestingly, SA treatments lead to an increase of ABA and proline in the barley leaves (Bandurska and Stroiński, 2005); however, the relationship between SA and ABA signals in water deficit remains unknown.
Arabidopsis microtubule-associated protein-18/plasma membrane-associated Ca2+-binding protein-2 (MAP18/PCaP2) is important for several physiological activities. For example, it is involved in Ca2+ binding and the organization of cortical microtubules (MTs) and F-actin. It also has a critical role in root hair, pollen tube growth, and directional cell growth (Wang et al., 2007; Kato et al., 2010, 2013; Zhu et al., 2013; Zhang et al., 2015; Kang et al., 2017). For example, the cell polarity and cortical microtubule array in line 2 of MAP18-overexpressing Arabidopsis (OE2) and line 18 of MAP18 RNAi transgenic Arabidopsis (R18) are altered (Wang et al., 2007). The T-DNA insertion line map18 (SALK_021652), which is confirmed as a knock-down mutant by qRT-PCR analysis, displays abnormal pollen tube growth and root hair growth (Kato et al., 2013; Zhu et al., 2013; Zhang et al., 2015; Kang et al., 2017).
Interestingly, the mRNA expression level of PCaP2 is induced by heat, cold, drought, ABA, SA, osmotic stress, and GA3 (Kato et al., 2010, 2013). This implies that it may function in response to abiotic stress and phytohormone signals. Root hairs are the main sites of water absorption in plants, which is important for water deficit tolerance (Worrall and Roughley, 1976; Zahran and Sprent, 1986; Schnall and Quatrano, 1992). Thus, we hypothesized that PCaP2 might be an important regulator of plant water deficit tolerance in various pathways, such as phytohormone signals and root hair growth, suggesting that PCaP2 might be a cross-talker between complex mechanisms involved in plant water deficit tolerance. In this study, we found that PCaP2 is vital for plant water deficit tolerance by responding to ABA and SA signals, regulating the expression of the key ABA- and SA-mediated genes, and affecting root hair growth. Collectively, our data provide novel evidence of the underlying complex mechanisms, especially of crosstalk between ABA and SA signaling pathways in plant water deficit tolerance.
Results
The Expression of PCaP2 Is Highly Induced in All Tissues in Water Deficit
To fully understand the expression pattern of PCaP2 under water deficit stress, we examined the expression of PCaP2 in more details by quantitative real-time PCR (qRT-PCR) and β-glucuronidase (GUS) staining. Firstly, the wild type (WT) plants were exposed to dehydration conditions for 1, 3, 6, 9, and 12 h. The qRT-PCR results showed that PCaP2 expression was highly induced by water deficit treatments from 1 to 12 h, with the peak level of 10-fold increased at 6 h treatment (Figure 1A). Furthermore, GUS staining showed that the promoter activity of PCaP2 was significantly increased after water deficit treatments for 6 h which was consistent with the results of qRT-PCR (Figure 1B) and PCaP2 expression was induced in primary roots and lateral roots, cotyledons, true leaves, and the whole seedlings after water deficit treatments (Figures 1B–D).
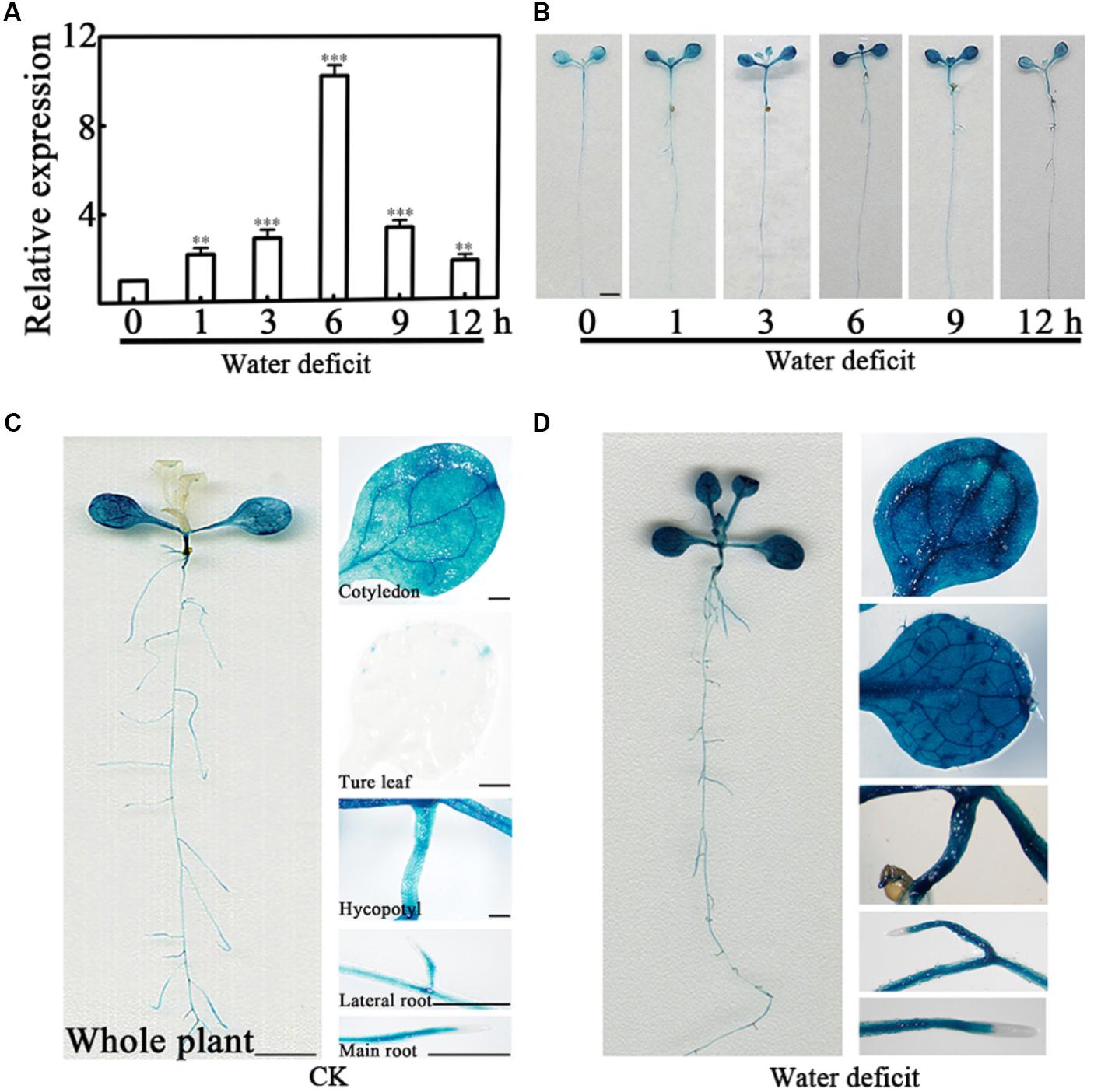
FIGURE 1. Expression pattern of PCaP2 under water deficit stress. (A) Relative expression of PCaP2 in response to water deficit. Fourteen-day-old WT seedlings were treated with water deficit for 0, 1, 3, 6, 9, and 12 h, and the expression of PCaP2 was detected by qRT-PCR. Data represent mean values of three biological replicates ± SE. The significant difference was determined by ANOVA in comparison to 0 h: ∗∗P < 0.01, ∗∗∗P < 0.001. (B) Comparison of PCaP2 expression levels treated with water deficit different times by GUS staining. Seven-day-old seedlings of pPCaP2::GUS transgenic plants were treated with water deficit for 0, 1, 3, 6, 9, and 12 h. Scale bar = 2.5 mm. (C, D) Analysis of the expression pattern of PCaP2 by GUS staining of pPCaP2::GUS transgenic seedlings in normal (without water deficit treatment) (C) and water deficit treatments for 6 h (D). At least 15 seedlings from each sample were used for every technical replicate and three biological replicates were conducted. The scale bar is 5 mm in the pictures of whole plant, and 0.25 mm in the pictures of cotyledons, true leaves, hypocotyls, lateral roots and main roots.
PCaP2-OE Plants Display Increased Tolerance While PCaP2 RNAi and Mutant Seedlings Are Hypersensitive in Response to Water Deficit
To elucidate the function of PCaP2 in plant tolerance to water deficit, the previous identified one PCaP2 overexpression (PCaP2-OE) line (Wang et al., 2007), one knockdown of T-DNA insertion (pcap2) line (SALK_021652; Kato et al., 2013; Zhu et al., 2013; Zhang et al., 2015; Kang et al., 2017), and one fully silenced PCaP2 RNA interference (PCaP2-RNAi) line (Wang et al., 2007) were used. The PCaP2 expression of these lines was analyzed by qRT-PCR which is consistent with the previous publications (Supplementary Figure S1). We firstly investigated their seed germination rates under normal or drought conditions. Under normal conditions, germination rates of PCaP2-RNAi and pcap2 seeds were lower than those of PCaP2-OE and WT seeds at 1 day, and then these seeds gradually showed similar germination rate. At 4 and 5 days, the germination of these seeds was identical (Figure 2A). Water deficit significantly inhibited the germination of all seeds at 1 day, germination rates of PCaP2-RNAi were lower from 1 to 4 days, then the germination of these seeds was identical germination at 5 days (Figure 2A).
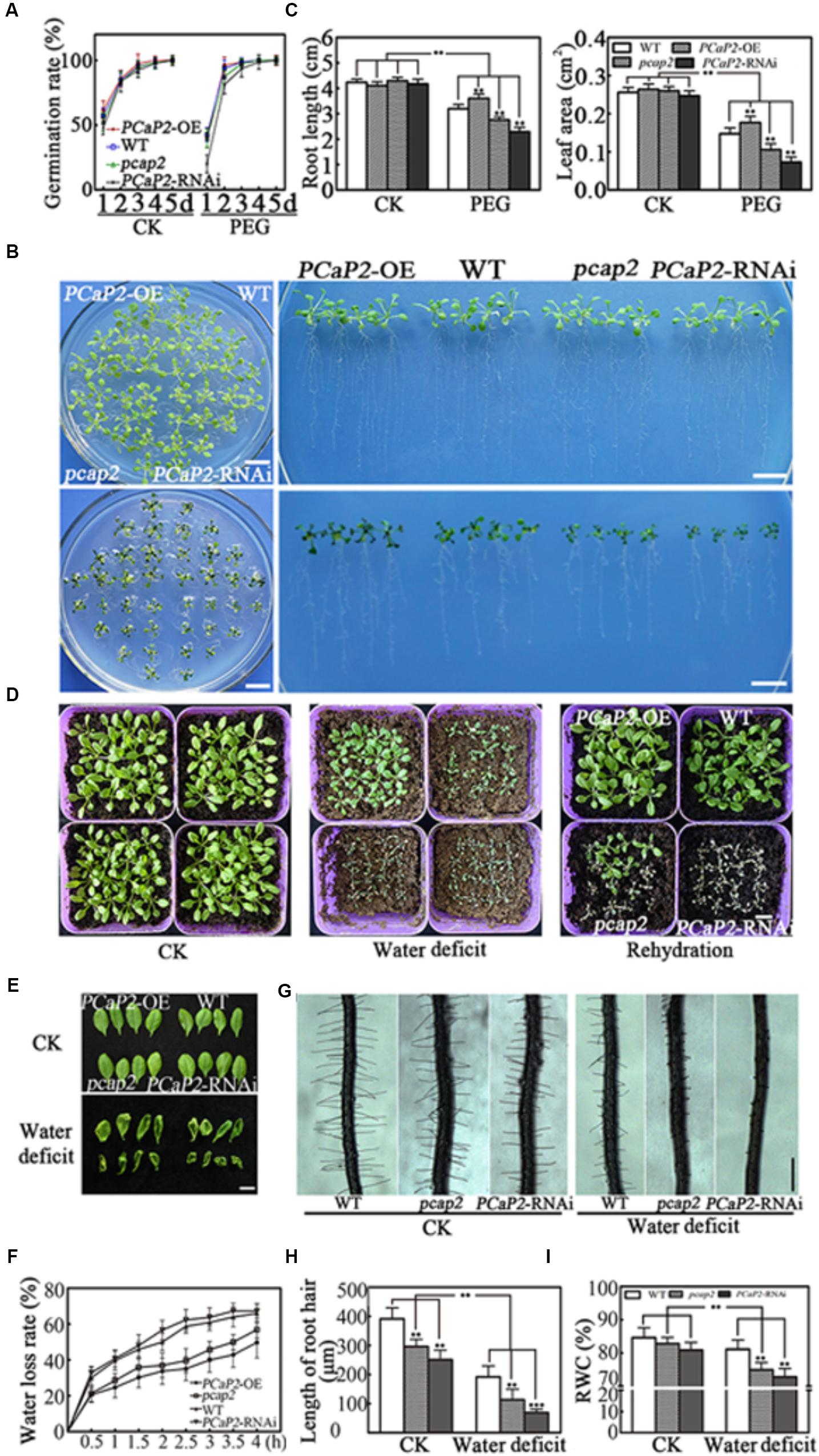
FIGURE 2. Effects of PCaP2 on Arabidopsis seed germination and seedling growth under water deficit stress. (A) Quantification of germination rates of the PCaP2-OE, WT, pcap2 and PCaP2-RNAi seeds in 1/2 MS medium or coated with 25 % PEG from 1 to 5 d. At least 50 seeds from each sample were used for every technical replicate and three biological replicates were conducted. (B) Phenotypes of the PCaP2-OE, WT, pcap2 and PCaP2-RNAi seedlings grown in water deficit. Scale bar = 1 cm. (C) The phenotypes statistics of the PCaP2-OE, WT, pcap2 and PCaP2-RNAi seedlings grown in water deficit. At least 70 roots or 200 leaves from about 70 seedlings from each sample were used for every technical replicate and three biological replicates were conducted. (D) The survival status of PCaP2-OE, WT, pcap2 and PCaP2-RNAi in normal, water deficit and rehydration matrix soil, respectively. Scale bar = 1 cm. (E) Phenotypes of leaves wilted of PCaP2-OE, WT, pcap2 and PCaP2-RNAi plants for 0 h (up) and 4 h (down) under dehydration stress. Scale bar = 1 cm. (F) Water loss measurements for genotypes. Water loss in detached leaves was measured at the time points indicated. Water loss was expressed as a percentage of initial fresh weight. At least 30 detached leaves from each sample were used for every technical replicate and three biological replicates were conducted. (G) Phenotypes of root hairs in WT, pcap2, and PCaP2-RNAi lines in 1/2 MS or coated with 15% PEG. Scale bar = 0.5 mm. (H) The statistics of average root hair length in (G). About 250 root hairs from 30 roots of each sample were used for every technical replicate and three biological replicates were conducted. (I) The relative water content (RWC) of WT, pcap2 and PCaP2-RNAi lines in 1/2MS or coated with 15% PEG. About 30 seedlings from each sample were used for every technical replicate and three biological replicates were conducted. All data are mean values of three biological replicates ± SE. The significant difference was determined by ANOVA: ∗P < 0.05, ∗∗P < 0.01, ∗∗∗P < 0.001.
Next, the growth and survival status of the four-day-old seedlings were observed in water deficit treatments. It was found that the grown status showed no significant differences among WT, PCaP2-OE, pcap2, and PCaP2-RNAi lines under normal conditions. The PCaP2-OE seedlings showed longer roots and larger leaves than WT while pcap2 and PCaP2-RNAi showed opposite growth phenotypes under water deficit (Figures 2B,C). The results of survival status after water deficit treatments showed that PCaP2-OE plants were more tolerant in response to water deficit than WT (Figures 2C,D) while pcap2 and PCaP2-RNAi plants exhibited sensitivity to water deficit. To determine recovery after water deficit, we re-watered these seedlings for 7 days. The results showed that PCaP2-OE plants, WT, and some pcap2 seedlings were recovered while all PCaP2-RNAi seedlings exhibited water deficit sensitivity (Figure 2D). The changes of leaf water loss and root water absorption in WT, PCaP2-OE, pcap2, and PCaP2-RNAi lines under water deficit conditions were observed. The results showed that pcap2 and PCaP2-RNAi lines lost water faster and wilted earlier than WT in dehydration stress while PCaP2-OE seedlings showed opposite phenotypes (Figures 2E,F). Additionally, the pcap2 and PCaP2-RNAi lines displayed shorter root hairs and less relative water content than WT in normal condition, the phenotypes were more significant in water deficit stress (Figures 2G–I). These results indicated that overexpression of PCaP2 enhanced the tolerance of plants to water deficit and down of PCaP2 led to plant water deficit hypersensitivity.
The PCaP2 Expression Is Highly Induced in Response to Exogenous ABA and SA Treatments
Abscisic acid and SA are important regulatory signals in water deficit stress, and the higher expression level of PCaP2 has been found in exogenous 100 μM ABA and 100 μM SA treatments (Kato et al., 2010; Trivedi et al., 2016). To determine PCaP2 in ABA and SA signaling pathways, the expression of PCaP2 was examined in exogenous ABA and SA treatments by qRT-PCR and GUS staining assay in more details. Because the expression of many genes in Arabidopsis can be induced by 10–100 μM ABA (Zhu et al., 2007, 2017; Kato et al., 2010; Tian et al., 2015) and we found the expression of PCaP2 is higher in 40 μM ABA treatments than that in 100 μM ABA treatment (Figure 3A; Kato et al., 2010). Thus, 40 μM ABA and 100 μM SA treatments were used in our studies. The results showed that the PCaP2 mRNA level was significantly increased with ABA and SA treatments from 1 to 12 h, the peaks appeared at 6 h ABA and 3 h SA treatments (Figures 3A,B). The expression level of PCaP2 was higher in 40 μM ABA than 100 μM SA treatments (Figures 3A,B). Further, the PCaP2 expression was induced in the whole seedlings, cotyledons, true leaves, hypocotyls, primary roots, and lateral roots by ABA and SA treatments (Figures 3B–E), which were consistent with the results from water deficit treatments.
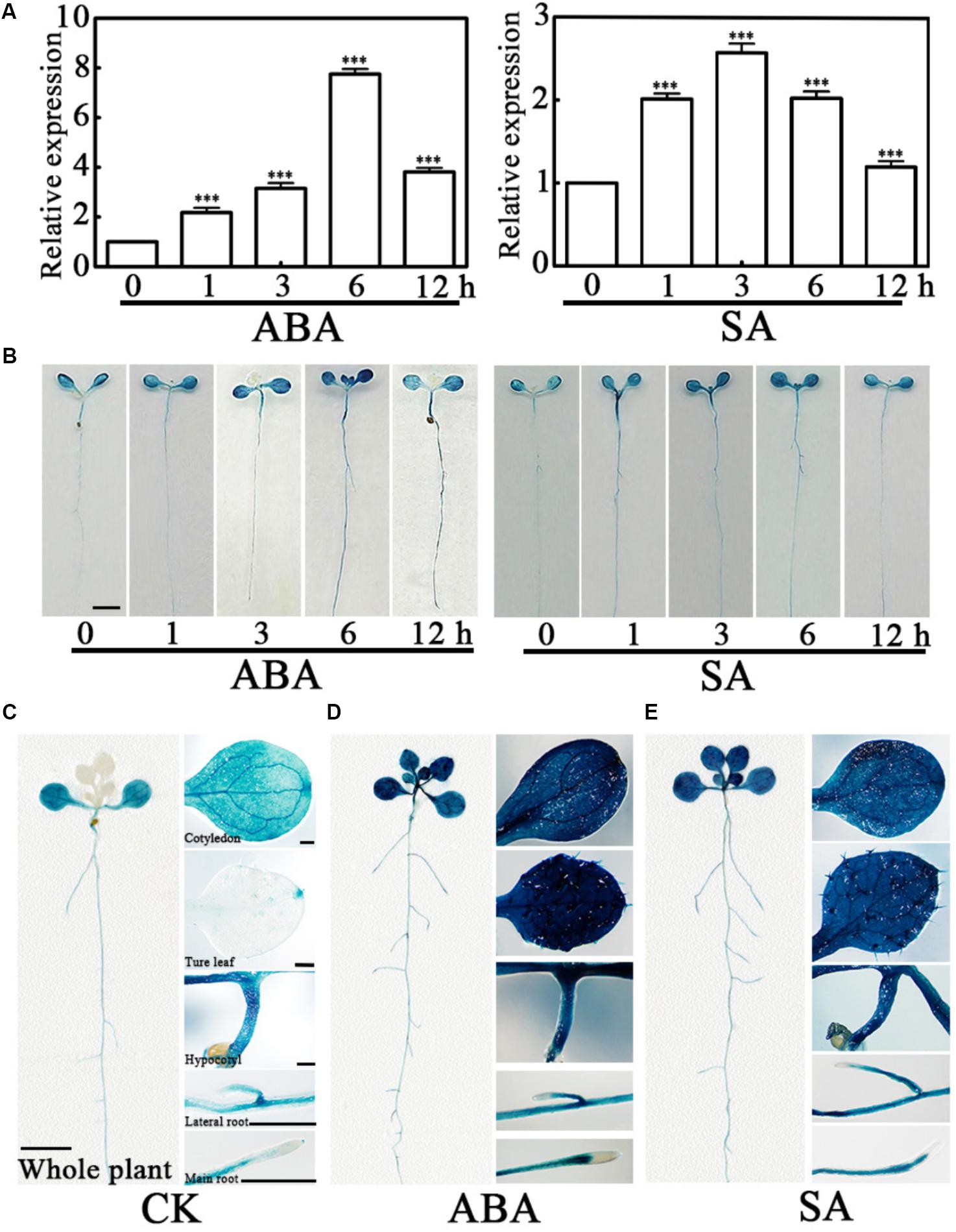
FIGURE 3. Expression pattern of PCaP2 with exogenous ABA or SA treatments. (A) Relative expression of PCaP2 in response to exogenous ABA and SA treatments. Fourteen-day-old seedlings were treated with 40 μM ABA or 100 μM SA for 0, 1, 3, 6, and 12 h by qRT-PCR analysis. Data represent mean values of three biological replicates ± SE. The significant difference was determined by ANOVA in comparison to 0 h: ∗∗∗P < 0.001. (B) Comparison of PCaP2 expression levels with exogenous ABA or SA treatments by GUS staining. Seven-day-old seedlings of pPCaP2::GUS transgenic plants were treated with 40 μM ABA or 100 μM SA for 0, 1, 3, 6, and 12 h. Scale bar = 2.5 mm. (C–E) Analysis of the expression pattern of PCaP2 by GUS staining of pPCaP2::GUS transgenic seedlings in normal (without ABA or SA treatments) (C) and 40 μM ABA treatments for 6 h (D) and 100 μM SA treatments for 6 h (E). At least 15 seedlings from each sample were used for every technical replicate and three biological replicates were conducted. The scale bar is 5 mm in the pictures of whole plants, and 0.25 mm in the pictures of cotyledons, true leaves, hypocotyls, lateral roots, and main roots.
PCaP2-OE Plants Show Increased Tolerance, While Its RNAi and Mutant Are More Sensitive in Exogenous ABA and SA Treatments
Then we tested the germination rate of seeds and seedling growth of WT, PCaP2-OE, pcap2, and PCaP2-RNAi lines under normal, ABA, and SA treatments. To choose the most appropriate concentrations for ABA and SA treatments, 0.01–40 μM of ABA and SA were tested in the previous experiments used (data not shown). The results showed WT and PCaP2-OE showed the similar germination rates. The PCaP2-RNAi and pcap2 showed much higher germination rates in 0.8 μM ABA and 0.3 mM SA treatments, compared to WT and PCaP2-OE (Figure 4A). The germination rates of PCaP2-RNAi and pcap2 seeds were lower than those of PCaP2-OE and WT seeds in 1-to-5-day ABA and in 1-to-3-day SA treatments. The germination rates of these seeds were similar at day 4 of SA treatment and identical at day 5 of SA treatment. The growth status of WT, PCaP2-OE, pcap2, and PCaP2-RNAi lines was significantly different under 0.5 μM. ABA and 0.05 mM SA treatments (Figures 4B,C). The PCaP2-OE seedlings showed larger leaf area and longer primary roots than WT while pcap2 and PCaP2-RNAi lines showed smaller leaves and shorter roots and root hairs, which were consistent with the water deficit inducible phenotypes (Figures 4B,C).
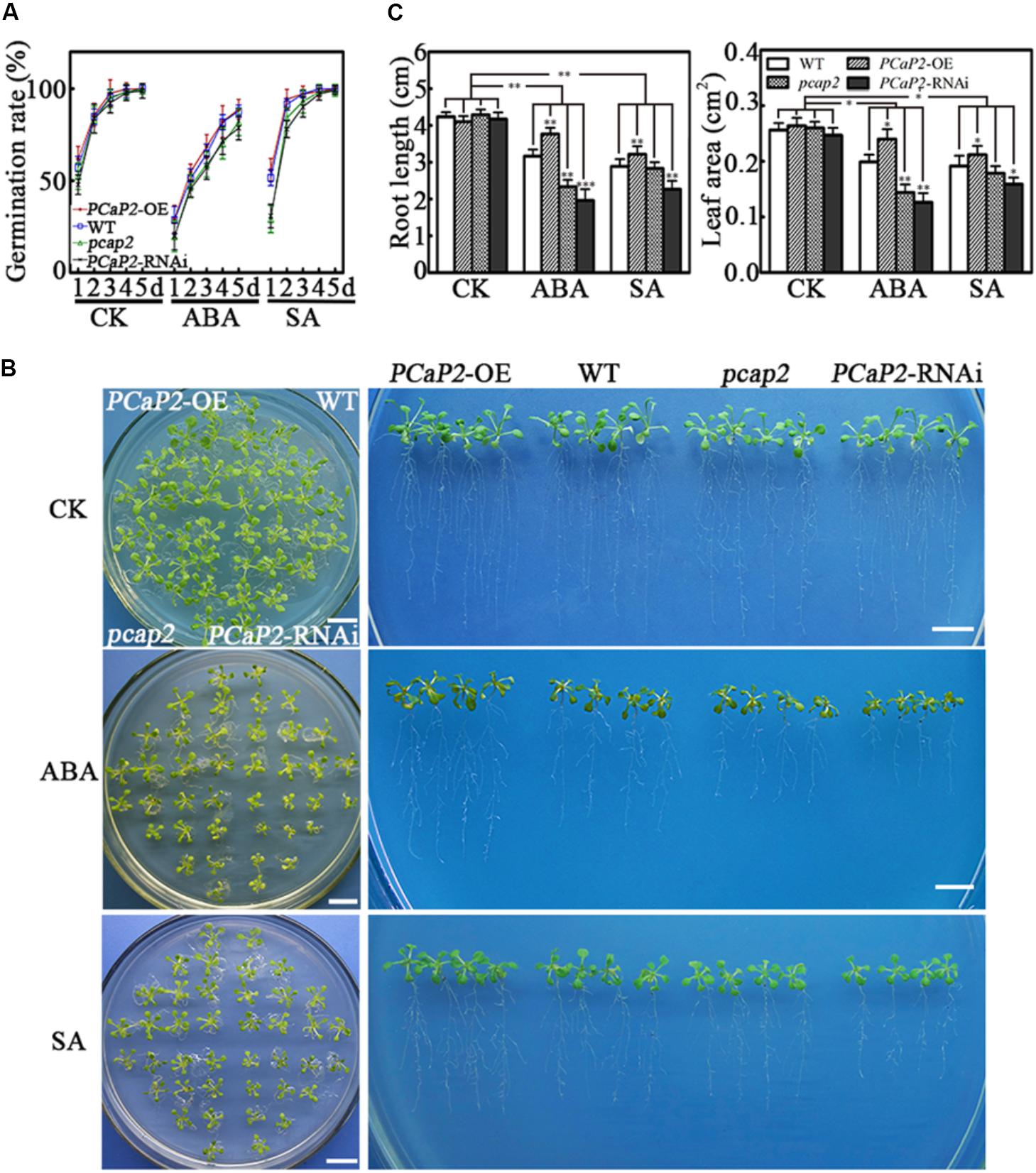
FIGURE 4. Effects of PCaP2 on Arabidopsis seed germination and seedling growth in ABA or SA treatments. (A) Quantification of germination rates of the PCaP2-OE, WT, pcap2, and PCaP2-RNAi seeds in 1/2 MS medium or mixed with 0.8 μM ABA and 0.3 mM SA from 1 to 5 days. A total of 40 seeds of each line were used for every technical replicate and three biological replicates were conducted. (B) Phenotypes of the PCaP2-OE, WT, pcap2, and PCaP2-RNAi seedlings grown in exogenous ABA and SA treatments. The seedlings were sown on 1/2 MS medium for 5 days, then transferred to 0.5 μM ABA or 0.05 mM SA medium and grew for 10 days. Scale bar = 1 cm. (C) The phenotype statistics of the PCaP2-OE, WT, pcap2, and PCaP2-RNAi seedlings grown in exogenous ABA and SA treatments. The main root length and leaf area of these seedlings were calculated after growth on 1/2 MS medium or supplemented with 0.5 μM ABA and 0.05 mM SA. At least 70 roots or 200 leaves from about 70 seedlings from each sample were used for every technical replicate and three biological replicates were conducted. All data are mean values of three biological replicates ± SE. The significant difference was determined by ANOVA: ∗P < 0.05, ∗∗P < 0.01, ∗∗∗P < 0.001.
PCaP2 Positively Regulates the Expression of SnRK2 Genes and PR Genes Under Water Deficit Stress
To analyze whether the role of PCaP2 in water deficit tolerance was mediated by ABA and SA signaling pathways, we firstly checked the PCaP2 expression of WT, PCaP2-OE, pcap2, and PCaP2-RNAi in water deficit conditions by qRT-PCR. The analysis showed that the increasing expression of PCaP2 in PCaP2-OE and the decreasing expression in pcap2 and PCaP2-RNAi were compared with that in WT under water deficit treatments (Supplementary Figure S1).
Next, we examined the expression of the following ABA-responsive genes, including SnRK2.2, -2.3, -2.6, ABF2, -3, -4 (Choi et al., 2000; Uno et al., 2000; Boudsocq et al., 2004; Gong et al., 2015; Jia et al., 2015); drought-inducible genes, including RD29A (Yamaguchi-Shinozaki and Shinozaki, 1994); KIN1 and KIN2 (Kurkela and Borg-Franck, 1992); and SA-responsive genes such as PR1, -2, -5 genes (Liu P. et al., 2013; Liu W.X. et al., 2013) in WT, PCaP2-OE, pcap2 and PCaP2-RNAi with dehydration conditions at 0, 6, and 12 h by qRT-PCR analysis. Under normal conditions, pcap2 and PCaP2-RNAi inhibited the expression of ABA- and drought-responsive genes, except SA-responsive genes (Figure 5), and PCaP2-OE increased the expression of SA-responsive genes, suggesting that PCaP2 might be the complex mechanisms in regulating gene expression in normal conditions. Under water deficit stress, the expression of all these genes, including ABA-, drought-, and SA-responsive genes, was significantly inhibited in pcap2 and PCaP2-RNAi while increased in PCaP2-OE, compared with that in WT, indicating that PCaP2 was involved in regulating drought-, ABA- and SA-responsive genes under water deficit stress.
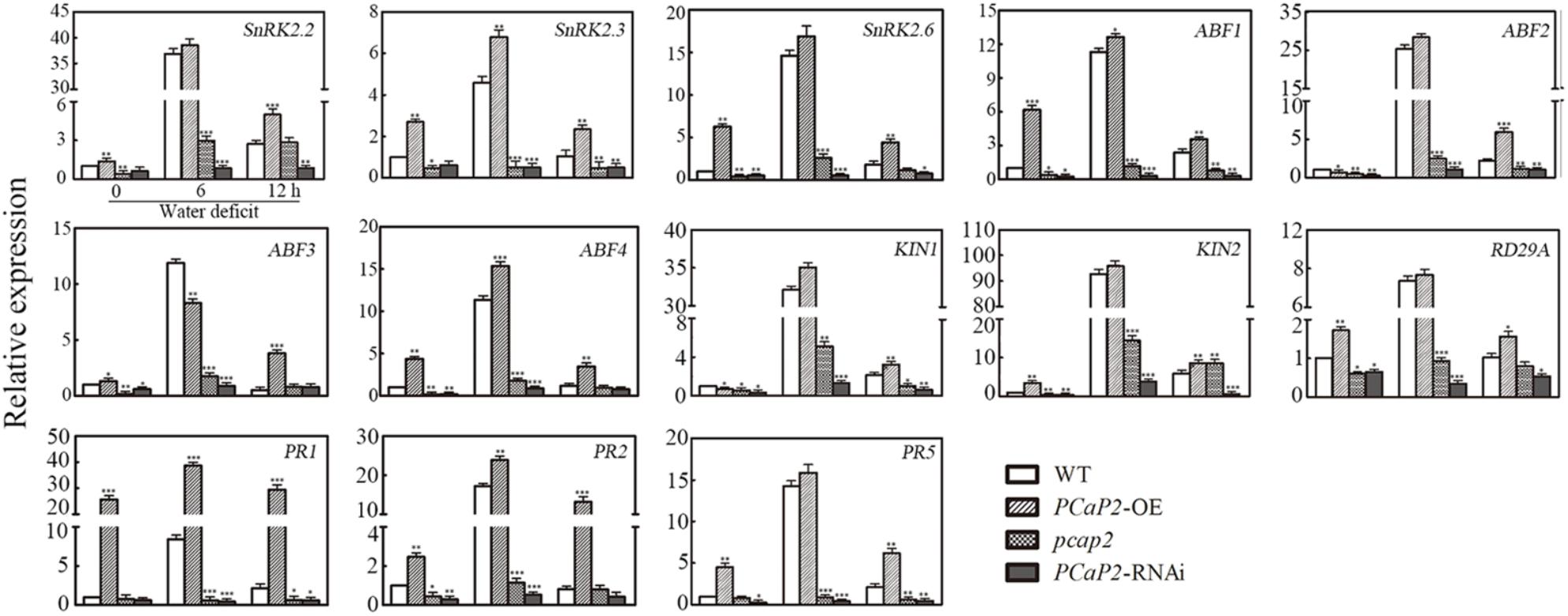
FIGURE 5. Effect of PCaP2 on the expression of ABA-, drought- and SA-responsive genes under water deficit. The relative gene expression of the 14-day-old seedlings of WT, PCaP2-OE, pcap2, and PCaP2-RNAi with water deficit treatments for 0, 6, and 12 h was determined by qRT-PCR analysis. Data represent mean values of three biological replicates ± SE. The significant difference was determined by ANOVA: ∗P < 0.05, ∗∗P < 0.01, ∗∗∗P < 0.001.
PCaP2 Positively Regulates ABA-Mediated SnRK2s Expression and SA-Mediated PRs Expression in Water Deficit
To further analyze whether the effect PCaP2 on gene expression in response to water deficit was mediated by ABA and SA signaling pathways, we firstly checked the PCaP2 expression of WT, PCaP2-OE, pcap2 and PCaP2-RNAi in ABA and SA treatments by qRT-PCR. The analysis showed that the increase expression of PCaP2 in PCaP2-OE and the decreasing expression in pcap2 and PCaP2-RNAi were compared with that in WT under ABA and SA treatments (Supplementary Figure S1). Next, we examined the expression of SnRK2.2, -2.3, -2.6 and PR1, -2, -5 genes in WT, PCaP2-OE, pcap2, and PCaP2-RNAi with ABA and SA treatments at 0, 6, and 12 h by qRT-PCR analysis. The results showed that the effect of PCaP2-OE, pcap2, and PCaP2-RNAi on affecting the ABA-responsive gene expression in ABA treatments, rather than SA treatments, were similar to that in drought treatments, (Figure 6A). In contrast, the effect of PCaP2-OE, pcap2, and PCaP2-RNAi on affecting the SA-responsive gene expression in SA treatments, rather than ABA treatments, were similar to that in drought treatments (Figure 6B). These results illustrated that PCaP2 regulated the expression of ABA-mediated SnRK2 genes and SA-mediated PR genes under water deficit stress, indicating that PCaP2 was a crosstalk factor in response to ABA and SA signals in water deficit.
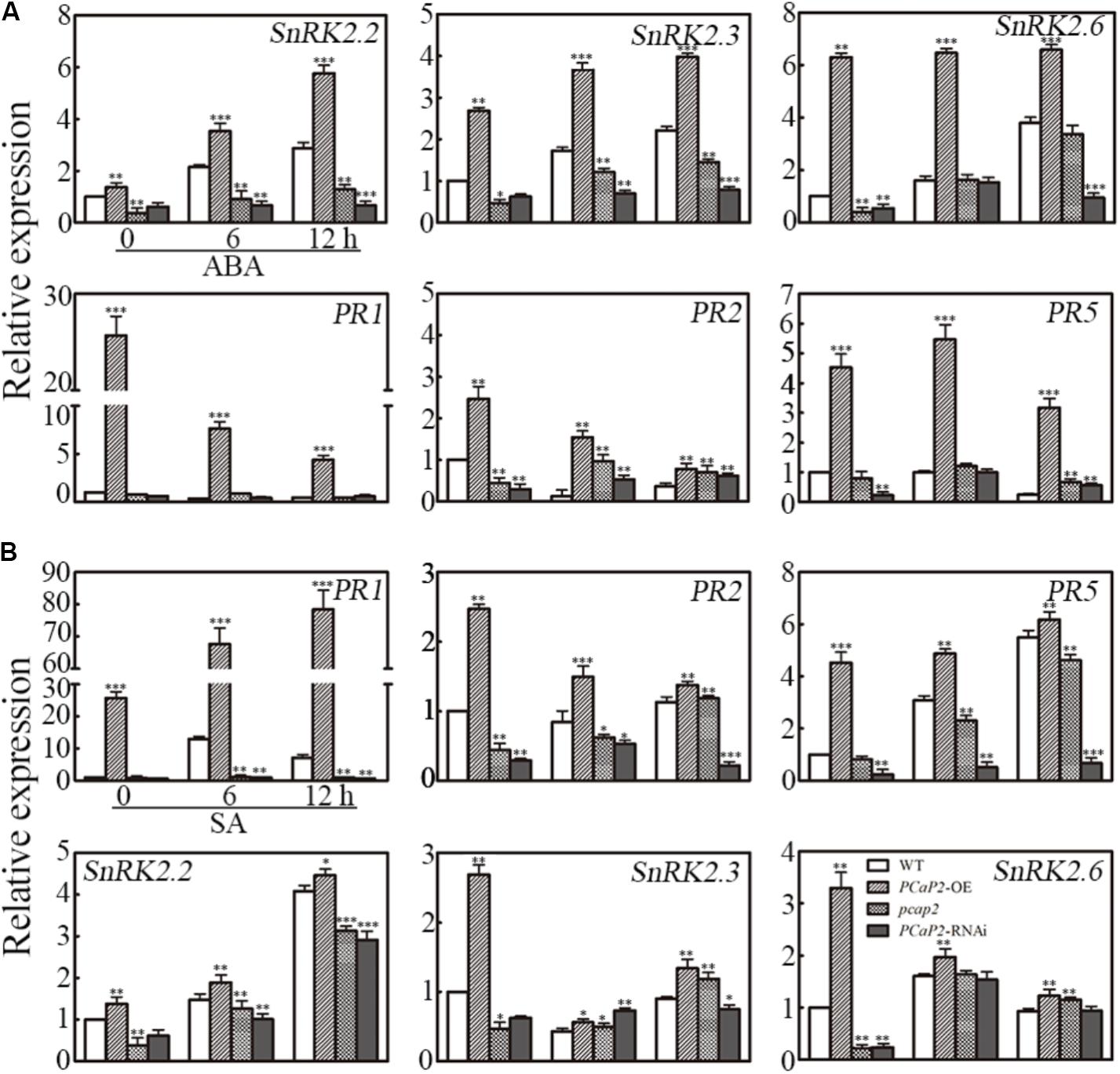
FIGURE 6. Effect of PCaP2 on the expression of SnRK2 and PR genes with exogenous ABA and SA. (A) The relative expression of SnRK2 genes and PR genes of the 14-day-old plants with 40 μM ABA treatments for 0, 6, and 12 h was determined in WT, PCaP2-OE, pcap2, and PCaP2-RNAi by qRT-PCR assay. (B) The relative expression of PR and SnRK2 genes of the 14-day-old plants with 100 μM SA treatments for 0, 6, and 12 h was determined in WT, PCaP2-OE, pcap2, and PCaP2-RNAi by qRT-PCR assay. Data represent mean values of three biological replicates ± SE. The significant difference was determined by ANOVA: ∗P < 0.05, ∗∗P < 0.01, ∗∗∗P < 0.001.
Discussion
Water deficit significantly influences plant growth, development, and productivity. One of the most important regulators in drought is ABA, and some cases of SA-mediated plant water deficit tolerance have been documented. However, details of crosstalk between ABA and SA signaling cascades in response to water deficit remain largely unknown. Previous studies have suggested that PCaP2 responds to some phytohormone signaling pathways and abiotic stress through mRNA expression pattern analysis (Kato et al., 2010). Our results further support a key role for PCaP2 in Arabidopsis water deficit tolerance and suggest that it is connected to the main ABA and SA signaling pathways.
Previous studies have shown that PCaP2 is mainly expressed in roots and flower tissues in normal conditions, and its mRNA level in whole seedlings is induced by drought as well as treatment with ABA or SA (Wang et al., 2007; Kato et al., 2010). Compared with previous studies, our data provide more detailed findings. High expression of PCaP2 in roots was found under normal and stress conditions. This might be related to its function in root water absorption and root hair growth in both normal and water deficit conditions. Additionally, PCaP2 was not expressed in true leaves under normal conditions, but was highly induced in water deficit and treatment with ABA or SA. This indicated that in true leaves, the increase of PCaP2 level is a key response to stress that is triggered by phytohormone signals, which may be associated with its function in controlling water deficit-induced leaf water loss.
Some ABA-sensitive seedlings show improved drought tolerance in soil, such as SnRK2s-OE and AREBs-OE, while some mutants show the same sensitivity to ABA and drought treatments, such as Atrgga and AtDi19-3 (Fujita et al., 2009; Qin et al., 2014; Ambrosone et al., 2015). Generally, ABA-sensitive mutants improve drought tolerance by regulating stomatal movement. However, changes in the drought tolerance of Atrgga and AtDi19-3 are not dependent on stomatal movement (Fujita et al., 2009; Qin et al., 2014; Ambrosone et al., 2015). In the present study, we used the previously identified PCaP2-OE line (Wang et al., 2007) pcap2 mutant (Kato et al., 2013; Zhu et al., 2013; Zhang et al., 2015; Kang et al., 2017) and PCaP2-RNAi lines (Wang et al., 2007), and our analysis of PCaP2 expression of these lines is the same with the prior findings. Our results showed that pcap2 and PCaP2-RNAi lines were more sensitive to ABA and drought, while PCaP2-OE plants were more resistant to ABA and drought stress. Although our results do not address whether PCaP2 can regulate stomatal movement, it is possible that PCaP2 has a similar mechanism to AtRGGA and AtDi19-3. PCaP2 do not fully depend on the regulation of stomatal movement in response to drought stress; PCaP2 functionality in plant water deficit tolerance can be borne out by regulating root hair growth to benefit water absorption and to induce the expression of numerous key drought-responsive genes (Figure 7). Furthermore, the water deficit-inducible phenotypes in fully silenced PCaP2-RNAi plants were greater in magnitude to those in knockdown pcap2 mutants. This suggests that the role of PCaP2 in water deficit is dependent on its inducible expression level. These results indicate that PCaP2 is a positive regulator of water deficit tolerance in Arabidopsis.
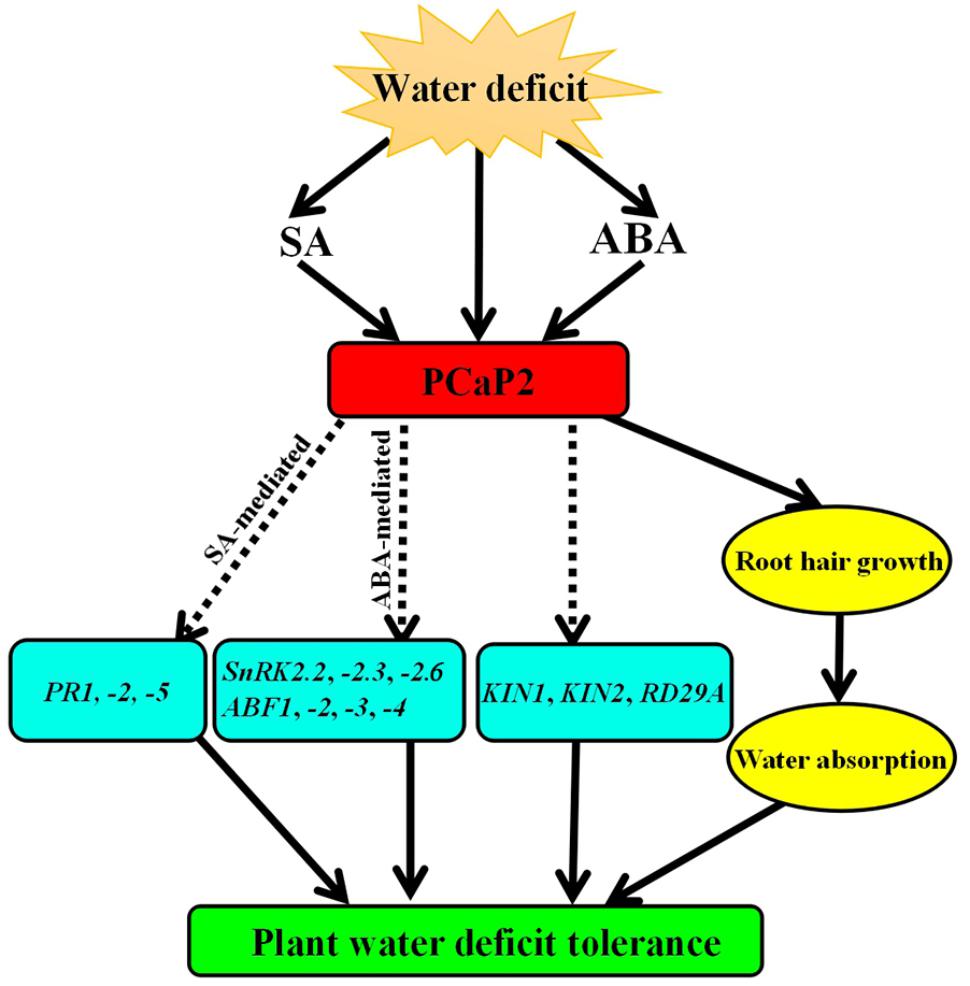
FIGURE 7. Working model of PCaP2 function in plant response to water deficit stress. Arrows ending solid lines indicate positive and direct regulation, arrows ending broken lines denote hypothetical or indirect regulation. Details of this model are discussed in the text.
Root hairs are the major water absorbing tissue in plants. Under water-limited conditions, root hair growth is significantly inhibited, which affects root water absorption and plant water deficit tolerance (Worrall and Roughley, 1976; Zahran and Sprent, 1986; Schnall and Quatrano, 1992). Our results showed that reduced expression of PCaP2 leads to shorter root hairs under normal conditions, which is consistent with previous results from Zhang et al. (2015). Moreover, significant inhibition of shorter root hair development was seen under water deficit conditions in pcap2 and PCaP2-RNAi, which could be one main pathway through which PCaP2 induces plant water deficit tolerance.
In Arabidopsis, SnRK2.2, -2.3, and -2.6 are crucial in the response to water deficit stress (Shi and Yang, 2014; Trivedi et al., 2016). Prior studies have shown that SnRK2.2, -2.3, and -2.6 triple mutants are insensitive to ABA and water deficit treatments (Fujii and Zhu, 2009; Fujita et al., 2009). Thus, PCaP2 mainly influences plant water deficit tolerance by regulating the ABA-mediated SnRK2 signaling pathway. It has been shown that SnRK2.6 functionally separates from SnRK2.2 and -2.3 under water deficit stress (Fujii et al., 2007; Kulik et al., 2011). Furthermore, SnRK2.2 and SnRK2.3 kinases regulate seed dormancy, germination, and seedling growth under water deficit conditions (Fujii et al., 2007; Fujita et al., 2009). SnRK2.6/OST1 plays an important role in stomatal movement (Vlad et al., 2009; Vahisalu et al., 2010; Brandt et al., 2012; Acharya et al., 2013; Imes et al., 2013; Grondin et al., 2015; Matrosova et al., 2015; Yin et al., 2016), suggesting that it is involved in plant drought tolerance by regulating stomatal movement. Some studies have further demonstrated that SnRK2.2, -2.3, and -2.6 are crucial in the response of Arabidopsis to ABA and water deficit stress (Shi and Yang, 2014; Trivedi et al., 2016). The expression of ABA-dependent transcription factors, such as ABIs and ABFs, is also stimulated by SnRK2s (Fujii et al., 2007). These factors mediate the expression of downstream ABA-inducible genes to improve plant water deficit resistance. Our results illustrated that PCaP2 increased SnRK2.2, -2.3, and -2.6 expression as well as SnRK2-mediated gene expression, such as ABF1, -2, -3, -4, KIN1, KIN2, and RD29, in water deficit stress and ABA treatments. Consequently, it can be speculated that water deficit stress-induced PCaP2 may affect the ABA-mediated SnRK2 signaling pathway and thus regulate drought-inducible gene expression, seed germination, seedling growth, and leaf water loss.
Compared to the ABA signaling pathway, relatively few cases of SA-mediated drought tolerance have been presented, Kang G. et al. (2012) and Kang G.Z. et al. (2012) identified 76 proteins as potentially involved in the SA signaling pathway in drought-exposed T. aestivum. Genetic and gene expression analysis of these proteins show that PR1, -2, and -5 are required in SA signaling in drought stress (Liu P. et al., 2013; Liu W.X. et al., 2013). Arabidopsis cpr5 and acd6 mutants exhibit SA accumulation, and their drought tolerance is improved by inducing SA-mediated expression of PR1, -2, and -5 (Liu P. et al., 2013). Additionally, PR1, -2, and -5 overexpression enhances drought tolerance in Arabidopsis (Liu W.X. et al., 2013; Qin et al., 2014).
Transcription factor Di19 regulates the expression of PR1, -2, and -5 in drought conditions (Liu W.X. et al., 2013; Qin et al., 2014). Water loss rates of PR1-, PR2-, and PR5-overexpressing lines are lower than those of WT plants, and the three genes are highly expressed in stomata, suggesting that PR1, PR2, and PR5 may regulate stomatal movement in response to drought tolerance (Liu W.X. et al., 2013; Qin et al., 2014). Additionally, PRs play significant roles in regulating phytohormone-signaling, such as auxin and JA (Thaler et al., 1999; Wang et al., 2001; Wen et al., 2008; Iglesias et al., 2011), suggesting that PR1, PR2, and PR5 may mediate the complex crosstalk between phytohormone signals under drought stress. However, complete mechanisms of PR actions under drought stress are largely unknown. Our results show that PCaP2 positively regulates the expression of PR1, -2, and -5 after SA and water deficit treatments, but not ABA treatment. In addition, PCaP2-RNAi and pcap2 lines lost water faster and wilted earlier than WT lines, which stands in contrast to the phenotypes of PR1-, PR2-, and PR5-overexpressing seedlings (Liu W.X. et al., 2013; Qin et al., 2014). However, the phenotypes of PCaP2-OE plants are consistent with those of PRs-overexpressing seedlings. Thus, these results support the idea that PCaP2 activates the key SA-mediated signaling pathway in response to water deficit, providing a new pathway to regulate PRs under water deficit conditions.
Interactions between SA and ABA signals have been shown to occur during abiotic stress, such as salt and cold stress. In S. lycopersicum, treatment with SA improves plant growth, osmotic adaptation, and ABA accumulation under normal conditions and during salt stress (Szepesi et al., 2009). Under cold conditions, exogenous ABA treatment increases endogenous SA level and oHCA content in Z. mays, suggesting that the ABA signal may combine with SA-related stress responses during cold stress (Szalai et al., 2011). Our results showed that ABA and SA can trigger PCaP2, and that PCaP2 then regulates the ABA-mediated SnRK2 signaling pathway as well as the expression of SA-mediated PR genes during water deficit stress. Thus, PCaP2 mediates crosstalk in response to ABA and SA signals during water deficit.
We have not interrogated whether PCaP2 can directly regulate SnRK2s and/or PRs in normal, water deficit, or exogenous ABA and SA conditions. However, previous studies have shown that PCaP2 can bind PtdInsPs, Ca2+ and Ca2+/CaM complexes. These ligands are important components of intracellular signaling networks involved in plant water deficit tolerance (Zielinski, 1998; Meijer and Munnik, 2003; Carlton and Cullen, 2005; Perera et al., 2008; DeFalco et al., 2009; Kleerekoper and Putkey, 2009; Luan, 2009; Xue et al., 2009; Kato et al., 2010, 2013). Our results also showed that PCaP2 regulated the expression of many key ABA-responsive genes, SA-responsive genes, and drought-inducible genes, including upstream genes such as SnRK2.2, -2.3, and -2.6. Thus, it is well-accepted that PCaP2 mainly functions as a Ca2+-binding protein to participate in intracellular signaling transduction under water deficit stress.
In conclusion, water deficit triggers ABA and SA accumulation, which, in turn, induce PCaP2 expression. PCaP2 then activates the expression of many key drought-responsive genes, including ABA-mediated genes (SnRK2.2, -2.3, -2.6, ABF1, -2, -3, -4) and SA-mediated genes (PR1, -2, -5) and drought-inducible genes (RD29A, KIN1 and KIN2), and affects root hair growth to increase water absorption, which improve plant water deficit tolerance (Figure 7). This study highlights the positive and important role that PCaP2 plays in plant water deficit tolerance by mediating crosstalk between ABA and SA signals, providing novel evidence relating to the underlying and complex mechanisms mediated by ABA and SA signals during plant water deficit tolerance.
Materials and Methods
Plant Materials, Growth Conditions, and Treatments
Arabidopsis ecotype Col-0 was the background for all transgenic and mutant plants in this study. The seeds of PCaP2-OE line (Wang et al., 2007), pcap2 mutant (Kato et al., 2013; Zhu et al., 2013; Zhang et al., 2015; Kang et al., 2017), and PCaP2-RNAi line (Wang et al., 2007) and PCaP2 promoter::GUS (pPCaP2::GUS) (Wang et al., 2007) were provided by Professor Ming Yuan, China Agricultural University, Beijing, China. All these seeds were used in the previous reports.
Surface sterilized Arabidopsis seeds were sown on MS plates with 1/2 MS (Murashige & Skoog) medium (pH adjusted to 5.8–6.0 with 1 M NaOH) with vitamins, 0.6 % phytoagar (PlantMedia), and 1% sucrose, and then in darkness at 4°C for 3 days. Seedlings were then transferred to a growth chamber at 22°C, with a 16 h/8 h (light/dark) photoperiod at approximately 120 μmol m-2 s-1. The 1/2 MS plus 1% sucrose media are usually used in water deficit, ABA, and SA treatments (Jayakannan et al., 2015; Schwalm et al., 2017; Zhu et al., 2017). PCaP2-OE, WT, pcap2, and PCaP2-RNAi were used for all experiments. For germination rate analysis, seeds were sown on 1/2 MS medium with 25 % PEG (dehydration), 0.8 μM ABA and 0.3 mM SA for 1, 2, 3, 4, 5 days, respectively. For seedling growth experiments, 4-day-old seedlings were transferred to soil for 7 days, then with 40 % PEG (dehydration), 0.5 μM ABA and 0.05 mM SA for 10 days. For survival experiments, 4-day-old seedlings were transferred to soil for 7 days, then were treated for 10 days without water, following with water for 7 days. At least 20 seedlings were harvested for observing in three biological replicates in each experiment. The best concentration of ABA and SA in the present paper was chosen by the prepared experiments for 0.01 to 40 μM of ABA and SA (data not shown), because the previous findings show that significantly different ABA and SA concentrations from 1 nM to 80 μM are used in seed germination and seedling growth experiments (Reyes and Chua, 2007; Zhu et al., 2007; Horváth et al., 2015; Huang et al., 2017). For water-loss assays, rosette leaves of comparable size from 4-week-old plants grown under long days were detached, placed on a Petri dish and weighted at a specified time (0, 0.5, 1, 1.5, 2, 2.5, 3, 3.5, and 4 h) after detachment. For root hair growth assays, 3-day-old seedlings were transferred to 1/2 MS medium with or without 15% PEG for 3 days, and the root hair length was assayed by ImageJ software1. Three-day-old seedlings transferred to 1/2 MS medium with or without 15% PEG for 7 days were used to test relative water content. Relative water content was measured as previous reports described (Brini et al., 2007).
RNA Isolation and Quantitative RT-PCR (qRT-PCR) Analysis
Total RNA was isolated from 1-day-old seedlings using RNasy plant mini kit (Qiagen), treated with RNase-Free DNase (Qiagen) at 37°C for 1 h to degrade genomic DNA, and 1 μg total RNA to synthesize cDNA by oligo-(dT) 20-primed reverse transcription by the Omniscript RT Kit (Qiagen). The cDNA was amplified using SuperReal PreMix Plus (SYBR Green, TIANGEN, China) in a 10 μL volume. The expression levels of 18S rRNA was used as an internal control. Analysis was performed using the BioRad Real-Time System CFX96TM C1000 Thermal Cycler (Singapore).
For the expression of PCaP2 under drought, ABA, and SA treatment assays, 14-day-old seedlings from WT plants were exposed 1/2 MS medium with or without drought, 40 μM ABA and 100 μM SA for 0, 1, 3, 6, 9, and 12 h. The previous finding showed the higher expression level of PCaP2 has been found in exogenous 100 μM ABA and 100 μM SA treatments (Kato et al., 2010). The expression of many genes can be increased in 10 to 100 μM ABA treatments in Arabidopsis (Zhu et al., 2007; Kato et al., 2010; Tian et al., 2015; Zhu et al., 2017). We found the expression level of PCaP2 can be induced in 40 μM ABA treatments, and the expression is higher than that in 100 μM ABA treatments (Figures 3A; Kato et al., 2010). Thus 40 μM ABA was used in our experiments. At each time point, all seedlings were immediately frozen by liquid nitrogen and then stored at -80°C for RNA preparation. To assay the expression of drought-, ABA- and SA-responsive genes under water deficit stress in WT and pcap2, WT and mutant seedlings grown at normal conditions for 14 days were harvested and treated by dehydration stress for 0, 6, 12 h. To assay the expression of SnRK2 and PR genes in WT and pcap2 treatment with ABA and SA, the seedlings of 14-day-old at normal condition were harvested and treated with 40 μM ABA and 100 μM SA for 0, 6, 12 h. Total RNA extraction and reverse transcription were performed as described above. All primer pairs used for qRT-PCR examination are listed in Supplementary Table S1. Each representative experiment was performed with at least three replicates.
Histochemical Staining of GUS Activity
Seven-day-old or 14-day-old pPCaP2::GUS seedlings treated with 40% PEG, 40 μM ABA, or 100 μM SA for 0, 1, 3, 6, and 12 h were collected for observing the changes of GUS activity. The GUS staining procedure was executed by the method mentioned in Wang et al. (2007). Samples were observed on an Olympus microscope equipped with a color CCD camera (Sutter Instrument; LAMBDA 10-2) or by an Epson scanner.
Statistical Analysis
The experiments were conducted at least three times, each of which contained three technical replicates. Data presented as the mean ± SE of three biological replicates. The significant difference was analyzed by SPSS statistical software (ver.16.0, SPSS Inc., Chicago, IL, United States) via one-way or two-way ANOVA (∗P < 0.05, ∗∗P < 0.01, ∗∗∗P < 0.001). In addition, we tested the normality of data about the main root length, leaf area, and root hair length under drought stress with Lilliefors corrected K-Sa test and Shapiro–Wilk test by SPSS (Supplementary Table S2). The detailed information of the chemicals and kits used in this study are listed in Supplementary Table S3.
Author Contributions
CW and XW designed the study. YW, LW, HL, BZ, and QC performed the experiments and data analysis. XL, SB, YL, and QW provided help in experimental methods. SZ participated in the discussion. MH and ST trained the use of experimental equipment. SY helped to revised the language and grammar. CW wrote the manuscript.
Funding
This research was supported by the National Natural Science Foundation of China (Nos. 31470358, 31500208, 31170232, and 31070157). Nation Melon Industry Technology System. The startup funds from Shenyang Agricultural University (www.syau.edu.cn) to XW. Basic Research Program of Ministry Education Foundation of Liaoning (110201132017005).
Conflict of Interest Statement
The authors declare that the research was conducted in the absence of any commercial or financial relationships that could be construed as a potential conflict of interest.
Acknowledgments
The authors thank Professor Ming Yuan of China Agricultural University providing the seeds of the Arabidopsis pcap2, PCaP2-RNAi and PCaP2-OE.
Supplementary Material
The Supplementary Material for this article can be found online at: https://www.frontiersin.org/articles/10.3389/fpls.2018.00578/full#supplementary-material
Abbreviations
ABA, abscisic acid; ABF, abscisic acid responsive element-binding factor; ABI, abscisic acid insensitive; AREBs/ABFs, ABRE-binding proteins/factors; Ca2+, calcium ion; CaM, calmodulin; CCD, charge-coupled device; CPK, calcium-dependent protein kinase; Di19, drought-induced 19; GA, gibberellin; GR, glutathione reductase; GST, glutathione s-transferase; GUS, β-glucuronidase; IAA, indole-3-acetic acid; JA, jasmonic acid; KIN, kinase; MDHAR, Monodehydroascorbate reductase; MAP18/PCaP2, microtubule-associated protein18/plasma membrane-associated Ca2+-binding protein-2; MT, microtubule; MS, Murashige Skoog; NO, nitric oxide; OST1, open stomata1; PA, phosphatidic acid; PCaP2-OE, PCaP2 overexpression; PCaP2-RNAi, PCaP2 RNA interference; PEG, polyethylene glycol; PR, pathogenesis-related; PtdInsPs, phosphatidylinositol phosphates; qRT-PCR, quantitative real-time PCR; RD29A, responsive to desiccation 29A; RT-PCR, reverse transcriptase-mediated PCR; SA, salicylic acid; S. lycopersicum, Solanum lycopersicum; SNF, sucrose non-fermenting; SnRK2, SNF1-related protein kinase 2; WT, wild type; Z. mays, Zea mays; RGGA, Arginine Glycine Glycine (RGG) box-containing RNA-binding protein; Di19, drought-induced protein19.
Footnotes
References
Acharya, B. R., Jeon, B. W., Zhang, W., and Assmann, S. M. (2013). Open Stomata 1 (OST1) is limiting in abscisic acid responses of Arabidopsis guard cells. New Phytol. 200, 1049–1063. doi: 10.1111/nph.12469
Ahlfors, R., Macioszek, V., Rudd, J., Brosché, M., Schlichting, R., Scheel, D., et al. (2004). Stress hormone-independent activation and nuclear translocation of mitogen-activated protein kinases in Arabidopsis thaliana during ozone exposure. Plant J. 40, 512–522. doi: 10.1111/j.1365-313X.2004.02229.x
Alam, M. M., Hasanuzzaman, M., Nahar, K., and Fujita, M. (2013). Exogenous salicylic acid ameliorates short-term drought stress in mustard (Brassica juncea L.) seedlings by up-regulating the antioxidant defense and glyoxalase system. Aust. J. Crop Sci. 7, 1053–1063.
Ambrosone, A., Batelli, G., Nurcato, R., Aurilia, V., Punzo, P., Bangarusamy, D. K., et al. (2015). The Arabidopsis RNA-binding protein AtRGGA regulates tolerance to salt and drought stress. Plant Physiol. 168, 292–306. doi: 10.1104/pp.114.255802
Bandurska, H., and Stroiński, A. (2005). The effect of salicylic acid on barley response to water deficit. Acta Physiol. Plant. 27, 379–386. doi: 10.1007/s11738-005-0015-5
Boudsocq, M., Barbier-Brygoo, H., and Laurière, C. (2004). Identification of nine sucrose nonfermenting 1-related protein kinases 2 activated by hyperosmotic and saline stresses in Arabidopsis thaliana. J. Biol. Chem. 279, 41758–41766. doi: 10.1074/jbc.M405259200
Brandt, B., Brodsky, D. E., Xue, S., Negi, J., Iba, K., Kangasjärvi, J., et al. (2012). Reconstitution of abscisic acid activation of SLAC1 anion channel by CPK6 and OST1 kinases and branched ABI1 PP2C phosphatase action. Proc. Natl. Acad. Sci. U.S.A. 109, 10593–10598. doi: 10.1073/pnas.1116590109
Brini, F., Hanin, M., Lumbreras, V., Amara, I., and Khoudi, H. H. (2007). Overexpression of wheat dehydrin DHN-5 enhances tolerance to salt and osmotic stress in Arabidopsis thaliana. Plant Cell Rep. 26, 2017–2026. doi: 10.1007/s00299-007-0412-x
Carlton, J. G., and Cullen, P. J. (2005). Coincidence detection in phosphoinositide signaling. Trends Cell Biol. 15, 540–547. doi: 10.1016/j.tcb.2005.08.005
Choi, H., Hong, J., Ha, J., Kang, J., and Kim, S. Y. (2000). ABFs, a family of ABA-responsive element binding factors. J. Biol. Chem. 275, 1723–1730. doi: 10.1074/jbc.275.3.1723
Cutler, S. R., Rodriguez, P. L., Finkelstein, R. R., and Abrams, S. R. (2010). Abscisic acid: emergence of a core signaling network. Annu. Rev. Plant Biol. 61, 651–679. doi: 10.1146/annurev-arplant-042809-112122
DeFalco, T. A., Bender, K. W., and Snedden, W. A. (2009). Breaking the code: Ca2+ sensors in plant signaling. Biochem. J. 425, 27–40. doi: 10.1042/BJ20091147
Fleta-Soriano, E., and Munné-Bosch, S. (2016). Stress memory and the inevitable effects of drought: a physiological perspective. Front. Plant Sci. 7:143. doi: 10.3389/fpls.2016.00143
Fujii, H., Verslues, P. E., and Zhu, J. K. (2007). Identification of two protein kinases required for abscisic acid regulation of seed germination, root growth and gene expression in Arabidopsis. Plant Cell 19, 485–494. doi: 10.1105/tpc.106.048538
Fujii, H., and Zhu, J. K. (2009). Arabidopsis mutant deficient in 3 abscisic acid-activated protein kinases reveals critical roles in growth, reproduction, and stress. Proc. Natl. Acad. Sci. U.S.A. 106, 8380–8385. doi: 10.1073/pnas.0903144106
Fujita, Y., Nakashima, K., Yoshida, T., Katagiri, T., Kidokoro, S., Kanamori, N., et al. (2009). Three SnRK2 protein kinases are the main positive regulators of abscisic acid signaling in response to water stress in Arabidopsis. Plant Cell Physiol. 50, 2123–2132. doi: 10.1093/pcp/pcp147
Geiger, D., Scherzer, S., Mumm, P., Marten, I., Ache, P., Matschi, S., et al. (2010). Guard cell anion channel SLAC1 is regulated by CDPK protein kinases with distinct Ca2+ affinities. Proc. Natl. Acad. Sci. U.S.A. 107, 8023–8028. doi: 10.1073/pnas.0912030107
Geiger, D., Scherzer, S., Mumm, P., Stange, A., Marten, I., Bauer, H., et al. (2009). Activity of guard cell anion channel SLAC1 is controlled by drought-stress signaling kinase-phosphatase pair. Proc. Natl. Acad. Sci. U.S.A. 106, 21425–21430. doi: 10.1073/pnas.0912021106
Gong, X., Liu, M., Zhang, L., Ruan, Y., Ding, R., Ji, Y., et al. (2015). Arabidopsis AtSUC2 and AtSUC4, encoding sucrose transporters, are required for abiotic stress tolerance in an ABA-dependent pathway. Physiol. Plant. 153, 119–136. doi: 10.1111/ppl.12225
Grondin, A., Rodrigues, O., Verdoucq, L., Merlot, S., Leonhardt, N., and Maurel, C. (2015). Aquaporins contribute to ABA-triggered stomatal closure through OST1-mediated phosphorylation. Plant Cell 27, 1945–1954. doi: 10.1105/tpc.15.00421
Gudesblat, G. E., Iusem, N. D., and Morris, P. C. (2007). Guard cell-specific inhibition of Arabidopsis MPK3 expression causes abnormal stomatal responses to abscisic acid and hydrogen peroxide. New Phytol. 173, 713–721. doi: 10.1111/j.1469-8137.2006.01953.x
Habibi, G. (2012). Exogenous salicylic acid alleviates oxidative damage of barley plants under drought stress. Acta Biol. Szeged. 56, 57–63.
Hao, F., Zhao, S., Dong, H., Zhang, H., Sun, L., and Chen, M. (2010). Nia1 and Nia2 are involved in exogenous salicylic acid-induced nitric oxide generation and stomatal closure in Arabidopsis. J. Integr. Plant Biol. 52, 298–307. doi: 10.1111/j.1744-7909.2010.00920.x
Hayat, S., Hasan, S. A., Fariduddin, Q., Ahmad, A., et al. (2008). Growth of tomato (Lycopersicon esculentum) in response to salicylic acid under water stress. J. Plant Interact. 3, 297–304. doi: 10.1080/17429140802320797
Horváth, E., Brunner, S., Bela, K., Papdi, C., and Szabados, L. T. (2015). Exogenous salicylic acid-triggered changes in the glutathione transferases and peroxidases are key factors in the successful salt stress acclimation of Arabidopsis thaliana. Funct. Plant Biol. 42, 1129–1140. doi: 10.1071/FP15119
Hua, D., Wang, C., He, J., Liao, H., Duan, Y., Zhu, Z., et al. (2012). A plasma membrane receptor kinase, GHR1, mediates abscisic acid and hydrogen peroxide-regulated stomatal movement in Arabidopsis. Plant Cell 24, 2546–2561. doi: 10.1105/tpc.112.100107
Huang, Y., Sun, M. M., Ye, Q., Wu, X. Q., Wu, W. H., and Chen, Y. F. (2017). Abscisic acid modulates seed germination via ABA insensitive5-mediated phosphate1. Plant Physiol. 175, 1661–1668. doi: 10.1104/pp.17.00164
Ichimura, K., Mizoguchi, T., Yoshida, R., Yuasa, T., and Shinozaki, K. (2000). Various abiotic stresses rapidly activate Arabidopsis MAP kinases ATMPK4 and ATMPK6. Plant J. 24, 655–665. doi: 10.1046/j.1365-313x.2000.00913.x
Iglesias, M. J., Terrile, M. C., and Casalongué, C. A. (2011). Auxin and salicylic acid signallings counteract during the adaptive response to stress. Plant Signal. Behav. 6, 452–454. doi: 10.4161/psb.6.3.14676
Imes, D., Mumm, P., Böhm, J., Al-Rasheid, K. A., Marten, I., Geiger, D., et al. (2013). Open stomata 1 (OST1) kinase controls R-type anion channel QUAC1 in Arabidopsis guard cells. Plant J. 74, 372–382. doi: 10.1111/tpj.12133
Jarzyniak, K. M., and Jasiński, M. (2014). Membrane transporters and drought resistance-a complex issue. Front. Plant Sci. 5:687. doi: 10.3389/fpls.2014.00687
Jayakannan, M., Bose, J., Babourina, O., Shabala, S., and Poschenrieder, C. (2015). The NPR1-dependent salicylic acid signalling pathway is pivotal for enhanced salt and oxidative stress tolerance in Arabidopsis. J. Exp. Bot. 66, 1865–1875. doi: 10.1093/jxb/eru528
Jia, W., Zhang, L., Wu, D., Liu, S., Gong, X., Cui, Z., et al. (2015). Sucrose transporter AtSUC9 mediated by a low sucrose level is involved in Arabidopsis abiotic stress resistance by regulating sucrose distribution and ABA accumulation. Plant Cell Physiol. 56, 1574–1587. doi: 10.1093/pcp/pcv082
Kang, E., Zheng, M., Zhang, Y., Yuan, M., Yalovsky, S., Zhu, L., et al. (2017). The microtubule-associated protein MAP18 affects ROP2 GTPase activity during root hair growth. Plant Physiol. 174, 202–222. doi: 10.1104/pp.16.01243
Kang, G., Li, G., Xu, W., Peng, X., Han, Q., Zhu, Y., et al. (2012). Proteomics reveals the effects of salicylic acid on growth and tolerance to subsequent drought stress in wheat. J. Proteome Res. 11, 6066–6079. doi: 10.1021/pr300728y
Kang, G. Z., Li, G. Z., Liu, G. Q., Xu, W., Peng, X. Q., Wang, C. Y., et al. (2013). Exogenous salicylic acid enhances wheat drought tolerance by influence on the expression of genes related to ascorbate-glutathione cycle. Biol. Plant 57, 718–724. doi: 10.1007/s10535-013-0335-z
Kang, G. Z., Peng, H. F., Han, Q. X., Wang, Y. H., and Guo, T. C. (2012). Identification and expression pattern of ribosomal L5 gene in common wheat (Triticum aestivum L.). Gene 493, 62–68. doi: 10.1016/j.gene.2011.11.023
Kato, M., Aoyama, T., and Maeshima, M. (2013). The Ca2+-binding protein PCaP2 located on the plasma membrane is involved in root hair development as a possible signal transducer. Plant J. 74, 690–700. doi: 10.1111/tpj.12155
Kato, M., Nagasaki-Takeuchi, N., Ide, Y., and Maeshima, M. (2010). An Arabidopsis hydrophilic Ca2+-binding protein with a PEVK-rich domain, PCaP2, is associated with the plasma membrane and interacts with calmodulin and phosphatidylinositol phosphates. Plant Cell Physiol. 51, 366–379. doi: 10.1093/pcp/pcq003
Khan, M. I. R., Iqbal, N., Masood, A., and Khan, N. A. (2012a). Variation in salt tolerance of wheat cultivars: role of glycinebetaine and ethylene. Pedosphere 22, 746–754. doi: 10.1016/S1002-0160(12)60060-5
Khan, M. I. R., Iqbal, N., Masood, A., Per, T. S., and Khan, N. A. (2013). Salicylic acid alleviates adverse effects of heat stress on photosynthesis through changes in proline production and ethylene formation. Plant Signal. Behav. 8:e26374. doi: 10.4161/psb.26374
Khan, M. I. R., Syeed, S., Nazar, R., and Anjum, N. A. (2012b). “An insight into the role of salicylic acid and jasmonic acid in salt stress tolerance,” in Phytohormones and Abiotic Stress Tolerance in Plants, eds N. A. Khan, R. Nazar, N. Iqbal, and N. A. Anjum (Berlin: Springer), 277–300.
Khokon, A. R., Okuma, E., Hossain, M. A., Munemasa, S., and Uraji, M. N. (2011). Involvement of extracellular oxidative burst in salicylic acid-induced stomatal closure in Arabidopsis. Plant Cell Environ. 34, 434–443. doi: 10.1111/j.1365-3040.2010.02253.x
Kleerekoper, Q. K., and Putkey, J. A. (2009). PEP-19, an intrinsically disordered regulator of calmodulin signaling. J. Biol. Chem. 284, 7455–7464. doi: 10.1016/j.tcb.2005.08.005
Kulik, A., Wawer, I., Krzywińska, E., Bucholc, M., and Dobrowolska, G. (2011). SnRK2 protein kinases-key regulators of plant response to abiotic stresses. OMICS 15, 859–872. doi: 10.1089/omi.2011.0091
Kurkela, S., and Borg-Franck, M. (1992). Structure and expression of kin2, one of two cold- and ABA-induced genes of Arabidopsis thaliana. Plant Mol. Biol. 19, 689–692. doi: 10.1007/BF00026794
Lee, S. C., Lan, W., Buchanan, B. B., and Luan, S. (2009). A protein kinase-phosphatase pair interacts with an ion channel to regulate ABA signaling in plant guard cells. Proc. Natl. Acad. Sci. U.S.A. 106, 21419–21424. doi: 10.1073/pnas.0910601106
Liu, P., Xu, Z. S., Pan-Pan, L., Hu, D., Chen, M., Li, L. C., et al. (2013). A wheat PI4K gene whose product possesses threonine autophophorylation activity confers tolerance to drought and salt in Arabidopsis. J. Exp. Bot. 64, 2915–2927. doi: 10.1093/jxb/ert133
Liu, W. X., Zhang, F. C., Zhang, W. Z., Song, L. F., Wu, W. H., and Chen, Y. F. (2013). Arabidopsis Di19 functions as a transcription factor and modulates PR1, PR2, and PR5 expression in response to drought stress. Mol. Plant 6, 1487–1502. doi: 10.1093/mp/sst031
Luan, S. (2009). The CBL-CIPK network in plant calcium signaling. Trends Plant Sci. 14, 37–42. doi: 10.1016/j.tplants.2008.10.005
Matrosova, A., Bogireddi, H., Mateo-Peñas, A., Hashimoto-Sugimoto, M., Iba, K., Schroeder, J. I., et al. (2015). The HT1 protein kinase is essential for red light-induced stomatal opening and genetically interacts with OST1 in red light and CO2 -induced stomatal movement responses. New Phytol. 208, 1126–1137. doi: 10.1111/nph.13566
Meijer, H. J., and Munnik, T. (2003). Phospholipid-based signaling in plants. Annu. Rev. Plant Biol. 54, 265–306. doi: 10.1146/annurev.arplant.54.031902.134748
Miura, K., Okamoto, H., Okuma, E., Shiba, H., Kamada, H., Hasegawa, P. M., et al. (2013). SIZ1 deficiency causes reduced stomatal aperture and enhanced drought tolerance via controlling salicylic acid-induced accumulation of reactive oxygen species in Arabidopsis. Plant J. 73, 91–104. doi: 10.1111/tpj.12014
Miura, K., and Tada, Y. (2014). Regulation of water, salinity, and cold stress responses by salicylic acid. Front. Plant Sci. 5:4. doi: 10.3389/fpls.2014.00004
Munne-Bosch, S., and Penuelas, J. (2003). Photo- and antioxidative protection, and a role for salicylic acid during drought and recovery in field-grown Phillyrea angustifolia plants. Planta 217, 758–766. doi: 10.1007/s00425-003-1037-0
Nakashima, K., Fujita, Y., Kanamori, N., Katagiri, T., Umezawa, T., Kidokoro, S., et al. (2009). Three Arabidopsis SnRK2 protein kinases, SRK2D/SnRK2.2, SRK2E/SnRK2.6/OST1 and SRK2I/SnRK2.3, involved in ABA signaling are essential for the control of seed development and dormancy. Plant Cell Physiol. 50, 1345–1363. doi: 10.1093/pcp/pcp083
Perera, I. Y., Hung, C. Y., Moore, C. D., Stevenson-Paulik, J., and Boss, W. F. (2008). Transgenic Arabidopsis plants expressing the type 1 inositol 5-phosphatase exhibit increased drought tolerance and altered abscisic acid signaling. Plant Cell 20, 2876–2893. doi: 10.1105/tpc.108.061374
Pilot, G., Lacombe, B., Gaymard, F., Cherel, I., Boucherez, J., Thibaud, J. B., et al. (2001). Guard cell inward K+ channel activity in Arabidopsis involves expression of the twin channel subunits KAT1 and KAT2. J. Biol. Chem. 276, 3215–3221. doi: 10.1074/jbc.M007303200
Qin, L. X., Li, Y., Li, D. D., Xu, W. L., Zheng, Y., and Li, X. B. (2014). Arabidopsis drought-induced protein Di19-3 participates in plant response to drought and high salinity stresses. Plant Mol. Biol. 86, 609–625. doi: 10.1007/s11103-014-0251-4
Raghavendra, A. S., Gonugunta, V. K., Christmann, A., and Grill, E. (2010). ABA perception and signalling. Trends Plant Sci. 15, 395–401. doi: 10.1016/j.tplants.2010.04.006
Raskin, I. (1992). Role of salicylic acid in plants. Annu. Rev. Plant Physiol. Plant Mol. Biol. 43, 439–463. doi: 10.1146/annurev.pp.43.060192.002255
Reyes, J. L., and Chua, N. H. (2007). Aba induction of miR159 controls transcript levels of two MYB factors during Arabidopsis seed germination. Plant J. 49, 592–606. doi: 10.1111/j.1365-313X.2006.02980.x
Saruhan, N., Saglam, A., and Kadioglu, A. (2012). Salicylic acid pretreatment induces drought tolerance and delays leaf rolling by inducing antioxidant systems in maize genotypes. Acta Physiol. Plant. 34, 97–106. doi: 10.1007/s11738-011-0808-7
Sato, A., Sato, Y., Fukao, Y., Fujiwara, M., Umezawa, T., Shinozaki, K., et al. (2009). Threonine at position 306 of the KAT1 potassium channel is essential for channel activity and is a target site for ABA-activated SnRK2/OST1/SnRK2.6 protein kinase. Biochem. J. 424, 439–448. doi: 10.1042/BJ20091221
Schnall, J. A., and Quatrano, R. S. (1992). Abscisic acid elicits the water-stress response in root hairs of Arabidopsis thaliana. Plant Physiol. 100, 216–218. doi: 10.1104/pp.100.1.216
Schwalm, C. R., Wrl, A., Michalak, A. M., Fisher, J. B., and Biondi, F. K. (2017). Global patterns of drought recovery. Nature 548, 202–205. doi: 10.1038/nature23021
Shi, Y., and Yang, S. H. (2014). “ABA regulation of the cold stress response in plants,” in Abscisic Acid: Metabolism, Transport and Signaling, ed. D. P. Zhang (Dordrecht, FL: Springer Science+Business Media), doi: 10.1007/978-94-017-9424-4_17
Szalai, G., Pál, M., and Janda, T. (2011). Abscisic acid may alter the salicylic acid-related abiotic stress response in maize. Acta Biol. Szeged. 55, 155–157.
Szepesi,Á, Csiszár, J., Gémes, K., Horváth, E., Horváth, F., Simon, M. L., et al. (2009). Salicylic acid improve acclimation to salt stress by stimulating abscisic aldehyde oxidase activity and abscisic acid accumulation, and increases Na+ content in leaves without toxicity symptoms in Solanum lycopersicum L. J. Plant Physiol. 166, 914–925. doi: 10.1016/j.jplph.2008.11.012
Thaler, J. S., Fidantsef, A. L., Duffey, S. S., and Bostock, R. M. (1999). Trade-offs in plant defense against pathogens and herbivores: a field demonstration of chemical elicitors of induced resistance. J. Chem. Ecol. 25, 1597–1609. doi: 10.1023/A:1020840900595
Tian, H., Guo, H., Dai, X., Cheng, Y., and Zheng, K. W. (2015). An ABA down-regulated bHLH transcription repressor gene, bHLH129 regulates root elongation and ABA response when overexpressed in Arabidopsis. Sci. Rep. 5:17587. doi: 10.1038/srep17587
Trivedi, D. K., Gill, S. S., and Tuteja, N. (2016). “Abscisic acid (ABA): biosynthesis, regulation, and role in abiotic stress tolerance,” in Abiotic Stress Response in Plants, eds N. Tuteja and S. S. Gill (Weinheim, FL: Wiley-VCHVerlag GmbH & Co. KGaA). doi: 10.1002/9783527694570.ch15
Umezawa, T. (2009). Type 2C protein phosphatases directly regulate abscisic acid-activated protein kinases in Arabidopsis. Proc. Natl. Acad. Sci. U.S.A. 13, 17588–17593. doi: 10.1073/pnas.0907095106
Uno, Y., Furihata, T., Abe, H., Yoshida, R., Shinozaki, K., and Yamaguchi-Shinozaki, K. (2000). Arabidopsis basic leucine zipper transcription factors involved in an abscisic acid-dependent signal transduction pathway under drought and high-salinity conditions. Proc. Natl. Acad. Sci. U.S.A. 97, 11632–11637. doi: 10.1073/pnas.190309197
Vahisalu, T., Puzõrjova, I., Brosché, M., Valk, E., Lepiku, M., Moldau, H., et al. (2010). Ozone-triggered rapid stomatal response involves the production of reactive oxygen species, and is controlled by SLAC1 and OST1. Plant J. 62, 442–453. doi: 10.1111/j.1365-313X.2010.04159.x
Virlouvet, L., and Fromm, M. (2015). Physiological and transcriptional memory in guard cells during repetitive dehydration stress. New Phytol. 205, 596–607. doi: 10.1111/nph.13080
Vlad, F., Rubio, S., Rodrigues, A., Sirichandra, C., Belin, C., Robert, N., et al. (2009). Protein phosphatases 2C regulate the activation of the Snf1-related kinase OST1 by abscisic acid in Arabidopsis. Plant Cell 21, 3170–3184. doi: 10.1105/tpc.109.069179
Wang, X., Zhu, L., Liu, B., Wang, C., Jin, L., Zhao, Q., et al. (2007). Arabidopsis MICROTUBULE-ASSOCIATED PROTEIN18 functions in directional cell growth by destabilizing cortical microtubules. Plant Cell 19, 877–889. doi: 10.1105/tpc.106.048579
Wang, Y., Mopper, S., and Hasenstein, K. H. (2001). Effects of salinity on endogenous ABA, IAA, JA, AND SA in iris hexagona. J. Chem. Ecol. 27, 327–342. doi: 10.1023/A:1005632506230
Weiner, J. J., Peterson, F. C., Volkman, B. F., and Cutler, S. R. (2010). Structural and functional insights into core ABA signaling. Curr. Opin. Plant Biol. 13, 495–502. doi: 10.1016/j.pbi.2010.09.007
Wen, P. F., Chen, J. Y., Wan, S. B., Kong, W. F., and Zhang, P. W. (2008). ). Salicylic acid activates phenylalanine ammonia-lyase in grape berry in response to high temperature stress. Plant Growth Regul. 55, 1–10. doi: 10.1007/s10725-007-9250-7
Worrall, V. S., and Roughley, R. G. (1976). The effect of moisture stress on infection of Trifolium subterraneum L. by Rhizobium trifolii Dang. J. Exp. Bot. 27, 1233–1247. doi: 10.1093/jxb/27.6.1233
Xue, H. W., Chen, X., and Mei, Y. (2009). Function and regulation of phospholipid signalling in plants. Biochem. J. 421, 145–156. doi: 10.1042/BJ20090300
Yamaguchi-Shinozaki, K., and Shinozaki, K. (1994). A novel cis-acting element in an Arabidopsis gene is involved in responsiveness to drought, low-temperature, or high-salt stress. Plant Cell 6, 251–264. doi: 10.1105/tpc.6.2.251
Yin, Y., Adachi, Y., Nakamura, Y., Munemasa, S., Mori, I. C., and Murata, Y. (2016). Involvement of OST1 protein kinase and PYR/PYL/RCAR receptors in methyl jasmonate-induced stomatal closure in Arabidopsis guard cells. Plant Cell Physiol. 57, 1779–1790. doi: 10.1093/pcp/pcw102
Yoshida, R., Hobo, T., Ichimura, K., Mizoguchi, T., Takahashi, F., Aronso, J., et al. (2002). ABA-activated SnRK2 protein kinase is required for dehydration stress signaling in Arabidopsis. Plant Cell Physiol. 43, 1473–1483. doi: 10.1093/pcp/pcf188
Zahran, H. H., and Sprent, J. I. (1986). Effects of sodium chloride and polyethylene glycol on root-hair infection and nodulation of Vicia faba L. plants by Rhizobium leguminosarum. Planta 167, 303–309. doi: 10.1007/BF00391332
Zhang, Y., Kang, E., Yuan, M., Fu, Y., and Zhu, L. (2015). PCaP2 regulates nuclear positioning in growing Arabidopsis thaliana root hairs by modulating filamentous actin organization. Plant Cell Rep. 34, 1317–1330. doi: 10.1007/s00299-015-1789-6
Zhu, L., Zhang, Y., Kang, E., Xu, Q., Wang, M., Rui, Y., et al. (2013). MAP18 regulates the direction of pollen tube growth in Arabidopsis by modulating F-actin organization. Plant Cell 25, 851–867. doi: 10.1105/tpc.113.110528
Zhu, S. Y., Yu, X. C., Wang, X. J., Zhao, R., and Li, Y. F. (2007). Two calcium-dependent protein kinases, cpk4 and cpk11, regulate abscisic acid signal transduction in Arabidopsis. Plant Cell 19, 3019–3036. doi: 10.1105/tpc.107.050666
Zhu, Y., Wang, B., Tang, K., Hsu, C. C., and Xie, S. D. (2017). An Arabidopsis nucleoporin NUP85 modulates plant responses to ABA and salt stress. PLoS Genet. 13:e1007124. doi: 10.1371/journal.pgen.1007124
Keywords: PCaP2, water deficit, ABA, SA, SnRK2, PR, Arabidopsis
Citation: Wang X, Wang Y, Wang L, Liu H, Zhang B, Cao Q, Liu X, Bi S, Lv Y, Wang Q, Zhang S, He M, Tang S, Yao S and Wang C (2018) Arabidopsis PCaP2 Functions as a Linker Between ABA and SA Signals in Plant Water Deficit Tolerance. Front. Plant Sci. 9:578. doi: 10.3389/fpls.2018.00578
Received: 29 November 2017; Accepted: 13 April 2018;
Published: 08 May 2018.
Edited by:
Erik T. Nilsen, Virginia Tech, United StatesReviewed by:
Yang Zhao, Shanghai Institutes for Biological Sciences (CAS), ChinaCristina Nali, Università degli Studi di Pisa, Italy
Caspar Christian Cedric Chater, University of Sheffield, United Kingdom
Copyright © 2018 Wang, Wang, Wang, Liu, Zhang, Cao, Liu, Bi, Lv, Wang, Zhang, He, Tang, Yao and Wang. This is an open-access article distributed under the terms of the Creative Commons Attribution License (CC BY). The use, distribution or reproduction in other forums is permitted, provided the original author(s) and the copyright owner are credited and that the original publication in this journal is cited, in accordance with accepted academic practice. No use, distribution or reproduction is permitted which does not comply with these terms.
*Correspondence: Che Wang, d2FuZ3dhbmdjaGVAMTYzLmNvbQ==
†These authors have contributed equally to this work.