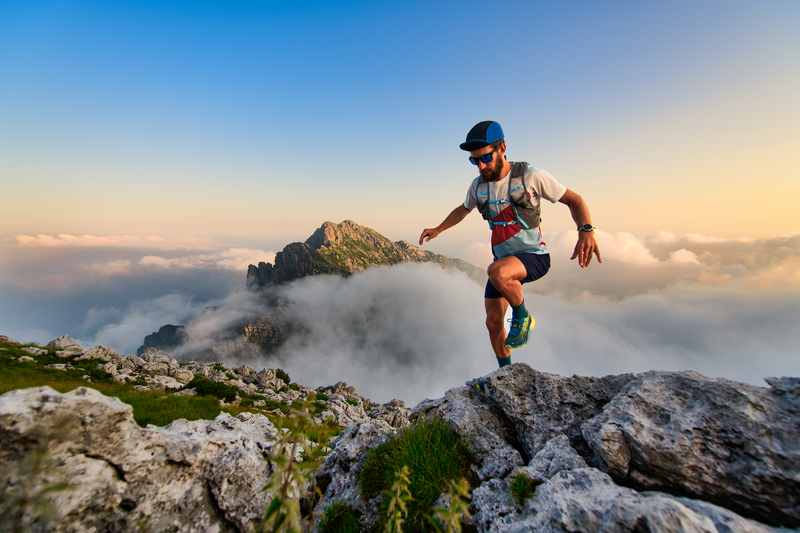
95% of researchers rate our articles as excellent or good
Learn more about the work of our research integrity team to safeguard the quality of each article we publish.
Find out more
ORIGINAL RESEARCH article
Front. Plant Sci. , 15 March 2018
Sec. Plant Nutrition
Volume 9 - 2018 | https://doi.org/10.3389/fpls.2018.00338
Ecological stoichiometry is an important aspect in the analysis of the changes in ecological system composition, structure, and function and understanding of plant adaptation in habitats. Leaf carbon (C), nitrogen (N), and phosphorus (P) concentrations in desert phreatophytes can be affected by different depths of groundwater through its effect on the adsorption and utilization of nutrient and plant biomass. We examined the biomass, soil organic C, available (mineral) N, and available P, and leaf C, N, and P concentrations of Alhagi sparsifolia grown at varying groundwater depths of 2.5, 4.5, and 11.0 m in 2015 and 2016 growing seasons in a desert-oasis ecotone in northwest China. The biomass of A. sparsifolia and the C, N, and P concentrations in soil and A. sparsifolia showed different responses to various groundwater depths. The leaf P concentration of A. sparsifolia was lower at 4.5 m than at 2.5 and 11.0 m likely because of a biomass dilution effect. By contrast, leaf C and N concentrations were generally unaffected by groundwater depth, thereby confirming that C and N accumulations in A. sparsifolia were predominantly determined by C fixation through the photosynthesis and biological fixation of atmospheric N2, respectively. Soil C, N, and P concentrations at 4.5 m were significantly lower than those at 11.0 m. Leaf P concentration was significantly and positively correlated with soil N concentration at all of the groundwater depths. The C:N and C:P mass ratios of A. sparsifolia at 4.5 m were higher than those at the other groundwater depths, suggesting a defensive life history strategy. Conversely, A. sparsifolia likely adopted a competitive strategy at 2.5 and 11.0 m as indicated by the low C:N and C:P mass ratios. To our knowledge, this study is the first to elucidate the variation in the C, N, and P stoichiometry of a desert phreatophyte at different groundwater depths in an arid ecosystem.
In ecological stoichiometry, relationships among multiple key elements in different ecological systems are examined (Elser et al., 2000; Cross et al., 2005; Rong et al., 2015; Zhan et al., 2017). Leaf ecological stoichiometry, especially carbon (C), nitrogen (N), and phosphorus (P) stoichiometry, is an important aspect in the analysis the changes in concerned communities and ecological system compositions, structures and functions (Han et al., 2005; Venterink and Güsewell, 2010; Gao et al., 2013; Rong et al., 2015; Li et al., 2017; Zeng et al., 2017). Biologically, C, N, and P are vital chemical elements and fundamental substances for plant growth (Sterner, 1995; Elser et al., 1996; Vrede et al., 2004; Wang et al., 2015; Butler et al., 2017). C remains relatively stable at 50% of dry mass composition and provides a structural basis for plants. N is an essential proteins element that plays an important role in enzyme activities. P participates in energy transfer in plant cells. N and P are crucial basic elements in nucleic acids (Marschner, 2012; Yan et al., 2016). C, N, and P concentrations generally change with plant growth because plant differ in photosynthetic capability, nutritional requirement, and adaptation to their environment (Elser et al., 1996; Rong et al., 2015; Wang et al., 2015; Zeng et al., 2017).
C, N, and P are coupled in terms of their biochemical functions in plants. C:N and C:P mass ratios are important indicators that represent the growth status and metabolic characteristics of plants and reflect the capacity of photosynthetic C fixation when N and P are absorbed simultaneously (Wang et al., 2015). Rong et al. (2015) indicated that C:N and C:P mass ratios can represent the balance between competitive and defensive life strategies in plants. High N and P concentrations in plants likely result in low C:N and C:P mass ratios, suggesting that plants adopt a competitive strategy with a high photosynthetic rate. On the contrary, high C:N and C:P mass ratios imply that plants adopt a strong defense life strategy with a low photosynthetic rate (Sterner and Elser, 2002; Wright et al., 2004; Poorter and Bongers, 2006; Shipley et al., 2006; Rong et al., 2015). For example, the leaf C:P mass ratio of a desert plant T. chinensis is positively related to soil salt concentration, suggesting that T. chinensis uses a defensive life history strategy under salt stress with high C:N and C:P mass ratios (Rong et al., 2015; Wang et al., 2015; Zeng et al., 2017).
N:P mass ratio represents a dynamic balance between soil nutrients and plant nutrition demands and indicates the nutrient limitation of plants at a community level (Koerselman and Meuleman, 1996; Aerts and Chapin, 1999; Güsewell, 2004; He et al., 2008; Nikolic et al., 2014; Rong et al., 2015). For example, in a habitat exposed to salt stress, the leaf N:P mass ratio of T. chinensis is less than 14, indicating that the growth of T. chinensis is limited by N at the beginning of the growing season; however, the leaf N:P mass ratio is greater than 16, suggesting that the P limitation at the middle and final growing seasons (Rong et al., 2015). Therefore, C:N, C:P, and N:P mass ratios are important indicators of the efficiencies of C fixation and nutrient utilization in plants. Thus, investigations on the variations in C, N, and P and C:N, C:P, and N:P mass ratios in plants help elucidate plant growth strategy and adaptability to changes in environments and habitats and thus provide a basis for the development of strategies and policies to facilitate ecological conservation and environmental protection (Rong et al., 2015; Wang et al., 2015; Zeng et al., 2017).
The ecological stoichiometry of a specific plant species and its adaptation to extreme environments have been widely investigated. Variations in leaf N and P concentrations at genus or species levels have also been examined (Reich and Oleksyn, 2004; Kang et al., 2011; Wu et al., 2012; Rong et al., 2015; Zeng et al., 2017). Many studies on ecological stoichiometry have been conducted in the presence of different environmental factors, such as nutrients, light, prescribed burning, and plantation age (Herbert et al., 2003; Striebel et al., 2008; Xing et al., 2013; Butler et al., 2017; Zeng et al., 2017). Li W. et al. (2013) and Li W. et al. (2015) also found that water depth gradients remarkable affected the C, N, and P concentrations and mass ratios of macrophytes in Lake Erhai.
The ecological stoichiometry of terrestrial plants, especially desert phreatophytes with deep root systems, at a reduced groundwater depth in arid regions has been rarely reported. In the arid region of southwestern Xinjiang in China, groundwater table gradually reaches deeper layers in desert-oasis transition ecotone because of intensive human intervention, indicating a shortage of water resources (Liu, 2007). The decline of groundwater depth affects plant physiological adaptations, such as biomass accumulation, photosynthesis, and water use efficiency, thereby influencing species composition and community structure in ecosystems (Nilsson et al., 1997; Rood et al., 2000; Horton et al., 2003; Imada et al., 2008; Li et al., 2010; Chen et al., 2011; Gui et al., 2013; Li C.J. et al., 2015).
As one of the dominant phreatophytes in an arid desert ecosystem, Alhagi sparsifolia Shap. is a spiny herb that belongs to Fabaceae family and grows in China, West Asia, America, North Africa, and Mediterranean regions (Zeng and Liu, 2012). A. sparsifolia is a dominant perennial species that stabilizes sand dunes, prevents land erosion in arid and semiarid regions between oasis and deserts, and supports a fragile desert ecosystem (Zeng and Liu, 2012; Li C.J. et al., 2015). A. sparsifolia has a high crude protein concentration and serves as a major food source for herds in an oasis foreland. Therefore, A. sparsifolia is a vital food of the local farmers’ large livestock; thus it has a remarkable economic value (Li H.F. et al., 2013; Zhang et al., 2018).
The deep root system of A. sparsifolia can reach groundwater, which is a major source of water and nutrients (Arndt et al., 2004; Zeng et al., 2006). In our previous studies, the decline in groundwater depth affects the leaf physiological parameters, root characteristics, clonal growth, and propagation traits of A. sparsifolia (Liu et al., 2009; Zhang et al., 2011; Gui et al., 2013; Zeng et al., 2013; Peng et al., 2014). Therefore, in the present study, we hypothesized that the depth of groundwater would have a major effect on the ecological stoichiometry of A. sparsifolia through its influence on the absorption and utilization of nutrients, namely, C, N, and P.
The study site was located at the Cele National Station of Observation and Research for Desert and Grassland Ecosystem (Cele Station; 37°00′56.37″ N, 80°43′81″ E) at the southern rim of the Taklamakan Desert in the Xinjiang Uygur Autonomous Region of China. This station is found at the desert-oasis transition ecotone, which is characterized by a 5–10 km belt of sparse phreatophytic species, dominated by A. sparsifolia (Zeng et al., 2013). The groundwater depth in this area ranges from 2.5 to 15.0 m. The study site has an elevation of 1366 m (asl), a mean annual temperature of 11.9°C, a mean annual potential evaporation of 2600 mm, and a mean annual precipitation of only 35 mm (Gui et al., 2013; Liu et al., 2016). Extreme temperatures can reach -31°C in winter and 49°C in summer. Thus, the climate is arid, with hot and dry summers and cold and dry winters. Soils in this area are sandy with a bulk density of 1.35 g/cm3 (Liu et al., 2013, 2016).
Three sites with varying groundwater depths were selected: 2.5 (37°01′18″ N, 80°42′29″ E), 4.5 (37°00′40″ N, 80°42′13″ E), and 11.0 m (37°00′33″ N, 80°42′25″ E). Each site has a large area of approximately 2 hectares. At each sampling stage, three plots with an area of 9 m2 were selected for each groundwater depth. In the experimental period from June to October in 2015 and 2016, samplings were conducted monthly. Therefore, total 30 plants were sampled over time in each plot and total 45 samplings were performed in each year. In this study, a large plot area and a replicate number were enough to minimize the effect of research location difference. As such, a typical randomized complete block design was not used. This experimental design is common in similar ecological studies investigating the adaptability of plants to adverse environments (Wang et al., 2015; Zeng et al., 2017). Precipitation at the Cele Station was 34.2 mm in 2015 and 43.4 mm in 2016. The average temperatures from June to October were 21.4°C in 2015 and 21.3°C in 2016. The groundwater depths in the three sites did not significantly fluctuate between 2015 and 2016.
At each sampling, three A. sparsifolia plants with similar canopy sizes, heights, and stem numbers were selected from each plot. Mature leaves with similar sizes were collected. Leaf samples from four directions and different parts were gathered though inquartation (Rong et al., 2015). The leaf samples were oven dried at 105°C for 15 min, and then at 70°C to a constant weight. All of the leaves were subsequently ground using a miller and sieved through a 1 mm mesh screen for chemical element analysis. The total organic C concentration was extracted with 0.8 mol/L K2Cr2O7 and determined using a modified Mebius method (Nelson and Sommers, 1982). The leaf N concentration was extracted with 10 mol/L NaOH and identified using Kjeldahl acid digestion (Sparks et al., 1996). The leaf P concentration was obtained through using colorimetric analysis after the samples were digested with 0.008 L H2SO4 (Thomas et al., 1967).
In a previous study, we observed no significant difference in the soil organic C, total and available N, and P concentrations below 50 cm soil depth around A. sparsifolia roots at 2.5, 4.5, and 11.0 m groundwater depths at the same research sites (Liu, 2011). However, soil organic C, total and available N, and P concentrations at a soil depth above 20 cm were significantly different at various different groundwater depths. Therefore, each soil sample around the canopy of A. sparsifolia was collected at only 0–20 cm depth in accordance with standardized collection protocols in this research from June to October. Nine soil samples were collected at each groundwater depth in each month, air dried, ground, and passed through a 2 mm sieve. Soil organic C was determined through digestion 0.8 mol/L K2Cr2O7 (Nelson and Sommers, 1975). Soil available (mineral) N was determined by a continuous flow analyzer after extraction with 2 M KCl solution was conducted (Li et al., 2011). Soil available P was determined by colorimetrically after extraction with 0.5 M NaHCO3 solution was performed (Olsen et al., 1954).
Turkey’s test was conducted for multiple comparisons of treatment means. Pearson correlation analysis was carried out to analyze the correlations of the leaf C, N, and P stoichiometry of A. sparsifolia and the corresponding soil C, N, and P stoichiometry. All of the analyses were performed with SPSS 19.0.
Groundwater depth remarkable affected the dry biomass of A. sparsifolia (Figure 1). In 2015 and 2016, dry biomass of A. sparsifolia at 2.5 m was significantly lower than those at 4.5 and 11.0 m (Figures 1A,B).
FIGURE 1. Variations in dry biomass of Alhagi sparsifolia at varying groundwater depths of 2.5, 4.5, and 11.0 m in 2015 (A) and 2016 (B) growing seasons. The vertical bars are standard deviations, and ∗ indicates significant difference at p < 0.05 level among the different groundwater depths.
In both years, groundwater depth remarkable affected the soil C, N, and P concentrations (Figures 2A–F). Whereas the soil C:N, C:P, and N:P mass ratios were hardly affected by groundwater depth (Figures 2G–L). The soil C, N, and P concentrations at 11.0 m were significant higher than those at 2.5 and 4.5 m in both years (Figures 2A–F). No significant variations in these concentrations were observed between 2.5 and 4.5 m.
FIGURE 2. Variations in soil C, N, and P stoichiometry at varying groundwater depths of 2.5, 4.5, and 11.0 m in 2015 (A,C,E,G,I,K) and 2016 (B,D,F,H,J,L) growing seasons. The vertical bars are standard deviations, and ∗ indicates significant difference at p < 0.05 level among the different groundwater depths.
In both years, the groundwater depth significantly affected the leaf P concentration and C:P and N:P mass ratios, but it did not influence the leaf C, N concentrations and C:N mass ratio in A. sparsifolia (Figure 3). The leaf P concentration at 4.5 m was significantly lower than those at 2.5 and 11.0 m (Figures 3E,F). By contrast, the leaf C:P and N:P mass ratios at 4.5 m were significantly higher than those at 2.5 and 11.0 m (Figures 3I–L).
FIGURE 3. Variations in leaf C, N, and P stoichiometry of A. sparsifolia at varying groundwater depths of 2.5, 4.5, and 11.0 m in 2015 (A,C,E,G,I,K) and 2016 (B,D,F,H,J,L) growing seasons. The vertical bars are standard deviations, and ∗ indicates significant difference at p < 0.05 level among the different groundwater depths.
The leaf N and P concentrations of A. sparsifolia at all groundwater depths were the highest in June, but these concentrations significantly decreased from July to October (Figures 3C–F). The leaf C:N and C:P mass ratios increased as the growing season continued (Figures 3G–J). The leaf C and N:P mass ratios were relatively stable in the growing seasons in 2015 and 2016 (Figures 3A,B,K,L).
Soil C concentration was positively correlated with soil N and P concentrations in both years (Table 1). The soil N concentration was correlated positively with soil P concentration. However, the relationship between soil and plant stoichiometric parameters was generally insignificant, but soil P concentration was positively correlated with leaf P concentration in 2015 (Table 2). For example, the plant C and N concentrations were not related to their respective concentrations in soils. Soil N concentration was positively correlated with leaf P concentration, and soil C:N mass ratio was negatively correlated with leaf P concentration in 2015 and 2016 (Table 2).
TABLE 2. Correlation of C, N, and P stoichiometry of A. sparsifolia and soil C, N, and P stoichiometry.
In our study, the biomass of A. sparsifolia increased at 4.5 m compared with that at 2.5 m, whereas this parameter decreased at 11.0 m (Figures 1A,B). This result is in agreement with the findings of Peng et al. (2014), who reported that the biomass of A. sparsifolia in an artificial simulation groundwater depth increases from 0.4 to 1.2 m and decrease at 1.8 and 2.2 m. These results are also consistent with the observation that groundwater is the major source of nutrients and water for A. sparsifolia (Arndt et al., 2004; Zeng et al., 2006), thereby confirming that the change in groundwater depths can remarkable affect the growth of A. sparsifolia. Therefore, if the groundwater resource of Cele oasis continuously declines, then the growth of A. sparsifolia may be severely inhibited.
In this study, the soil C, N, and P concentrations at 11.0 m were significantly higher than those at 2.5 and 4.5 m in 2015 and 2016 (Figures 2A–F). This result is consistent with previous findings, which revealed an increase in soil N concentration with declining groundwater depth in dune slack soils (Rhymes et al., 2016). An increase in soil N concentration at deep groundwater depth is likely caused by a reduction in soil moisture content, which can inhibit denitrification rates, leading to increased N retention and eutrophication effect (Rhymes et al., 2016). Similarly, we believed that dry soil with declining groundwater depth affects the movement and cycle of soil C and P, resulting in increased retention and eutrophication effect in soil C and P. Hence, soil C and P concentrations were higher at 11.0 m than at 2.5 and 4.5 m.
The leaf P concentration and the C:P and N:P mass ratios in A. sparsifolia were significantly affected by groundwater depths in both years (Figure 3). This results is similar to previous findings, indicating that the leaf P concentrations and C:P and N:P mass ratios of the submersed macrophytes are affected by increasing groundwater depths (Li W. et al., 2015). The effect of groundwater depth on leaf P concentration is likely attributed to soil and groundwater that serve as the only sources of P absorbed by A. sparsifolia in the current study; thus this phenomenon can be significantly affected by external environments (Hedin, 2004; Rong et al., 2015). Our results are similar to the observation of Zhang et al. (2018), who demonstrated that soil P concentration significantly affects the growth of A. sparsifolia in deserts. By contrast, our previous studies revealed that high and concentrations in the xylem sap of A. sparsifolia indicate sufficient nutrient supply through groundwater (Arndt et al., 2004; Zeng et al., 2006). However, in the present study, the soil P concentration also showed significant influenced the P uptake of A. sparsifolia in 2015. Therefore, the soil and groundwater depth acted as sources of P absorbed by A. sparsifolia. The leaf P concentration at 4.5 m was obviously depressed, corresponding with a lower soil N concentration. This result is consistent with that of Wang et al. (2015), who found that shortage in N availability hinders P uptake from soils into plants.
Leaf C concentration in A. sparsifolia was relatively stable at 400 g kg-1 regardless of the groundwater depth. Our results are consistent with previous findings, which demonstrated that the C concentration of T. chinensis, a desert plant, is also stable in response to environmental stresses (Rong et al., 2015). Stable C in plant tissues is due to C accumulation determined by C fixation through photosynthesis (Wang et al., 2015). Hence, for leaf C concentration, photosynthesis is more important than soil nutrient concentration. Similar to C concentration, the N concentration of A. sparsifolia was not affected by groundwater depth. N seemed not being a limiting growth factor for A. sparsifolia to adapt to declining groundwater depth in a desert habitat because A. sparsifolia is a leguminous plant that can fix atmospheric N2. This result is consistent with the previous findings, which indicated N2 fixation from air contributes more than 80% of the total leaf N for almost half of A. sparsifolia in the Taklamakan Desert (Arndt et al., 2004).
In this study, the C:P and N:P mass ratios were affected by groundwater depth because of the changes in P concentration in A. sparsifolia. C:N and C:P mass ratios reflect the ability of plants to assimilate C when they simultaneously absorb N and P (Koerselman and Meuleman, 1996; Aerts and Chapin, 1999; Güsewell, 2004; He et al., 2008; Rong et al., 2015). Here, the leaf C:P mass ratio increased at 4.5 m and then decreased at 11.0 m, suggesting that the significant reduction of groundwater level may inhibit the ability of plants to absorb P. Previous studies suggested that the leaf N:P ratio is a vital indicator of the limiting nutrient elements of plants and widely used in a series of ecological stoichiometric studies (Koerselman and Meuleman, 1996; Tessier and Raynal, 2003; Güsewell, 2004; Wu et al., 2012). For example, Güsewell (2004) considered that a N:P mass ratio lower than 10 indicates N limitation for terrestrial plants, while a N:P mass ratio greater than 20 corresponds to P limitation. Zhang et al. (2004) suggested that a N:P mass ratio lower than 21 represents N limitation, while a mass N:P ratio greater than 23 shows P limitation. However, in wetland ecosystems, plant growth is limited by P when N:P > 16 and restricted by N when N:P < 14. Plant growth is also limited by restricted by N and P when 14 < N:P < 16 (Koerselman and Meuleman, 1996; Wu et al., 2012). In the present study, the leaf N:P mass ratio of A. sparsifolia at 2.5, 4.5, and 11.0 m ranged between 13.20 and 19.34, between 16.53 and 27.15, and between 11.34 and 23.14 in 2015, respectively, and between 9.64 and 14.70, between 12.15, and 17.90, and between 9.51 and 14.96 in 2016, respectively. These results suggested neither N nor P was a limiting factor of A. sparsifolia growth at different groundwater depths. This result was consistent a previous finding, which revealed that A. sparsifolia is a leguminous plant that can fix atmospheric N2 from the atmosphere; atmospheric N2 fixation contributes more than 80% of the total leaf N for almost half of A. sparsifolia in the Taklamakan Desert (Arndt et al., 2004). Therefore, A. sparsifolia was not limited by N in its habitat.
The leaf N:P mass ratio of A. sparsifolia at 4.5 m was significantly higher than those at 2.5 and 11.0 m (Figures 3I–L). The leaf P concentration at 4.5 m was significantly lower than those at 2.5 and 11.0 m (Figures 3E,F). These results suggested that the growth of A. sparsifolia at 4.5 m was affected by P concentration likely because P concentration in A. sparsifolia at 4.5 m might be diluted by increased plant biomass (Figure 1). This assumption is supported by the fact that soil P concentration at 4.5 m was similar to that at 2.5 m. Moreover, this result is consistent with a previous finding, which revealed that soil P concentration significantly affects the growth of A. sparsifolia in deserts (Zhang et al., 2018).
Although previous studies found that significantly positive correlations exist between soil nutritional status and plant nutrient concentration, these studies did not conclude a solid relationship between soil nutrient concentration and plant C, N, and P stoichiometry (Wang et al., 2015; Zeng et al., 2017). In our study, a positive relationship was observed between soil N and P concentrations of A. sparsifolia (Table 2), suggesting that the increased level of soil N may have increased the uptake of N in plants; as a result, A. sparsifolia absorbs more P because of elemental homeostasis in plants (Elser et al., 2000; Cross et al., 2005; Rong et al., 2015; Zhan et al., 2017). Therefore, in our study, soil N was a key factor that affected the C, N, and P stoichiometry of A. sparsifolia under different groundwater depths because soil N concentration was also significantly correlated with soil P concentration and C:P mass ratio (Table 1). Furthermore, this result was supported by previous findings, indicating that soil P concentration is positively associated with soil N (Cleveland and Liptzin, 2007; Hume et al., 2016), and shortage of N availability in soil affects the P uptake of plants (Wang et al., 2015). Therefore, groundwater depths significantly influenced soil nutrients, especially soil N, resulting in variations in the leaf P concentration, C:P mass ratio of A. sparsifolia because of the interactive relationships of soil C, N, and P. In contrast to previous studies, our study demonstrated that the source of nutrients absorbed by A. sparsifolia was not only groundwater but also soil.
Previous studies showed that competitive and defensive life strategies are determined by the changes in C:N and C:P mass ratios (Rong et al., 2015). A higher plant growth rate is mainly accompanied by low C:N and C:P mass ratios. These two parameters are also effective in reflecting plant growth status and health condition; for example, a rapid growth rate is strongly correlated with decreased C:P mass ratio (Elser et al., 2000; Sterner and Elser, 2002). Rong et al. (2015) reported that high C:N and C:P mass ratios in the middle stage of T. chinensis growth indicate that a defensive strategy of plants grown in coastal wetlands because of high temperatures, intensive soil evaporation, and plant transpiration. In our study, the leaf C:N and C:P mass ratios of A. sparsifolia at 4.5 m were significantly higher than those at 2.5 and 11.0 m (Figures 3G,J), suggesting that A. sparsifolia at 4.5 m probably employed a defensive strategy. This result is also consistent with the finding that the largest biomass (Figure 1) and lowest leaf P concentration of A. sparsifolia were observed at 4.5 m among the various groundwater depths (Figures 3E,F). A. sparsifolia at 2.5 and 11.0 m groundwater depths probably undertook a competitive strategy because of the lower leaf C:N and C:P ratios (Figures 3G,J), lower biomass of A. sparsifolia (Figure 1), and higher leaf P concentration (Figures 3E,F) than those at 4.5 m.
Further specific studies are needed to investigate the efficiency of the resorption and utilization of C, N, and P at the declining groundwater depth. This study helps facilitate the ecological conservation and environmental protection of the desert-oasis ecotone of the southern rim of the Taklamakan Desert in China.
Groundwater depth significantly influenced the biomass, soil C, N, and P concentrations, and leaf P concentration of A. sparsifolia. A. sparsifolia had larger biomass and lower leaf P concentration at 4.5 m than at 2.5 and 11.0 m groundwater depths. The soil C, N, and P concentrations at 4.5 m were significantly lower than those at 11.0 m. The soil N concentration was significantly and positively correlated with leaf P concentration at all of the groundwater depths. The leaf P concentration of A. sparsifolia was lower at 4.5 m than at 2.5 and 11.0 m likely because of a biomass dilution effect. By contrast, the leaf C and N concentrations were generally unaffected by groundwater depth, confirming that C and N accumulations in A. sparsifolia were predominantly determined by C fixation through photosynthesis and biological atmospheric N2 fixation, respectively. As a result, the C:N and C:P mass ratios of A. sparsifolia at 4.5 m were higher than those at the groundwater depths, implying a defensive life history strategy. Conversely, A. sparsifolia likely adopted a competitive strategy at 2.5 and 11.0 m as indicated by the lower C:N, and C:P mass ratios that those at 4.5 m. Our results could facilitate the understanding of the C, N, and P stoichiometry of desert phreatophytes and their responses to the declining groundwater depth in the desert-oasis ecotone of northwest China. This study could further enrich the existing stoichiometric studies.
BZ and FZ: conception and design, analysis and interpretation and critical revision of the article, and overall responsibility; XG and MS: revise manuscript; LL, YL, GL, and CH: data analysis; DG: data collection.
This study was supported by the Key Program of Joint Funds of the National Natural Science Foundation of China and the Government of Xinjiang Uygur Autonomous Region of China (No. U1603233), the National Natural Science Foundation of China (Nos. 31500367, 41371516), and the China 1000 Talent Program (No. Y472171).
The authors declare that the research was conducted in the absence of any commercial or financial relationships that could be construed as a potential conflict of interest.
We would like to thank Dr. Bo Liu, Shenglong Zhao, Hanlin Luo, Shiming Li, and Bo Wang for assistance with in field work at the Cele station; Mingfang Hu for assistance in soil and plant elemental analysis; Rong Huang for modifications in this paper. We are also grateful to the three anonymous referees for their reviewing of this manuscript.
Aerts, R., and Chapin, F. III. (1999). The mineral nutrition of wild plants revisited: a reevaluation of processes and patterns. Adv. Ecol. Res. 30, 1–67. doi: 10.1016/S0065-2504(08)60016-1
Arndt, S. K., Kahmen, A., Arampatsis, C., Popp, M., and Adams, M. (2004). Nitrogen fixation and metabolism by groundwater-dependent perennial plants in a hyperarid desert. Oecologia 141, 385–394. doi: 10.1007/s00442-004-1655-7
Butler, O. M., Lewis, T., and Chen, C. R. (2017). Prescribed fire alters foliar stoichiometry and nutrient resorption in the understorey of a subtropical eucalypt forest. Plant Soil 410, 181–191. doi: 10.1007/s11104-016-2995-x
Chen, Y. P., Chen, Y. N., Xu, C. C., and Li, W. H. (2011). Photosynthesis and water use efficiency of Populus euphratica in response to changing groundwater depth and CO2 concentration. Environ. Earth Sci. 62, 119–125. doi: 10.1007/s12665-010-0502-x
Cleveland, C. C., and Liptzin, D. (2007). C:N:P stoichiometry in soil: is there a “Redfield ratio” for the microbial biomass? Biogeochemistry 85, 235–252. doi: 10.1007/s10533-007-9132-0
Cross, W. F., Benstead, J. P., Frost, P. C., and Thomas, S. A. (2005). Ecological stoichiometry in freshwater benthic systems: recent progress and perspectives. Freshw. Biol. 50, 1895–1912. doi: 10.1111/j.1365-2427.2005.01458.x
Elser, J., Sterner, R., Gorokhova, E., Fagan, W., Markow, T., Cotner, J., et al. (2000). Biological stoichiometry from genes to ecosystems. Ecol. Lett. 3, 540–550. doi: 10.1046/j.1461-0248.2000.00185.x
Elser, J. J., Dobberfuhl, D. R., MacKay, N. A., and Schampel, J. H. (1996). Organism size, life history, and N:P stoichiometry. Bioscience 46, 674–684. doi: 10.2307/1312897
Gao, Y., Yu, G., and He, N. (2013). Equilibration of the terrestrial water, nitrogen, and carbon cycles: advocating a health threshold for carbon storage. Ecol. Eng. 57, 366–374. doi: 10.1016/j.ecoleng.2013.04.011
Gui, D. W., Zeng, F. J., Liu, Z., and Zhang, B. (2013). Characteristics of the clonal propagation of Alhagi sparsifolia Shap. (Fabaceae) under different groundwater depths in Xinjiang, China. Rangeland J. 35, 355–362. doi: 10.1071/RJ13004
Güsewell, S. (2004). N:P ratios in terrestrial plants: variation and functional significance. New Phytol. 164, 243–266. doi: 10.1111/j.1469-8137.2004.01192.x
Han, W. X., Fang, J. Y., Guo, D. L., and Zhang, Y. (2005). Leaf nitrogen and phosphorus stoichiometry across 753 terrestrial plant species in China. New Phytol. 168, 377–385. doi: 10.1111/j.1469-8137.2005.01530.x
He, J. S., Wang, L., Flynn, D. F., Wang, X., Ma, W., and Fang, J. Y. (2008). Leaf nitrogen: phosphorus stoichiometry across Chinese grassland biomes. Oecologia 155, 301–310. doi: 10.1007/s00442-007-0912-y
Hedin, L. O. (2004). Global organization of terrestrial plant–nutrient interactions. Proc. Natl. Acad. Sci. U.S.A. 101, 10849–10850. doi: 10.1073/pnas.0404222101
Herbert, D. A., Williams, M., and Rastetter, E. B. (2003). A model analysis of N and P limitation on carbon accumulation in Amazonian secondary forest after alternate land-use abandonment. Biogeochemistry 65, 121–150. doi: 10.1023/A:1026020210887
Horton, J. L., Hart, S. C., and Kolb, T. E. (2003). Physiological condition and water source use of Sonoran Desert riparian trees at the Bill Williams River, Arizona, USA. Isotopes Environ. Health Stud. 39, 69–82. doi: 10.1080/1025601031000096772
Hume, A., Chen, H. Y. H., Taylor, A. R., Kayahara, G. J., and Man, R. (2016). Soil C:N:P dynamics during secondary succession following fire in the boreal forest of central Canada. For. Ecol. Manag. 369, 1–9. doi: 10.1016/j.foreco.2016.03.033
Imada, S., Yamanaka, N., and Tamai, S. (2008). Water table depth affects Populus alba fine root growth and whole plant biomass. Funct. Ecol. 22, 1018–1026. doi: 10.1111/j.1365-2435.2008.01454.x
Kang, H., Zhuang, H., Wu, L., Liu, Q., Shen, G., Berg, B., et al. (2011). Variation in leaf nitrogen and phosphorus stoichiometry in Picea abies across Europe: an analysis based on local observations. For. Ecol. Manag. 261, 195–202. doi: 10.1016/j.foreco.2010.10.004
Koerselman, W., and Meuleman, A. F. (1996). The vegetation N:P ratio: a new tool to detect the nature of nutrient limitation. J. Appl. Ecol. 33, 1441–1450. doi: 10.2307/2404783
Li, C. J., Zeng, F. J., Zhang, B., Liu, B., Guo, Z. C., Gao, H. H., et al. (2015). Optimal root system strategies for desert phreatophytic seedlings in the search for groundwater. J. Arid Land 7, 462–474. doi: 10.1007/s40333-015-0006-3
Li, H. F., Zeng, F. J., Gui, D. W., Zhang, L. G., Song, C., Luo, W. C., et al. (2013). Effects of cutting and burning on regeneration of Alhagi sparsifolia Shap. on the southern fringe of the Taklamakan Desert, North-west China. Rangeland J. 34, 389–397. doi: 10.1071/RJ12020
Li, L., Gao, X. P., Gui, D. W., Liu, B., Zhang, B., and Li, X. Y. (2017). Stoichiometry in aboveground and fine roots of Seriphidium korovinii in desert grassland in response to artificial nitrogen addition. J. Plant Res. 130, 689–697. doi: 10.1007/s10265-017-0930-8
Li, W., Cao, T., Ni, L. Y., Zhang, X., Zhu, G. R., and Xie, P. (2013). Effects of water depth on carbon, nitrogen and phosphorus stoichiometry of five submersed macrophytes in an in situ experiment. Ecol. Eng. 61, 358–365. doi: 10.1016/j.ecoleng.2013.09.028
Li, W., Cao, T., Ni, L. Y., Zhu, G. R., Zhang, X. L., Fu, H., et al. (2015). Size-dependent C, N and P stoichiometry of three submersed macrophytes along water depth gradients. Environ. Earth Sci. 74, 3733–3738. doi: 10.1007/s12665-015-4295-9
Li, X. F., Wang, J., Huang, D., Wang, L. X., and Wang, K. (2011). Allelopathic potential of Artemisia frigida and successional changes of plant communities in the northern China steppe. Plant Soil 341, 383–398. doi: 10.1007/s11104-010-0652-3
Li, X. Y., Lin, L. S., Zhao, Q., Zhang, X. M., and Thomas, F. M. (2010). Influence of groundwater depth on species composition and community structure in the transition zone of Cele oasis. J. Arid Land 2, 235–242.
Liu, B., He, J. X., Zeng, F. J., Lei, J. Q., and Arndt, S. K. (2016). Life span and structure of ephemeral root modules of different functional groups from a desert system. New Phytol. 211, 103–112. doi: 10.1111/nph.13880
Liu, B., Zeng, F.-J., Arndt, S.-K., He, J.-X., Luo, W.-C., and Song, C. (2013). Patterns of root architecture adaptation of a phreatophytic perennial desert plant in a hyperarid desert. S. Afr. J. Bot. 86, 56–62. doi: 10.1016/j.sajb.2013.02.003
Liu, B., Zeng, F. J., Guo, H. F., and Zeng, J. (2009). Effects of groundwater table on growth characteristics of Alhagi sparsifolia Shap. seedlings. Chin. J. Ecol. 28, 237–242.
Liu, M. (2007). Study on Groundwater Spatiotemporal Distribution Law and its Environmental Effects in Hotan Oasis. Master thesis, Xi’an University of Technology, Shaanxi.
Liu, Z. (2011). Root Distribution of Alhagi sparsifolia Shap. Response to Different Water Conditions. Master thesis, Chinese Academy of Sciences, Beijing.
Marschner, P. (2012). Marschner’s Mineral Nutrition of Higher Plants: Functions of Macronutrients. London: Academic Press, 135–189.
Nelson, D., and Sommers, L. E. (1982). “Total carbon, organic carbon, and organic matter,” in Methods of Soil Analysis: Chemical and Microbiological Properties, Pt 2, eds A. L. Page, R. H. Miller, and D. R. Keeney (Madison, WI: Soil Science Society of America), 539–579.
Nelson, D. W., and Sommers, L. E. (1975). A rapid and accurate method for estimating organic carbon in soil. Proc. Indiana Acad. Sci. 84, 456–462.
Nikolic, N., Böcker, R., Kostic-Kravljanac, L., and Nikolic, M. (2014). Assembly processes under severe abiotic filtering: adaptation mechanisms of weed vegetation to the gradient of soil constraints. PLoS One 9:e114290. doi: 10.1371/journal.pone.0114290
Nilsson, C., Jansson, R., and Zinko, U. (1997). Long-term responses of river-margin vegetation to water-level regulation. Science 276, 798–800. doi: 10.1126/science.276.5313.798
Olsen, S. R., Cole, C. V., Watanabe, F. S., and Dean, L. A. (1954). Estimation of available organic carbon in soil. Proc. Indiana Acad. Sci. 84, 456–462.
Peng, S. L., Zeng, F. J., Wang, H. T., Guo, J. H., Gao, H. H., Luo, W. C., et al. (2014). Effects of water table depth on leaf morphology and growth of Alhagi sparsifolia seedlings. J. Desert Res. 34, 390–404.
Poorter, L., and Bongers, F. (2006). Leaf traits are good predictors of plant performance across 53 rain forest species. Ecology 87, 1733–1743. doi: 10.1890/0012-9658 (2006)87[1733:LTAGPO]2.0.CO;2
Reich, P. B., and Oleksyn, J. (2004). Global patterns of plant leaf N and P in relation to temperature and latitude. Proc. Natl. Acad. Sci. U.S.A. 101, 11001–11006. doi: 10.1073/pnas.0403588101
Rhymes, J., Jones, L., Wallace, H., Jones, T. G., Dunn, C., and Fenner, N. (2016). Small changes in water levels and groundwater nutrients alter nitrogen and carbon processing in dune slack soils. Soil Biol. Biochem. 99, 28–35. doi: 10.1016/j.soilbio.2016.04.018
Rong, Q. Q., Liu, J. T., Cai, Y. P., Lu, Z. H., Zhao, Z. Z., Yue, W. C., et al. (2015). Leaf carbon, nitrogen and phosphorus stoichiometry of Tamarix chinensis Lour. in the Laizhou Bay coastal wetland, China. Ecol. Eng. 76, 57–65. doi: 10.1016/j.ecoleng.2014.03.002
Rood, S. B., Zanewich, K., Stefura, C., and Mahoney, J. M. (2000). Influence of water table decline on growth allocation and endogenous gibberellins in black cottonwood. Tree Physiol. 20, 831–836. doi: 10.1093/treephys/20.12.831
Shipley, B., Lechowicz, M. J., Wright, I., and Reich, P. B. (2006). Fundamental trade-offs generating the worldwide leaf economics spectrum. Ecology 87, 535–541. doi: 10.1890/05-1051
Sparks, D. L., Page, A., Helmke, P., Loeppert, R., Soltanpour, P., Tabatabai, M., et al. (1996). Methods of Soil Analysis: Chemical Methods, Pt 3. Madison, WI: Soil Science Society of America Inc.
Sterner, R. W. (1995). Elemental Stoichiometry of Species in Ecosystems. Linking Species & Ecosystems. Berlin: Springer, 240–252. doi: 10.1007/978-1-4615-1773-3_23
Sterner, R. W., and Elser, J. J. (2002). Ecological Stoichiometry: The Biology of Elements from Molecules to the Biosphere. Princeton, NJ: Princeton University Press.
Striebel, M., Sporl, G., and Stibor, H. (2008). Light-induced changes of plankton growth and stoichiometry: experiments with natural phytoplankton communities. Limnol. Oceanogr. 53, 513–522. doi: 10.4319/lo.2008.53.2.0513
Tessier, J. T., and Raynal, D. J. (2003). Use of nitrogen to phosphorus ratios in plant tissue as an indicator of nutrient limitation and nitrogen saturation. J. Appl. Ecol. 40, 523–534. doi: 10.1046/j.1365-2664.2003.00820.x
Thomas, R., Sheard, R., and Moyer, J. (1967). Comparison of conventional and automated procedures for nitrogen, phosphorus, and potassium analysis of plant material using a single digestion. Agron. J. 59, 240–243. doi: 10.2134/agronj1967.00021962005900030010x
Venterink, H. O., and Güsewell, S. (2010). Competitive interactions between two meadow grasses under nitrogen and phosphorus limitation. Funct. Ecol. 24, 877–886. doi: 10.1111/j.1365-2435.2010.01692.x
Vrede, T., Dobberfuhl, D. R., Kooijman, S., and Elser, J. J. (2004). Fundamental connections among organism C:N:P stoichiometry, macromolecular composition, and growth. Ecology 85, 1217–1229. doi: 10.1890/02-0249
Wang, Z., Lu, J., Yang, M., Yang, H., and Zhang, Q. (2015). Stoichiometric characteristics of carbon, nitrogen, and phosphorus in leaves of differently aged Lucerne (Medicago sativa) Stands. Front. Plant Sci. 6:1062. doi: 10.3389/fpls.2015.01062
Wright, I. J., Reich, P. B., Westoby, M., Ackerly, D. D., Baruch, Z., Bongers, F., et al. (2004). The worldwide leaf economics spectrum. Nature 428, 821–827. doi: 10.1038/nature02403
Wu, T. G., Yu, M. K., Wang, G. G., Dong, Y., and Cheng, X. R. (2012). Leaf nitrogen and phosphorus stoichiometry across forty-two woody species in Southeast China. Biochem. Syst. Ecol. 44, 255–263. doi: 10.1016/j.bse.2012.06.002
Xing, W., Wu, H. P., Hao, B. B., and Liu, G. H. (2013). Stoichiometric characteristics and responses of submerged macrophytes to eutrophication in lakes along the middle and lower reaches of the Yangtze River. Ecol. Eng. 54, 16–21. doi: 10.1016/j.ecoleng.2013.01.026
Yan, W. M., Zhong, Y. Q. W., Zheng, S. X., and Shangguan, Z. P. (2016). Linking plant leaf nutrients/stoichiometry to water use efficiency on the Loess Plateau in China. Ecol. Eng. 87, 124–131. doi: 10.1016/j.ecoleng.2015.11.034
Zeng, F. J., Bleby, T. M., Landman, P. A., Adams, M. A., and Arndt, S. K. (2006). Water and nutrient dynamics in surface roots and soils are not modified by short-term flooding of phreatophytic plants in a hyperarid desert. Plant Soil 279, 129–139. doi: 10.1007/s11104-005-0498-2
Zeng, F. J., Song, C., Guo, H. F., Liu, B., Luo, W. C., Gui, D. W., et al. (2013). Responses of root growth of Alhagi sparsifolia Shap. (Fabaceae) to different simulated groundwater depths in the southern fringe of the Taklimakan Desert, China. J. Arid Land 5, 220–232. doi: 10.1007/s40333-013-0154-2
Zeng, Q. C., Lal, R., Chen, Y. N., and An, S. S. (2017). Soil, leaf and root ecological stoichiometry of Caragana korshinskii on the Loess Plateau of China in relation to plantation age. PLoS One 12:e0168890. doi: 10.1371/journal.pone.0168890
Zhan, S. X., Wang, Y., Zhu, Z. C., Li, W. H., and Bai, Y. F. (2017). Nitrogen enrichment alters plant N: P stoichiometry and intensifies phosphorus limitation in a steppe ecosystem. Environ. Exp. Bot. 134, 21–32. doi: 10.1016/j.envexpbot.2016.10.014
Zhang, B., Gui, D. W., Gao, X. P., Shareef, M., Li, L., and Zeng, F. J. (2018). Controlling soil factor in plant growth and salt tolerance of leguminous plant Alhagi sparsifolia Shap. in saline deserts, northwest China. Contemp. Probl. Ecol. 11, 111–121. doi: 10.1134/S199542551801002X
Zhang, L. X., Bai, Y. F., and Han, X. G. (2004). Differential responses of N:P stoichiometry of Leymus chinensis and Carex korshinskyi to N additions in a steppe ecosystem in nei mongol. Acta Bot. Sin. 46, 259–270.
Keywords: ecological stoichiometry, desert phreatophyte, Alhagi sparsifolia, groundwater depth, leguminous plant, plant growth strategy
Citation: Zhang B, Gao X, Li L, Lu Y, Shareef M, Huang C, Liu G, Gui D and Zeng F (2018) Groundwater Depth Affects Phosphorus But Not Carbon and Nitrogen Concentrations of a Desert Phreatophyte in Northwest China. Front. Plant Sci. 9:338. doi: 10.3389/fpls.2018.00338
Received: 01 November 2017; Accepted: 28 February 2018;
Published: 15 March 2018.
Edited by:
Miroslav Nikolic, University of Belgrade, SerbiaCopyright © 2018 Zhang, Gao, Li, Lu, Shareef, Huang, Liu, Gui and Zeng. This is an open-access article distributed under the terms of the Creative Commons Attribution License (CC BY). The use, distribution or reproduction in other forums is permitted, provided the original author(s) and the copyright owner are credited and that the original publication in this journal is cited, in accordance with accepted academic practice. No use, distribution or reproduction is permitted which does not comply with these terms.
*Correspondence: Fanjiang Zeng, emVuZ2ZqQG1zLnhqYi5hYy5jbg==
Disclaimer: All claims expressed in this article are solely those of the authors and do not necessarily represent those of their affiliated organizations, or those of the publisher, the editors and the reviewers. Any product that may be evaluated in this article or claim that may be made by its manufacturer is not guaranteed or endorsed by the publisher.
Research integrity at Frontiers
Learn more about the work of our research integrity team to safeguard the quality of each article we publish.