- 1Jilin Provincial Key Laboratory of Grassland Farming, Northeast Institute of Geography and Agroecology, Chinese Academy of Sciences, Changchun, China
- 2College of Life Sciences, Northeast Normal University, Changchun, China
- 3Department of Biology, University of Kentucky, Lexington, KY, United States
- 4Department of Plant and Soil Sciences, University of Kentucky, Lexington, KY, United States
Since seed reserves can influence seed germination, the quantitative and qualitative differences in seed reserves may relate to the germination characteristics of species. The purpose of our study was to evaluate the correlation between germination and seed reserves, as well as their mobilization during germination of six grassland species (Chloris virgata, Kochia scoparia, Lespedeza hedysaroides, Astragalus adsurgens, Leonurus artemisia, and Dracocephalum moldavica) and compare the results with domesticated species. We measured starch, protein, and fat content in dry seeds and the initial absorption of water during imbibition. Starch, soluble protein, fat, and soluble sugar content also were determined at five stages during germination. Starch, protein, and fat reserves in dry seeds were not significantly correlated with germination percentage and rate (speed), but soluble sugar and soluble protein contents at different germination stages were positively significantly correlated with germination rate for the six species. Starch was mainly used during seed imbibition, and soluble protein was used from the imbibition stage to the highest germination stage. Fat content for all species remained relatively constant throughout germination for six species, regardless of the proportion of other seed reserves in the seeds. Our results for fat utilization differ from those obtained for cultivated grasses and legumes. These results provide new insight on the role of seed reserves as energy resources in germination for wild species.
Introduction
Seed germination is the beginning of the life history for seed plants (Donohue et al., 2010; Nonogaki et al., 2010; Lai et al., 2015). It has been suggested that one way in which the environment indirectly affects seed germination is through the types and amounts of compounds transferred from the mother plant to the seeds (Donohue, 2009; Baskin and Baskin, 2014; Li et al., 2017). This transfer of compounds to seeds includes carbohydrates, proteins and lipids, which are the major reserves in most seeds (Alencar et al., 2012).
The definition of germination sensu stricto is the complex process from water uptake of dry seeds (imbibition) to radicle protrusion through the seed coat (Weitbrecht et al., 2011; Rajjou et al., 2012). With regard to germination, there are two fundamental seed biology questions. What metabolic activities are involved, and how are energy reserves used to support embryo growth and radicle protrusion (Rosental et al., 2014)? Although seed reserve mobilization is usually thought to be a post-germination process (Eastmond and Graham, 2001; Pritchard et al., 2002), some studies have found that seed reserve mobilization or degradation of starch (Galland et al., 2017), protein (Yang et al., 2007; Rosental et al., 2014), and lipid (Sreenivasulu et al., 2008) occurs during germination. Also, storage protein (e.g., 11S globulins) mobilization can occur during the seed maturation process, albeit to a lower extent than during seed germination and seedling establishment (Job et al., 1997; Bourgne et al., 2000; Gallardo et al., 2001).
It has been reported that seed reserve content is correlated with germination percentages and/or rates (speed) (Soriano et al., 2014). For example, the starch content in Citrullus lanatus seeds was positively correlated with germination rate but not with the final germination percentage (Wang et al., 2011). Soluble sugar (such as glucose and sucrose) was positively correlated with germination percentage of Medicago truncatula seeds (Vandecasteele et al., 2011). Protein content was positively correlated with germination percentage of Pinus pinaster (Wahid and Bounoua, 2013) and the germination rate of Solanum tuberosum (Bhatt et al., 1989) seeds. No significant correlation was found between fat content and germination percentage of Linum usitatissimum seeds (Kanmaz and Ova, 2015), but fatty acid content was negatively correlated with germination percentage of Gossypium spp. seeds (Hoffpauir et al., 1950). Thus, the influence of seed reserves on germination depends on the amount of reserve and the plant species. However, little information is available about the effect of the combination of reserves on germination traits at the interspecific level (Soriano et al., 2011). Different seed reserves may have different roles during germination for various species (Gu et al., 2016). For example, oil is utilized during germination of Sorghum bicolor seeds (Elmaki et al., 1999), but it is not essential for germination of Arabidopsis thaliana seeds (Pritchard et al., 2002). Therefore, we hypothesized that neither starch, protein nor fat content of dry seeds may be significantly correlated to germination percentages and rates at the interspecific level.
The mobilization of seed reserves during germination varies with the amount of reserves and the species. Starch was present in the highest amount, and its degradation was highest among the various seed reserves used during germination of Oryza sativa (Palmiano and Juliano, 1972), Sorghum bicolor (Elmaki et al., 1999), and Avena sativa (Xu et al., 2011) seeds. Protein was mainly mobilized and used during germination of the legume species Dalbergia nigra (Ataíde et al., 2013). Seeds of Helianthus annuus (Erbaş et al., 2016) and Sterculia urens (Satyanarayana et al., 2011) have high protein and oil content, which decreased dramatically during germination of these two species. Therefore, we hypothesized that the seed reserve present in the highest amount is the one that is most heavily utilized during germination. Additionally, the various metabolic processes that occur during germination sensu stricto start at different times for individual seeds. Consequently, it is difficult to determine the boundary between germination and seedling growth for a seed population, as seeds do not complete the germination process synchronously (Gallardo et al., 2001). In this context, it is worth noting that seeds are known to be extremely heterogeneous in biochemical terms (Still and Bradford, 1997; Bourgne et al., 2000). Thus, in our study we divided the seed germination process of six species into different stages, including imbibition, 1% germination, 50% germination, highest germination and finally the seedling stage to investigate seed reserve mobilization.
The relationship between seed reserves and germination has been studied mainly in economically-important species such as Oryza sativa (Sun et al., 2015), Glycine max (Bellaloui et al., 2017), Sorghum bicolor (Elmaki et al., 1999), Linum usitatissimum (Kanmaz and Ova, 2015) and in the model plant species Medicago truncatula (Vandecasteele et al., 2011) and Arabidopsis thaliana (Shrestha et al., 2016). These previous studies on seed reserve mobilization during germination have given little attention to wild grassland species and have mainly focused either on only one type of reserves (Kim et al., 2009; Shaik et al., 2014) or a single species (usually crops) (Goyoaga et al., 2011; Ataíde et al., 2013; Lopes et al., 2013).
In our study, we selected six wild species from the Songnen grassland in northeast China to test our hypotheses. These species were selected because the most abundant storage reserve in the seeds was starch, protein, or lipid, and their germination percentages were high, allowing us to collect samples during different germination stages for the seed populations. Specifically, we asked: (1) Does the type and amount of seed reserve influence germination? (2) Is the most abundant reserve compound the most heavily used one during germination? Also, we compared the results for these grassland species with those for cultivated species. Such comparisons would provide some new insights on seed physiology of wild species and on changes that may have occurred in seed reserve utilization/physiology during the domestication of crop species.
Materials and Methods
Study Species, Seed Collection, and Storage
The six wild species selected for study include Chloris virgata, Kochia scoparia, Lespedeza hedysaroides, Astragalus adsurgens, Leonurus artemisia, and Dracocephalum moldavica. These species occur in four families and four of them are annuals and two are perennials (Table 1). Seeds of C. virgata have endosperm but those of the other five species do not have endosperm. Seeds of each species were collected during autumn 2015 from wild populations on the Songnen plain (123° 44–47′ E, 44° 40–45′ N) situated in northeast China. In this region, the average annual temperature and precipitation are around 5°C and 400 mm (calculated from the recent 30 years), respectively (Dong et al., 2011). Seeds were dry-stored in cloth bags at room temperature until the start of the experiment in May 2016. One hundred seeds were selected randomly and weighed with an electronic balance (0.1 mg, METTLER TOLEDO ME204), with five replicates for each species. Thousand seed weights were calculated.

TABLE 1. Family, life cycle, photosynthetic pathway (P), main seed reserve proportion in dry seeds and 1000 seed mass of the six species in this study.
Water Uptake during Imbibition
Seeds were incubated on the surface of two layers of filter paper moistened with distilled water in 10 cm diameter Petri dishes. Four replicates of 25 seeds each were used for each species. Seeds were incubated at a constant temperature of 25°C, with a 12-h daily photoperiod (hereafter light) (Sylvania cool white fluorescent lamps, 25 μmol photons m-2 s-1, PAR). The rate of water absorption was determined for each species, and the experiment was terminated when the first seed(s) with an emerged radicle was(were) observed. According to a preliminary experiment, germination started between 2 and 42 h after seeds were placed on wet filter paper, depending on the species. During the imbibition period, total seed mass in each Petri dish was measured 5–6 times from the beginning of incubation (dry seeds) with the same time interval for each species (Supplementary Table S1). Surface water was removed from seeds with filter paper each time they were weighed. The seeds were put back into the dishes after they were weighed. Water uptake (%) was calculated as (W2–W1) × 100/W1. W1 and W2 represent the mass of seeds at time zero and at various sampling times during imbibition, respectively.
Germination Characteristics
Seeds were immersed in 70% (v/v) ethanol for 1 min for surface sterilization and then washed three times with distilled water. Seeds were incubated on the surface of two layers of filter paper moistened with distilled water in 10 cm diameter Petri dishes, and there were three replicates of 50 seeds each for each species. Distilled water was added when necessary to keep the filter paper moist. The experiment was conducted in light at 25°C. Except for the two fast germinating species, C. virgata and K. scoparia, which were checked at 2 h intervals, germination was recorded every 12 h. Seeds were considered to be germinated when the radicle emerged. The experiment lasted for a total of 7 days, at which time germination was completed. The germination percentage (number of germinated seeds/total seeds) and rate or speed (the reciprocal of time to 50% germination in days) were calculated.
Dynamics of Seed Reserves during Germination
Total starch, protein, and fat content were measured in dry seeds of the six species. To analyze starch content, samples of dry seeds were ground and extracted twice with 80% ethanol and then twice with 52% perchloric acid. Starch content was determined with the anthrone-sulfuric acid method (Fernandes et al., 2012) at 640 nm with a Spectrometer (723 UV-VIS, Shandong, China). Samples of dry seeds were ground and extracted with 98% sulfuric acid. Total nitrogen content of dry seeds was measured with a Continuous Flowing Analyzer (Kjeltec 8400, Germany) and then total protein content was calculated by multiplying nitrogen content by the constant 6.25 (Soriano et al., 2011). To analyze fat content, dry samples of seeds were ground and ethylic-ether was used as extraction buffer. Concentration of fat was determined by the Soxhlet extraction method (Soriano et al., 2011) using Solvent Extractor SER (VELP Scientifica, Italy). The content of starch, protein, and fat (mg/g) in dry seeds was also calculated as a percent of seed mass for the six species (Table 1).
For measuring reserve mobilization during germination of the six species, 0.5 g seeds were weighed and put on moist filter paper in each of 15 Petri dishes for each species and incubated in light at 25°C. Three dishes (biological replicates) of seeds were taken for each species at each of five stages (Supplementary Table S2): imbibition (middle time from incubation to the start of germination, stage 1), start of germination (1% germination, stage 2), 50% germination (stage 3), highest germination (stage 4) and early seedling stage (around 80% with visible cotyledon (s), stage 5). Among the stages, only samples from stage 5 can be treated as seedlings with both shoot and root. Seeds from other stages were imbibed and/or had an emerged radicle. The experiment was performed three times. The first, second and third times the experiment was done, starch and soluble sugar, soluble protein, and fat were analyzed, respectively. The methods of starch and fat measurements during germination were the same as those described above for dry seeds. To analyze the protein fraction that can be used for germination, we measured the content of water-soluble protein (hereafter soluble protein). This part of soluble protein that we extracted could be surmised to be albumin fraction (Osborne, 1924). It is treated as one of the seed reserves in our study, but it may contain other substances, e.g., enzymes. One hundred milligrams of fresh seeds/seedlings were manually ground and extracted with distilled water. After centrifugation, soluble protein content was measured using the Coomassie Brilliant Blue (mixture of Coomassie Brilliant Blue G250, 90% ethanol, 85% phosphoric acid, and distilled water) method (Bradford, 1976) and determined at 595 nm with a Spectrometer (723 UV-VIS, Shandong, China). To analyze water-soluble sugar (hereafter soluble sugar) dynamics, seeds/seedlings were dried at 50°C for 72 h and manually ground. Distilled water was added to the samples and warmed at 80°C for 1 h. After centrifugation, soluble sugar content was determined with the anthrone-sulfuric acid method (Yemm and Willis, 1954) at 625 nm with a Spectrometer (723 UV-VIS, Shandong, China). To compare the changes of each seed reserve during germination at the same scale, the concentration (or content, mg/g) of starch, soluble protein, fat, and soluble sugar at each stage from imbibition (stage 1) to early seedling (stage 5) relative to content present at the imbibition stage was calculated as (C2–C1) × 100/C1, C1 and C2 represent the content (mg/g) at imbibition stage (stage 1) and content (mg/g) at the four subsequent sampling stages (stages 2–5) during germination and early seedling growth, respectively.
Statistical Analysis
Germination data were transformed (arcsine) before statistical analysis was conducted to ensure homogeneity of variance. The correlations between starch, protein, and fat contents in dry seeds, soluble protein and soluble sugar at different germination stages, and germination characters (percentage and rate) were tested by Pearson correlation analysis. Regression analysis was used to fit the relationship between seed reserves and germination stages. One-way ANOVA was used to analyze the effect of species on germination rate and the effect of germination stages on seed reserve contents. Multiple comparison tests were used to compare differences among treatment means at 0.05 level. Statistical analyses were carried out in SPSS (version 18.0, SPSS, Inc., Chicago, IL, United States).
Results
Water Uptake during Imbibition
Seeds of K. scoparia and C. virgata quickly absorbed water during the first 20 min and 2 h of incubation, respectively, with the percentage of water absorbed approaching or exceeding half of the final amount (Figure 1). Imbibition of C. virgata and K. scoparia seeds reached 73 and 81.6% after 10 and 2 h, respectively. For L. hedysaroides and A. adsurgens, water was rapidly imbibed during the first 8 h, after which seeds continued to absorb water. The quick water uptake stage for L. artemisia and D. moldavica was 7 h, after which water content reached 54 and 223%, respectively. We stopped measuring water absorption by D. moldavica seeds after 14 h, because seeds became covered with mucilage that made it impossible to accurately determine the amount of water imbibed by the seeds. Except for D. moldavica with a 223% increase, the highest water uptake during the imbibition of the studied species ranged from 73 to 117%.
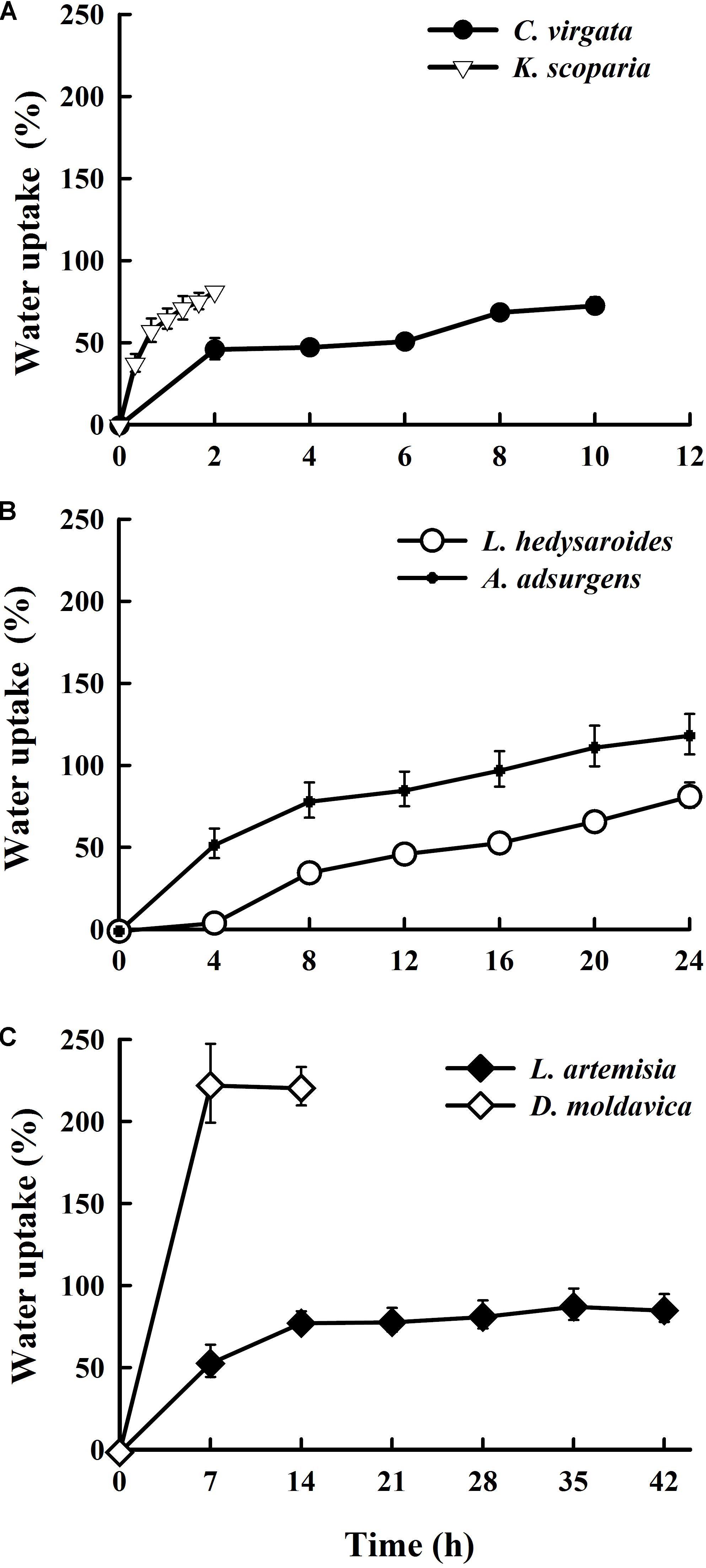
FIGURE 1. Water uptake (%) of Chloris virgata, Kochia scoparia (A), Lespedeza hedysaroides, Astragalus adsurgens (B), Leonurus artemisia, and Dracocephalum moldavica (C) seeds during imbibition at 25°C.
Germination Percentage and Rate
Germination of the five species was ≥80% (Figure 2), with that of A. adsurgens 67%. Seeds of K. scoparia and C. virgata started to germinate after 2 and 10 h, respectively, and reached the highest germination percentage within 8 and 20 h, respectively. Seeds of L. hedysaroides and A. adsurgens began to germinate after 24 h and achieved the maximum after 108 and 84 h, respectively. While seeds of L. artemisia and D. moldavica started to germinate after 44 h and gradually reached the highest germination after 126 h.
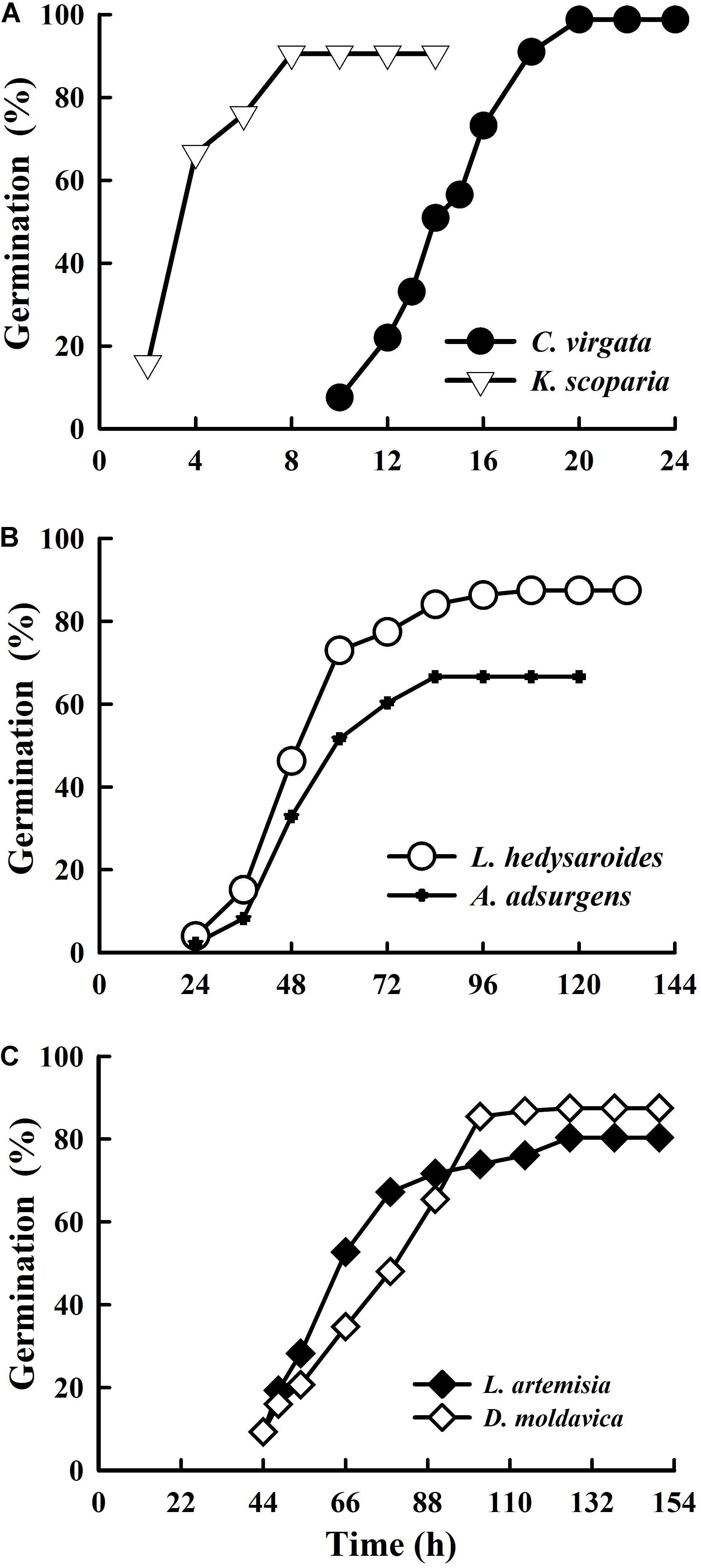
FIGURE 2. Cumulative seed germination percentages of Chloris virgata, Kochia scoparia (A), Lespedeza hedysaroides, Astragalus adsurgens (B), Leonurus artemisia and Dracocephalum moldavica (C) seeds in light at 25°C.
The germination rate of K. scoparia seeds was significantly higher than that of C. virgata seeds (Figure 3), which was 7.05 and 1.67, respectively, both significantly higher than that of the other four species (P < 0.05). Germination rates of the other species were not significantly different from each other, which ranged from 0.31 to 0.48 (P < 0.05).
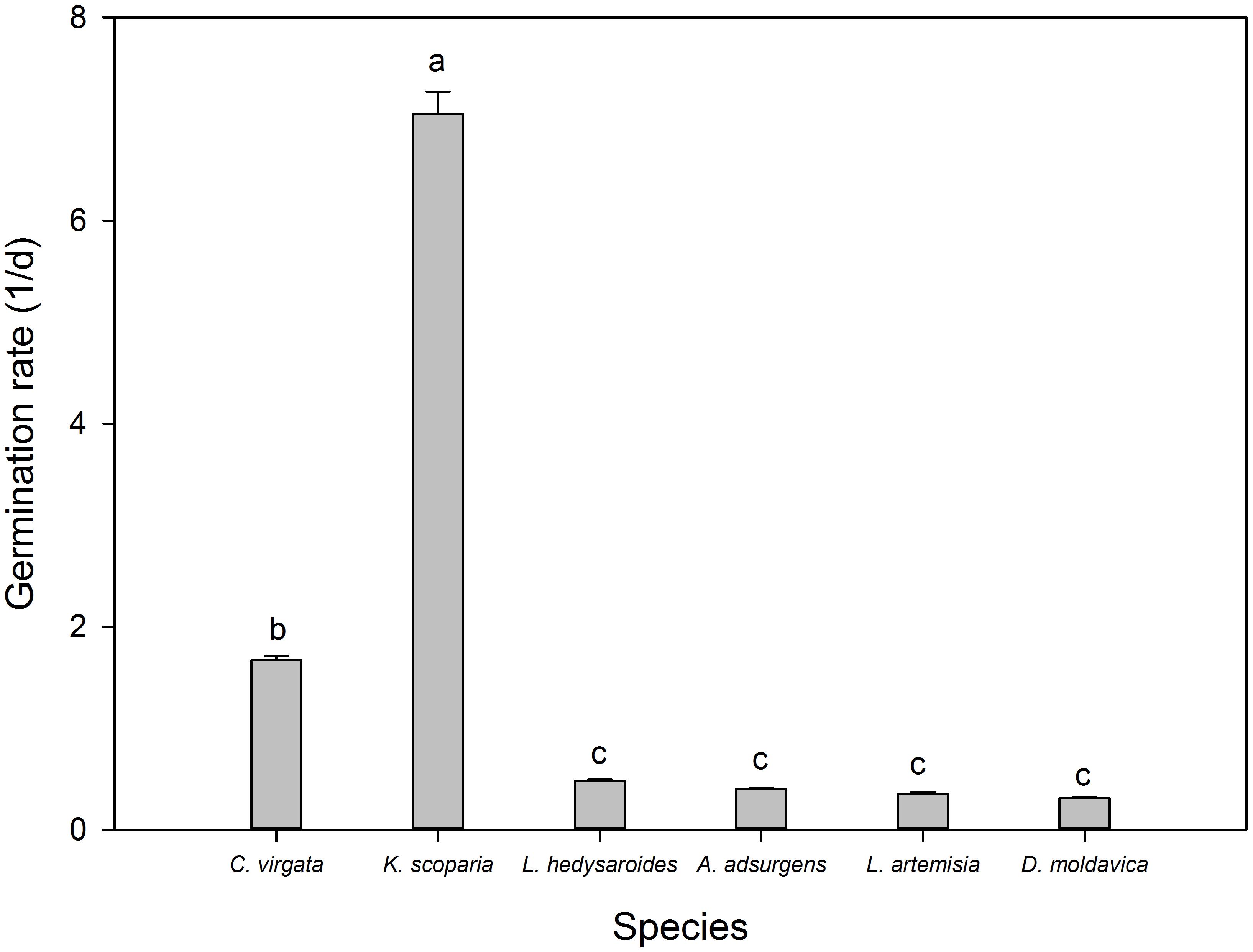
FIGURE 3. Germination rate of the six species Kochia scoparia, Chloris virgata, Astragalus adsurgens, Lespedeza hedysaroides, Leonurus artemisia, and Dracocephalum moldavica. Rates with different lowercase letters differed significantly at 0.05 level.
The Relationship between Seed Reserve Content and Germination
Dry seeds of C. virgata had a relatively high starch content (62.2%), and those of K. scoparia, A. adsurgens, and L. hedysaroides had high levels of starch (47.3–48.2%) and protein (23.3–24.2%) (Table 1). Seeds of L. artemisia had a relatively high fat and starch content (35.8 and 35.2%, respectively). Seeds of D. moldavica had more starch (25.6%) than protein (17.0%) or fat (20.0%), but the percentage of fat was higher than that of the non-Lamiaceae species. Germination percentages and rates were not correlated with the contents of the three major reserves in dry seeds of the six species, but germination rate was significantly correlated with soluble sugar and soluble protein contents at different germination stages for all species (P < 0.05, Table 2 and Supplementary Table S4). Soluble sugar content was positively correlated with the starch content and negatively correlated with the fat content in dry seeds (P < 0.01). Soluble protein content was positively correlated with the protein content in dry seeds (P < 0.01). Additionally, the 1000 seed weight of the six species varied from 0.45 to 2.07 g (Table 1), and germination rate had a significant negative correlation with 1000 seed weight for all species (P < 0.01) (Table 2).
Dynamics of Seed Reserves during Germination
The starch and soluble protein content of the six species significantly decreased with the germination stage (P < 0.05, Figure 4), but fat content of the six species did not significantly change with germination stage (P > 0.05). The soluble sugar content of four of the six species significantly increased with germination stage (P < 0.05), with that of A. adsurgens increasing and then decreasing and that of D. moldavica remaining unchanged.
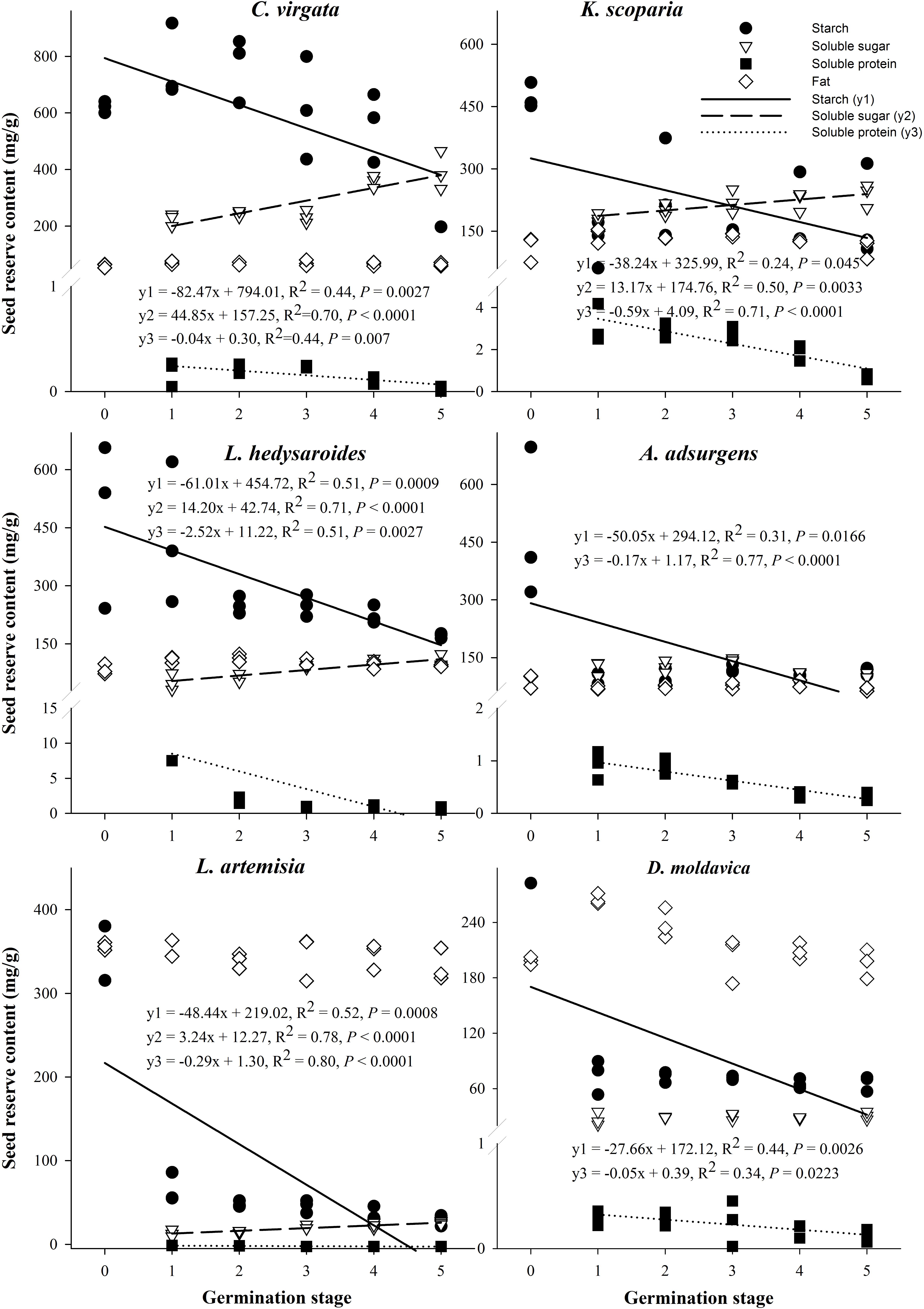
FIGURE 4. Regression analysis between starch, fat, soluble protein, soluble sugar, and germination stage for the six species Kochia scoparia, Chloris virgata, Astragalus adsurgens, Lespedeza hedysaroides, Leonurus artemisia, and Dracocephalum moldavica. (0) Dry seeds; (1) imbibition; (2) 1% germination; (3) 50% germination; (4) highest germination; (5) early seedling.
For all species except C. virgata and L. hedysaroides, there was a significant decrease in starch when imbibed seeds were compared to dry seeds, and this was the main period of starch utilization (Figure 4 and Supplementary Table S3). The main period of starch utilization for L. hedysaroides was during the imbibition (stage 1) to 1% germination (stage 2) (Figure 5), but the decrease in starch was not significant (P < 0.05). For C. virgata, starch content decreased significantly during the highest germination (stage 4) to early seedling stage (stage 5). The decrease in starch content ranged from 10.1 to 89.7% during the period from dry seeds to highest germination (stage 4) for the six species. Soluble protein content decreased to a larger proportion than the other two reserves from imbibition (stage 1) to the early seedling stage (stage 5) for the six species (Figure 5). However, a significant decrease occurred at different germination stages and the decrease ranged from 39.1 to 93.9% during imbibition (stage 1) to highest germination (stage 4) for the six species.
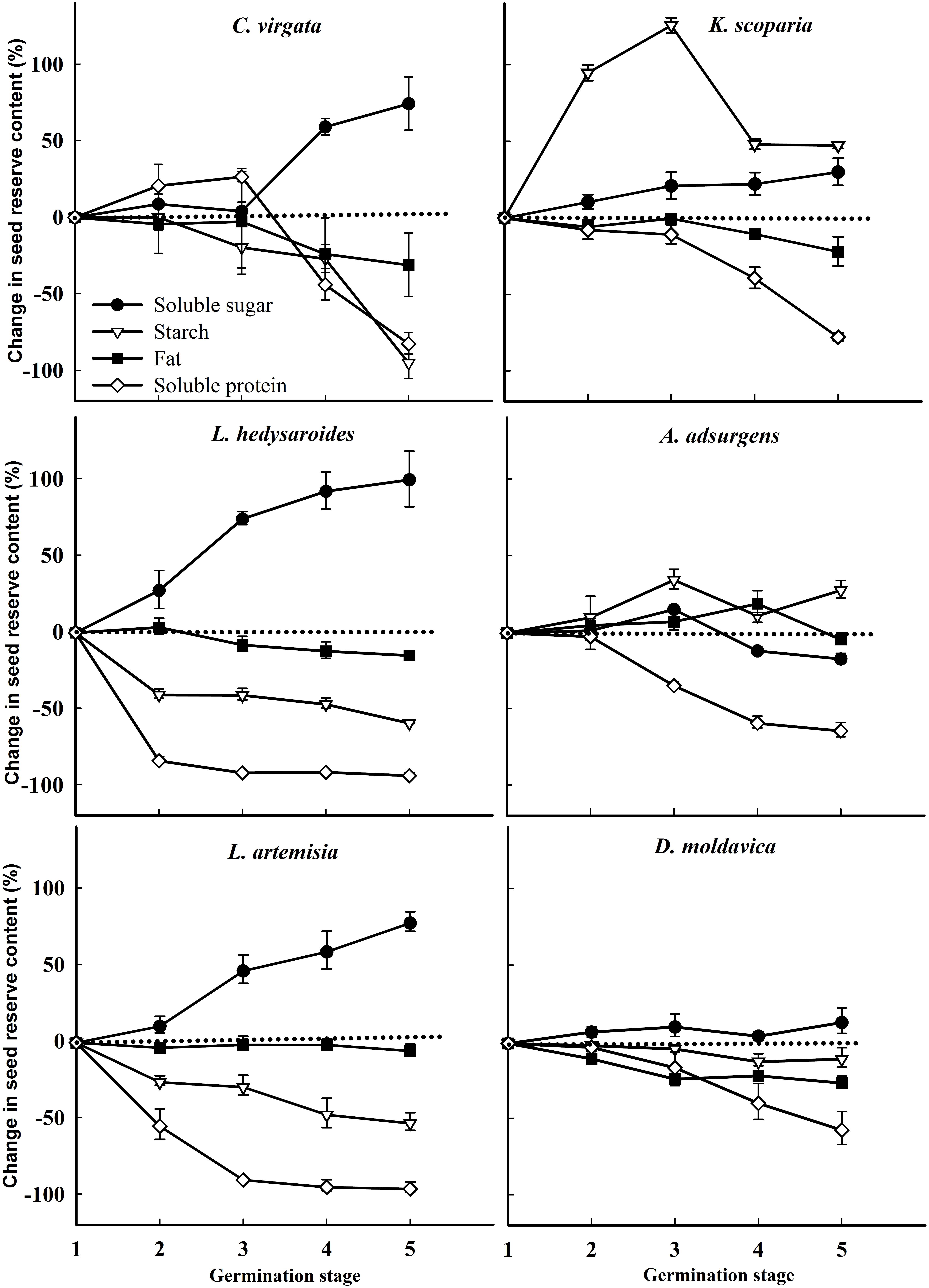
FIGURE 5. Change (%) in seed reserve content (mg/g) for Chloris virgata, Kochia scoparia, Lespedeza hedysaroides, Astragalus adsurgens, Leonurus artemisia, and Dracocephalum moldavica at various germination stages (relative to imbibed seeds). (1) Imbibition; (2) 1% germination; (3) 50% germination; (4) highest germination; (5) early seedling. The change in seed reserves was calculated from data in Supplementary Table S3.
Discussion
Water absorption during germination is related to the structure of the testa (Debeaujon et al., 2000; Dang et al., 2014) and the chemical composition of seeds (Brancalion et al., 2008). The two legume species A. adsurgens and L. hedysaroides have water-impermeable seed coats (physical dormancy), but the seeds imbibed water. Thus, the water gap (an anatomical structure in a water-impermeable seed coat that opens thereby permitting water entry into the seed, e.g., the lens on a legume seed) must have opened during storage (Baskin, 2003). However, the water gap is small, which would help explain the relatively slow rate of imbibition (Figure 1). Compared with the high-starch seeds (C. virgata) with a relatively low final percentage of water uptake, the high-protein seeds (K. scoparia, L. hedysaroides, and A. adsurgens) had a higher water absorption percentage at the end of imbibition, as protein contains more hydrophilic radicals than starch (Brancalion et al., 2008). Also, seeds with high protein content are known to imbibe more water than fatty seeds (Benech-Arnold and Sanchez, 2004), but it was not always the case in our study. Seeds of L. artemisia (20.2% protein) imbibed a similar amount of water (around 80%) as the two high-protein species K. scoparia (24.2% protein) and L. hedysaroides (23.3% protein), but less water than the seeds of A. adsurgens with 23.8% protein. For fat-storing D. moldavica seeds, water uptake reached 223%. This is likely related to the mucilage of the D. moldavica seed coat (Huang and Gutterman, 2004). Mucilage is a kind of pectinaceous polysaccharide, which can increase water uptake by seeds (Yang et al., 2012).
Our first hypothesis was supported by the result that germination percentages and rates were not significantly correlated with the three seed reserves in dry seeds (P > 0.05). Interspecific studies on the correlation between the three reserves and germination are lacking, limiting the comparison of our results with others, except that Soriano et al. (2011) found a positive relationship between germination rate and seed nitrogen content for 19 tree species from a tropical deciduous forest in Mexico. However, germination rate in our study was positively and significantly related to the soluble sugar and protein content at different germination stages (P < 0.05, Table 2 and Supplementary Table S4). Soluble sugar is the main direct substance required during germination (Jennifer et al., 2002). The soluble sugar content gradually increased during germination of the six study species, which is consistent with the results from most studies (Ahmad et al., 2010; Lopes et al., 2013; Erbaş et al., 2016; Yang et al., 2016). On the other hand, the soluble sugar content was positively correlated with starch content but negatively correlated with fat content in dry seeds (P < 0.05). This means that soluble sugar may have mainly come from, but was not limited to, starch degradation during germination for quick energy for the embryo (Kwangho, 2005; Lopes et al., 2013). Further, the starch content was negatively related to the fat content in dry seeds of the six species (r = -0.71, P = 0.11), which may partially be explained by the negative correlation between fat content and soluble sugar.
Contrary to our second hypothesis, starch was used as the energy source mainly during imbibition for the six species (Figure 4 and Supplementary Table S3, except C. virgata). The percentage of starch reduction during germination varied with species. From the dry seed (stage 0) to highest germination (stage 4), the starch content of the starchy seeds of C. virgata decreased only 10.1%, while that of the fatty seeds of D. moldavica and L. artemisia decreased 73.7 and 89.7%, respectively, and that of the high-protein seeds of L. hedysaroides, K. scoparia, and A. adsurgens decreased 52.9–78.7%. Thus, the fatty seeds may utilize more starch during germination than the starchy seeds. The previous studies have shown similar results. For example, the starch level in the high-starch seeds of Sorghum bicolor was reduced only 13.5% after 1–3 days from sowing (Yang et al., 2016). The total starch of four legumes (Vigna unguiculata, Canavalia ensiformis, Stizolobium niveum, Lablab purpureus) decreased 11.8–35.2% during germination (Benítez et al., 2013). The fatty seeds of Aniba rosaeodora showed a 29.5% reduction in starch level during germination (Lima et al., 2008). The carbohydrates level decreased 75% during germination of Jatropha curcas seeds, which have 40% lipids (Lopes et al., 2013).
Soluble protein was utilized for a large proportion (39.1–93.9%) from imbibition (stage 1) to the highest germination (stage 4) and even the early seedling stage (Figure 5), irrespective of the relative percentage of starch, protein, and fat in dry seeds. Starch is degraded by hydrolysis to sugars (Levin, 1974), and protein is degraded by the proteases and converted to polypeptide and amino acids during germination (Palmiano and Juliano, 1972). These hydrolysis products may meet the energy needs of seeds for germination (Bewley and Black, 1994; Wahid and Bounoua, 2013). The fat content remained relatively constant during germination for the six species. This study considered seed reserve degradation and utilization for the energy needs for germination. However, some components of seed reserves may promote the transition from the quiescent to the active state during germination (Catusse et al., 2008), which is certainly important and not amount dependent.
Compared with the allied crop species, we found a new result that fat in seeds of the six wild species seemed to be less important than in seeds of crop species as energy resources during germination. The fat content remained constant for the six study species (Figure 4). The lipid concentration in seeds of the woody legume Dalbergia nigra remained constant or increased slightly during germination (Ataíde et al., 2013). However, lipid content decreased 34.3 and 36.5% during seed germination of Pisum sativum (El-Adawy et al., 2003) and Sorghum bicolor (Elmaki et al., 1999), respectively. Some crop species had a great decrease of fat content during germination, e.g., 70% decrease for A. sativa and L. angustifolius (Leonova et al., 2010; Rumiyati et al., 2012). Components of fat in seeds of wild species may have other roles such as controlling the developmental switch from dormancy to germination (Footitt et al., 2002), assisting seed defense and dispersal (Finkelstein and Grubb, 2002) and fueling seedling growth after germination (Pinfield-Wells et al., 2005).
To our knowledge, this is the first study to investigate the dynamics of the three main seed reserves during germination of wild grassland species with different relative proportions of starch, protein, and fat in the seeds. We found different patterns of reserve mobilization during seed germination of wild species compared to those in domesticated crop species.
Author Contributions
HZ and HY conceived and designed the work. MZ and LQ performed the experiments. MZ and HZ analyzed the data and wrote the paper. HZ, HY, and CB revised the manuscript.
Funding
This study was funded by the National Natural Science Foundation of China (41571055) and the National Basic Research Program of China (2015CB150800).
Conflict of Interest Statement
The authors declare that the research was conducted in the absence of any commercial or financial relationships that could be construed as a potential conflict of interest.
Acknowledgments
We are thankful for Dr. Baisen Zhang from Australia for the comments on a previous version of the paper.
Supplementary Material
The Supplementary Material for this article can be found online at: https://www.frontiersin.org/articles/10.3389/fpls.2018.00234/full#supplementary-material
References
Ahmad, I. Z., Kamal, A., and Arif, J. M. (2010). Alteration of sugar and protein contents in Nigella sativa L. seeds during different phases of germination. Aspects Appl. Biol. 96, 415–420.
Alencar, N. L., Innecco, R., Gomes-Filho, E., Gallão, M. I., Alvarez-pizarro, J. C., Prisco, J. T., et al. (2012). Seed reserve composition and mobilization during germination and early seedling establishment of Cereus jamacaru D.C. ssp. jamacaru (Cactaceae). An. Acad. Bras. Cienc. 84, 823–832. doi: 10.1590/S0001-37652012000300024
Ataíde, G. D. M., Borges, E. E. D. L., Guimarães, V. M., Flores, A. V., and Bicalho, E. M. (2013). Alterations in seed reserves of Dalbergia nigra ((Vell.) Fr All. Ex Benth.) during hydration. J. Seed Sci. 35, 56–63. doi: 10.1590/S2317-15372013000100008
Baskin, C. C. (2003). Breaking physical dormancy in seeds – focusing on the lens. New Phytol. 158, 229–232. doi: 10.1046/j.1469-8137.2003.00751.x
Baskin, C. C., and Baskin, J. M. (2014). Seeds: Ecology, Biogeography, and Evolution of Dormancy and Germination, 2nd Edn. San Diego, CA: Elsevier.
Bellaloui, N., Smith, J. R., Mengistu, A., Ray, J. D., and Gillen, A. M. (2017). Evaluation of exotically-derived soybean breeding lines for seed yield, germination, damage, and composition under dryland production in the Midsouthern USA. Front. Plant Sci. 8:176. doi: 10.3389/fpls.2017.00176
Benech-Arnold, R. L., and Sanchez, R. A. (2004). “Handbook of seed physiology,” in Applications to Agriculture, eds R. L. Benech-Arnold and R. A. Sanchez (New York, NY: Food Products Press).
Benítez, V., Cantera, S., Aguilera, Y., Mollá, E., Esteban, R. M., Díaz, M. F., et al. (2013). Impact of germination on starch, dietary fiber and physicochemical properties in non-conventional legumes. Food Res. Int. 50, 64–69. doi: 10.1016/j.foodres.2012.09.044
Bewley, J. D., and Black, M. (1994). Seeds: Physiology of Development and Germination. New York, NY: Plenum Press.
Bhatt, A. K., Bhalla, T. C., Agrawal, H. O., and Upadhya, M. D. (1989). Effect of seed size on protein and lipid contents, germination and imbibition in true potato seeds. Potato Res. 32, 477–481. doi: 10.1007/BF02358504
Bourgne, S., Job, C., and Job, D. (2000). Sugarbeet seed priming: solubilization of the basic subunit of 11-S globulin in individual seeds. Seed Sci. Res. 10, 153–161. doi: 10.1017/S0960258500000167
Bradford, M. M. (1976). A rapid and sensitive method for the quantitation of microgram quantities of protein utilizing the principle of protein-dye binding. Anal. Biochem. 72, 248–254. doi: 10.1016/0003-2697(76)90527-3
Brancalion, P. H., Tay, D., Rodrigues, R. R., Novembre, A. D., and Cunha, L. D. (2008). Seed imbibition of five Brazilian native tree seeds. Acta Hortic. 771, 77–81. doi: 10.17660/ActaHortic.2008.771.10
Catusse, J., Rajjou, L., Job, C., and Job, D. (2008). “Proteome of seed development and germination,” in Plant Proteomics: Technologies, Strategies, and Applications, eds G. K. Argrawal and R. Rakwal (Hoboken, NJ: John Wiley & Sons), 191–206.
Dang, T. V., Velusamy, V., and Park, E. (2014). Structure and chemical composition of wild soybean seed coat related to its permeability. Pak. J. Bot. 46, 1847–1857.
Debeaujon, I., Leon-Kloosterziel, K. M., and Koornneef, M. (2000). Influence of the testa on seed dormancy, germination, and longevity in Arabidopsis. Plant Physiol. 122, 403–413. doi: 10.1104/pp.122.2.403
Dong, G., Guo, J., Chen, J., Sun, G., Gao, S., Hu, L. J., et al. (2011). Effects of spring drought on carbon sequestration, evapotranspiration and water use efficiency in the songnen meadow steppe in northeast China. Ecohydrology 4, 211–224. doi: 10.1002/eco.200
Donohue, K. (2009). Completing the cycle: material effects as the missing link in plant life histories. Philos. Trans. R. Soc. B 364, 1059–1074. doi: 10.1098/rstb.2008.0291
Donohue, K., de Casas, R. R., Burghardt, L., Kovach, K., and Willis, C. G. (2010). Germination, postgermination, adaptation, and species ecological ranges. Annu. Rev. Ecol. Evol. Syst. 41, 293–319. doi: 10.1146/annurev-ecolsys-102209-144715
Eastmond, P. J., and Graham, I. A. (2001). Re-examining the role of the glyoxylate cycle in oilseeds. Trends Plant Sci. 6, 72–77. doi: 10.1016/S1360-1385(00)01835-5
El-Adawy, T. A., Rahma, E. H., El-Bedawey, A. A., and El-Beltagy, A. E. (2003). Nutritional potential and functional properties of germinated mung bean, pea and lentil seeds. Plant Food Hum. Nutr. 58, 1–13. doi: 10.1023/B:QUAL.0000040339.48521.75
Elmaki, H. B., Babiker, E. E., and Tinay, A. H. E. (1999). Changes in chemical composition, grain malting, starch and tannin contents and protein digestibility during germination of sorghum cultivars. Food Chem. 64, 331–336. doi: 10.1016/S0308-8146(98)00118-6
Erbaş, S., Tonguç, M., Karakurt, Y., and Şanli, A. (2016). Mobilization of seed reserves during germination and early seedling growth of two sunflower cultivars. J. Appl. Bot. Food Qual. 89, 217–222. doi: 10.5073/JABFQ.2016.089.028
Fernandes, B., Dragone, G., Abreu, A. P., Geada, P., Teixeira, J., and Vicente, A. (2012). Starch determination in Chlorella vulgaris-a comparison between acid and enzymatic methods. J. Appl. Phycol. 24, 1203–1208. doi: 10.1007/s10811-011-9761-5
Finkelstein, S., and Grubb, P. J. (2002). Lipid concentration in the embryo-endosperm fraction of seeds of Australian tropical lowland rainforest trees: relevance to defence and dispersal. Seed Sci. Res. 12, 173–180. doi: 10.1079/ssR2002109
Footitt, S., Slocombe, S. P., Larner, V., Kurup, S., Wu, Y., Larson, T., et al. (2002). Control of germination and lipid mobilization by COMATOSE, the Arabidopsis homologue of human ALDP. EMBO J. 21, 2912–2922. doi: 10.1093/emboj/cdf300
Galland, M., He, D., Lounifi, I., Arc, E., Clément, G., Balzergue, S., et al. (2017). An integrated “multi-omics” comparison of embryo and endosperm tissue-specific features and their impact on rice seed quality. Front. Plant Sci. 8:1984. doi: 10.3389/fpls.2017.01984
Gallardo, K., Job, C., Groot, S. P. C., Puype, M., Demol, H., Vandekerckhove, J., et al. (2001). Proteomic analysis of Arabidopsis seed germination and priming. Plant Physiol. 126, 835–848. doi: 10.1104/pp.126.2.835
Goyoaga, C., Burbano, C., Cuadrado, C., Romero, C., Guillamon, E., Varela, A., et al. (2011). Content and distribution of protein, sugars and inositol phosphates during the germination and seedling growth of two cultivars of Vicia faba. J. Food Comp. Anal. 24, 391–397. doi: 10.1016/j.jfca.2010.11.002
Gu, J., Chao, H., Gan, L., Guo, L., Zhang, K., Li, Y., et al. (2016). Proteomic dissection of seed germination and seedling establishment in Brassica napus. Front. Plant Sci. 7:1482. doi: 10.3389/fpls.2016.01482
Hoffpauir, C. L., Poe, S. E., Wiles, L. U., and Martha, H. (1950). Germination and free fatty acids in seed stock lots of cottonseed. J. Am. Oil Chem. Soc. 27, 347–348. doi: 10.1007/BF02634974
Huang, Z. Y., and Gutterman, Y. (2004). Value of the mucilaginous pellicle to seeds of the sand-stabilizing desert woody shrub Artemisia sphaerocephala (Asteraceae). Trees 18, 669–676. doi: 10.1007/s00468-004-0349-4
Jennifer, P. C. T., Reiter, W. D., and Gibson, S. I. (2002). Mobilization of seed storage lipid by Arabidopsis seedlings is retarded in the presence of exogenous sugars. BMC Plant Biol. 2:4. doi: 10.1186/1471-2229-2-4
Job, C., Kersulec, A., Ravasio, L., Chareyre, S., Pepin, R., and Job, D. (1997). The solubilization of the basic subunit of sugarbeet seed 11-S globulin during priming and early germination. Seed Sci. Res. 7, 225–243. doi: 10.1017/S0960258500003585
Kanmaz, E. Ö., and Ova, G. (2015). The effect of germination time on moisture, total fat content and fatty acid composition of flaxseed (Linum usitatissimum L.) sprouts. Gida 40, 249–254.
Kim, S. T., Wang, Y. M., Kang, S. Y., Kim, S. G., Rakwal, R., Kim, Y. C., et al. (2009). Developing rice embryo proteomics reveals essential role for embryonic proteins in regulation of seed germination. J. Proteome Res. 8, 3598–3605. doi: 10.1021/pr900358s
Kwangho, P. (2005). Variation in sugar and starch content during the germination of rice cultivar suitable for direct sowing. Korean J. Crop Sci. 50, 384–386.
Lai, L. M., Tian, Y., Wang, Y. J., Zhao, X. C., Jiang, L. H., Baskin, J. M., et al. (2015). Distribution of three congeneric shrub species along an aridity gradient is related to seed germination and seedling emergence. AoB Plants 7:plv071. doi: 10.1093/aobpla/plv071
Leonova, S., Grimberg, A., Marttila, S., Stymne, S., and Carlsson, A. S. (2010). Mobilization of lipid reserves during germination of oat (Avena sativa L.), a cereal rich in endosperm oil. J. Exp. Bot. 61, 3089–3099. doi: 10.1093/jxb/erq141
Levin, D. A. (1974). The oil content of seeds: an ecological perspective. Am. Nat. 108, 193–206. doi: 10.1086/282899
Li, R., Chen, L. J., Wu, Y. P., Zhang, R., Baskin, C. C., Baskin, J. M., et al. (2017). Effects of cultivar and maternal environment on seed quality in Vicia sativa. Front. Plant Sci. 8:1411. doi: 10.3389/fpls.2017.01411
Lima, R. B. S., Gonçalves, J. F. C., Pando, S. C., Fernandes, A. V., and Santos, A. L. W. (2008). Primary metabolite mobilization during germination in rosewood (Aniba rosaedora Ducke) seeds. Rev. Arvore 32, 19–25. doi: 10.1590/S0100-67622008000100003
Lopes, L. D. S., Gallão, M. I., and Bertini, C. H. C. M. (2013). Mobilisation of reserves during germination of Jatropha seeds. Rev. Ciênc. Agron. 44, 371–378. doi: 10.1590/S1806-66902013000200021
Nonogaki, H., Bassel, G. W., and Bewley, J. D. (2010). Germination - still a mystery. Plant Sci. 179, 574–581. doi: 10.1016/j.plantsci.2010.02.010
Palmiano, E. P., and Juliano, B. O. (1972). Biochemical changes in the rice grain during germination. Plant Physiol. 49, 751–756. doi: 10.1104/pp.49.5.751
Pinfield-Wells, H., Rylott, E. L., Gilday, A. D., Graham, S., Job, K., Larson, T. R., et al. (2005). Sucrose rescues seedling establishment but not germination of Arabidopsis mutants disrupted in peroxisomal fatty acid catabolism. Plant J. 43, 861–872. doi: 10.1111/j.1365-313X.2005.02498.x
Pritchard, S. L., Charlton, W. L., Baker, A., and Graham, I. A. (2002). Germination and storage reserve mobilization are regulated independently in Arabidopsis. Plant J. 31, 639–647. doi: 10.1046/j.1365-313X.2002.01376.x
Rajjou, L., Duval, M., Galardo, K., Catusse, J., Bally, J., Job, C., et al. (2012). Seed germination and vigor. Annu. Rev. Plant Biol. 63, 507–533. doi: 10.1146/annurev-arplant-042811-105550
Rosental, L., Nonogaki, H., and Fait, A. (2014). Activation and regulation of primary metabolism during seed germination. Seed Sci. Res. 24, 1–15. doi: 10.1017/S0960258513000391
Rumiyati, R., James, A. P., and Jayasena, J. (2012). Effect of germination on the nutritional and protein profile of Australian Sweet Lupin. Food Nutr. Sci. 3, 621–626. doi: 10.4236/fns.2012.35085
Satyanarayana, B., Devi, P. S., and Arundathi, A. (2011). Biochemical changes during seed germination of Sterculia urens Roxb. Not. Sci. Biol. 3, 105–108.
Shaik, S. S., Carciofi, M., Martens, H. J., Hebelstrup, K. H., and Blennow, A. (2014). Starch bioengineering affects cereal grain germination and seedling establishment. J. Exp. Bot. 65, 2257–2270. doi: 10.1093/jxb/eru107
Shrestha, P., Callahan, D. L., Singh, S. P., Petrie, J. R., and Zhou, X. R. (2016). Reduced triacylglycerol mobilization during seed germination and early seedling growth in Arabidopsis containing nutritionally important polyunsaturated fatty acids. Front. Plant Sci. 7:419. doi: 10.3389/fpls.2016.01402
Soriano, D., Huante, P., Gamboa-debuen, A., and Orozco-segovi, A. (2014). Effects of burial and storage on germination and seed reserves of 18 tree species in a tropical deciduous forest in Mexico. Oecologia 174, 33–44. doi: 10.1007/s00442-013-2753-1
Soriano, D., Orozcosegovia, A., Márquezguzmán, J., Kitajima, K., Buen, A. G., and Huante, P. (2011). Seed reserve composition in 19 tree species of a tropical deciduous forest in Mexico and its relationship to seed germination and seedling growth. Ann. Bot. 107, 939–951. doi: 10.1093/aob/mcr041
Sreenivasulu, N., Usadel, B., Winter, A., Radchuk, V., Scholz, U., Stein, N., et al. (2008). Barley grain maturation and germination: metabolic pathway and regulatory network communalities and differences highlighted by new MapMan/PageMan profiling tools. Plant Physiol. 146, 1738–1758. doi: 10.1104/pp.107.111781
Still, D. W., and Bradford, K. J. (1997). Endo-ß-Mannanase activity from individual tomato endosperm caps and radicle tips in relation to germination rates. Plant Physiol. 113, 21–29. doi: 10.1104/pp.113.1.21
Sun, J., Wu, D., and Xu, J. (2015). Characterisation of starch during germination and seedling development of a rice mutant with a high content of resistant starch. J. Cereal Sci. 62, 94–101. doi: 10.1016/j.jcs.2015.01.002
Vandecasteele, C., Teulat-Merah, B., Morère-Le, P. M. C., Leprince, O., Ly, V. B., Viau, L., et al. (2011). Quantitative trait loci analysis reveals a correlation between the ratio of sucrose/raffinose family oligosaccharides and seed vigour in Medicago truncatula. Plant Cell Environ. 34, 1473–1487. doi: 10.1111/j.1365-3040.2011.02346.x
Wahid, N., and Bounoua, L. (2013). The relationship between seed weight, germination and biochemical reserves of maritime pine (Pinus pinaster Ait.) in Morocco. New For. 44, 385–397. doi: 10.1007/s11056-012-9348-2
Wang, T., Sistrunk, L. A., Leskovar, D. I., and Cobb, B. G. (2011). Characteristics of storage reserves of triploid watermelon seeds: association of starch and mean germination time. Seed Sci. Technol. 39, 318–326. doi: 10.15258/sst.2011.39.2.05
Weitbrecht, K., Müller, K., and Leubner-Metzger, G. (2011). First off the mark: early seed germination. J. Exp. Bot. 62, 3289–3309. doi: 10.1093/jxb/err030
Xu, T. M., Tian, B. Q., Sun, Z. D., and Xie, B. J. (2011). Changes of three main nutrient during Oat germination. Nat. Prod. Res. 23, 534–537.
Yang, P., Li, X., Wang, X., Chen, H., Chen, F., and Shen, S. (2007). Proteomic analysis of rice (Oryza sativa) seeds during germination. Proteomics 7, 3358–3368. doi: 10.1002/pmic.200700207
Yang, R., Wang, P., Elbaloula, M. F., and Gu, Z. (2016). Effect of germination on main physiology and biochemistry metabolism of Sorghum seeds. Biosci. J. 32, 378–383. doi: 10.14393/BJ-v32n2a2016-30895
Yang, X., Baskin, J. M., Baskin, C. C., and Huang, Z. (2012). More than just a coating: ecological importance, taxonomic occurrence and phylogenetic relationships of seed coat mucilage. Perspect. Plant Ecol. 14, 434–442. doi: 10.1016/j.ppees.2012.09.002
Keywords: fat, germination, protein, seed reserve mobilization, soluble sugar, starch, wild species
Citation: Zhao M, Zhang H, Yan H, Qiu L and Baskin CC (2018) Mobilization and Role of Starch, Protein, and Fat Reserves during Seed Germination of Six Wild Grassland Species. Front. Plant Sci. 9:234. doi: 10.3389/fpls.2018.00234
Received: 19 December 2017; Accepted: 09 February 2018;
Published: 27 February 2018.
Edited by:
Abraham J. Escobar-Gutiérrez, Institut National de la Recherche Agronomique (INRA), FranceReviewed by:
Dominique Job, Centre National de la Recherche Scientifique (CNRS), FranceVíctor Arturo González-Hernández, Inicio COLPOS, Mexico
Copyright © 2018 Zhao, Zhang, Yan, Qiu and Baskin. This is an open-access article distributed under the terms of the Creative Commons Attribution License (CC BY). The use, distribution or reproduction in other forums is permitted, provided the original author(s) and the copyright owner are credited and that the original publication in this journal is cited, in accordance with accepted academic practice. No use, distribution or reproduction is permitted which does not comply with these terms.
*Correspondence: Hongxiang Zhang, emhhbmdob25neGlhbmdAaWdhLmFjLmNu Hong Yan, eWFuaDYwM0BuZW51LmVkdS5jbg==
†These authors have contributed equally to this work.