- 1Institute of Biology, Dahlem Centre of Plant Sciences, Free University Berlin, Berlin, Germany
- 2Institute of Landscape and Plant Ecology, University of Hohenheim, Stuttgart, Germany
In the spring oilseed rape (OSR) cultivar ‘Mozart’ grown under optimal N supply (NO) or mild N deficiency (NL) the transcriptome changes associated with progressing age until early senescence in developmentally old lower canopy leaves (leaf #4) and younger higher canopy leaves (leaf #8) were investigated. Twelve weeks old NO and NL plants appeared phenotypically and transcriptomically identical, but thereafter distinct nutrition-dependent differences in gene expression patterns in lower and upper canopy leaves emerged. In NO leaves #4 of 14-week-old compared to 13-week-old plants, ∼600 genes were up- or downregulated, whereas in NL leaves #4 ∼3000 genes were up- or downregulated. In contrast, in 15-week-old compared to 13-week-old upper canopy leaves #8 more genes were up- or downregulated in optimally N-supplied plants (∼2000 genes) than in N-depleted plants (∼750 genes). This opposing effect of N depletion on gene regulation was even more prominent among photosynthesis-related genes (PSGs). Between week 13 and 14 in leaves #4, 99 of 110 PSGs were downregulated in NL plants, but none in NO plants. In contrast, from weeks 13 to 16 in leaves #8 of NL plants only 11 PSGs were downregulated in comparison to 66 PSGs in NO plants. Different effects of N depletion in lower versus upper canopy leaves were also apparent in upregulation of autophagy genes and NAC transcription factors. More than half of the regulated NAC and WRKY transcription factor, autophagy and protease genes were specifically regulated in NL leaves #4 or NO leaves #8 and thus may contribute to differences in senescence and nutrient mobilization in these leaves. We suggest that in N-deficient plants the upper leaves retain their N resources longer than in amply fertilized plants and remobilize them only after shedding of the lower leaves.
Introduction
In the past three decades the worldwide oilseed rape acreage has expanded nearly threefold to 36 million ha and the production has increased even fivefold to 73 million tons in 2013 (Food and Agriculture Organization of the United Nations)1. In winter oilseed rape production fertilization with up to 200 kg nitrogen (N) ha-1 year-1 is common practice. Although oilseed rape (OSR) has a high uptake capacity for inorganic N, its nitrogen use efficiency (NUE; for definitions see Masclaux-Daubresse et al., 2010; Xu et al., 2012) is low. Only 50–60% of the applied N is recovered in the plants and at the time of harvest 80% of the total plant N is localized in the seeds (Schjoerring et al., 1995; Jensen et al., 1997; Malagoli et al., 2005a; Rathke et al., 2006). Accordingly, winter OSR production has a high N balance surplus that often exceeds the limit of 60 kg ha-1 year-1 that is effective since 2009 in Germany (Düngeverordnung)2 and the European Union (Nitrates Directive3). To meet these requirements without compromising seed yield, the development of cultivars with improved NUE at reduced fertilizer input is an important agricultural goal in OSR breeding.
Two factors determining the NUE are the N-uptake ability of the plants and the N-remobilization efficiency from old, senescing leaves during pod development and seed ripening. N-uptake increases in young plants approximately until flowering, but stagnates or even decreases during pod ripening and contributes only a minor fraction of the N in the seeds (Schjoerring et al., 1995; Rossato et al., 2001; Malagoli et al., 2005a; Gombert et al., 2010). Indeed, the majority of N required for seed filling and pod ripening is mobilized from senescing leaves and stems (Malagoli et al., 2005a; Gombert et al., 2010). Although the N-efficiency of winter OSR can also be enhanced by breeding cultivars with enhanced N-uptake ability (Schulte auf’m Erley et al., 2007), strengthening the N remobilization activity of leaves during the vegetative phase is a promising approach for improving the NUE of oilseed rape (Gironde et al., 2015). In a simulation model of N partitioning, Malagoli et al. (2005b) came to the conclusion that by optimizing N remobilization from leaves at lower nodes and N retranslocation from vegetative to reproductive tissues, OSR yield could be increased by 15%.
Yet, the shed leaves from lower nodes still have a high N content of up to 3.5% whereas leaves from upper nodes contain at the time of abscission only 1% residual N (Malagoli et al., 2005a). What limits N remobilization from early senescing leaves? Phloem loading of amino acids from degraded leaf proteins appears not to be the limiting step (Tilsner et al., 2005). In many winter OSR cultivars the onset of senescence and abscission of lower node leaves occurs already during the vegetative stages before the development of pods and seeds. This lack of sink organs supposedly leads to a low N remobilization rate from early leaves (Schjoerring et al., 1995; Rossato et al., 2001; Noquet et al., 2004; Malagoli et al., 2005a). Accordingly, winter cultivars with a delayed leaf senescence phenotype (‘functional stay-green’; reviewed in Thomas and Ougham, 2014) tend to have a higher N-efficiency (Schulte auf’m Erley et al., 2007; Gregersen et al., 2013; Koeslin-Findeklee et al., 2015a,b).
The onset of leaf senescence is regulated by multiple, endogenous and environmental factors, among them N deficiency (Gregory, 1937; Mei and Thimann, 1984; Masclaux-Daubresse et al., 2007; Bieker and Zentgraf, 2013; Koeslin-Findeklee et al., 2015b). However, the developmental response to N deficiency and timing of senescence initiation are not uniform throughout the plant body. During development of winter OSR plants senescence progresses sequentially from the bottom toward the top and the sink leaves in young plants later turn into source leaves during pod ripening (reviewed by Avice and Etienne, 2014). N-deprivation triggers earlier onset of senescence in older leaves, whereas in young leaves at higher nodes senescence is delayed (Etienne et al., 2007; Desclos et al., 2008). Thus, the spatially and temporally concerted modulation of senescence initiation is a promising target to improve the N-efficiency of OSR, but it requires a deeper understanding of the metabolic and transcriptional changes associated with leaf senescence initiation and progression in different parts of the plant. Koeslin-Findeklee et al. (2015b) identified in a study of transcriptomic changes following senescence induction by N-depletion in leaves from a lower node of two B. napus winter cultivars differing in their stay-green properties and N-efficiency, a large number of cultivar-specifically regulated, senescence-associated genes, but they did not address leaf-rank specific expression differences.
In this study we report that in the doubled haploid OSR spring cultivar ‘Mozart’ senescence progression and the effect of N-limitation are similar as in winter OSR cultivars and we present a genome-wide developmental transcription analysis of plants grown under standard or reduced N-supply. The developmental transcription changes in lower and upper canopy leaves of plants grown under low N-fertilization indicated that in old (source) leaves senescence was initiated earlier and this onset was accompanied by extensive transcriptional reprogramming. In contrast, in young (sink) leaves at a node below the inflorescence, transcriptional reprogramming was delayed in N-depleted plants. We identified transcription regulator, autophagy and protease genes that were specifically regulated in N-depleted lower canopy leaves or in upper leaves under ample N supply, and genes that were expressed senescence-associated in oilseed rape, but not in Arabidopsis. We hypothesize that some of these genes may have OSR-specific functions in N-remobilization during N-deficiency induced leaf senescence and contribute to differences in senescence execution and nutrient mobilization in upper and lower canopy leaves.
Materials and Methods
Plant Material and Growth Conditions
Oilseed rape spring cultivar Brassica napus cv. ‘Mozart’ plants (BSA Nr. RAS 502, supplied by Norddeutsche Pflanzenzucht Hans-Georg Lembke KG – NPZ, Hohenlieth, Germany) were cultivated in solid medium in growth chambers that simulated the daylight length and average daily temperature profile between 1991 and 2005 in South–West Germany from March 15th (day 0: sowing) onward (Supplementary Table 1). Light intensity (photon flux density) during daylight phases was approximately 1000 μmol m-2 s-1. The average CO2 concentration during illumination was 396 ppm which approximates ambient atmospheric conditions. During the dark phase the CO2 concentration increased by approximately 100 ppm. A more detailed description of nursing, growth and physiological parameters of the plants analyzed in this study are presented in Franzaring et al. (2011). Leaf disks from early developing leaf #4 (at 78, 85, 92, and 99 days after sowing, DAS) and leaf #8 (at 92 and 106 DAS) were collected from plants grown at optimal (NO) or low (NL) N supply. For optimal N nutrition, NH4NO3 was supplied in three equal gifts to each pot at germination (0 DAS; extended BBCH-scale stage GS0; Meier, 2001), 72 DAS (GS35) and 79 DAS (GS59) at an equivalent of 150 kg N ha-1 t. For NL plants fertilizer gifts were reduced by half (75 kg N ha-1 t). For each leaf sample three biological replicates from different plants were collected. Before harvesting, relative chlorophyll levels of the leaves were determined using a Konica Minolta SPAD-502 chlorophyll meter. For each leaf, SPAD values from two positions were measured and averaged.
RNA Isolation
After freezing and grinding the samples in liquid nitrogen, total RNA was isolated by a hot phenol method as described (Drechsler et al., 2015). Total RNA was purified further using the RNeasy Mini Kit (Qiagen, Hilden, Germany). RNA quality was monitored on an Agilent 2100 Bioanalyzer (Agilent Technologies, Santa Clara, CA, United States).
Brassica napus Custom Microarray Design and Functional Annotation
The Brassica napus custom microarray was designed and processed as described in Koeslin-Findeklee et al. (2015b). Briefly, after a probe-preselection strategy established by ImaGenes GmbH (Berlin, Germany; now Source BioScience)4 (Weltmeier et al., 2011) 60,955 probes representing 59,577 targets (EST clusters termed in this paper B. napus ‘unigenes’) were selected for the production of microarrays in the Agilent 8 × 60k format. Microarray design (GPL19044) and expression data (Series entry GSE97653) are deposited in the NCBI Gene Expression Omnibus (GEO) repository. To assign putative functions to the 59,577 B. napus ‘unigenes,’ they were locally BLASTed (RRID:SCR_004870) against the TAIR10 Arabidopsis thaliana cDNA collection (Lamesch et al., 2012) using the BioEdit alignment editor5 (RRID:SCR_007361). Putative functions were attributed to B. napus unigenes based on the annotation of the most homologous Arabidopsis thaliana genes with a BLAST E-value ≤ 10-6. When multiple B. napus unigenes had the same Arabidopsis homolog, the unigene with the lowest E-value that is significantly regulated in any one sample was selected for further analysis.
Processing and Bioinformatic Analysis of Microarray Data
Microarray expression data readouts were generated by the Agilent Feature Extraction software. The raw data files were processed, normalized and analyzed with the Bioconductor package LIMMA6 (RRID:SCR_006442; Smyth, 2004). The read.maimages function was used to load the data into an RGList object. Background subtraction and quantile normalization was performed followed by statistical analysis (moderated t-test). The average of replicated spots was calculated using the avereps function. A design matrix was built for the linear modeling function and the intensity values were applied as lmFit function. Contrast matrices representing comparisons between different harvest time points and N treatments were created and applied to modeled data for computing the statistical significance. Regulated unigenes (≥3-fold expression change and Benjamini-Hochberg-corrected p-value Padj < 0.05) were clustered by their temporal expression profiles with the Short Time-series Expression Miner (STEM) software, RRID:SCR_005016, using default settings (Ernst and Bar-Joseph, 2006). Grouping of unigenes into functional categories was performed with the BAR Classification SuperViewer Tool w/Bootstrap7 (RRID:SCR_006748) using MapMan (RRID:SCR_003543) categories as annotation source (Provart and Zhu, 2003; Provart et al., 2003). Enriched GO-terms were identified with the DAVID Bioinformatics Resources 6.8 in the GOTERM_BP_DIRECT term compilation using default settings8 (RRID:SCR_003033; Huang et al., 2009a,b). Heat maps of differentially regulated genes were created using MultiExperiment Viewer (MeV)9 (RRID:SCR_001915; Saeed et al., 2006). Arabidopsis thaliana transcription factors were compiled from the AGRIS AtTFDB10 (RRID:SCR_006928; Yilmaz et al., 2011), autophagy-related genes from the Autophagy database11 (RRID:SCR_002671; Homma et al., 2011), and peptidases from the MEROPS database12(RRID:SCR_002671; Rawlings et al., 2016).
qPCR Primer Design and Assay for B. napus ‘Unigenes’
Primers for quantitative real-time PCR (qPCR) were calculated by QuantPrime13 (Arvidsson et al., 2008) after importing the B. napus unigene assemblies (Supplementary Table 13). One μg DNase I-digested total RNA was used for cDNA synthesis using SuperScript III Reverse Transcriptase (ThermoFisher Scientific). qPCR reactions were performed in 5 μl total volume including 2.5 μl Power SYBR Green Master Mix (ThermoFisher Scientific), 0.5 μM forward and reverse primers and 0.5 μl cDNA. UP1 and UBC9 were used as reference genes (Chen et al., 2010). The thermal profile used for all qPCRs was: 2 min 50°C > 10 min 95°C > (15 s 95°C > 1 min 60°C)40x. Data were analyzed by the 2-ΔΔCt method (Schmittgen and Livak, 2008).
Results
The Transcriptome Response to Reduced N Supply Differs in Early and Late Oilseed Rape Leaves
The aim of this study was to investigate if in spring oilseed rape (OSR) a mild N deficiency can be detected at the transcriptomic level, if the transcriptome response differs in developmentally older (source) leaves at a lower node and younger (sink) leaves at a higher node, and if the developmental response to N-deficiency resembles that in winter OSR cultivars. Brassica napus cv. ‘Mozart’ plants were raised under controlled conditions in a growth chamber under optimal N supply (NO) or N supply reduced by 50% (NL). Morphological, physiological and performance data of the same plants we investigated in this study were previously reported by Franzaring et al. (2011). The NL conditions caused only subtle developmental and growth phenotypes (Supplementary Figure 1), flowering started on average only 2 days later than in NO plants (Figure 3 in Franzaring et al., 2011), but seed yield was reduced (Table 1 in Franzaring et al., 2011) The early developing leaf #4 and the late developing leaf #8, located at the base of a flower developing side shoot, were harvested as representatives of old (source) leaves and young (sink) leaves, respectively. Leaf #4 was harvested at four different time points during development (78, 85, 92, and 99 days after sowing, DAS). Leaf #8 was harvested at the two time points 92 DAS and 106 DAS. Under both N treatments, at 92 DAS leaves #4 were still alive and attached to the stem, whereas at 106 DAS on most plants they were dead and shed (Figure 1 and Supplementary Figure 1).
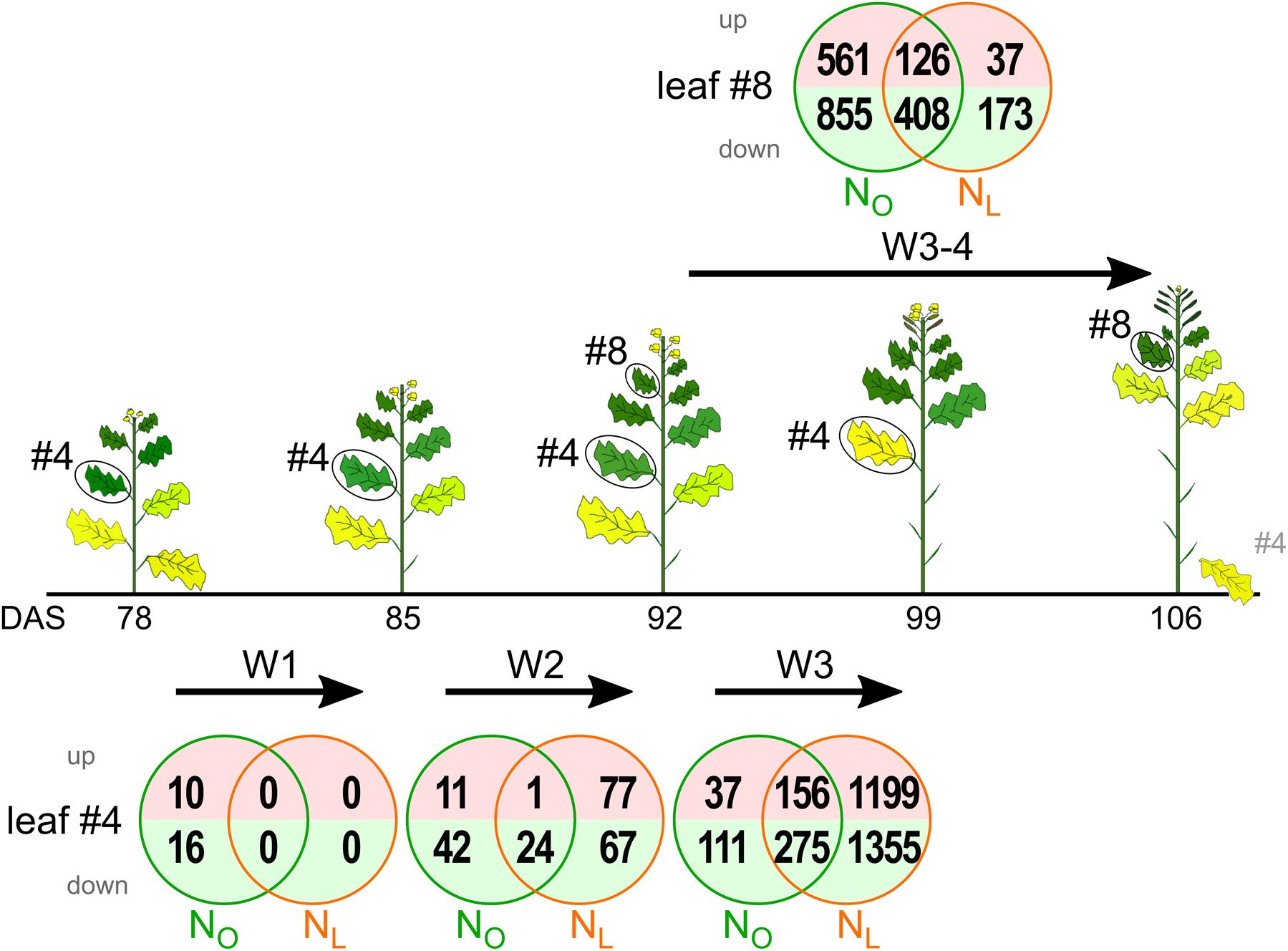
FIGURE 1. Transcriptome changes in oilseed rape leaf 4 (#4) and leaf 8 (#8) during development under optimal N supply (NO) and low N supply (NL). Leaves #4 and #8 were harvested between 78 and 106 days after sowing (DAS) as indicated below the sketches of the oilseed rape plants. The Venn diagrams display the numbers of upregulated and downregulated genes highlighted in light red and lime green, respectively. The examined developmental intervals are termed week 1 (W1), week 2 (W2), week 3 (W3) and weeks 3 + 4 (W3–4). Depicted are genes with significant, ≥3-fold expression changes (Padj < 0.05, n = 3).
Transcription analysis was performed using a B. napus custom microarray representing 59,577 ‘unigenes’ (Koeslin-Findeklee et al., 2015b). For 54,095 (91%) of the B. napus ‘unigenes’ 19,185 homologs were identified in Arabidopsis thaliana (Supplementary Table 2). For only 5,522 of these Arabidopsis genes one single B. napus unigene is represented on the microarray, whereas for 71% more than one B. napus unigene exist (Supplementary Table 3). These unigenes include representatives in each of the 66 biological categories in the MapMan metabolic pathway visualizer (Thimm et al., 2004). In 41 (sub-)categories more than 70% of the corresponding genes are represented by a homologous B. napus unigene (Supplementary Table 4).
Under optimal N supply in leaf #4 the number of B. napus unigenes up- or downregulated relative to the previous harvest time point with significant (Padj < 0.05) and ≥3-fold expression changes progressively increased from week 1 (26 genes) to week 2 (78 genes) to week 3 (579 genes) of the observation period (Figure 1 and Supplementary Table 5). The same trend, but with a much steeper increment, was observed in plants that were grown under reduced N fertilization. In the first week the transcriptome did not change at all, whereas 1 week later 169 regulated genes appeared and in week 3 the number of regulated genes jumped to 2,985. In both growth conditions and all time intervals the downregulated genes outnumbered the upregulated genes.
In the upper canopy leaf #8, 1,950 genes were up- or downregulated in NO plants and 744 in NL plants. Thus, the relation of regulated genes in NO and NL plants was inverse compared to leaf #4. Also in leaf #8 the downregulated genes outnumbered the upregulated genes under both N fertilization regimes.
Reduced N Supply Correlates with Differential Senescence Progression in Lower and Upper Canopy Leaves
The observed massive increase in gene regulation might indicate the onset of leaf senescence, which is known to be accompanied by transcriptome reorganization (Buchanan-Wollaston et al., 2005; van der Graaff et al., 2006; Koeslin-Findeklee et al., 2015b). We therefore tracked senescence initiation by measuring chlorophyll content and expression of chlorophyll A/B binding protein gene BnCAB1, Brassica napus drought 22 kD protein gene BnD22 and the senescence associated genes BnSAG12-1 and BnSAG2 by qPCR.
In leaves #4 of NO plants, BnSAG12-1 (Figure 2A) and BnSAG2 (Figure 2B) expression by trend increased already in week 1 and continued to increase throughout weeks 2 and 3. In NL leaves, upregulation of these two genes started only in week 2. Under both N treatments BnCAB1 transcription appeared to decline in week 1 (Figure 2C), but the expression change was not significant (Supplementary Table 6). BnD22, whose expression level was approximately 3.5-fold higher under low N-conditions at the beginning of the observation period than under optimal N supply (Supplementary Table 5) as has also been reported by Desclos et al. (2008), displayed no significant expression change in weeks 1 and 2 (Supplementary Table 6), but a rapid decline in week 3 (Figure 2D). Expression of both SAGs and CAB, but not BnD22, indicated upcoming senescence one to 2 weeks before also a decline in chlorophyll was measurable (Figure 2E).
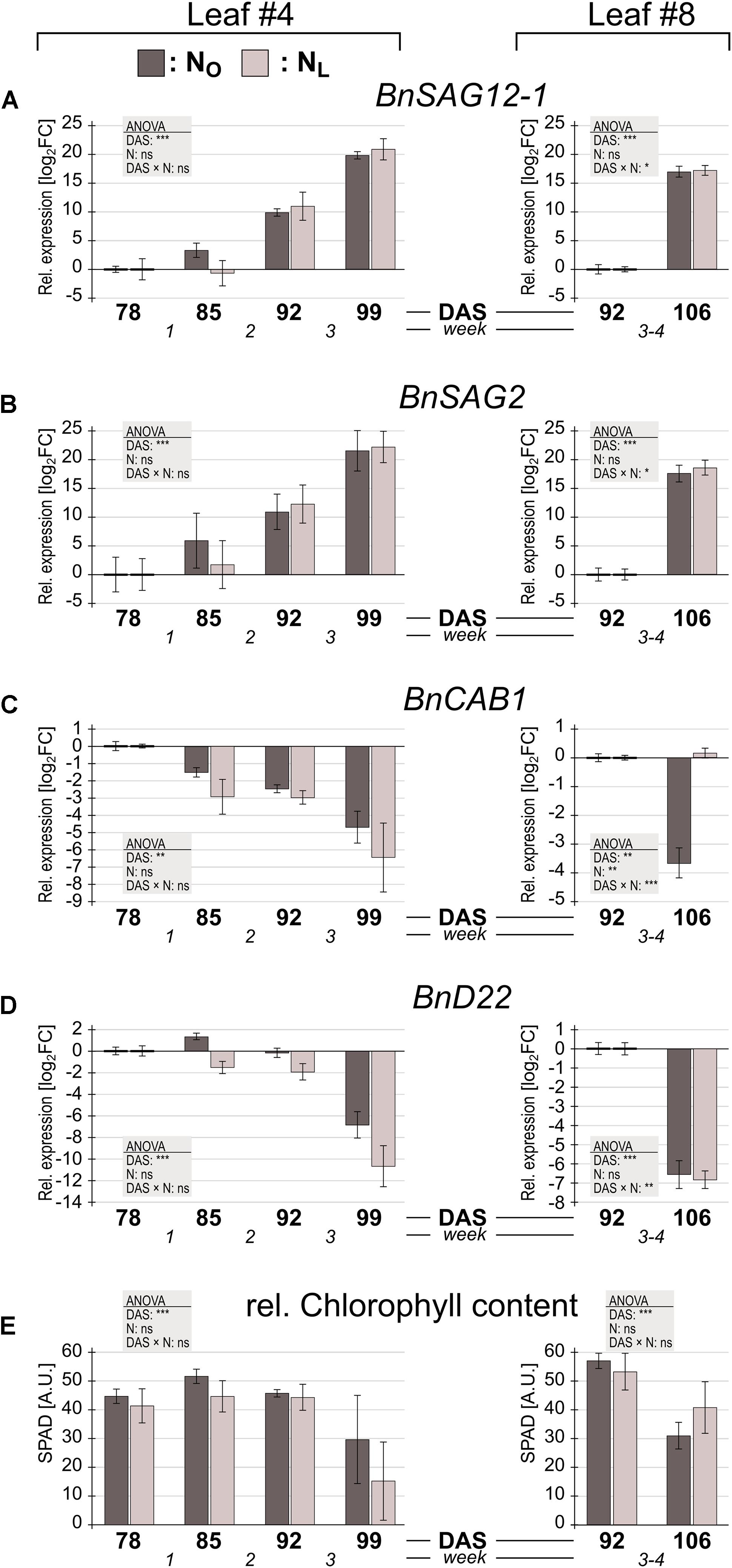
FIGURE 2. Senescence marker gene expression and chlorophyll content in Brassica napus leaves of plants grown under optimal or reduced N supply. B. napus leaves #4 and #8 of plants grown under optimal N supply (NO; dark gray columns) or under reduced N supply (NL; light gray columns) were harvested at the indicated days after sowing (DAS). Relative expression levels were determined by qPCR. For leaf #4, expression changes relative to the level at 78 DAS, and for leaf #8, expression changes relative to the level at 92 DAS are shown of (A) senescence associated gene BnSAG12-1, (B) senescence associated gene BnSAG2, (C) chlorophyll a/b binding protein gene BnCAB1 and (D) B. napus gene BnD22. (E) Relative chlorophyll contents at each harvest time point are shown as SPAD values. Error bars indicate the standard error of the means (n = 3). Significant expression differences by N treatment, over time and by interaction of the two parameters was calculated by two way ANOVA (∗P < 0.05; ∗∗P < 0.01; ∗∗∗P < 0.001). Subsequently a Tukey’s HSD post hoc test was done to identify significant differences between all different harvest time points (Supplementary Table 6).
In leaf #8, the equal upregulation of BnSAG12-1 and BnSAG2 and downregulation of BnD22 in NO and in NL leaves indicates that senescence has started during weeks 3–4. The regulation of BnCAB1 was strikingly different in NO and NL leaves #8. Under low N supply BnCAB1 expression was maintained, whereas under optimal N supply its transcription declined.
Neither in the lower or the upper leaves the differences between NL and NO plants in senescence marker gene expression and chlorophyll content were significant, whereas the expression pattern of BnCAB1 is very different in NL and NO leaves #8. We therefore compared the expression levels of the 110 OSR homologs of A. thaliana photosynthesis-related genes (PSG) on the microarray (Supplementary Table 7). In the optimally N-supplied leaves #4, none of these genes were significantly up- or downregulated between 78 DAS and 99 DAS (except one downregulated gene in week 2). In striking contrast, in leaves #4 grown under reduced N fertilization, 94% of the PSGs were downregulated (4 PSGs in week 2 and another 99 PSGs in week 3). In the upper canopy leaves #8 the pattern was opposite: in NO leaves 66 PSGs were downregulated compared to 11 downregulated PSGs in NL leaves.
In summary, these data and the higher total number of up- and downregulated genes in NL leaves #4 (Figure 1) indicate that a mild N deficiency leads not to a significant earlier initiation, but to a more rapid progression of senescence once it has started. In contrast, in the upper canopy leaf #8 mild N deficiency causes a delay in senescence progression. Thus, in spring OSR the effect of N-deprivation on senescence in older and younger leaves is similar as in winter OSR (Etienne et al., 2007; Desclos et al., 2008).
N Fertilization-Dependent Gene Expression
To identify genes with similar expression change profiles during development under optimal and reduced N supply in the lower canopy leaf #4, the regulated genes were clustered by their expression profiles and assigned to 50 predefined model temporal expression profiles (Supplementary Table 8). Of the 665 genes up- or downregulated in NO leaves #4, four clusters with eight profiles had a statistically significant number of genes assigned (Figure 3A). Overall, 291 of the genes (44%) are allocated to a cluster of four downregulated profiles and 140 genes (21%) fall into a cluster of two upregulated profiles. In leaves #4 of NL plants, 2941 genes (94% of the 3111 regulated genes) are assigned to 15 model temporal expression profiles with a statistically significant number of genes (Figure 3B). Of these, 1174 genes (38%) are allotted to a cluster of four downregulated profiles and 987 genes (32%) to a cluster of three upregulated profiles, respectively. A conspicuous contrast between the transcriptomes of the developmentally younger upper canopy leaves #8 compared to the older leaves #4 is that in leaves #8 a higher number of genes is regulated during the 2 weeks observation interval in plants grown under optimal than under reduced N supply (Figure 1).
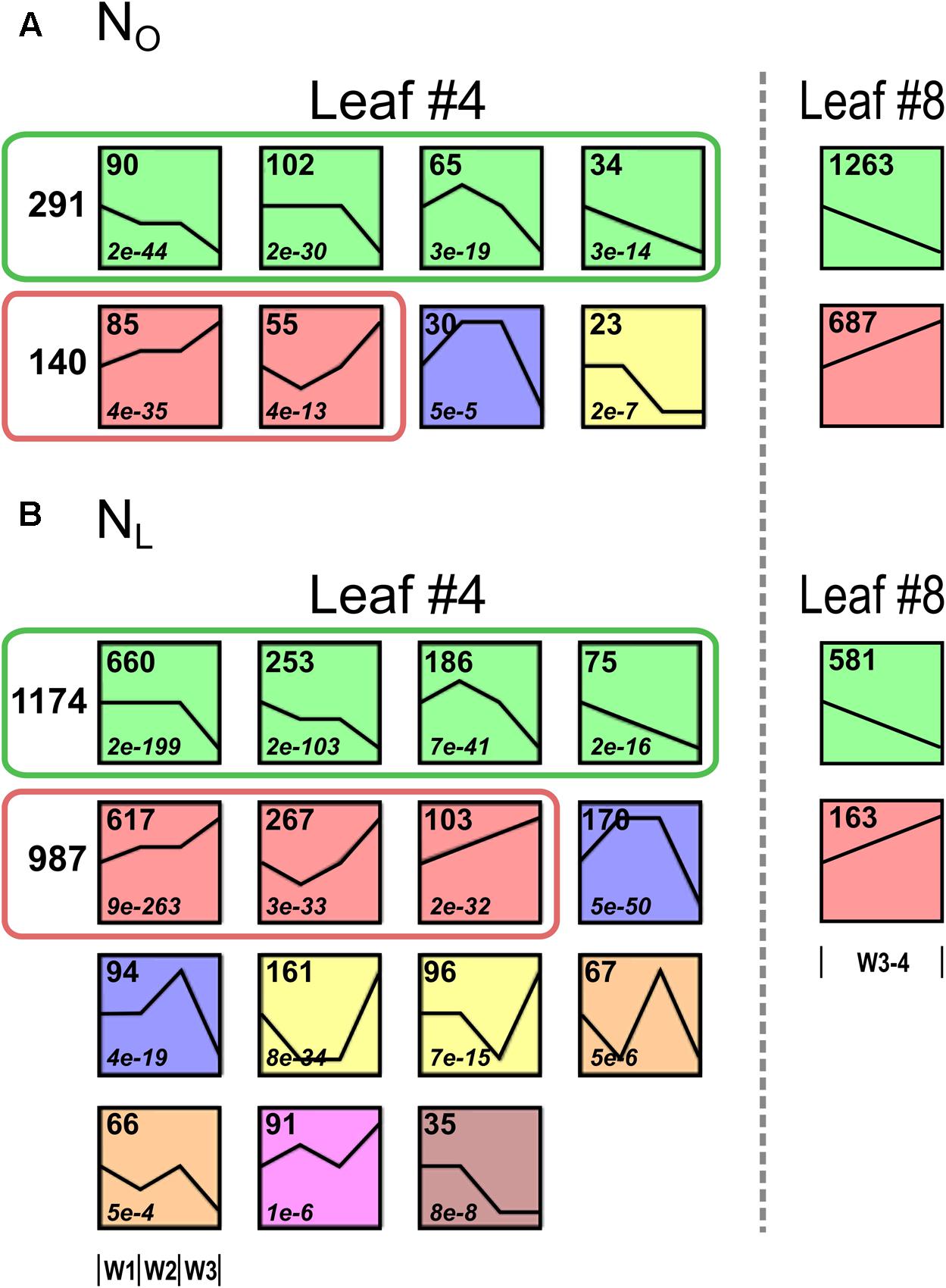
FIGURE 3. Clustering of regulated genes with similar temporal expression profiles in leaf #4. The leaf #4 genes regulated during development under optimal and reduced N supply were clustered according to their temporal expression profiles. The mean expression values (log2) of all differentially regulated genes (≥3-fold expression change in W1, W2, or W3 and Padj < 0.05) were grouped by STEM using default settings. Each box represents one of 50 predefined expression profiles. Depicted are only profiles with a statistically significant number of genes assigned. Profiles with the same color are similar and defined as one cluster. The number of genes belonging to each model expression profile is shown in the top left corner of each box. (A) Of the 655 regulated genes under optimal N supply (NO), 484 genes are allocated to four clusters. Two hundred and ninety one genes are allocated to one ‘downregulated’ cluster that is subdivided in four profiles (green boxes) and 140 genes are allocated to one ‘upregulated’ cluster subdivided in two profiles (red boxes). To the right the numbers of up- and downregulated NO leaf #8 genes are shown. (B) Of the 3111 regulated genes under reduced N supply (NL), 2771 genes are allocated to seven clusters. 1174 genes are allocated to one ‘downregulated’ cluster that is subdivided in four profiles (green boxes) and 987 genes are allocated to one ‘upregulated’ cluster subdivided in three profiles (red boxes). To the right the numbers of up- and downregulated NO leaf #8 genes are shown.
Functional Classification of N Fertilization-Dependently Regulated Genes
To identify the most highly regulated N deficiency-responsive and senescence-associated pathways in leaves #4 and #8, we performed a Gene Ontology term enrichment analysis with the up- and downregulated genes in the two leaf #4 STEM clusters and in leaf #8 (Figure 3). To visualize considerably regulated biological processes in N-deficient leaves #4, which show the most progressed senescence symptoms, all significantly enriched (P < 0.05) GO terms for the up- and downregulated genes are displayed in Figures 4, 5, respectively, and listed in Supplementary Table 9. The most significantly upregulated processes in NL leaves #4 include cell wall weakening, cellular response to N starvation, intracellular bulk degradation of cytoplasmic components like chloroplasts and mitochondria (autophagy, mitophagy) and general leaf senescence activities. NL leaves #4 shared almost 40% of upregulated GO terms with NO leaves #8, but only 19 and 13% with NO leaves #4 and NL leaves #8, respectively. Of the 73 significantly downregulated GO terms in NL leaves #4, approximately one quarter are associated with photosynthesis and related pathways, and another ∼20% encompass biosynthesis of chlorophyll, amino acids, fatty acids, glucose, amylopectin, alkanes, and plastoquinone. In optimally N-supplied leaves #8, 40 of these GO terms (55%) were also downregulated, but only ∼30% in NO leaves #4 and in N-deficient leaves #8. In agreement with photosynthetic gene and senescence marker gene expression levels (Figure 2 and Supplementary Table 7), the GO term analysis suggests that senescence was most advanced in NL leaves #4 at 99 DAS, followed by NO leaves #8 at 106 DAS, NL leaves #8 at 106 DAS and NO leaves #4 at 99 DAS. Accordingly, N-deficiency induced in developmentally old leaves of the spring OSR cultivar ‘Mozart’ the accelerated progression of senescence and remobilization of nutrients, whereas in developmentally younger leaves in the upper canopy it led to a delay in senescence progression.
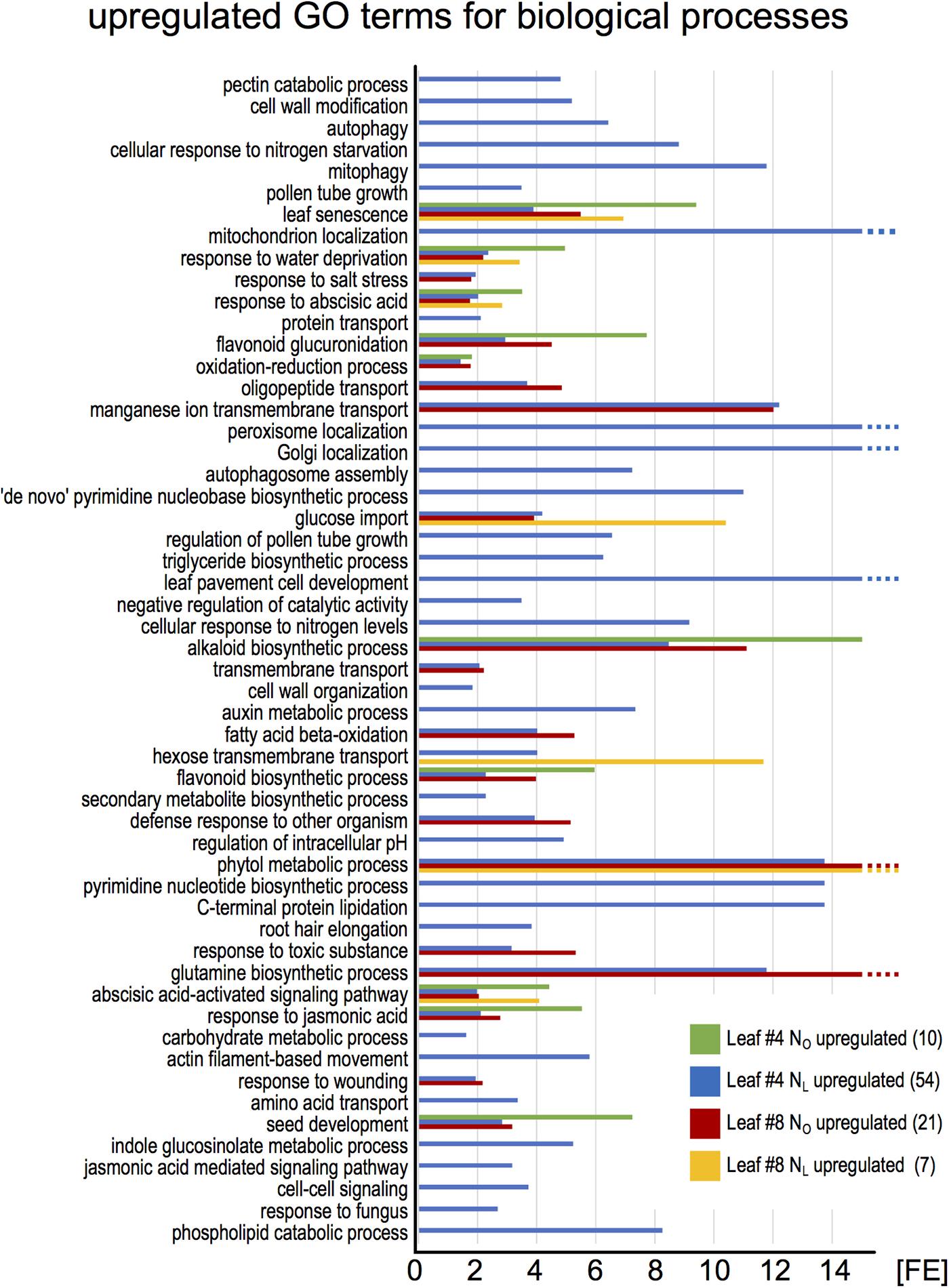
FIGURE 4. Enriched Gene Ontology terms among upregulated leaf #4 genes. The upregulated genes in all samples (red pictograms in Figure 2) were analyzed for enriched Gene Ontology terms using the DAVID Bioinformatics Resources 6.8. The blue bars represent all significantly enriched GO_BP_DIRECT terms in NL leaves #4 (P < 0.05). Those terms that were also enriched in other samples are shown as green, red, and yellow bars. The lack of a bar indicates that the fraction of upregulated genes in a GO term is not significantly higher than the overall fraction of upregulated genes. The numbers in brackets denote the count of enriched GO terms.
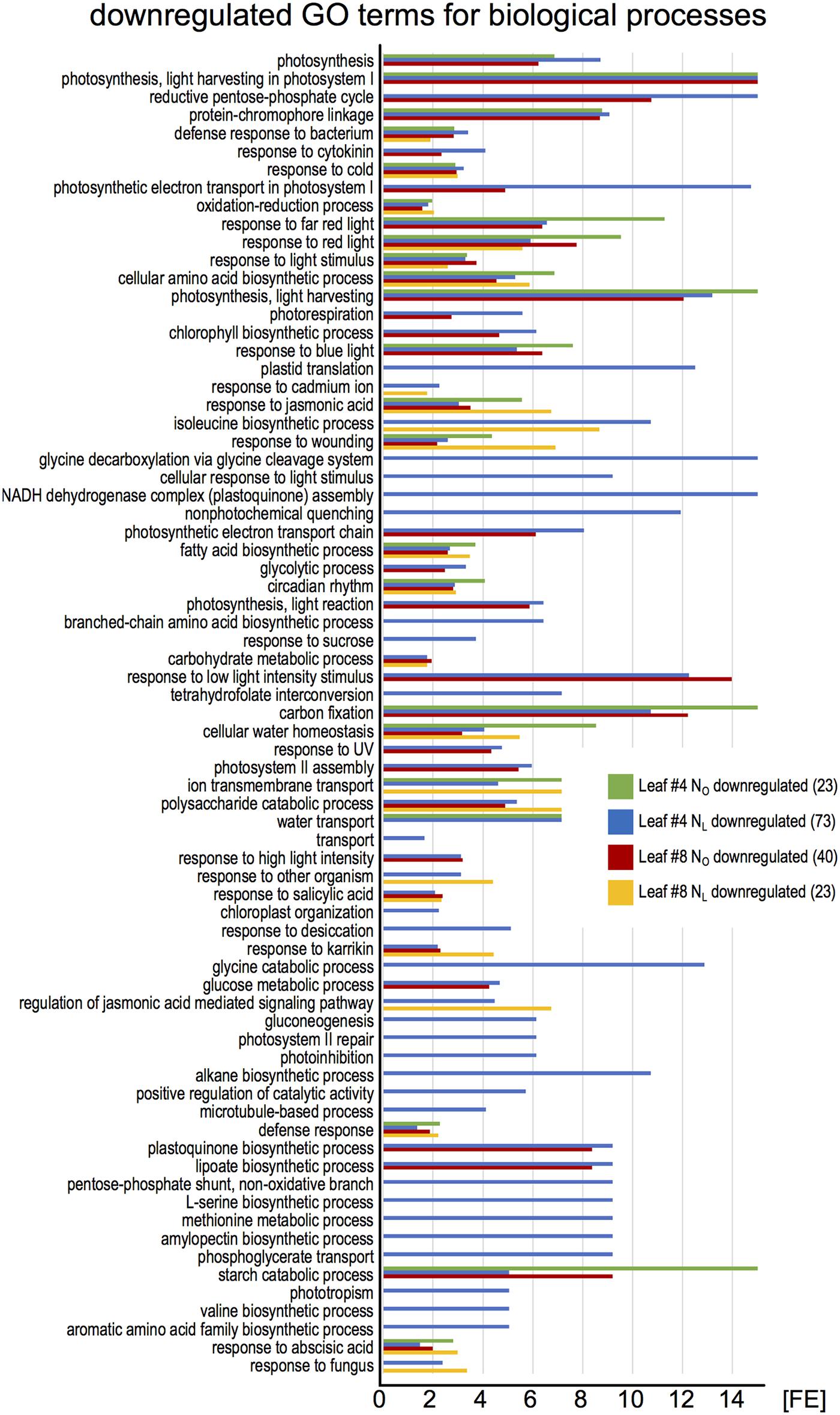
FIGURE 5. Enriched Gene Ontology terms among downregulated leaf #4 genes. The downregulated genes in all samples (green pictograms in Figure 2) were analyzed for enriched Gene Ontology terms using the DAVID Bioinformatics Resources 6.8. The blue bars represent all significantly enriched GO_BP_DIRECT terms in NL leaves #4 (P < 0.05). Those terms that were also enriched in other samples are shown as green, red, and yellow bars. The lack of a bar indicates that the fraction of downregulated genes in a GO term is not significantly higher than the overall fraction of downregulated genes. The numbers in brackets denote the count of enriched GO terms.
In an independent approach to identify up- or downregulated biological pathways, the temporally regulated genes in NO and NL leaves #4 were grouped by their putative biological functions according to the MapMan classification (Thimm et al., 2004), and categories enriched or depleted for regulated genes were identified. Although the total number of regulated genes was five times higher in NL compared to NO plants, the majority of functional gene categories showed no significant differences in the fractions of regulated genes (Supplementary Figure 2). This is consistent with the weak phenotypic differences between NO and NL plants (Supplementary Figure 1). However, major differences are apparent in the categories tetrapyrrole synthesis and photosynthesis, oxidative pentose phosphate pathway (OPP) and C1-metabolism. In leaves #4 of NL plants, half of the genes associated with photosynthesis (97 of 206 genes in this category) and 25% of the genes involved in chlorophyll biosynthesis (12 of 48 genes in the tetrapyrrole category) were downregulated, whereas in NO plants only 5% of the photosynthesis and no chlorophyll biosynthesis genes were downregulated. Thus, the gene expression data reflect the reduced chlorophyll content in NL plants (Figure 2). Also downregulated in NL, but not in NO plants, were the oxidative pentose phosphate pathway, which generates reductants required for various biosynthetic processes, including fatty acid synthesis and inorganic N and S assimilation (Kruger and von Schaewen, 2003; Bussell et al., 2013) and the one-carbon (C1) metabolism pathway. This pathway is also connected to the S-assimilation pathway by supplying C1 units for the synthesis of S-methylmethionine (SMM), which is transported from source leaves via the phloem to sink organs (Hanson and Roje, 2001). The MapMan classification of up- and downregulated leaf #8 genes revealed overall less differences in significantly regulated categories between NO and NL plants compared to the older leaves #4 (Supplementary Figure 3). However, noticeable differences are apparent in the categories tetrapyrrole synthesis and photosynthesis. Opposite to leaves #4, in leaves #8 in both categories a large fraction of the genes was downregulated in NO, but not in NL plants, suggesting that, although the chlorophyll content had not yet much declined between 92 DAS and 106 DAS, senescence was more advanced in leaves #8 of plants grown under optimal N supply than in plants grown under reduced N fertilization.
Senescence- and N Deficiency-Associated Transcription Factor Genes
A major process during leaf senescence is remobilization of N and other nutritional degradation products from source to sink organs. The initiation and progression of senescence is orchestrated by transcription factors (TFs) and thus the identification of senescence-associated TFs that are responsive to N deficiency conditions is crucial for understanding the parameters that determine the N-efficiency of oilseed rape. We therefore investigated the range of B. napus homologs of Arabidopsis TFs that were differentially regulated upon N-deprivation in source leaves #4 and sink leaves #8. In total, 271 regulated OSR homologs of Arabidopsis TFs were found in 37 of the 51 Arabidopsis TF families (Yilmaz et al., 2011), and in most families more genes were down- than upregulated (Supplementary Table 10). Under both N-regimes, almost all TF genes in leaf #4 were regulated exclusively in week 3, only nine genes showed regulation during weeks 1 or 2 (Supplementary Table 11). Analogous to the frequencies of total regulated genes (Figure 1), between 92 and 99 DAS in N-deficient leaves #4, 3.6-fold more putative TF genes were transcriptionally regulated (78 genes up and 104 genes down) than in optimally N-supplied leaves #4 (22 genes up, 29 genes down). In leaf #8, between 92 and 106 DAS threefold more TF genes were regulated in NO (48 genes up, 92 genes down) than in NL plants (15 genes up, 21 genes down). None of the TF genes were oppositely regulated in NO and NL leaves #4 or leaves #8, or in NL leaf #4 and NO leaf #8. Twelve genes were upregulated exclusively in the senescing NL #4 and NO #8 leaves, among them the NAP/NAC029 homolog, and thus are leaf rank-independent senescence-associated TFs. Twenty-four TF genes were downregulated solely in the NL #4 and NO #8 leaves, among them the WRKY53 homolog, suggesting that they are controlling pathways that are downregulated during senescence. The WRKY, Whirly and NAC families deserve special attention, because members of these families were reported to play key roles in controlling leaf senescence and plastid stability in Arabidopsis. In contrast to most other TF families, the NAC genes were predominantly upregulated in senescing leaves #4 and #8 (Figure 6 and Supplementary Table 11). With the only exception of JUB1, in senescing Arabidopsis leaves the corresponding genes are also upregulated (Breeze et al., 2011). Eleven of the 19 regulated OSR NAC genes were specifically regulated in NL leaves #4 or NO leaves #8, which indicates differences in the regulation of downstream processes in the two canopy levels. Other than the NAC factors, most of the regulated WRKY genes were downregulated and it appears that in this TF family more transcriptional reprogramming occured in NO leaves #8 than in NL leaves #4 (Figure 6).
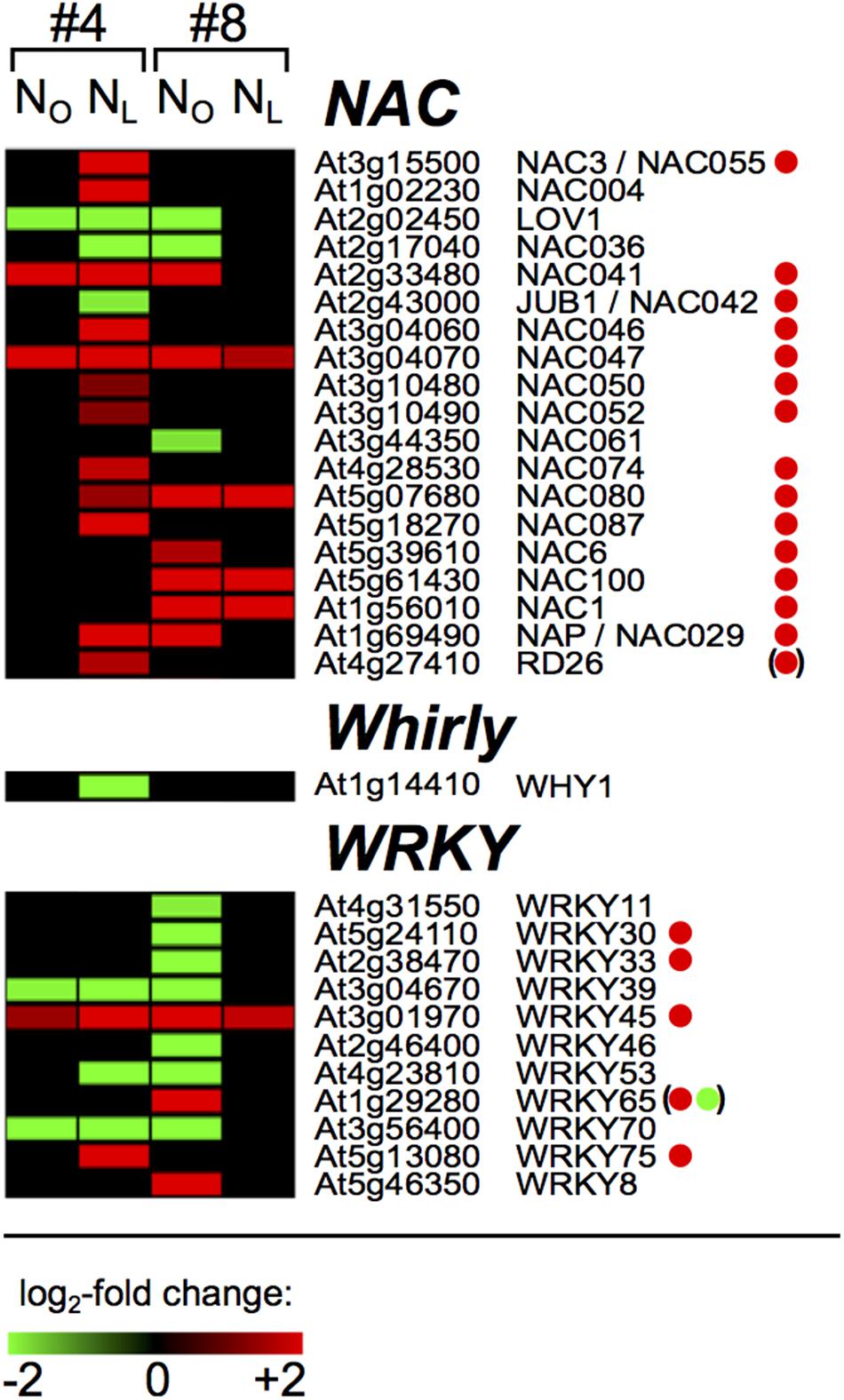
FIGURE 6. Senescence associated oilseed rape NAC, Whirly and WRKY transcription factor genes. The heat maps display the upregulated (red bars) or downregulated (green bars) Brassica napus unigene homologs of Arabidopsis thaliana NAC, Whirly and WRKY family transcription factors in leaf #4 between 92 and 99 DAS and in leaf #8 between 92 and 106 DAS as indicated on top of the columns. Depicted are genes with expression ratios ≥ 3 and Padj < 0.05 (n = 3). Green and red dots denote genes that were reported as leaf senescence-associated down- and upregulated in Arabidopsis thaliana by Breeze et al. (2011). Dots in parentheses indicate that this gene was not steadily regulated and WRKY65 was first up- and later downregulated in the course of leaf senescence.
N-Deficiency Associated Expression of Protein Degradation Genes
The plant-specific developmental process of leaf senescence safeguards the coordinate degradation of proteins, lipids and nucleic acids and remobilization of the resulting low molecular weight nutrients from the senescing leaves to sink organs. Chloroplasts are the most important resource for nitrogen remobilized from senescing source leaves, and autophagy is a crucial process for degradation of chloroplasts during senescence and in response to starvation (Ishida et al., 2014; Michaeli et al., 2014; Izumi et al., 2017). Autophagy mutant plants suffer from premature senescence accompanied by accelerated cell death (reviewed in Minina et al., 2014). Senescence is also accompanied by the activation of various peptidases. To identify the senescence-associated OSR homologs of Arabidopsis autophagy genes in leaves #4 and #8 we matched them against the autophagy database (Homma et al., 2011).
We identified 28 OSR homologs of Arabidopsis autophagy(-related) genes that showed ≥ 3-fold changes in transcription levels (Figure 7A and Supplementary Table 12). In N-deficient leaves #4, 19 autophagy gene homologs were upregulated, among them ten ATG core genes that are essential for autophagosome formation (reviewed in Michaeli et al., 2016; Have et al., 2017). Seven of these autophagy core genes and seven autophagy-related genes are also upregulated in senescing Arabidopsis leaves (van der Graaff et al., 2006; Breeze et al., 2011). Remarkably, although also in NO leaves #4 senescence initiated during week 3 of the observation period, as was indicated by marker gene expression (Figure 2) and enrichment of the GO term ‘leaf senescence’ (Figure 4), except for ATG4a (see below) none of the autophagy core genes were regulated yet in week 3. Also in N-deficient leaves #8, where senescence was delayed, no activation of the autophagy genes was observed. In NO leaves #8, ATG7, ATG8a and six autophagy-related genes were upregulated, however, the intensification of the autophagy pathway was clearly lower than in N-deficient leaves #4. Only two genes were regulated in both leaves #4 and #8 and independent of the N supply. ATG4a, which encodes a cysteine protease involved in the ATG8 ubiquitination-like pathway and is linked to autophagosome formation, was downregulated in all four samples. In Arabidopsis, ATG4a is transcriptionally induced by sudden N-depletion and carbon-starvation (Yoshimoto et al., 2004; Rose et al., 2006), but it has not been reported in the context of senescence yet. PLDP2 was upregulated in all four samples. This gene is also in Arabidopsis upregulated during senescence and was reported to regulate vesicle trafficking and to play a role in Pi-starvation (Breeze et al., 2011). The downregulation of the salicylic-acid responsive PR1 gene (Ward et al., 1991) in all samples except NO leaves #4 is consistent with the corresponding downregulation of the GO term ‘response to salicylic acid’ in these samples.
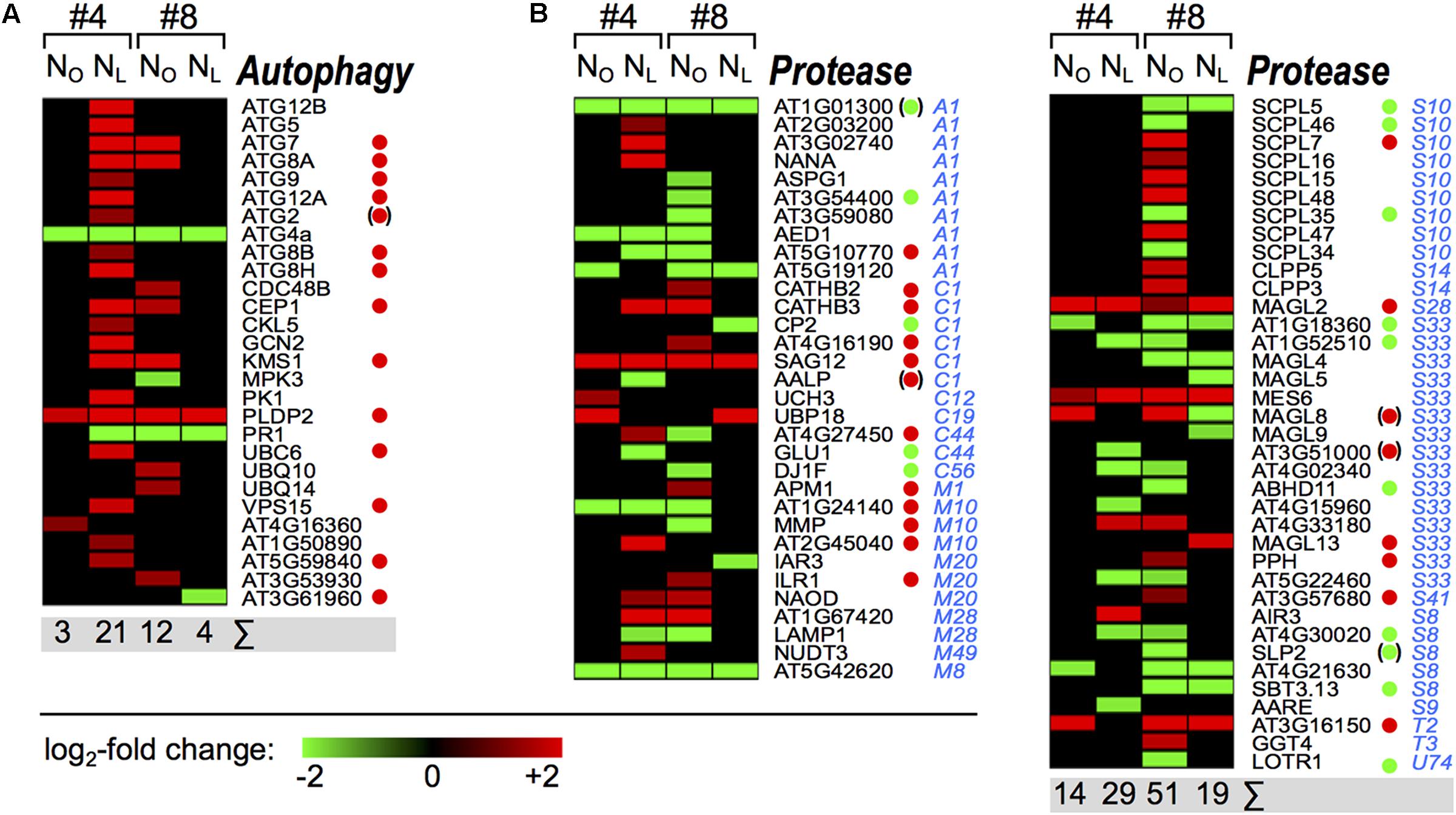
FIGURE 7. Senescence associated oilseed rape autophagy and protease genes. The heat maps display the upregulated (red bars) or downregulated (green bars) Brassica napus unigene homologs of Arabidopsis thaliana (A) autophagy genes and (B) peptidase genes in leaf #4 between 92 and 99 DAS and in leaf #8 between 92 and 106 DAS as indicated on top of the columns. Peptidase family affiliations (MEROPS Peptidase Database) are indicated in blue letters. Depicted are genes with expression ratios ≥ 3 and Padj < 0.05 (n = 3). Green and red dots denote genes that were reported as leaf senescence-associated down- and upregulated in Arabidopsis thaliana by Breeze et al. (2011). Dots in parentheses indicate that this gene was not steadily regulated in the course of leaf senescence.
In addition to autophagy-related proteins, transcriptional and proteomic studies identified in various plant species a large number of senescence-associated, mostly upregulated peptidases from diverse families (reviewed in Roberts et al., 2012). Yet, in winter OSR, only few senescence-associated proteases and protease inhibitors were reported (Etienne et al., 2007; Desclos et al., 2009). We identified in spring OSR ‘Mozart’ overall 69 up- or downregulated OSR homologs of all A. thaliana peptidases listed in the MEROPS peptidase database (Rawlings et al., 2016) (Figure 7B). For 35 of these, Breeze et al. (2011) observed leaf senescence-associated regulation of the corresponding Arabidopsis genes; 30 of them were regulated in the same direction. Other than with the autophagy-related proteins, regulation of protease genes was heterogeneous and more genes were down- than upregulated (37 vs. 32). Remarkably, in contrast to all other gene classes, the highest number of regulated protease genes occured in NO leaves #8. It is tempting to speculate that in spite of the onset of senescence under ample N supply in leaves #8 (Figure 4), dismantling of chloroplasts and degradation of chlorophyll is still pending (Figure 2) and therefore autophagy is not massively upregulated yet (Figure 7A). However, at that stage leaves #8 likely act already as source leaves and provide nutrients for pod development and seed filling (Supplementary Figure 1, Franzaring et al., 2011). The prominent regulation of many proteases in these leaves may be associated with an elevated nutrient export activity.
Discussion
Transcriptome Reprogramming in N Supply-Dependent Senescence of Lower and Upper Node B. napus Leaves
Since the divergence of the ancestral Brassicaceae into the Arabidopsis and Brassica lineages ∼17 million years ago (Cheung et al., 2009), genome triplication, allopolyploidization of the B. napus parental B. rapa and B. oleracea genomes, and gene loss events occurred, with the consequence that the modern OSR genome contains zero to more than six orthologs of any Arabidopsis gene (Rana et al., 2004). This complicates the identification of orthology relationships between Arabidopsis and B. napus genes and prevents the distinction between multiple related B. napus genes when using Arabidopsis microarrays. We therefore used a microarray with 60 nt-probes based predominantly on three EST libraries from B. napus, B. rapa, and B. oleracea and a smaller number of other publically available ESTs (Trick et al., 2009).
In previous studies of the transcriptome response to N starvation in Arabidopsis thaliana (Wang et al., 2003; Scheible et al., 2004; Balazadeh et al., 2014) and winter oilseed rape (Koeslin-Findeklee et al., 2015b), plants were grown hydroponically in low N medium and transcription analysis was performed after nitrate re-addition. This treatment may invoke a rapid and temporal plant response to nutrient shock (Wang et al., 2001; Peng et al., 2007) and thus may not fully reflect the plant adaptive responses to long-term low N conditions. Here, we compared the OSR transcriptome in plants of a spring cultivar grown under optimal or low N fertilization in solid medium under seasonal climate simulating conditions, which is more similar to field conditions (Franzaring et al., 2012).
In the developmentally early winter OSR leaves, senescence typically begins during flowering but before the seed filling stage, and the leaves are shed before the developing reproductive organs have reached their maximal sink strength. This is considered as one reason for the relatively inefficient N remobilization and high residual N content in the fallen leaves that, moreover, increases with N fertilization (Schjoerring et al., 1995; Hocking et al., 1997; Rossato et al., 2001; Noquet et al., 2004; Malagoli et al., 2005a). In cauline leaves in the upper canopy, senescence initiates later and the N content of fallen leaves is lower, indicating a more efficient N remobilization from these leaves driven by the higher sink strength of the developing pods during seed filling (Malagoli et al., 2005a; Etienne et al., 2007). In this study we aimed to determine if the chronology of senescence initiation in different canopy levels is similar in a spring OSR cultivar and how differences between early and late leaves are reflected in their transcriptomes.
Taking the expression changes of the senescence marker genes BnSAG12-1, BnSAG2 and BnCAB1 as indicators, under optimal as well as low N supply the first signs of senescence initiation appeared in the lower canopy leaf #4 already in week 1 of the observation period. In this early senescence phase the chlorophyll content is not a useful indicator for senescence or N deprivation (Figure 2), as had also been observed by Gombert et al. (2006). In the following 2 weeks senescence progressed under both N regimes, but more rapidly in the N deficient plants as is indicated by the massive downregulation of PSGs. In the upper canopy leaf #8 the BnSAG12-1, BnSAG2 and BnD22 expression changes did not show a difference in the senescence status of NO and NL leaves. However, downregulation of BnCAB and many PSGs indicated that N deficiency led to a delay of senescence progression in younger leaves. This conclusion was corroborated by the extent of transcriptome reprogramming and the affected metabolic processes. In leaves #4 essentially no change in gene regulation in either NO or NL leaves was observed in week 1. One week later at an overall low level already twice as many genes were regulated in NL compared to NO leaves, and in week 3 in N-deficient leaves the number of regulated genes increased another 15-times to almost 3,000 regulated genes, whereas under ample N supply less than 600 genes were regulated. The effect of N deprivation was opposite in the upper canopy leaves #8, where 2.5-times more genes were regulated in NO plants. The functional classification of regulated genes revealed that senescence-associated transcriptome reprogramming in spring oilseed rape cv. ‘Mozart’ comprises largely the same biological processes as in Arabidopsis thaliana (Buchanan-Wollaston et al., 2005; van der Graaff et al., 2006; Breeze et al., 2011).
Divergent Regulation of Transcription Factors in Senescing Young and Old Leaves
The age-dependent expression of thousands of senescence-associated genes is orchestrated by transcription factors, many of which are themselves transcriptionally regulated during senescence. Several of these TFs are also induced by various biotic or abiotic stresses, indicating that senescence is an integrated response of plants to endogenous developmental signals and environmental cues (Woo et al., 2013). In Arabidopsis, transcriptomic analyses revealed the enrichment of upregulated TF genes of the NAC, WRKY, AP2/EREBP, MYB, C2H2 zinc-finger, bZIP, and GRAS families during leaf senescence (Buchanan-Wollaston et al., 2005; van der Graaff et al., 2006; Balazadeh et al., 2008, 2010; Breeze et al., 2011). We observed also in spring OSR a senescence-associated transcriptional reorganization in all these TF families. The comparison of the expression changes of the 112 senescence-associated TF genes that were identified in Arabidopsis by Breeze et al. (2011) and here in OSR reveals largely congruent transcription increases or decreases (Supplementary Table 11). Interestingly though, we note in OSR that 107 TF genes were only in NL leaf #4 and 65 genes only in NO leaves #8 more than threefold up- or downregulated, which indicates distinct regulation of individual senescence processes and nutrient remobilization in upper and lower canopy leaves. Also noticeable is a virtually perfect congruence of TF gene regulation in certain families between OSR and Arabidopsis (NAC, C2C2, C2H2) and more divergent regulation in others (AP2-EREBP, C3H, homeobox).
The expression profiles of well characterized key regulators of senescence in OSR and Arabidopsis attest that, in spite of their very different sporophyte architectures, the regulatory network controlling senescence is similar in these two Brassicaceae. For example, in both species the positive regulators of chlorophyll degradation NAC046 and NAC055 and the senescence promoting NAP/NAC029 factor are upregulated (Figure 6) (Guo and Gan, 2006; Hickman et al., 2013; Oda-Yamamizo et al., 2016). On the other hand, the negative regulator of senescence WRKY70 (Ülker et al., 2007; Zentgraf et al., 2010; Besseau et al., 2012) and the early induced WRKY53 factor, which interacts with other senescence regulators (Hinderhofer and Zentgraf, 2001; Miao et al., 2004; Zentgraf et al., 2010), were downregulated. However, in a few cases also divergent regulation of senescence-controlling factors in Arabidopsis and OSR was observed. The NAC family member JUB1, which was identified as a longevity-promoting factor in Arabidopsis (Wu et al., 2012), was downregulated in NL leaves #4. Surprisingly, it was found to be induced in Arabidopsis during leaf senescence (Breeze et al., 2011). Noteworthy is also the downregulation of the OSR homolog of WHY1 in NL leaves #4 (Figure 6), because this gene is involved in maintaining chloroplast stability. The Arabidopsis WHY1 gene, which is one of only three Whirly family genes in this plant, is required for chloroplast genome stability (Marechal et al., 2009), and the barley WHIRLY1 ortholog is involved in premature senescence induction under photooxidative stress (Kucharewicz et al., 2017).
Chloroplast Decomposition and Protein Degradation Pathway Activation in Senescing Leaves
The critical role of autophagy for the disassembly of chloroplasts, mitochondria and other cellular structures in the course of senescence has been extensively demonstrated in Arabidopsis (reviewed by Michaeli et al., 2016; Have et al., 2017). During developmental and starvation-induced senescence, entire chloroplasts can be degraded by autophagy (Minamikawa et al., 2001; Wada et al., 2009). Other than in A. thaliana, where 9 of 15 upregulated autophagy genes were activated in leaves that were not even fully expanded yet and showed no signs of senescence (Breeze et al., 2011), in OSR we do not observe activation of autophagy genes in NO leaves #4 or NL leaves #8, while senescence was initiated in these leaves. However, in the more advanced senescence stages in NL #4 and NO #8 leaves, more OSR autophagy genes appear to be upregulated than in Arabidopsis (Breeze et al., 2011), although we did not consider all statistically significantly regulated genes but only those exhibiting ≥ 3-fold transcriptional changes. A possible explanation could be that autophagy is a generic, auto-cleaning process required to remove obsolete cell components and maintain cellular integrity. It is thus constitutively active at a low level which might be sufficient during early senescence. Only when senescence progresses it may become necessary to boost the autophagy pathway.
The more pronounced transcriptional activation of autophagy genes in N-deficient leaves #4 compared to NO leaves #8 could indicate that the cell death program, the last phase of senescence, has started in the lower canopy leaves, whereas the young upper canopy leaves #8 have to stay alive to serve as source leaves for nutrient remobilization toward the developing pods. This course of events is known from winter OSR genotypes (reviewed in Avice and Etienne, 2014). Consistent with this hypothesis is the much higher number of regulated protease genes in NO leaves #8 which may be involved in protein turnover and N remobilization, but not in executing cell death.
Differences between OSR and Arabidopsis are also apparent in the regulation of senescence-associated peptidase genes, which play a crucial role in providing nitrogen transport molecules like amino acids for developing sink organs (reviewed by Masclaux-Daubresse et al., 2010). Similar to the group of autophagy genes, in OSR more peptidase genes are differentially regulated than in Arabidopsis (Breeze et al., 2011), and a larger fraction of these genes is downregulated. These differences might indicate a partly different orchestration of the senescence course in OSR, which may reflect the more complex architecture and morphological development of OSR plants compared to A. thaliana. Recently, by protease activity profiling Poret et al. (2016) identified in senescing B. napus leaves after 23 days of N-starvation an activity increase relative to plants grown with ample N-supply of 17 serine- and cysteine-proteases with homology to 10 Arabidopsis proteases including SAG12, AALP, and AARE. In our study, both AALP and AARE are downregulated only in N-deficient leaves #4 (Figure 7B). However, transcription data do not always reflect protein level or activity data, as has also been reported for metabolic flux data (Schwender et al., 2014), and especially proteases are frequently regulated at the post-transcriptional level.
Conclusion
We found evidence that the sequence of senescence initiation and progression and also the effects of N-limitation are similar in the spring OSR cultivar ‘Mozart’ and in winter OSR cultivars. Like in winter OSR, long-term, mild N deficiency leads in spring OSR to premature shutdown of PSGs and senescence in lower canopy source leaves, whereas in upper canopy sink leaves senescence progression is delayed. The onset of senescence is accompanied by a massive reprogramming of the transcriptome. The affected regulatory and metabolic pathways are overall similar to those in Arabidopsis, but we identified transcription regulator and protein degradation genes that are specifically regulated in N-depleted lower canopy leaves or in upper leaves under ample N supply, and genes that are senescence-associatedly expressed in oilseed rape, but not in Arabidopsis. In future studies it will be interesting to address the question whether these genes fulfill specific tasks in N-remobilization during N deficiency-induced leaf senescence and if their regulation affects the nitrogen use efficiency of oilseed rape.
Author Contributions
VS-R: acquisition, analysis, and interpretation of data; writing the manuscript. JF: acquisition of data and design of the work. AF: design of the work. RK: conception and design of the work; acquisition, analysis, and interpretation of data; writing the manuscript.
Funding
This research was supported by the Deutsche Forschungsgemein schaft (Forschergruppe FOR 948 grant no. KU715/10–2 to RK).
Conflict of Interest Statement
The authors declare that the research was conducted in the absence of any commercial or financial relationships that could be construed as a potential conflict of interest.
Acknowledgments
The authors thank Samuel Arvidsson for help with QuantPrime, Stefan Bieker, and Ulrike Zentgraf for help with plant harvesting, and Christine Rausch for instructing VS-R during the early phase of the project.
Supplementary Material
The Supplementary Material for this article can be found online at: https://www.frontiersin.org/articles/10.3389/fpls.2018.00048/full#supplementary-material
Footnotes
- ^http://faostat3.fao.org
- ^https://www.gesetze-im-internet.de/d_v_2017/
- ^http://ec.europa.eu/environment/water/water-nitrates/index_en.html
- ^www.sourcebioscience.com
- ^www.mbio.ncsu.edu/BioEdit/bioedit.html
- ^www.bioconductor.org/packages/release/bioc/html/limma.html
- ^http://bar.utoronto.ca/ntools/cgi-bin/ntools_classification_superviewer.cgi
- ^https://david.ncifcrf.gov
- ^www.tm4.org/
- ^http://arabidopsis.med.ohio-state.edu
- ^www.tanpaku.org/autophagy/index.html
- ^http://merops.sanger.ac.uk
- ^http://quantprime.mpimp-golm.mpg.de
References
Arvidsson, S., Kwasniewski, M., Riano-Pachon, D. M., and Mueller-Roeber, B. (2008). QuantPrime–a flexible tool for reliable high-throughput primer design for quantitative PCR. BMC Bioinformatics 9:465. doi: 10.1186/1471-2105-9-465
Avice, J. C., and Etienne, P. (2014). Leaf senescence and nitrogen remobilization efficiency in oilseed rape (Brassica napus L.). J. Exp. Bot. 65, 3813–3824. doi: 10.1093/jxb/eru177
Balazadeh, S., Riano-Pachon, D. M., and Mueller-Roeber, B. (2008). Transcription factors regulating leaf senescence in Arabidopsis thaliana. Plant Biol. (Stuttg) 10(Suppl. 1), 63–75. doi: 10.1111/j.1438-8677.2008.00088.x
Balazadeh, S., Schildhauer, J., Araujo, W. L., Munne-Bosch, S., Fernie, A. R., Proost, S., et al. (2014). Reversal of senescence by N resupply to N-starved Arabidopsis thaliana: transcriptomic and metabolomic consequences. J. Exp. Bot. 65, 3975–3992. doi: 10.1093/jxb/eru119
Balazadeh, S., Siddiqui, H., Allu, A. D., Matallana-Ramirez, L. P., Caldana, C., Mehrnia, M., et al. (2010). A gene regulatory network controlled by the NAC transcription factor ANAC092/AtNAC2/ORE1 during salt-promoted senescence. Plant J. 62, 250–264. doi: 10.1111/j.1365-313X.2010.04151.x
Besseau, S., Li, J., and Palva, E. T. (2012). WRKY54 and WRKY70 co-operate as negative regulators of leaf senescence in Arabidopsis thaliana. J. Exp. Bot. 63, 2667–2679. doi: 10.1093/jxb/err450
Bieker, S., and Zentgraf, U. (2013). “Plant senescence and nitrogen mobilization and signaling,” in Senescence and Senescence-Related Disorders, eds Z. Wang and I. Hiroyuki (Rijeka: InTech), 53–83. doi: 10.5772/54392
Breeze, E., Harrison, E., McHattie, S., Hughes, L., Hickman, R., Hill, C., et al. (2011). High-resolution temporal profiling of transcripts during Arabidopsis leaf senescence reveals a distinct chronology of processes and regulation. Plant Cell 23, 873–894. doi: 10.1105/tpc.111.083345
Buchanan-Wollaston, V., Page, T., Harrison, E., Breeze, E., Lim, P. O., Nam, H. G., et al. (2005). Comparative transcriptome analysis reveals significant differences in gene expression and signalling pathways between developmental and dark/starvation-induced senescence in Arabidopsis. Plant J. 42, 567–585. doi: 10.1111/j.1365-313X.2005.02399.x
Bussell, J. D., Keech, O., Fenske, R., and Smith, S. M. (2013). Requirement for the plastidial oxidative pentose phosphate pathway for nitrate assimilation in Arabidopsis. Plant J. 75, 578–591. doi: 10.1111/tpj.12222
Chen, X., Truksa, M., Shah, S., and Weselake, R. J. (2010). A survey of quantitative real-time polymerase chain reaction internal reference genes for expression studies in Brassica napus. Anal. Biochem. 405, 138–140. doi: 10.1016/j.ab.2010.05.032
Cheung, F., Trick, M., Drou, N., Lim, Y. P., Park, J. Y., Kwon, S. J., et al. (2009). Comparative analysis between homoeologous genome segments of Brassica napus and its progenitor species reveals extensive sequence-level divergence. Plant Cell 21, 1912–1928. doi: 10.1105/tpc.108.060376
Desclos, M., Dubousset, L., Etienne, P., Le Caherec, F., Satoh, H., Bonnefoy, J., et al. (2008). A proteomic profiling approach to reveal a novel role of Brassica napus drought 22 kD/water-soluble chlorophyll-binding protein in young leaves during nitrogen remobilization induced by stressful conditions. Plant Physiol. 147, 1830–1844. doi: 10.1104/pp.108.116905
Desclos, M., Etienne, P., Coquet, L., Jouenne, T., Bonnefoy, J., Segura, R., et al. (2009). A combined N-15 tracing/proteomics study in Brassica napus reveals the chronology of proteomics events associated with N remobilisation during leaf senescence induced by nitrate limitation or starvation. Proteomics 9, 3580–3608. doi: 10.1002/pmic.200800984
Drechsler, N., Zheng, Y., Bohner, A., Nobmann, B., von Wirén, N., Kunze, R., et al. (2015). Nitrate-dependent control of shoot K homeostasis by the nitrate transporter1/peptide transporter family member NPF7.3/NRT1.5 and the Stelar K+ Outward Rectifier SKOR in Arabidopsis. Plant Physiol. 169, 2832–2847. doi: 10.1104/pp.15.01152
Ernst, J., and Bar-Joseph, Z. (2006). STEM: a tool for the analysis of short time series gene expression data. BMC Bioinformatics 7:191. doi: 10.1186/1471-2105-7-191
Etienne, P., Desclos, M., Le Goua, L., Gombert, J., Bonnefoy, J., Maurel, K., et al. (2007). N-protein mobilisation associated with the leaf senescence process in oilseed rape is concomitant with the disappearance of trypsin inhibitor activity. Funct. Plant Biol. 34, 895–906. doi: 10.1071/Fp07088
Franzaring, J., Gensheimer, G., Weller, S., Schmid, I., and Fangmeier, A. (2012). Allocation and remobilisation of nitrogen in spring oilseed rape (Brassica napus L. cv. Mozart) as affected by N supply and elevated CO2. Environ. Exp. Bot. 83, 12–22. doi: 10.1016/j.envexpbot.2012.03.015
Franzaring, J., Weller, S., Schmid, I., and Fangmeier, A. (2011). Growth, senescence and water use efficiency of spring oilseed rape (Brassica napus L. cv. Mozart) grown in a factorial combination of nitrogen supply and elevated CO2. Environ. Exp. Bot. 72, 284–296. doi: 10.1016/j.envexpbot.2011.04.003
Gironde, A., Poret, M., Etienne, P., Trouverie, J., Bouchereau, A., Le Caherec, F., et al. (2015). A profiling approach of the natural variability of foliar N remobilization at the rosette stage gives clues to understand the limiting processes involved in the low N use efficiency of winter oilseed rape. J. Exp. Bot. 66, 2461–2473. doi: 10.1093/jxb/erv031
Gombert, J., Etienne, P., Ourry, A., and Le Dily, F. (2006). The expression patterns of SAG12/Cab genes reveal the spatial and temporal progression of leaf senescence in Brassica napus L. with sensitivity to the environment. J. Exp. Bot. 57, 1949–1956. doi: 10.1093/jxb/erj142
Gombert, J., Le Dily, F., Lothier, J., Etienne, P., Rossato, L., Allirand, J. M., et al. (2010). Effect of nitrogen fertilization on nitrogen dynamics in oilseed rape using N-15-labeling field experiment. J. Plant Nutr. Soil Sci. 173, 875–884. doi: 10.1002/jpln.200800270
Gregersen, P. L., Culetic, A., Boschian, L., and Krupinska, K. (2013). Plant senescence and crop productivity. Plant Mol. Biol. 82, 603–622. doi: 10.1007/s11103-013-0013-8
Gregory, F. G. (1937). Mineral nutrition of plants. Annu. Rev. Biochem. 6, 557–578. doi: 10.1146/annurev.bi.06.070137.003013
Guo, Y., and Gan, S. (2006). AtNAP, a NAC family transcription factor, has an important role in leaf senescence. Plant J. 46, 601–612. doi: 10.1111/j.1365-313X.2006.02723.x
Hanson, A. D., and Roje, S. (2001). One-carbon metabolism in higher plants. Annu. Rev. Plant Physiol. Plant Mol. Biol. 52, 119–137. doi: 10.1146/annurev.arplant.52.1.119
Have, M., Marmagne, A., Chardon, F., and Masclaux-Daubresse, C. (2017). Nitrogen remobilization during leaf senescence: lessons from Arabidopsis to crops. J. Exp. Bot. 68, 2513–2529. doi: 10.1093/jxb/erw365
Hickman, R., Hill, C., Penfold, C. A., Breeze, E., Bowden, L., Moore, J. D., et al. (2013). A local regulatory network around three NAC transcription factors in stress responses and senescence in Arabidopsis leaves. Plant J. 75, 26–39. doi: 10.1111/tpj.12194
Hinderhofer, K., and Zentgraf, U. (2001). Identification of a transcription factor specifically expressed at the onset of leaf senescence. Planta 213, 469–473. doi: 10.1007/s004250000512
Hocking, P. J., Randall, P. J., and DeMarco, D. (1997). The response of dryland canola to nitrogen fertilizer: partitioning and mobilization of dry matter and nitrogen, and nitrogen effects on yield components. Field Crops Res. 54, k201–220. doi: 10.1016/S0378-4290(97)00049-X
Homma, K., Suzuki, K., and Sugawara, H. (2011). The autophagy database: an all-inclusive information resource on autophagy that provides nourishment for research. Nucleic Acids Res. 39, D986–D990. doi: 10.1093/nar/gkq995
Huang, D. W., Sherman, B. T., and Lempicki, R. A. (2009a). Bioinformatics enrichment tools: paths toward the comprehensive functional analysis of large gene lists. Nucleic Acids Res. 37, 1–13. doi: 10.1093/nar/gkn923
Huang, D. W., Sherman, B. T., and Lempicki, R. A. (2009b). Systematic and integrative analysis of large gene lists using DAVID bioinformatics resources. Nat. Protoc. 4, 44–57. doi: 10.1038/nprot.2008.211
Ishida, H., Izumi, M., Wada, S., and Makino, A. (2014). Roles of autophagy in chloroplast recycling. Biochim. Biophys. Acta 1837, 512–521. doi: 10.1016/j.bbabio.2013.11.009
Izumi, M., Ishida, H., Nakamura, S., and Hidema, J. (2017). Entire photodamaged chloroplasts are transported to the central vacuole by autophagy. Plant Cell 29, 377–394. doi: 10.1105/tpc.16.00637
Jensen, L. S., Christensen, L., Mueller, T., and Nielsen, N. E. (1997). Turnover of residual N-15-labelled fertilizer N in soil following harvest of oilseed rape (Brassica napus L). Plant Soil 190, 193–202. doi: 10.1023/A:1004253611044
Koeslin-Findeklee, F., Becker, M. A., van der Graaff, E., Roitsch, T., and Horst, W. J. (2015a). Differences between winter oilseed rape (Brassica napus L.) cultivars in nitrogen starvation-induced leaf senescence are governed by leaf-inherent rather than root-derived signals. J. Exp. Bot. 66, 3669–3681. doi: 10.1093/jxb/erv170
Koeslin-Findeklee, F., Rizi, V. S., Becker, M. A., Parra-Londono, S., Arif, M., Balazadeh, S., et al. (2015b). Transcriptomic analysis of nitrogen starvation- and cultivar-specific leaf senescence in winter oilseed rape (Brassica napus L.). Plant Sci. 233, 174–185. doi: 10.1016/j.plantsci.2014.11.018
Kruger, N. J., and von Schaewen, A. (2003). The oxidative pentose phosphate pathway: structure and organisation. Curr. Opin. Plant Biol. 6, 236–246. doi: 10.1016/S1369-5266(03)00039-6
Kucharewicz, W., Distelfeld, A., Bilger, W., Muller, M., Munne-Bosch, S., Hensel, G., et al. (2017). Acceleration of leaf senescence is slowed down in transgenic barley plants deficient in the DNA/RNA-binding protein WHIRLY1. J. Exp. Bot. 68, 983–996. doi: 10.1093/jxb/erw501
Lamesch, P., Berardini, T. Z., Li, D., Swarbreck, D., Wilks, C., Sasidharan, R., et al. (2012). The Arabidopsis Information Resource (TAIR): improved gene annotation and new tools. Nucleic Acids Res. 40, D1202–D1210. doi: 10.1093/nar/gkr1090
Malagoli, P., Laine, P., Rossato, L., and Ourry, A. (2005a). Dynamics of nitrogen uptake and mobilization in field-grown winter oilseed rape (Brassica napus) from stem extension to harvest: I. Global N flows between vegetative and reproductive tissues in relation to leaf fall and their residual N. Ann. Bot. 95, 853–861. doi: 10.1093/aob/mci091
Malagoli, P., Laine, P., Rossato, L., and Ourry, A. (2005b). Dynamics of nitrogen uptake and mobilization in field-grown winter oilseed rape (Brassica napus) from stem extension to harvest. II. An 15N-labelling-based simulation model of N partitioning between vegetative and reproductive tissues. Ann. Bot. 95, 1187–1198. doi: 10.1093/aob/mci131
Marechal, A., Parent, J. S., Veronneau-Lafortune, F., Joyeux, A., Lang, B. F., and Brisson, N. (2009). Whirly proteins maintain plastid genome stability in Arabidopsis. Proc. Natl. Acad. Sci. U.S.A. 106, 14693–14698. doi: 10.1073/pnas.0901710106
Masclaux-Daubresse, C., Daniel-Vedele, F., Dechorgnat, J., Chardon, F., Gaufichon, L., and Suzuki, A. (2010). Nitrogen uptake, assimilation and remobilization in plants: challenges for sustainable and productive agriculture. Ann. Bot. 105, 1141–1157. doi: 10.1093/aob/mcq028
Masclaux-Daubresse, C., Purdy, S., Lemaitre, T., Pourtau, N., Taconnat, L., Renou, J. P., et al. (2007). Genetic variation suggests interaction between cold acclimation and metabolic regulation of leaf senescence. Plant Physiol. 143, 434–446. doi: 10.1104/pp.106.091355
Mei, H. S., and Thimann, K. V. (1984). The relation between nitrogen deficiency and leaf senescence. Physiol. Plant. 62, 157–161. doi: 10.1111/j.1399-3054.1984.tb00364.x
Meier, U. (ed.) (2001). BBCH Monograph - Growth Stages of Mono-and Dicotyledonous Plants. Bonn: Federal Biological Research Centre for Agriculture and Forestry.
Miao, Y., Laun, T., Zimmermann, P., and Zentgraf, U. (2004). Targets of the WRKY53 transcription factor and its role during leaf senescence in Arabidopsis. Plant Mol. Biol. 55, 853–867. doi: 10.1007/s11103-004-2142-6
Michaeli, S., Galili, G., Genschik, P., Fernie, A. R., and Avin-Wittenberg, T. (2016). Autophagy in plants–what’s new on the menu? Trends Plant Sci. 21, 134–144. doi: 10.1016/j.tplants.2015.10.008
Michaeli, S., Honig, A., Levanony, H., Peled-Zehavi, H., and Galili, G. (2014). Arabidopsis ATG8-INTERACTING PROTEIN1 is involved in autophagy-dependent vesicular trafficking of plastid proteins to the vacuole. Plant Cell 26, 4084–4101. doi: 10.1105/tpc.114.129999
Minamikawa, T., Toyooka, K., Okamoto, T., Hara-Nishimura, I., and Nishimura, M. (2001). Degradation of ribulose-bisphosphate carboxylase by vacuolar enzymes of senescing French bean leaves: immunocytochemical and ultrastructural observations. Protoplasma 218, 144–153. doi: 10.1007/BF01306604
Minina, E. A., Bozhkov, P. V., and Hofius, D. (2014). Autophagy as initiator or executioner of cell death. Trends Plant Sci. 19, 692–697. doi: 10.1016/j.tplants.2014.07.007
Noquet, C., Avice, J. C., Rossato, L., Beauclair, P., Henry, M. P., and Ourry, A. (2004). Effects of altered source-sink relationships on N allocation and vegetative storage protein accumulation in Brassica napus L. Plant Sci. 166, 1007–1018. doi: 10.1016/j.plantsci.2003.12.014
Oda-Yamamizo, C., Mitsuda, N., Sakamoto, S., Ogawa, D., Ohme-Takagi, M., and Ohmiya, A. (2016). The NAC transcription factor ANAC046 is a positive regulator of chlorophyll degradation and senescence in Arabidopsis leaves. Sci. Rep. 6:23609. doi: 10.1038/srep23609
Peng, M., Bi, Y. M., Zhu, T., and Rothstein, S. J. (2007). Genome-wide analysis of Arabidopsis responsive transcriptome to nitrogen limitation and its regulation by the ubiquitin ligase gene NLA. Plant Mol. Biol. 65, 775–797. doi: 10.1007/s11103-007-9241-0
Poret, M., Chandrasekar, B., van der Hoorn, R. A., and Avice, J. C. (2016). Characterization of senescence-associated protease activities involved in the efficient protein remobilization during leaf senescence of winter oilseed rape. Plant Sci. 246, 139–153. doi: 10.1016/j.plantsci.2016.02.011
Provart, N., and Zhu, T. (2003). A browser-based functional classification superviewer for Arabidopsis genomics. Curr. Comput. Mol. Biol. 2003, 271–272.
Provart, N. J., Gil, P., Chen, W., Han, B., Chang, H. S., Wang, X., et al. (2003). Gene expression phenotypes of Arabidopsis associated with sensitivity to low temperatures. Plant Physiol. 132, 893–906. doi: 10.1104/pp.103.021261
Rana, D., van den Boogaart, T., O’Neill, C. M., Hynes, L., Bent, E., Macpherson, L., et al. (2004). Conservation of the microstructure of genome segments in Brassica napus and its diploid relatives. Plant J. 40, 725–733. doi: 10.1111/j.1365-313X.2004.02244.x
Rathke, G. W., Behrens, T., and Diepenbrock, W. (2006). Integrated nitrogen management strategies to improve seed yield, oil content and nitrogen efficiency of winter oilseed rape (Brassica napus L.): a review. Agric. Ecosyst. Environ. 117, 80–108. doi: 10.1016/j.agee.2006.04.006
Rawlings, N. D., Barrett, A. J., and Finn, R. (2016). Twenty years of the MEROPS database of proteolytic enzymes, their substrates and inhibitors. Nucleic Acids Res. 44, D343–D350. doi: 10.1093/nar/gkv1118
Roberts, I. N., Caputo, C., Criado, M. V., and Funk, C. (2012). Senescence-associated proteases in plants. Physiol. Plant. 145, 130–139. doi: 10.1111/j.1399-3054.2012.01574.x
Rose, T. L., Bonneau, L., Der, C., Marty-Mazars, D., and Marty, F. (2006). Starvation-induced expression of autophagy-related genes in Arabidopsis. Biol. Cell 98, 53–67. doi: 10.1042/BC20040516
Rossato, L., Laine, P., and Ourry, A. (2001). Nitrogen storage and remobilization in Brassica napus L. during the growth cycle: nitrogen fluxes within the plant and changes in soluble protein patterns. J. Exp. Bot. 52, 1655–1663. doi: 10.1093/jexbot/52.361.1655
Saeed, A. I., Bhagabati, N. K., Braisted, J. C., Liang, W., Sharov, V., Howe, E. A., et al. (2006). TM4 microarray software suite. Methods Enzymol. 411, 134–193. doi: 10.1016/S0076-6879(06)11009-5
Scheible, W. R., Morcuende, R., Czechowski, T., Fritz, C., Osuna, D., Palacios-Rojas, N., et al. (2004). Genome-wide reprogramming of primary and secondary metabolism, protein synthesis, cellular growth processes, and the regulatory infrastructure of Arabidopsis in response to nitrogen. Plant Physiol. 136, k2483–2499. doi: 10.1104/pp.104.047019
Schjoerring, J. K., Bock, J. G. H., Gammelvind, L., Jensen, C. R., and Mogensen, V. O. (1995). Nitrogen incorporation and remobilization in different shoot components of field-grown winter oilseed rape (Brassica napus L) as affected by rate of nitrogen application and irrigation. Plant Soil 177, 255–264. doi: 10.1007/BF00010132
Schmittgen, T. D., and Livak, K. J. (2008). Analyzing real-time PCR data by the comparative C(T) method. Nat. Protoc. 3, 1101–1108. doi: 10.1038/nprot.b2008.73
Schulte auf’m Erley, G., Wijaya, K.-A., Ulas, A., Becker, H., Wiesler, F., and Horst, W. J. (2007). Leaf senescence and N uptake parameters as selection traits for nitrogen efficiency of oilseed rape cultivars. Physiol. Plant. 130, 519–531. doi: 10.1111/j.1399-3054.2007.00921.x
Schwender, J., Konig, C., Klapperstuck, M., Heinzel, N., Munz, E., Hebbelmann, I., et al. (2014). Transcript abundance on its own cannot be used to infer fluxes in central metabolism. Front. Plant. Sci. 5:668. doi: 10.3389/fpls.2014.00668
Smyth, G. K. (2004). Linear models and empirical bayes methods for assessing differential expression in microarray experiments. Stat. Appl. Genet. Mol. Biol. 3, 1–25. doi: 10.2202/1544-6115.1027
Thimm, O., Blasing, O., Gibon, Y., Nagel, A., Meyer, S., Kruger, P., et al. (2004). MAPMAN: a user-driven tool to display genomics data sets onto diagrams of metabolic pathways and other biological processes. Plant J. 37, 914–939. doi: 10.1111/j.1365-313X.2004.02016.x
Thomas, H., and Ougham, H. (2014). The stay-green trait. J. Exp. Bot. 65, k3889–3900. doi: 10.1093/jxb/eru037
Tilsner, J., Kassner, N., Struck, C., and Lohaus, G. (2005). Amino acid contents and transport in oilseed rape (Brassica napus L.) under different nitrogen conditions. Planta 221, 328–338. doi: 10.1007/s00425-004-1446-8
Trick, M., Cheung, F., Drou, N., Fraser, F., Lobenhofer, E. K., Hurban, P., et al. (2009). A newly-developed community microarray resource for transcriptome profiling in Brassica species enables the confirmation of Brassica-specific expressed sequences. BMC Plant Biol. 9:50. doi: 10.1186/1471-2229-9-50
Ülker, B., Shahid Mukhtar, M., and Somssich, I. E. (2007). The WRKY70 transcription factor of Arabidopsis influences both the plant senescence and defense signaling pathways. Planta 226, 125–137. doi: 10.1007/s00425-006-0474-y
van der Graaff, E., Schwacke, R., Schneider, A., Desimone, M., Flügge, U. I., and Kunze, R. (2006). Transcription analysis of Arabidopsis membrane transporters and hormone pathways during developmental and induced leaf senescence. Plant Physiol. 141, 776–792. doi: 10.1104/pp.106.079293
Wada, S., Ishida, H., Izumi, M., Yoshimoto, K., Ohsumi, Y., Mae, T., et al. (2009). Autophagy plays a role in chloroplast degradation during senescence in individually darkened leaves. Plant Physiol. 149, 885–893. doi: 10.1104/pp.108.130013
Wang, R. C., Okamoto, M., Xing, X. J., and Crawford, N. M. (2003). Microarray analysis of the nitrate response in Arabidopsis roots and shoots reveals over 1,000 rapidly responding genes and new linkages to glucose, trehalose-6-phosphate, iron, and sulfate metabolism. Plant Physiol. 132, 556–567. doi: 10.1104/pp.103.021253
Wang, Y. H., Garvin, D. F., and Kochian, L. V. (2001). Nitrate-induced genes in tomato roots. Array analysis reveals novel genes that may play a role in nitrogen nutrition. Plant Physiol. 127, 345–359. doi: 10.1104/pp.127.1.345
Ward, E. R., Uknes, S. J., Williams, S. C., Dincher, S. S., Wiederhold, D. L., Alexander, D. C., et al. (1991). Coordinate gene activity in response to agents that induce systemic acquired resistance. Plant Cell 3, 1085–1094. doi: 10.1105/tpc.3.10.1085
Weltmeier, F., Maser, A., Menze, A., Hennig, S., Schad, M., Breuer, F., et al. (2011). Transcript profiles in sugar beet genotypes uncover timing and strength of defense reactions to Cercospora beticola Infection. Mol. Plant Microbe Interact. 24, 758–772. doi: 10.1094/mpmi-08-10-0189
Woo, H. R., Kim, H. J., Nam, H. G., and Lim, P. O. (2013). Plant leaf senescence and death - regulation by multiple layers of control and implications for aging in general. J. Cell Sci. 126(Pt 21), 4823–4833. doi: 10.1242/jcs.109116
Wu, A., Allu, A. D., Garapati, P., Siddiqui, H., Dortay, H., Zanor, M. I., et al. (2012). JUNGBRUNNEN1, a reactive oxygen species-responsive NAC transcription factor, regulates longevity in Arabidopsis. Plant Cell 24, 482–506. doi: 10.1105/tpc.111.090894
Xu, G., Fan, X., and Miller, A. J. (2012). Plant nitrogen assimilation and use efficiency. Annu. Rev. Plant. Biol. 63, 153–182. doi: 10.1146/annurev-arplant-042811-105532
Yilmaz, A., Mejia-Guerra, M. K., Kurz, K., Liang, X., Welch, L., and Grotewold, E. (2011). AGRIS: the Arabidopsis gene regulatory information server, an update. Nucleic Acids Res. 39, D1118–D1122. doi: 10.1093/nar/gkq1120
Yoshimoto, K., Hanaoka, H., Sato, S., Kato, T., Tabata, S., Noda, T., et al. (2004). Processing of ATG8s, ubiquitin-like proteins, and their deconjugation by ATG4s are essential for plant autophagy. Plant Cell 16, 2967–2983. doi: 10.1105/tpc.104.025395
Keywords: autophagy, Brassica napus, leaf senescence, N remobilization, N-deficiency, oilseed rape, transcriptome, transcription factor
Citation: Safavi-Rizi V, Franzaring J, Fangmeier A and Kunze R (2018) Divergent N Deficiency-Dependent Senescence and Transcriptome Response in Developmentally Old and Young Brassica napus Leaves. Front. Plant Sci. 9:48. doi: 10.3389/fpls.2018.00048
Received: 02 October 2017; Accepted: 10 January 2018;
Published: 01 February 2018.
Edited by:
Dragana Miladinović, Institute of Field and Vegetable Crops, SerbiaReviewed by:
Frédéric Marsolais, Agriculture and Agri-Food Canada (AAFC), CanadaAstrid Wingler, University College Cork, Ireland
Copyright © 2018 Safavi-Rizi, Franzaring, Fangmeier and Kunze. This is an open-access article distributed under the terms of the Creative Commons Attribution License (CC BY). The use, distribution or reproduction in other forums is permitted, provided the original author(s) and the copyright owner are credited and that the original publication in this journal is cited, in accordance with accepted academic practice. No use, distribution or reproduction is permitted which does not comply with these terms.
*Correspondence: Reinhard Kunze, cmVpbmhhcmQua3VuemVAZnUtYmVybGluLmRl