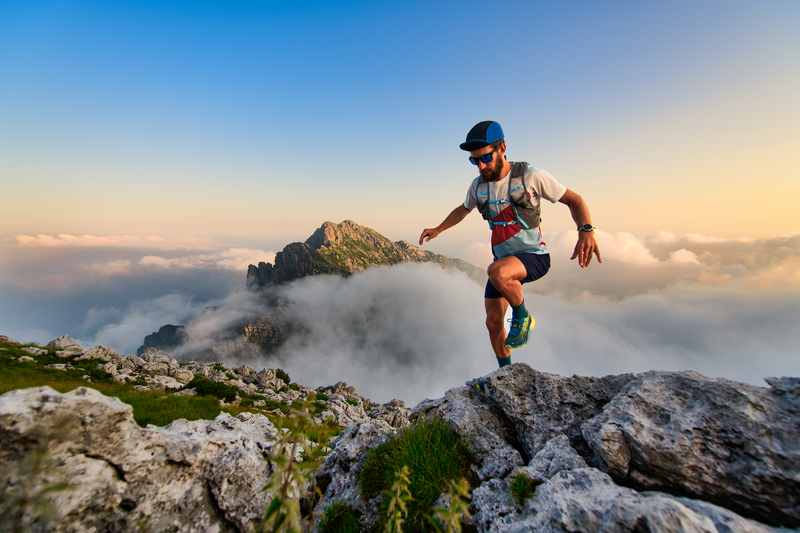
95% of researchers rate our articles as excellent or good
Learn more about the work of our research integrity team to safeguard the quality of each article we publish.
Find out more
ORIGINAL RESEARCH article
Front. Plant Sci. , 20 November 2017
Sec. Plant Biotechnology
Volume 8 - 2017 | https://doi.org/10.3389/fpls.2017.01997
This article is part of the Research Topic Novel Insights into Algal Biology and Biotechnology View all 13 articles
Concentrating algal cells by flocculation as a prelude to centrifugation could significantly reduce the energy and cost of harvesting the algae. However, how variation in phenotypic traits such as cell surface features, cell size and motility alter the efficiency of metal cation and pH-induced flocculation is not well understood. Our results demonstrate that both wild-type and cell wall-deficient strains of the green unicellular alga Chlamydomonas reinhardtii efficiently flocculate (>90%) at an elevated pH of the medium (pH 11) upon the addition of divalent cations such as calcium and magnesium (>5 mM). The trivalent ferric cation (at 10 mM) proved to be essential for promoting flocculation under weak alkaline conditions (pH ∼8.5), with a maximum efficiency that exceeded 95 and 85% for wild-type CC1690 and the cell wall-deficient sta6 mutant, respectively. Near complete flocculation could be achieved using a combination of 5 mM calcium and a pH >11, while the medium recovered following cell removal could be re-cycled without affecting algal growth rates. Moreover, the absence of starch in the cell had little overall impact on flocculation efficiency. These findings contribute to our understanding of flocculation in different Chlamydomonas strains and have implications with respect to inexpensive methods for harvesting algae with different phenotypic traits. Additional research on the conditions (e.g., pH and metal ions) used for efficient flocculation of diverse algal groups with diverse characteristics, at both small and large scale, will help establish inexpensive procedures for harvesting cell biomass.
Over the past decade there has been an increasing interest in microalgae as a promising feedstock for sustainable, large-scale production of commodities such as food, feed, chemicals, materials and fuels (Abomohra et al., 2016; Waghmare et al., 2016). For all of the products that could potentially be developed, overcoming technical issues and reducing production costs will be the primary determinants of feasibility for achieving sustainable algal biomass production (Laurens et al., 2017). Among key technical issues, a major challenge for commercial-scale applications is the ability to inexpensively harvest large quantities of microalgal biomass from dilute cultures. Conventional methods like centrifugation are fast and effective, but also costly and energy intensive, making it only suitable for the production of high-value biomass or metabolites (Demirbas, 2017). The various other techniques that have been used to harvest microalgae include membrane filtration (Lorente et al., 2017), foam fractionation (Ndikubwimana et al., 2016a), chemical/biological flocculation (Chatsungnoen and Chisti, 2016; Ndikubwimana et al., 2016b), electrolytic coagulation (Fayad et al., 2017), ultrasonic aggregation (Wang et al., 2015), magnetic separation (Safarik et al., 2017) and gravity sedimentation (Griffiths et al., 2012). Each of these methods has been reported, with some promising results under specific production conditions.
To generate algal biomass in an economically feasible, environmentally friendly way requires selection of appropriate harvesting technologies. Several factors must be considered when selecting the harvesting strategy, including strain phenotype, ionic strength and pH conditions of the culture medium, recycling of spent medium, and the final quality of harvested biomass. Chemical flocculation with polyvalent metal ions or polymeric flocculants is widely used in water treatment and has been shown to efficiently separate microalgal cells from its growth medium (Chatsungnoen and Chisti, 2016). For some species, flocculation can also be stimulated by changing the pH of the medium. However, increasing the pH can lead to precipitation of magnesium, calcium, phosphate and carbonate salts along with the algal cells (Vandamme et al., 2012; Wu et al., 2012). In contrast, decreasing the pH neutralizes negative charges on the cell surface, which decreases dispersal forces among the cells thereby promoting flocculation; the flocculated material can then be collected in a much smaller total culture volume (Liu et al., 2013) and be further concentrated by centrifugation. It was recently shown that the power consumption during the harvesting of microalgae by coagulation flocculation was much lower relative to the power consumption of conventional centrifugation [only 2.1 kWh/kg for Chlorella vulgaris and 0.2 kWh/kg for Phaeodactylum tricornutum (Vandamme et al., 2011) compared to ∼16 kWh/kg when assuming a microalga biomass concentration of 0.5 kg/m3 in open ponds (Danquah et al., 2009)]. In sum, algal cell flocculation prior to harvesting by centrifugation could significantly reduce the energy ‘cost’ of the collection procedure (Xu et al., 2011).
Although it appears from previous studies that flocculation is an efficient technique to pre-harvest microalgal cells, there is still uncertainty about how general the procedure is for different species and for diverse strains of a single species that are phenotypically distinct, which would include differences in cell size, surface charge properties and motility. It would also be valuable to explore how cation and pH triggered flocculation is impacted when the production strains are lacking or deficient in a cell wall; such strains may be more amenable to molecular and genetic engineering as well as cell disruption.
To further develop simple, inexpensive methods to facilitate algal cell harvesting by flocculation, we evaluated flocculation of the green unicellular algae Chlamydomonas reinhardtii (hereafter Chlamydomonas) using a combination of metal cations in addition to medium of different pHs. Different strains with different physical and biological properties (cell wall, size, motility, etc.) were examined in these studies to determine if specific physical characteristics impact the extent or efficiency of flocculation. We therefore analyzed several Chlamydomonas strains with different properties including two wild-type strains, CC124 and CC1690 (also known as 21gr+), that are widely used in the laboratory, and the cell wall deficient (cw-) strain cw15 sta6 (BAFJ5, lack flagella), which was derived from strain CC330 by random insertional mutagenesis with the ARG7-containing plasmid (Zabawinski et al., 2001); cw15 sta6 is unable to synthesize starch as a consequence of the STA6 gene deletion and lacks motility as it does not have a flagellum (Wang et al., 2009). Since the sta6 mutant displays normal growth rates (relative to wild-type cells) in acetate-supplemented medium but does not synthesize starch, many studies over the last two decades have explored carbon partitioning in this strain and its potential to synthesize high levels of lipids for the production of sustainable, renewable liquid fuels (Siaut et al., 2011; Blaby et al., 2013; Goodenough et al., 2014). In addition, we examined CC400, which is a cw- strain that synthesizes starch, and a sta6 strain (CC4567) genetically rescued for the starchless phenotype by transformation with a wild-type copy of the STA6 gene [sta6::STA6, hereafter designated C6 (Li et al., 2010)]; these latter strains help distinguish the impact of starch accumulation and cell wall synthesis on flocculation. Overall, our findings challenge the idea that immobile strains that lack flagella and/or cell walls more readily flocculate, while at the same time furthering our understanding of flocculation in distinct Chlamydomonas strains. Similar experiments can now be performed with a range of microalgae that show promise for industrial applications.
Chlamydomonas reinhardtii cells were cultured in sterile Tris-Acetate-Phosphate (TAP) (Chlamydomonas Resource Center1) and high salt (HS) medium (Sueoka, 1960) adjusted to pH 7.2. For all experiments the cells were grown in triplicate in Erlenmeyer Flasks at 25°C with shaking. The C. reinhardtii strains (Supplementary Table S1) used in these experiments were standard parental strains CC124 (137c mt- nit1 nit2) and CC1690 (mt+ NIT1 NIT2), the cell wall deficient strain CC400 (cw15), the cell wall-deficient mutant with a lesion at the STA6 locus CC4348 (cw15 arg7-7 nit1 nit2 sta6-1::ARG7) that is generally denoted sta6, and the sta6 rescued strain CC4567, generally denoted sta6-C6, which was generated by transformating sta6 with the plasmid pSL-STA6; this plasmid carries a genomic copy of the wild-type STA6 gene (cw15 arg7-7 nit1 nit2 sta6-1::ARG7 STA6). These strains were obtained from the Chlamydomonas Resource Center. Before use in flocculation experiments, 5 mL of cells grown photoautotrophically in HS medium were inoculated into 100 mL of TAP medium in a 250-mL Erlenmeyer flask that was exposed to continuous light (100 μmol photons m-2s-1) with agitation (150 rpm) at 25°C for 8 days.
Flocculation experiments were performed at an algal density of ∼0.7 g dry weight per liter. The effects of pH were examined for algal suspensions by either increasing or decreasing the pH with the addition of sodium hydroxide (NaOH) and hydrochloric acid (HCl) from 2 M stock solutions. Experiments were performed in 100-mL beakers that were magnetically stirred and mixed vigorously for 2 min during and immediately after pH adjustments. For metal cation treatments, 5 M stock solutions of ferric chloride (FeCl3), calcium chloride (CaCl2), and magnesium chloride (MgCl2) were prepared and each was added to the medium to generate final concentrations of 0, 1, 2.5, 5, 10, and 30 mM.
To evaluate flocculation, 20 mL aliquots of the cultures were collected from the beakers, transferred to disposable test tubes and incubated without agitation for 15 min. Following this potential ‘flocculation period,’ an aliquot of culture was withdrawn (from the middle of the ‘clarified zone’) and used to determine both OD750 and the absolute number of cells per mL. The flocculation efficiency was calculated according to the equation: flocculation efficiency (%) = (1-A/B) × 100 (where A represents cell number in the clarified zone and B the cell number of the reference, untreated control).
Cell numbers were determined by counting intact cells using Countess II FL (Life Technology, United States). Microscopic snapshots were taken on a Leica optical microscope (LEITZ DMRB, Germany) which also enabled determination of cell diameter. A Malvern Zetasizer 2000HSA (Malvern, United Kingdom) was used to measure the zeta potential of the algal cultures in deionized water (cells were collected from the growth medium by centrifugation and resuspended in deionized water) (Liu et al., 2013), which relates to the surface charge of individual cells. For dry cell weight, the algal cells were collected by centrifugation at 12,000 g for 5 min, washed twice with distilled water, dried at 105°C for 24 h, and weighed to obtain total dry biomass. The dried cell powder was extracted with a solvent mixture of chloroform and methanol (2:1, v/v) to calculate the lipid content (Fan et al., 2014). The starch content was analyzed using a commercial enzymatic Starch Assay Kit (SA-20, Sigma–Aldrich). The concentrations of the metal cations in the spent medium were measured using inductively coupled-atomic emission spectrometry (ICP-AES). The operating conditions for ICP-AES instrument were as previously described (Matsuura et al., 2001). The measuring wavelength and atomic/ionic lines were as follows, Fe (259.9 nm, II), Ca (317.9 nm, II) and Mg (279.0 nm, II).
Following 30 min of coagulation-flocculation, spent supernatants were tested for their ability to support algal growth (media recyclability). The pH value of the spent medium was adjusted to approximately neutral by adding HCl, while macronutrients and micronutrients were added according to the TAP medium recipe, except that we did not include acetate and added CaCl2 to 50% of the level present in TAP medium. The adjusted supernatants were tested for their ability to sustain photoautotrophic growth of Chlamydomonas (25°C, 100 μmol photons m-2s-1, bubbled with 1% CO2).
All experiments were performed in triplicate (biological) and the data, presented as a mean ± standard deviation (SD), were further analyzed by Student’s t-test (n = 3). Asterisks indicate a significant difference from the control.
We first examined three different Chlamydomonas strains to determine whether different phenotypic characteristics [difference in cell wall, flagella and size (Zabawinski et al., 2001; Siaut et al., 2011); Table 1] impact the tendency of the cells to flocculate in response to various pHs and metal cations. The strains used were the ‘wild-type’ strains CC124 and CC1690, which contain a cell wall and flagella, but significantly differ in their genetic background (Gallaher et al., 2015), the starchless mutant, sta6, which has neither a cell wall nor a flagella (Zabawinski et al., 2001; Wang et al., 2009; Siaut et al., 2011) and its complemented strain, C6, as well as CC400 (Table 1). Eight days following inoculation, the cell densities of all of the cultures increased from 5 × 105 to between 4 × 107 and 9 × 107 cell mL-1 (Supplementary Figure S1). All strains were spherical, although the average diameter of the sta6 mutant was much smaller than those of the other strains; 6.29 ± 0.37 μm (CC124), 6.88 ± 0.43 μm (CC1690), and 2.29 ± 0.20 μm (sta6) (Table 1 and Supplementary Figure S2). The maximal dry cell weight ranged from 0.63 g/L (for sta6, which attained a density of 8.81 × 107) to 0.78 g/L (for CC1690, which attained a density of 4.53 × 107); similar biomass yields of 0.5–1 g/L are considered typical for photoautotrophic cultivation of oleaginous algae in open raceway ponds (Rawat et al., 2013).
The efficiency of cell flocculation in culture was first investigated as a function of pH (Figure 1A). Since an increase in metal precipitates would occur at the higher pH values and impact the OD measurements, the flocculation efficiency was calculated according to the absolute cell number. Less than 10% of flocculation effect on cell were observed when the pH of the cultures was varied between 5 and 9 for all measured strains. In this pH range, the flocculation efficiency was less than 10% for all five of the Chlamydomonas strains. However, the flocculating efficiency increased for CC124 and CC1690 in both the acidic and alkaline pH range. In the pH range of 11–13, the flocculation efficiency was highest for the cell wall-containing strains (45–70% for CC1690 and CC124) but relatively low (∼15–30%) with significant difference for the cell wall-deficient strains (CC400, sta6 and sta6-C6). In the acidic range the flocculation efficiency was maximal at pH 4.0 for CC124 (32%) and pH 2.5 for CC1690 (26%). Overall, these results demonstrate that the highest flocculation efficiencies are obtained at high pH, although the efficiency values can be markedly different for the strains, probably a consequence differences in phenotypic characteristics, including the presence/absence of the cell wall (Table 1 and Figure 1A).
FIGURE 1. Flocculation efficiency and charge characteristics (Zeta potential) as a function of pH in three Chlamydomonas reinhardtii strains. (A) flocculation efficiency; (B) zeta potential. Sometimes the data points are not aligned, as it is quite difficult to adjust the same pH values in the solution for each strain. The data was analyzed for significant differences using the Student’s t-test (n = 3) (all mutants in comparison with the two wild-types). Asterisks indicate a significant difference from the control (∗p < 0.05, ∗∗p < 0.01).
Similar to the flocculation efficiencies, the zeta potential, which is widely used for quantification of the cell surface charge, was also pH dependent (Figure 1B). Over an 8.5-1.5 pH interval, the zeta potential showed a sharp increase from -24 to ∼0 mV. The profile of the zeta potential may have some link to flocculation efficiency; at pH 3–4, when the flocculation efficiency peaked, the cells were electrically near neutral. The zeta potential in the acidic region appeared unaffected by the presence/absence of a cell wall or by differences in cell size among the strains. However, at pH 10–13, the zeta potentials of both of wild-type strains moderately rose from ∼-25 to ∼-15 mV. In the three cell wall deficient mutants the zeta potential remained approximately the same (Figure 1B).
Several self-flocculation studies of microalgae involving pH adjustment of the growth medium have been performed (Wu et al., 2012; Liu et al., 2013). Increasing or decreasing the pH of the medium can increase the flocculation efficiency by up to 90% for green microalgae, suggesting an effective strategy for harvesting the cells. In our studies the cells were also subjected to a range of pH conditions, however, self-flocculation for wild-type cells was only relatively efficient at elevated pH (more than 10), and was never very high for cell wall deficient and starchless mutant cells (Figure 1A).
Tris-Acetate-Phosphate medium has high ionic strength with initial metal concentrations of Fe (∼1.0 mg/L), Ca (∼18.2 mg/L), and Mg (∼9.7 mg/L); after growth and pH adjustment, most of the metal salts were taken up and utilized by the algal cells. The background concentrations after flocculation were about 0.11, 1.83, and 0.94 mg/L for Fe, Ca, and Mg, respectively. Hence, it is likely that some of the flocculation elicited at high pH in the absence of additional metals (Figure 1A) is due to the presence of low concentrations of metal ions still present in the TAP media.
We selected the divalent (Ca2+ and Mg2+) and trivalent (Fe3+) cation metals for further investigating the potential for harvesting Chlamydomonas cells by flocculation. We first used cells cultured in TAP medium and grown to stationary phase, with the pH of the medium for all of the cultures reaching ∼8.5. As shown in Figure 2, divalent cations (Ca2+, Mg2+) alone did not appear to impact flocculation efficiency beyond what was observed in the absence of supplementing cations (less than 10% after 10 min for the wild-type CC1690 (Figure 2A) and the various mutants (Figures 2B–D). The monovalent cation (K+) showed similar flocculation efficiencies as that of the divalent cations, although the resulting flocculants appeared more susceptible to disaggregation (data not shown). Although flocculation efficiencies following treatment with divalent cations were relatively low, the flocculation yields were somewhat higher in wild-type cells than in the mutant (5–6% for CC1690 compared to 2–3% for sta6; compare panel a to panel b in Figure 2); this could be explained by phenotypic differences such as cell size and/or motility (Table 1). In contrast, the trivalent ferric ion elicited strong flocculation, with efficiencies at 10 mM Fe3+ exceeding 95 and 85% for CC1690 and sta6, respectively. A further increase in the Fe3+ concentration to 30 mM caused a marked drop in flocculation efficiency to ∼18 and 50% for CC1690 and sta6, respectively (Figures 2A,B).
FIGURE 2. Flocculation efficiency as a function of different metal cations and their concentrations in wild-type and cell wall-deficient Chlamydomonas reinhardtii strain at near neutral pH conditions. (A) Wild-type CC1690; (B) cell wall deficient mutant sta6; (C) CC400; (D) sta6-C6. Cells were grown to stationary phase and the pH of the medium was ∼8.5. The data was analyzed for significant differences using the Student’s t-test (n = 3) (each mutant in comparison with wild-type). Asterisks indicate a significant difference from the control (wild-type) (∗p < 0.05, ∗∗p < 0.01).
Since the pH adjustment and some cations were effective in eliciting self-flocculation for wild-type cells (Figures 1A, 2), we further explored flocculation using different metal cations at a range of pH conditions and quantified the levels of cations in the medium before and after flocculation at three different pHs (Table 2). The concentrations of Fe3+, Ca2+, and Mg2+ in the medium remained almost unchanged before and after cell flocculation at pH 4.0. However, following flocculation at pH 8.5, ∼25% of the Fe3+ and 72–84% of the Ca2+ and Mg2+ remained in the medium. Finally, cell flocculation at pH 12.0 resulted in precipitation of the majority of the metal ions from the medium, i.e., only 9.6–11.2%, 1.5–2.3%, and 18.2–29.3% of Fe3+, Ca2+, and Mg2+ remained in the medium, respectively. These results suggest that precipitation of the metal ions during flocculation occurred when the conditions were alkaline and possibly explain the relatively high flocculation efficiencies when such conditions are applied. Moreover, while precipitation of multivalent metal cations may co-occur with cells during flocculation at high pH, flocculation at low pH does not appear to be accompanied by metal ion precipitation and as a consequence, the low pH medium after flocculation still contains high cation levels and could not be used for the initiation of new cultures (Liu et al., 2013). Furthermore, metal cation precipitation during flocculation of three cell wall deficient mutants (sta6, CC400, and sta6-C6), all of which are also lacking flagella (Table 1), was generally no significant difference (Student’s t-test, p > 0.05) with what was observed for CC1690 and CC124, under any of the conditions examined (Table 2).
TABLE 2. Metal cation concentrations (5 mM of chlorides addition to the medium) before (C1) and after (C2) flocculation for wild-type and cell wall deficient Chlamydomonas reinhardtii strains at different pH level.
Since the flocculation efficiencies and charge characteristics as a function of pH were similar between CC1690 and CC124 (both are CW+), we analyzed CC1690 as a ‘wild-type’ representative for additional experiments. Since the highest flocculation efficiencies were observed at a high pH (Figure 1A), we evaluated the impact of metal cation concentrations at pH 11.5 on the flocculation efficiencies of both CC1690 and sta6. The flocculation efficiencies of CC1690 and sta6 peaked at 5–10 mM Ca2+ and Mg2+ (Figures 3A,B), with the highest flocculating activities resulting in aggregation of 90–95% of the cells (Table 1). For all divalent cations used in our study, the flocculation efficiencies either declined to some extent or remained the same when the ion concentration was elevated to 30 mM. Furthermore, Mg2+ was slightly less effective at eliciting aggregation than Ca2+ (Figure 3 and Supplementary Figure S2), and the highest flocculation efficiencies, when using Mg2+, were 89.1 and 81.9% for CC1690 and sta6, respectively. In contrast, at pH 11.5 the ferric ions generally caused some increase in flocculation at 1–5 mM, with a negative impact at higher concentrations (10 and 30 mM); at 1 mM Fe3+, the maximal flocculation efficiencies were 69.2 and 39.3%, for CC1690 and sta6, respectively. Additionally, as a consequence of flocculation with Fe3+, both the cells and supernatant turned sandy beige, while for the cultures that were subjected to Ca2+- and Mg2+-stimulated flocculation, a change in medium color did not occur (Supplementary Figure S3).
FIGURE 3. Flocculation efficiency as a function of different metal cations and their concentrations in wild-type and cell wall deficient Chlamydomonas reinhardtii strains at pH 11.5. (A) Wild-type CC1690; (B) cell wall deficient mutant sta6; (C) CC400; (D) sta6-C6. Cells were grown to stationary phase and the pH of the medium was ∼11.5. The data was analyzed for significant differences using the Student’s t-test (n = 3) (each mutant in comparison with wild-type). Asterisks indicate a significant difference from the control (wild-type) (∗p < 0.05, ∗∗p < 0.01).
Previous studies have demonstrated that flocculation elicited by lowering the pH is likely the result of neutralizing of the negative surface charges of the cells by protonation of carboxyl and/or sulfate groups (Wyatt et al., 2012; Liu et al., 2013). However, in this work we demonstrated that cell flocculation occurs at high pH using Ca2+ and Mg2+ ions, and therefore the flocculation reaction is likely to result from a chemical precipitation of the calcium and/or magnesium salts (Vandamme et al., 2012; Beuckels et al., 2013). Indeed, self-flocculation greatly reduced the level of metal cations in the medium (Table 2). Hence, our results confirm that coagulation-flocculation could be induced by the addition of metal salts (Figure 3 and Supplementary Figure S2), and that high pH (greater than 10) is required for this process to occur (Table 1).
A recent study showed that 83% of the cell wall deficient Chlamydomonas cw15 cells that were nitrogen deprived flocculated when supplemented with Ca2+ under slightly alkaline conditions (Scholz et al., 2011). Moreover, Scholz et al. (2011) demonstrated that fresh TAP medium was less active in eliciting cell flocculation than nitrogen-free TAP medium. These results suggest that a flocculation inhibitory component of the medium was consumed during growth and/or that a metabolic byproduct released into the medium by nitrogen starved cells promotes flocculation (Scholz et al., 2011).
Since we compared the ‘wild-type’ cw+ (CC1690) with the sta6 cw- starchless mutant, we sought to clarify whether or not cellular starch impacts flocculation efficiency. To address this issue we compared sta6 to two cw- strains, CC400 (widely used wall-deficient mutant) and CC4567 (sta6-C6) (complemented sta6 created by transformation of BAFJ5 with the plasmid pSL-STA6 harboring a genomic copy of the wild-type STA6 gene) that are not impaired in starch production (Li et al., 2010). Importantly, the average size of sta6 was smaller than CC400 and sta6-C6 (Supplementary Figure S2) and the cellular components, such as total lipids and starch contents among the strains varied from 11–45% to 0–39%, respectively (Table 1), with the highest lipid and no detectible starch in sta6. Despite differences in cells size and content of organic polymers, no substantial difference of Zeta potential was observed among the different strains (Table 1). Cation content in the medium derived from flocculated cells from each strain at a given pH exhibited similar levels (Table 2), although the level of cation in the medium was highly dependent on the pH value.
Flocculation efficiency at different divalent cation concentrations (pH 11.5) showed no significant difference between CC1690, CC400, sta6-C6, and sta6; all peaked at 5–10 mM Ca2+ and Mg2+ (with highest flocculating efficiencies of around 94 and 80%, respectively) (Figures 3C,D). These results suggest that cellular starch content has little overall impact on Chlamydomonas flocculation.
In this study, differences in flocculation efficiencies observed for wild-type and mutant cells are probably explained by the absence/presence of a cell wall and/or flagella. Intuitively, cells such as sta6, which lack flagella, might be thought to be more amenable to aggregation than motile cells since they might be more susceptible to the metal salt coagulants formed under strong alkali conditions. In addition, the loss of starch synthesis in sta6 does not cause re-direction of fixed carbon to the synthesis of more protein or lipid; the sta6 culture appears to accumulate ∼20% less biomass (because the cells are smaller) when grown in nutrient-replete medium (Krishnan et al., 2015), even though the cell density is higher. However, it was also shown that following growth under some stress conditions, sta6 cells could accumulate more lipids, which makes them float to the surface of the culture (Goodenough et al., 2014). Based on the flocculation features of sta6 reported here, we suggest that this strain can be effectively used for future biofuel research and production.
A highly important consideration in a large scale industrial application is the capacity to reuse the spent growth medium. The metal cation concentrations that remain in the medium following cell flocculation can have a marked and direct impact on media re-cycling capabilities. We therefore assessed the recyclability of the spent medium following photoautotrophic cultivation. Using 5–10 mM calcium with cultures of CC1690 and sta6 we achieved efficient flocculation (>90%; Figure 3) and then determined whether the used medium affected the growth of new cultures. To address this, we compared the CC1690 and sta6 growth rates over a period of 10 days in fresh and recycled medium. At any given time point the growth rate (for both CC1690 and sta6) was similar regardless of whether the cells were cultured in fresh or recycled medium (Figure 4). Moreover, both strains maintained their ability to flocculate with the addition of Ca2+ to the medium as long as there was a near neutral or alkaline environment during cultivation. Thus, residual Ca2+ or coagulant does not appear to be an obstacle to medium reuse. We also observed that cells grown in the spent supernatant, which contained a small quantity of residual Ca2+, required less Ca2+ to flocculate. However, the amount of Ca2+ salts that precipitate was likely higher in the flocculated biomass; further analysis is required to evaluate whether the Ca2+ that precipitates with the flocculated cells interferes with specific microalgal biomass applications, e.g., biofuels production or bio-refinery.
Our experiments demonstrated that self-flocculation by direct pH adjustment does not work well for Chlamydomonas and that it depends on the presence of sufficiently high metal salts (>5 mM). The highest flocculation efficiency was achieved using a combination of added Ca2+ with elevated pH (>10). Slightly more metal cation appears to be needed to flocculate sta6 (cell wall deficient, starchless mutant) potentially owing to the lack of motility, the different surface structure or the smaller cell size (Sathe and Durand, 2016). We also confirmed that cellular starch content has little overall impact on flocculation of Chlamydomonas, and importantly, the flocculated medium could be re-reused. Further examination of the flocculation mechanism would entail examining additional differences in flocculation characteristics among cells with different phenotypes, the impact of metal salts on flocculation when the culture is scaled up and whether or not the findings apply to other algal groups.
Additional research on the conditions (e.g., pH and metal ions) used for efficient flocculation of diverse algal groups with diverse characteristics, at both small and large scale, would help establish inexpensive procedures for harvesting cell biomass.
Conceived and designed the experiments: JF. Performed the experiments: JF and LZ. Analyzed the data: JF, SS, YB, and AG. Wrote the paper: JF, SS, YB, and AG.
The authors declare that the research was conducted in the absence of any commercial or financial relationships that could be construed as a potential conflict of interest.
This work was sponsored by Natural Science Foundation of Shanghai 17ZR1406700, National Natural Science Foundation of China 21505044, and the “Chenguang Program” supported by Shanghai Education Development Foundation and Shanghai Municipal Education Commission 14CG27. The authors gratefully acknowledge financial support from China Scholarship Council 201606745004.
The Supplementary Material for this article can be found online at: https://www.frontiersin.org/articles/10.3389/fpls.2017.01997/full#supplementary-material
FIGURE S1 | Cell growth of different Chlamydomonas strains.
FIGURE S2 | Light Microscopy of Chlamydomonas cells. (a) CC1690; (b) CC124; (c) sta6; (d) CC400; (e) sta6-C6. Scale bars: 10 μm.
FIGURE S3 | Microscopic images of Chlamydomonas cells flocculated by metal cations and elevated pH (11.5). (a) Wild-type CC1690 before treatment; (b) wild-type CC1690 after treated with 5 mM FeCl3; (c) wild-type CC1690 after treated with 5 mM CaCl2; (d) wild-type CC1690 after treated with 5 mM MgCl2; (e) cell wall deficient sta6 mutant before treatment; (f) sta6 mutant after treatment with 5 mM FeCl3; (g) sta6 mutant after treated with 5 mM CaCl2; (h) sta6 mutant after treated with 5 mM MgCl2. Scale bars: 10 μm.
Abomohra, A. E.-F., Jin, W., Tu, R., Han, S.-F., Eid, M., and Eladel, H. (2016). Microalgal biomass production as a sustainable feedstock for biodiesel: current status and perspectives. Renew. Sustain. Energy Rev. 64, 596–606. doi: 10.1016/j.rser.2016.06.056
Beuckels, A., Depraetere, O., Vandamme, D., Foubert, I., Smolders, E., and Muylaert, K. (2013). Influence of organic matter on flocculation of Chlorella vulgaris by calcium phosphate precipitation. Biomass Bioenergy 54, 107–114. doi: 10.1016/j.biombioe.2013.03.027
Blaby, I. K., Glaesener, A. G., Mettler, T., Fitz-Gibbon, S. T., Gallaher, S. D., Liu, B., et al. (2013). Systems-level analysis of nitrogen starvation–induced modifications of carbon metabolism in a Chlamydomonas reinhardtii starchless mutant. Plant Cell 25, 4305–4323. doi: 10.1105/tpc.113.117580
Chatsungnoen, T., and Chisti, Y. (2016). Harvesting microalgae by flocculation–sedimentation. Algal Res. 13, 271–283. doi: 10.1016/j.algal.2015.12.009
Danquah, M. K., Ang, L., Uduman, N., Moheimani, N., and Forde, G. M. (2009). Dewatering of microalgal culture for biodiesel production: exploring polymer flocculation and tangential flow filtration. J. Chem. Technol. Biotechnol. 84, 1078–1083. doi: 10.1002/jctb.2137
Demirbas, A. (2017). Production economics of high-quality microalgae. Energy Sour. B Econ. Plan. Policy 12, 395–401. doi: 10.1080/15567249.2015.1057655
Fan, J., Cui, Y., Wan, M., Wang, W., and Li, Y. (2014). Lipid accumulation and biosynthesis genes response of the oleaginous Chlorella pyrenoidosa under three nutrition stressors. Biotechnol. Biofuels 7:17. doi: 10.1186/1754-6834-7-17
Fayad, N., Yehya, T., Audonnet, F., and Vial, C. (2017). Harvesting of microalgae Chlorella vulgaris using electro-coagulation-flocculation in the batch mode. Algal Res. 25, 1–11. doi: 10.1016/j.algal.2017.03.015
Gallaher, S. D., Fitz-Gibbon, S. T., Glaesener, A. G., Pellegrini, M., and Merchant, S. S. (2015). Chlamydomonas genome resource for laboratory strains reveals a mosaic of sequence variation, identifies true strain histories, and enables strain-specific studies. Plant Cell 27, 2335–2352. doi: 10.1105/tpc.15.00508
Goodenough, U., Blaby, I., Casero, D., Gallaher, S. D., Goodson, C., Johnson, S., et al. (2014). The path to triacylglyceride obesity in the sta6 strain of Chlamydomonas reinhardtii. Eukaryot. Cell 13, 591–613. doi: 10.1128/EC.00013-14
Griffiths, M. J., van Hille, R. P., and Harrison, S. T. (2012). Lipid productivity, settling potential and fatty acid profile of 11 microalgal species grown under nitrogen replete and limited conditions. J. Appl. Phycol. 24, 989–1001. doi: 10.1007/s10811-011-9723-y
Krishnan, A., Kumaraswamy, G. K., Vinyard, D. J., Gu, H., Ananyev, G., Posewitz, M. C., et al. (2015). Metabolic and photosynthetic consequences of blocking starch biosynthesis in the green alga Chlamydomonas reinhardtii sta6 mutant. Plant J. 81, 947–960. doi: 10.1111/tpj.12783
Laurens, L. M., Chen-Glasser, M., and McMillan, J. D. (2017). A perspective on renewable bioenergy from photosynthetic algae as feedstock for biofuels and bioproducts. Algal Res. 24, 261–264. doi: 10.1016/j.algal.2017.04.002
Li, Y., Han, D., Hu, G., Dauvillee, D., Sommerfeld, M., Ball, S., et al. (2010). Chlamydomonas starchless mutant defective in ADP-glucose pyrophosphorylase hyper-accumulates triacylglycerol. Metab. Eng. 12, 387–391. doi: 10.1016/j.ymben.2010.02.002
Liu, J., Zhu, Y., Tao, Y., Zhang, Y., Li, A., Li, T., et al. (2013). Freshwater microalgae harvested via flocculation induced by pH decrease. Biotechnol. Biofuels 6:98. doi: 10.1186/1754-6834-6-98
Lorente, E., Hapoñska, M., Clavero, E., Torras, C., and Salvadó, J. (2017). Microalgae fractionation using steam explosion, dynamic and tangential cross-flow membrane filtration. Bioresour. Technol. 237, 3–10. doi: 10.1016/j.biortech.2017.03.129
Matsuura, H., Hokura, A., Katsuki, F., Itoh, A., and Haraguchi, H. (2001). Multielement determination and speciation of major-to-trace elements in black tea leaves by ICP-AES and ICP-MS with the aid of size exclusion chromatography. Anal. Sci. 17, 391–398. doi: 10.2116/analsci.17.391
Ndikubwimana, T., Chang, J., Xiao, Z., Shao, W., Zeng, X., Ng, I. S., et al. (2016a). Flotation: a promising microalgae harvesting and dewatering technology for biofuels production. Biotechnol. J. 11, 315–326. doi: 10.1002/biot.201500175
Ndikubwimana, T., Zeng, X., Murwanashyaka, T., Manirafasha, E., He, N., Shao, W., et al. (2016b). Harvesting of freshwater microalgae with microbial bioflocculant: a pilot-scale study. Biotechnol. Biofuels 9:47. doi: 10.1186/s13068-016-0458-5
Rawat, I., Kumar, R. R., Mutanda, T., and Bux, F. (2013). Biodiesel from microalgae: a critical evaluation from laboratory to large scale production. Appl. Energy 103, 444–467. doi: 10.1016/j.apenergy.2012.10.004
Safarik, I., Pospiskova, K., Baldikova, E., and Safarikova, M. (2017). “Magnetic particles for microalgae separation and biotechnology,” in Food Bioactives, ed. M. Puri (Berlin: Springer), 153–169.
Sathe, S., and Durand, P. M. (2016). Cellular aggregation in Chlamydomonas (Chlorophyceae) is chimaeric and depends on traits like cell size and motility. Eur. J. Phycol. 51, 129–138. doi: 10.1080/09670262.2015.1107759
Scholz, M., Hoshino, T., Johnson, D., Riley, M. R., and Cuello, J. (2011). Flocculation of wall-deficient cells of Chlamydomonas reinhardtii mutant cw15 by calcium and methanol. Biomass Bioenergy 35, 4835–4840. doi: 10.1016/j.biombioe.2011.08.020
Siaut, M., Cuiné, S., Cagnon, C., Fessler, B., Nguyen, M., Carrier, P., et al. (2011). Oil accumulation in the model green alga Chlamydomonas reinhardtii: characterization, variability between common laboratory strains and relationship with starch reserves. BMC Biotechnol. 11:7. doi: 10.1186/1472-6750-11-7
Sueoka, N. (1960). Mitotic replication of deoxyribonucleic acid in Chlamydomonas reinhardi. Proc. Natl. Acad. Sci. U.S.A. 46, 83–91. doi: 10.1073/pnas.46.1.83
Vandamme, D., Foubert, I., Fraeye, I., Meesschaert, B., and Muylaert, K. (2012). Flocculation of Chlorella vulgaris induced by high pH: role of magnesium and calcium and practical implications. Bioresour. Technol. 105, 114–119. doi: 10.1016/j.biortech.2011.11.105
Vandamme, D., Pontes, S. C. V., Goiris, K., Foubert, I., Pinoy, L. J. J., and Muylaert, K. (2011). Evaluation of electro-coagulation–flocculation for harvesting marine and freshwater microalgae. Biotechnol. Bioeng. 108, 2320–2329. doi: 10.1002/bit.23199
Waghmare, A. G., Salve, M. K., LeBlanc, J. G., and Arya, S. S. (2016). Concentration and characterization of microalgae proteins from Chlorella pyrenoidosa. Bioresour. Bioprocess. 3:16. doi: 10.1186/s40643-016-0094-8
Wang, W., Lee, D.-J., and Lai, J.-Y. (2015). Aggregate formation affects ultrasonic disruption of microalgal cells. Bioresour. Technol. 198, 907–912. doi: 10.1016/j.biortech.2015.09.099
Wang, Z. T., Ullrich, N., Joo, S., Waffenschmidt, S., and Goodenough, U. (2009). Algal lipid bodies: stress induction, purification, and biochemical characterization in wild-type and starchless Chlamydomonas reinhardtii. Eukaryot. Cell 8, 1856–1868. doi: 10.1128/EC.00272-09
Wu, Z., Zhu, Y., Huang, W., Zhang, C., Li, T., Zhang, Y., et al. (2012). Evaluation of flocculation induced by pH increase for harvesting microalgae and reuse of flocculated medium. Bioresour. Technol. 110, 496–502. doi: 10.1016/j.biortech.2012.01.101
Wyatt, N. B., Gloe, L. M., Brady, P. V., Hewson, J. C., Grillet, A. M., Hankins, M. G., et al. (2012). Critical conditions for ferric chloride-induced flocculation of freshwater algae. Biotechnol. Bioeng. 109, 493–501. doi: 10.1002/bit.23319
Xu, L., Brilman, D. W. W., Withag, J. A., Brem, G., and Kersten, S. (2011). Assessment of a dry and a wet route for the production of biofuels from microalgae: energy balance analysis. Bioresour. Technol. 102, 5113–5122. doi: 10.1016/j.biortech.2011.01.066
Zabawinski, C., Van Den Koornhuyse, N., D’Hulst, C., Schlichting, R., Giersch, C., Delrue, B., et al. (2001). Starchless mutants of Chlamydomonas reinhardtii lack the small subunit of a heterotetrameric ADP-glucose pyrophosphorylase. J. Bacteriol. 183, 1069–1077. doi: 10.1128/JB.183.3.1069-1077.2001
Keywords: flocculation, cell wall deficient, Chlamydomonas, multivalent metal ions, microalgae, pH, algal biotechnology
Citation: Fan J, Zheng L, Bai Y, Saroussi S and Grossman AR (2017) Flocculation of Chlamydomonas reinhardtii with Different Phenotypic Traits by Metal Cations and High pH. Front. Plant Sci. 8:1997. doi: 10.3389/fpls.2017.01997
Received: 08 September 2017; Accepted: 08 November 2017;
Published: 20 November 2017.
Edited by:
Agnieszka Ludwików, Adam Mickiewicz University in Poznań, PolandReviewed by:
Jon Pittman, University of Manchester, United KingdomCopyright © 2017 Fan, Zheng, Bai, Saroussi and Grossman. This is an open-access article distributed under the terms of the Creative Commons Attribution License (CC BY). The use, distribution or reproduction in other forums is permitted, provided the original author(s) or licensor are credited and that the original publication in this journal is cited, in accordance with accepted academic practice. No use, distribution or reproduction is permitted which does not comply with these terms.
*Correspondence: Jianhua Fan, amhmYW5AZWN1c3QuZWR1LmNu; amhmYW5zdGVyQGdtYWlsLmNvbQ==
Disclaimer: All claims expressed in this article are solely those of the authors and do not necessarily represent those of their affiliated organizations, or those of the publisher, the editors and the reviewers. Any product that may be evaluated in this article or claim that may be made by its manufacturer is not guaranteed or endorsed by the publisher.
Research integrity at Frontiers
Learn more about the work of our research integrity team to safeguard the quality of each article we publish.