- 1State Key Laboratory for Pharmaceutical Biotechnology, NJU–NFU Joint Institute for Plant Molecular Biology, College of Life Sciences, Nanjing University, Nanjing, China
- 2National Key Laboratory of Plant Molecular Genetics, Institute of Plant Physiology and Ecology, Shanghai Institutes for Biological Sciences, Chinese Academy of Sciences, Shanghai, China
- 3Department of Plant and Microbial Biology, University of California, Berkeley, Berkeley, CA, United States
Rice (Oryza sativa; background Nipponbare) contains nine HKT (high-affinity K+ transport)-like genes encoding membrane proteins belonging to the superfamily of Ktr/TRK/HKT. OsHKTs have been proposed to include four selectivity filter-pore-forming domains homologous to the bacterial K+ channel KcsA, and are separated into OsHKT1s with Na+-selective activity and OsHKT2s with Na+-K+ symport activity. As a member of the OsHKT2 subfamily, OsHKT2;4 renders Mg2+ and Ca2+ permeability for yeast cells and Xenopus laevis oocytes, besides K+ and Na+. However, physiological functions related to Mg2+ in planta have not yet been identified. Here we report that OsHKT2;4 from rice (O. sativa; background Nipponbare) functions as a low-affinity Mg2+ transporter to mediate Mg2+ homeostasis in plants under high-Mg2+ environments. Using the functional complementation assay in Mg2+-uptake deficient Salmonella typhimurium strains MM281 and electrophysiological analysis in X. laevis oocytes, we found that OsHKT2;4 could rescue the growth of MM281 in Mg2+-deficient conditions and induced the Mg2+ currents in oocytes at millimolar range of Mg2+. Additionally, overexpression of OsHKT2;4 to Arabidopsis mutant lines with a knockout of AtMGT6, a gene encoding the transporter protein necessary for Mg2+ adaptation in Arabidopsis, caused the Mg2+ toxicity to the leaves under the high-Mg2+ stress, but not under low-Mg2+ environments. Moreover, this Mg2+ toxicity symptom resulted from the excessive Mg2+ translocation from roots to shoots, and was relieved by the increase in supplemental Ca2+. Together, our results demonstrated that OsHKT2;4 is a low-affinity Mg2+ transporter responsible for Mg2+ transport to aerials in plants under high-Mg2+ conditions.
Introduction
Apart from atmospheric oxygen and soil-derived water, plants require a range of minerals for their growth and development. As two major essential mineral nutrients for plant growth, K and Mg are available to plants in the ionic form (K+ and Mg2+), and are transported into root cells by the plasma membrane-localized channels and transporters. Up to now, most studies are focused on identifying the active, high-affinity channels and transporters, which function in K+ and Mg2+ uptake from the nutrient-deficient environments (Hirsch et al., 1998; Li et al., 2001; Chérel et al., 2014; Mao et al., 2014). However, large majority of channels and transporters necessary for plants adaptation to nutrient-enriched conditions remain unknown.
Due to its key role in salt tolerance, high-affinity K+ transporters (HKTs) family has been widely studied and most of its members are characterized as being permeable for specific ions in heterologous expression systems (Uozumi et al., 2000; Horie et al., 2001; Mäser et al., 2002b; Garciadeblas et al., 2003; Yao et al., 2010). HKTs in plants and their K+ transporter (Trk and Ktr) counterparts in fungi and bacteria form a HKT/Trk/Ktr superfamily (Rodriguez-Navarro, 2000; Corratgé-Faillie et al., 2010; Yamaguchi et al., 2013). Plant HKT transporters are divided into two subgroups based on phylogenetic analyses to date (Mäser et al., 2002a; Platten et al., 2006; Horie et al., 2009; Hauser and Horie, 2010). Group I HKT members (HKT1s) are associated with retrieval of Na+ from xylem in root or sheath restricting transport and accumulation of salt in sensitive leaf tissues (Davenport et al., 2007; Munns and Tester, 2008). Grass species evolved a second class of HKT proteins, and comprehensive analysis of this group II HKTs (HKT2s) has been made in rice (Oryza sativa L.) with up to four members, OsHKT2;1, OsHKT2;2, OsHKT2;3, and OsHKT2;4 characterized for the structure, expression, and function (Ariyarathna et al., 2016). Most of HKT2s members function as Na+/K+ transporters with a role in maintaining Na+/K+ homeostasis in plants (Horie et al., 2007, 2011; Lan et al., 2010; Yao et al., 2010; Nieves-Cordones et al., 2016). OsHKT2;4 seems to be an exception as it exhibited permeability to a wide range of cations, including Ca2+ and Mg2+ when it was expressed in Xenopus laevis oocytes (Lan et al., 2010; Horie et al., 2011). However, its physiological function in rice is still unknown.
The Arabidopsis genome contains a single HKT homolog, AtHKT1;1, which functions as a Na+-selective uniporter and is not permeable to Ca2+ and Mg2+ (Davenport et al., 2007; Møller et al., 2009; Lan et al., 2010), suggesting that there are alternative transporters responsible for Ca2+ and Mg2+ transport in Arabidopsis. Ca2+ and Mg2+ are two of the most abundant divalent cations in living plant cells. Ca2+ is utilized to strengthen cell walls and a versatile messenger in almost all physiological processes in plants (Tang and Luan, 2017). The prominent role of Mg2+ is as the central atom of the chlorophyll molecule (Larkin, 2016), and it also participates in cation balance and activation of various enzymes in many fundamental processes (Shaul, 2002; Knoop et al., 2005; Bose et al., 2013). Although Ca2+ and Mg2+ are essential macronutrients required for plant growth, their overdose in the environment is toxic to plants (Tang et al., 2015; Oda et al., 2016). Thus, the transporters responsible for Ca2+ and Mg2+ homeostasis is of great importance for plant survival under low or high Ca2+ and Mg2+ conditions (Miedema et al., 2001; Li et al., 2008; Hermans et al., 2013; Mao et al., 2014; Oda et al., 2016). In contrast to the ambiguous research in Ca2+ transport, a family of Mg2+ transporters in Arabidopsis named as AtMGT (Li et al., 2001) or AtMRS2 (Gebert et al., 2009) has been studied extensively, and is found to play pivotal roles in Mg2+ transport and homeostasis in Arabidopsis. One of its members, AtMGT6/MRS2-4, is a high-affinity Mg2+ transporter, and loss-of-function of AtMGT6/MRS2-4 caused the severe growth retardation of Arabidopsis plants under low-Mg2+ conditions (Mao et al., 2014). Interestingly, AtMGT6/MRS2-4 also confers plants adaptation to high-Mg2+ conditions (Oda et al., 2016). Thus, atmgt6 plant with loss-of-function of AtMGT6/MRS2-4 displays the deficient Mg2+ transport under wide range of Mg2+ concentrations, and is a promising expression system to examine whether the potential transporters possess physiological functions relevant to Mg2+ in plants.
Although OsHKT2;4 was demonstrated to be permeable for Mg2+ in X. laevis oocytes (Lan et al., 2010; Horie et al., 2011), this has been challenged in an independent study (Sassi et al., 2012). Here, we applied the Salmonella typhimurium MM281, a bacteria mutant lacking Mg2+ transport capacity useful for identifying the Mg2+ transport activities of potential transporters (Li et al., 2001; Gebert et al., 2009), to analyze the possible Mg2+ transport through OsHKT2;4. Furthermore, its function on Mg2+ homeostasis was also explored in X. laevis oocytes and transgenic atmgt6 Arabidopsis lines. Our results revealed that OsHKT2;4 is an effective Mg2+ transporter in maintaining Mg2+ homeostasis, probably through functional coordination with MGT-type transporters in planta.
Materials and Methods
Plant Materials and Growth Conditions
Arabidopsis thaliana Columbia (Col-0) ecotype was used in this study. The T-DNA insertion mutant atmgt6 (SALK_203866) was obtained from the Arabidopsis Biological Resource Center. Homozygous individuals of atmgt6 were screened by PCR using primers listed in Supplementary Table 1. For on-plate growth assays, seeds were sterilized with 75% ethanol for 2 min, washed three times, and sown on half-strength Murashige and Skoog (MS) medium containing 0.75 mM Mg2+, 1.5 mM Ca2+, 1% sucrose (Sigma) and solidified with 0.8% phytoblend (Caisson Labs). The plates were kept at 4°C for 2 days and then were placed vertically in growth chamber under 90 μmol⋅m-2⋅s-1 light intensity with a 16 h light/8 h dark photoperiod. Three-day-old seedlings were transferred onto media containing various ions as indicated in the figure legends. For hydroponic cultures, 7-day-old seedlings germinated in half-strength MS (1/2 MS) were transferred to one-sixth-strength (1/6 MS) hydroponic medium containing 0.25 mM Mg2+ and 0.5 mM Ca2+ without sucrose for another 7 days. Plants were then transferred to hydroponic 1/6 MS media containing various contents of Mg2+. Plant materials were harvested for further analyses 2 days after treatment.
Functional Complementation of Mg2+-Transport by Salmonella typhimurium Mutant Strain MM281
The S. typhimurium mutant MM281, which lacks the Mg2+ transporter-CorA, MgtA, and MgtB, is used as a system for functional complementation analysis of candidate Mg2+-transporter genes. MM281 competent cells were transformed with empty pTrc99A vector, AtMGT10-pTrc99A or OsHKT2;4-pTrc99A plasmid by electroporation. Cells were plated onto LB medium containing 10 mM Mg2+ and indicated antibiotics (34 μg⋅mL-1 chloramphenicol and 100 μg⋅mL-1 ampicillin), and incubated at 37°C overnight. The transformants were confirmed by PCR amplification and individual positive ones were grown in liquid LB medium containing 10 mM Mg2+ and antibiotics as indicated above. Fifty micrometer IPTG was applied for the induction of protein expression. The liquid cultures were adjusted to OD600 = 1.0, diluted in a 10-fold series, and spotted 3 μL onto N-minimal medium supplemented with different concentrations of MgSO4 and the antibiotics. Growth of different strains was pictured after incubation at 37°C for 2 days. The growth rate of the three strains in liquid medium was also monitored as previously described (Mao et al., 2014). After growing in liquid LB medium to OD600 of 0.6–0.8, cells were harvested by centrifugation at 5000 × g for 10 min, washed twice with distilled water to remove excess Mg2+, and resuspended in distilled water. N-minimal medium was prepared with various concentrations of MgSO4 (0.1, 0.5, 1, and 10 mM). Cells were then adjusted to a final OD600 of 0.001–0.002. The growth of the cultures was monitored and was plotted as a function of growth time.
Plasmid Construction and Plant Transformation
For the constructs used in functional complementation assay in MM281 strain, the OsHKT2;4 cDNA fragment was amplified using the primers OsHKT2;4-FC and OsHKT2;4-RC and ligated to the pTrc99A vector. For overexpressing OsHKT2;4 in wild type and the atmgt6 mutant, the genomic fragment (containing a 1.92 kb promoter region upstream of the ATG starting codon and 1731 bp coding region of OsHKT2;4) was amplified using primer pair OsHKT2;4-OE-F and OsHKT2;4-OE-R and cloned into pCAMBIA1300 vector. This construct was introduced into Agrobacterium tumefaciens strain GV3101 by electroporation and was selected on 1/2 MS medium containing kanamycin. The selected positive transformant was used to transform developing floral tissues of 4-week-old atmgt6 plants using the flora dip method (Clough and Bent, 1998). For expression in X. laevis oocytes, OsHKT2;4 cDNA was cloned into the pGEMHE vector downstream from the T7 promoter using primers OsHKT2;4-FP and OsHKT2;4-RP. All primer pairs were listed in Supplementary Table 1.
Gene Expression Analysis
Total RNA was extracted from rosette leaves using the TRizol Reagent (Invitrogen), and the first-strand cDNA was synthesized by M-MLV Reverse Transcriptase (Promega) following the manufacturer’s instructions. The semi-quantitative RT-PCR analysis of gene expression using cDNA of Col-0, atmgt6, OE29, and OE24 followed by a 26 cycles of PCR amplification. AtActin2 (AT3G18780) was used as the internal reference. Primers used are listed in the Supplementary Table 1.
Expression in Xenopus laevis Oocytes and Two-Electrode Voltage Clamp
cRNA was synthesized from 1 μg linearized DNA template using a mMessage mMachine in vitro transcription kit (Ambion) according to the manufacturer’s recommendations and stored at -80°C. Stage V to VI X. laevis oocytes were harvested, defolliculated, and cultured in ND96 solution containing 96 mM NaCl, 2 mM KCl, 1.8 mM CaCl2, 1 mM MgCl2, 25 μg⋅mL-1 gentamicin, pH 7.4 adjusted with 5 mM HEPES/NaOH. Approximately 50 ng of cRNA, in a total volume of 23 nL, was injected into each X. laevis oocyte. Oocytes of 2 days after injection were used for two-electrode voltage-clamp analysis. The perfusion solution was used as described previously (Lan et al., 2010) with some modifications. The perfusion solution contained (in mM) 1 K-gluconate, 1 Na-gluconate, 185 mannitol, and 10 Mes-Tris (pH 7.4). The recording pipette contained 3 M KCl. The currents were recorded by hyperpolarized pulses of a 0.2 s prepulse at -40 mV followed by voltage steps of 60 to -150 mV (in 15 mV decrements, 1.8 s duration) followed by a 1.5 s deactivation at 0 mV. The current-voltage (I-V) curves plot current values at the end of each voltage-clamp episode (t = 2 s, n = 6 for each group).
Ion Content Measurement
Two-week-old hydroponically grown plants were exposed to solution containing different concentrations of Mg2+. After 2-day exposure, both the roots and shoots were harvested and sampled for analysis. The dry weight (DW) of the samples was measured after drying for 48 h at 60°C. Subsequently, the samples were digested in 0.5 ml of 70% HNO3 at 100°C for 30 min on a digester (DigiBlock ED16, LabTech). Ion concentration was measured by Inductively Coupled Plasma-Mass Spectrometry (ICP-MS) (PerkinElmer NexION 300).
Results
OsHKT2;4 Rescued the Growth of Bacterial Strain MM281 in the Mg2+-Deficient Medium
To determine whether OsHKT2;4 functions in Mg2+ transport, a cDNA fragment containing the complete open reading frame with 1530 bases encoded a protein of 509 residues was cloned and expressed in S. typhimurium mutant strain MM281. MM281 is incapable of loading Mg2+ into cellular compartment, as it lacks three functional Mg2+ transporters CorA, MgtA, and MgtB, and its growth is retarded or arrested when the culture medium contains less than 10 mM Mg2+ (Townsend et al., 1995; Li et al., 2001). Therefore, complementation of this strain has proved useful in identifying and developing information about potential Mg2+ transporters, including AtMGTs (Li et al., 2001, 2008; Hicks et al., 2003; Mao et al., 2008; Chen et al., 2009; Mao et al., 2014).
We used AtMGT10 (AT5G22830), also named as AtMRS2-11, as the positive control in the complementation assay of MM281 due to its high affinity in Mg2+ transport in MM281 system (Li et al., 2001). As shown in Figure 1A, the MM281 mutant strains exogenously expressing empty vector pTrc99A, AtMGT10-pTrc99A, or OsHKT2;4-pTrc99A grew normally in the medium with 10 mM Mg2+. The strains expressing OsHKT2;4-pTrc99A exhibited faster growth than those expressing the empty pTrc99A vector in the media containing low concentrations of Mg2+ (1 and 2 mM), and still grew but to a less extent in the media containing 500 and 100 μM Mg2+, while the control did not grow at these conditions (Figure 1A), suggesting that OsHKT2;4 renders the mutant strains more tolerant to Mg2+ deficiency by enhancing the Mg2+ transport activity. However, OsHKT2;4 was less effective to restore the growth of mutant strains compared with AtMGT10 in the media containing insufficient Mg2+. For example, AtMGT10 rescued MM281 growth in medium containing 10 μM Mg2+ (Figure 1A), as shown before (Li et al., 2001), while OsHKT2;4 did not (Figure 1A). These results indicated that although OsHKT2;4 had the Mg2+ transport activity similar to AtMGT10, it might have the kinetic property with lower affinity to Mg2+ in heterologous MM281 system.
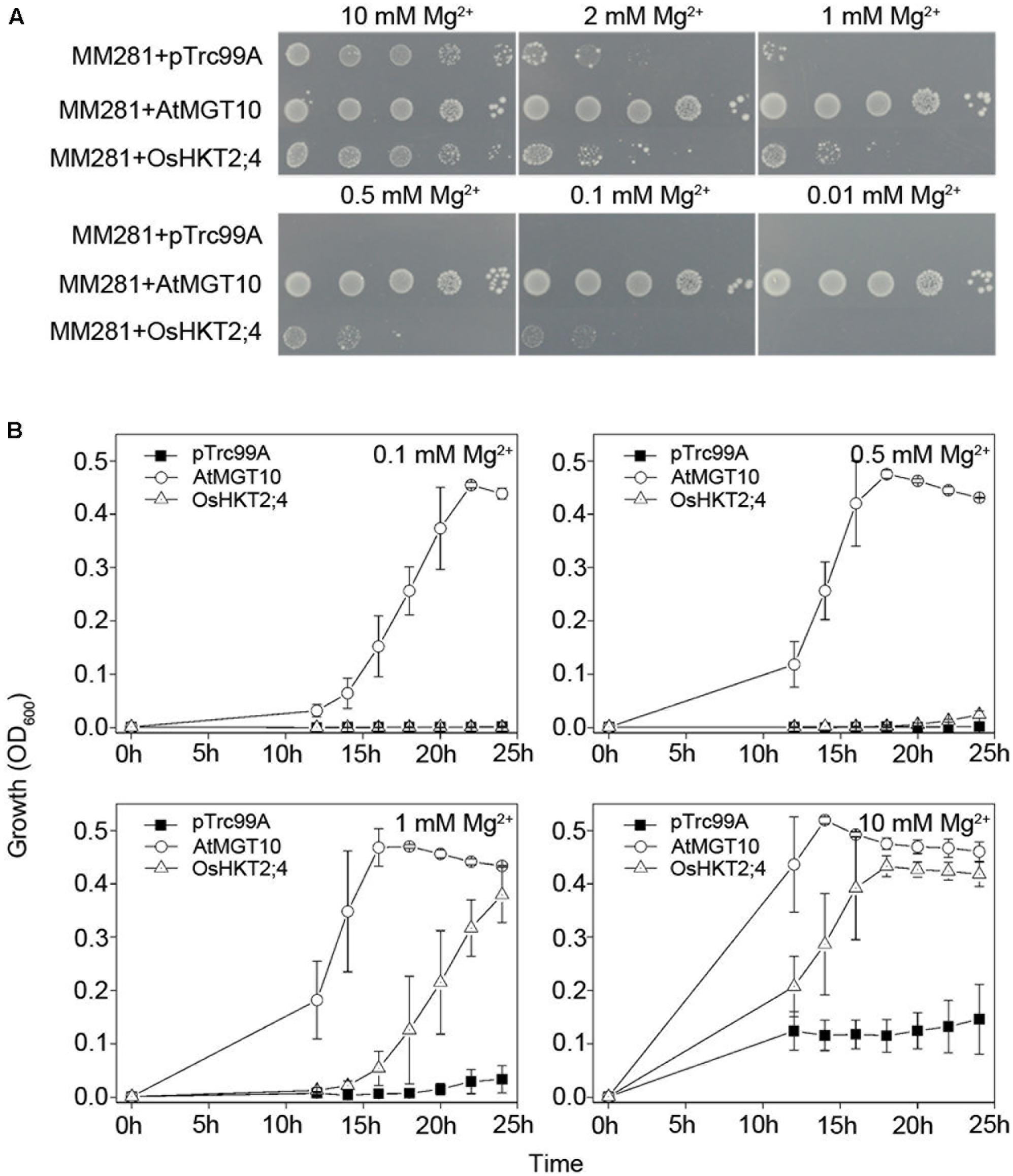
FIGURE 1. Complementation of growth defects of bacterial mutant strains MM281 by OsHKT2;4 under the low-Mg2+ conditions. (A) Growth of bacterial strains on N-minimal medium containing 0.01, 0.1, 0.5, 1, 2, or 10 mM Mg2+. The strains used in this assay were the strains MM281 transformed with the empty pTrc99A vector only (MM281+pTrc99A), coding sequence of MGT10 in the pTrc99A vector (MM281+MGT10), or coding sequence of OsHKT2;4 in pTrc99A vector (MM281+OsHKT2;4). From left to right is a 10-fold dilution series of bacterial cultures. (B) Growth curves of bacterial strains in liquid cultures. Bacterial cells described in (A) were grown in N-minimal liquid medium containing increasing concentrations of Mg2+ from 0.1 to 10 mM. Aliquots of the cultures were taken and monitored every 2 h by OD600 readings for the cell density from 10 to 24 h. Data are represented as the mean ± SD, n = 3.
To further verify the complementation of OsHKT2;4 for the growth of MM281 tested in the agar plates (Figure 1A), the bacteria were cultured in the liquid media containing 0.1, 0.5, 1, or 10 mM Mg2+, and their growth curves were established within 24 h after cultured. As shown in Figure 1B, the strain expressing AtMGT10 grew the most rapidly at these Mg2+ concentrations, supporting that AtMGT10 is a high-affinity Mg2+ transporter. In addition, MM281 expressing OsHKT2;4 displayed faster growth than those with empty vector pTrc99A under the conditions in which the Mg2+ concentration was 1 or 10 mM. By contrast, in the presence of 0.1 or 0.5 mM Mg2+, the strains expressing OsHKT2;4 displayed the similar rate of growth to the strains expressing empty vector pTrc99A. These results were consistent with the ones observed on the agar plates, and demonstrated that OsHKT2;4 might mediate low-affinity Mg2+ uptake in vivo.
Mg2+-Dependent Currents Generated by OsHKT2;4 Expressing X. laevis Oocytes under High-Mg2+ Conditions
To further assess the transporting properties of OsHKT2;4 under different Mg2+ concentrations, two-electrode voltage-clamp experiment using X. laevis oocytes was performed. OsHKT2;4-dependent currents were recorded from the oocytes injected with OsHKT2;4 cRNA or the oocytes injected with water perfused with different Mg2+ concentrations. The oocytes injected with water produced small endogenous currents in perfusion medium with 6 mM Mg2+ (Figures 2A-a). In contrast, OsHKT2;4-expressing oocytes generated the larger currents in the solutions containing 1.2, 6, and 20 mM Mg2+ (Figures 2A-b–d). The current-voltage relationship displayed the currents from OsHKT2;4-expressing oocytes perfused with 6 or 20 mM Mg2+ were significantly larger than those from OsHKT2;4-expressing oocytes perfused with 0.3 or 1.2 mM Mg2+. It was noteworthy that the currents from OsHKT2;4-expressing oocytes perfused with 0.3 mM Mg2+ were similar to those with 1.2 mM Mg2+, implying they might not be Mg2+ sensitive under low-Mg2+ conditions (Figure 2B).
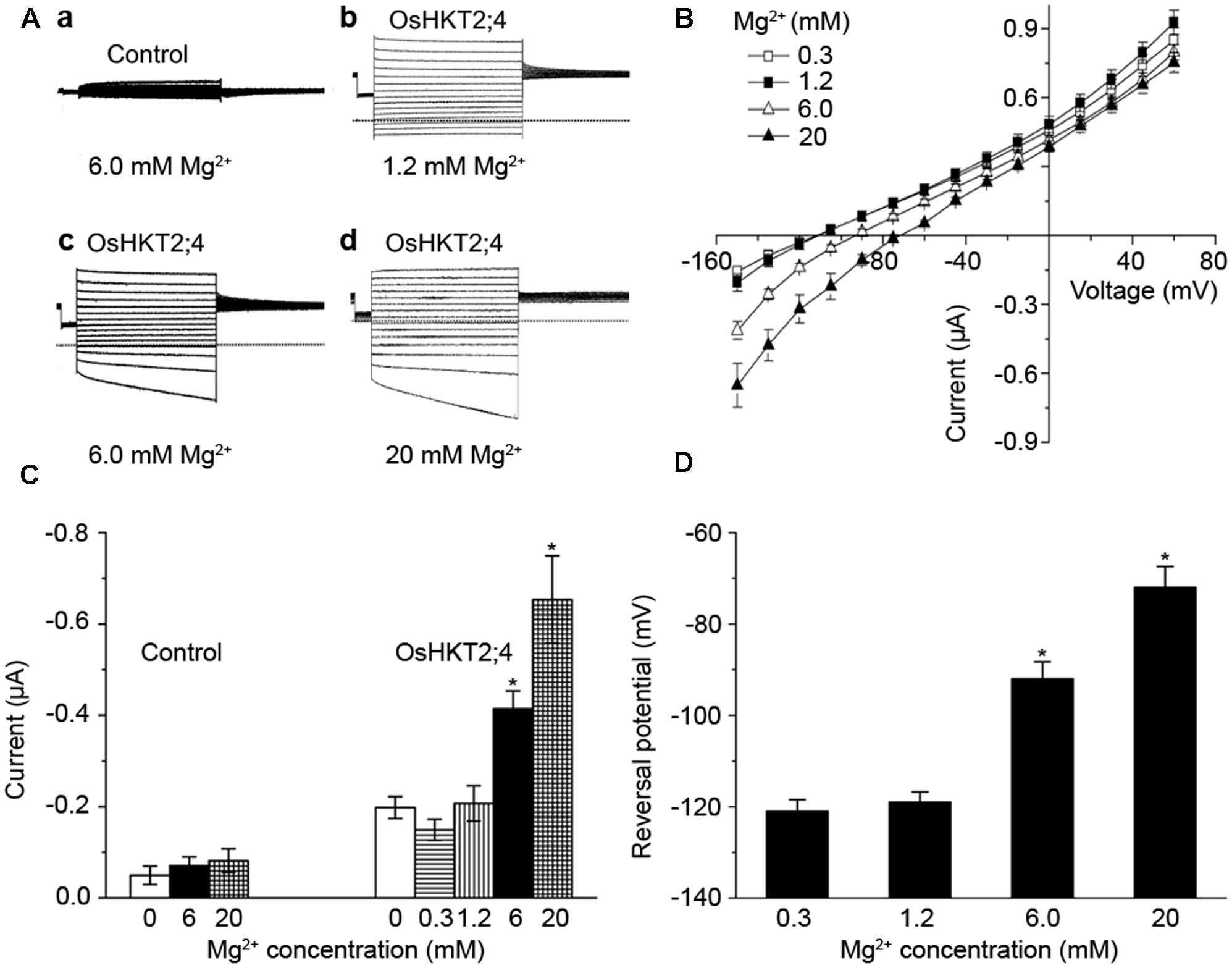
FIGURE 2. The oocytes expressing OsHKT2;4 produced Mg2+ currents under the high-Mg2+ conditions. (A) The typical current traces generated from the oocytes injected with water perfused with (a) 6 mM Mg2+ (Control) and from the oocytes expressing OsHKT2;4 (OsHKT2;4) perfused with (b) 1.2 mM, (c) 6 mM or (d) 20 mM Mg2+. Dotted lines represent the zero current level. (B) The current-voltage relationships deduced from the oocytes expressing OsHKT2;4 perfused with 0.3, 1.2, 6 or 20 mM Mg2+. Summarized current data are from 8 cells/condition. (C) The current amplitudes at –150 mV recorded from the oocytes injected with water (Control) and oocytes expressing OsHKT2;4 (OsHKT2;4) perfused with different Mg2+ concentrations. (D) Reversal potentials of currents generated from the oocytes expressing OsHKT2;4 in the presence of various concentrations of Mg2+ as indicated in the figure. Data in Figure 2 are presented as representative recordings or as mean ± SE of n (n = 6) observations with three repetitions, in which n is the number of samples. Asterisks indicate statistically significant differences compared with data from oocytes expressing OsHKT2;4 perfused with 1.2 mM Mg2+ (Unpaired student’s t-test, ∗P < 0.05).
To test this possibility, we compared the amplitude and reversal potential of the currents generated from the oocytes perfused with 0, 0.3, 1.2, 6, or 20 mM Mg2+. The currents generated from the oocytes expressing OsHKT2;4 perfused with 0.3 or 1.2 mM Mg2+ displayed the similar levels of the amplitudes and reversal potentials, even were similar to those without Mg2+ (Figures 2C,D). These Mg2+ insensitive currents were larger than the currents from the oocytes injected with water (Figure 2C), and thus they may result from other ions, such as Na+ or K+ currents generated by OsHKT2;4, as suggested by the previous studies (Lan et al., 2010; Horie et al., 2011). By contrast, in the presence of 6 or 20 mM Mg2+, the oocytes expressing OsHKT2;4 produced the currents with larger amplitudes and less negative reversal potentials compared with the others (Figures 2C,D). Thus, these results further supported the hypothesis that OsHKT2;4 exhibits permeability for Mg2+ only under the conditions containing high-Mg2+ concentrations.
Overexpression of OsHKT2;4 Enhanced the Sensitivity of atmgt6 to High Mg2+ But Not to Low Mg2+
We have shown previously that AtMGT6, a Mg2+ deficiency-induced Mg2+ transporter, mediates directly Mg2+ uptake in roots and is required for plant adaptation to low-Mg2+ environment (Mao et al., 2014). An independent study reported ethyl methanesulfonate (EMS)-mutagenized AtMGT6, or named as AtMRS2-4, caused plant growth defects under both low and high-Mg2+ conditions (Oda et al., 2016). Considering the critical role of AtMGT6 in Mg2+ acquisition, we suggested the activity of Mg2+ transport conducted by OsHKT2;4 might be covered by this transporter, and thus generated transgenic OsHKT2;4 Arabidopsis lines with the disruption of AtMGT6 to examine the potential relevance of OsHKT2;4 to Mg2+ responses in planta. We used an Arabidopsis T-DNA insertion line (SALK_203866), in which T-DNA was inserted into the third exon of AtMGT6 gene (Figure 3A). Transcript of AtMGT6 in the line SALK_203866 was not detected by RT-PCR (Figure 3C), indicating that the T-DNA insertion line was a knockout allele, and referred to as atmgt6 line hereafter. We then expressed the coding region of OsHKT2;4 into atmgt6 line driven by its native promoter (Figure 3B). The transformants were screened by hygromycin, and were further analyzed for the expression levels of OsHKT2;4 by RT-PCR. We selected two of them as the representative transgenic lines due to their relatively high OsHKT2;4 expression levels, and referred to as OE29 and OE34, respectively, to perform subsequent experiments (Figure 3C).
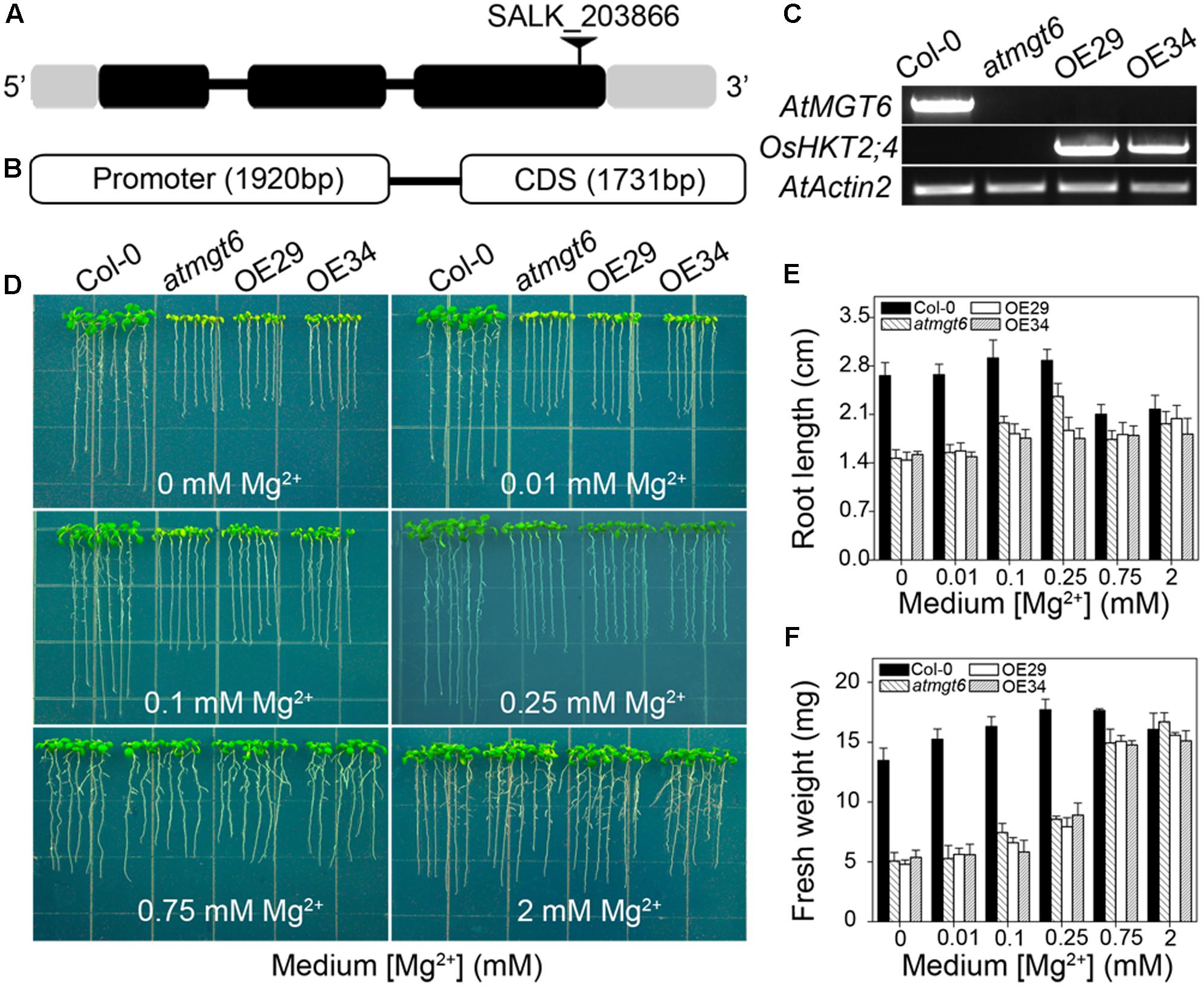
FIGURE 3. Genetic characterization and phenotypic analysis of atmgt6 mutant lines and their OsHKT2;4 overexpressed lines in low-Mg2+ conditions. (A) Scheme of AtMGT6 gene structure and position of the T-DNA insertion of SALK_203866. The gray boxes indicate 5′ and 3′ untranslated regions, and black boxes and lines indicate exons and introns, respectively. The T-DNA insertion is shown as the triangle above the gene diagram. (B) Gene fragment of OsHKT2;4, including its promoter and CDS region that was introduced into atmgt6 lines. (C) Semi-quantitative mRNA levels of AtMGT6 and OsHKT2;4 by RT-PCR analysis in wild type (Col-0), AtMGT6 knockout mutant (atmgt6), and two atmgt6 lines overexpressing OsHKT2;4 (OE29 and OE34). AtActin2 was used as the internal standard. (D) The growth of Col-0, atmgt6, OE29, and OE34 under the Mg2+-deficient conditions. After planted in half-strength Murashige and Skoog (1/2 MS) medium for 3 days, Col-0, atmgt6, OE29, and OE34 were transferred to one-sixth-strength MS (1/6 MS) medium containing 0, 0.01, 0.1, 0.25, 0.75, and 2 mM Mg2+ in total, and were photographed after growing for 7 days. Quantitative analyses of primary root length (E) and whole-plant fresh weight (F) of Col-0, atmgt6, OE29, and OE34 under the Mg2+-deficient conditions described in (D). Six independent 10-day-old seedlings of each genotype were gathered as one biological repeat for root length and fresh weight measurement. Data are represented as the mean ± SD, n = 3, in which n is the number of biological repeat.
We examined the growth of the Col-0, atmgt6, and transgenic lines OE29 and OE34 in Mg2+-depleted medium supplemented with various contents of Mg2+ as indicated in Figure 3D. The mutant atmgt6 exhibited growth defects in the medium containing 0, 0.01, 0.1, or 0.25 mM Mg2+, and had lower fresh weight and shorter roots than those of the Col-0 plants, while the growth retardation could be rescued in the Mg2+-sufficient medium (2 mM Mg2+) (Figure 3D), consistent with the idea that AtMGT6 confers low-Mg2+ tolerance for Arabidopsis (Mao et al., 2014; Oda et al., 2016). However, OsHKT2;4 overexpression could not rescue the growth deficiency of atmgt6 in low-Mg2+ conditions as expected, as transgenic lines OE29 and OE34 displayed the similar growth phenotype to atmgt6 with no significant differences on fresh weight and root length under these tested conditions (Figures 3D–F). These results suggested that OsHKT2;4 might not function in low-Mg2+ conditions in planta.
As shown in the experiments in vitro, OsHKT2;4 exhibiting Mg2+ transport activity in both heterologous MM281 system and X. laevis oocytes happened only at high external Mg2+ concentrations (Figures 1, 2). Therefore, we presumed that OsHKT2;4 might mediate Mg2+ transport when plants were cultivated under the Mg2+ abundant conditions, though it was unable to function in low-Mg2+ conditions in planta (Figures 3D–F). To conduct assessment of the sensitivity to high-Mg2+ condition of OsHKT2;4, we used 1/6 MS medium supplemented with several concentrations of Mg2+ (2, 4, 6, 8, and 10 mM) for growth assays. After growing on 1/6 MS for 2 weeks, both the root length and fresh weight of transgenic lines OE29 and OE34 were comparable to those of atmgt6 under the normal condition. However, addition of 2 mM Mg2+ resulted in growth arrest of OE29 and OE34 compared with atmgt6. Further increases of extra Mg2+ (up to 10 mM) demonstrated a consistent dosage-dependent inhibitory manner (Figure 4A). Quantitative analysis of root length (Figure 4B) and fresh weight (Figure 4C) indicated that, compared with Col-0 and atmgt6, the aerial parts of OE29 and OE34 exhibited a more severe growth retardation in Mg2+-abundant conditions, while their root length were not altered. Taken together, these results demonstrated that OsHKT2;4 results in Mg toxicity on aerial tissues in high-Mg2+ conditions in Arabidopsis, supporting the idea of low-affinity Mg2+ uptake of OsHKT2;4.
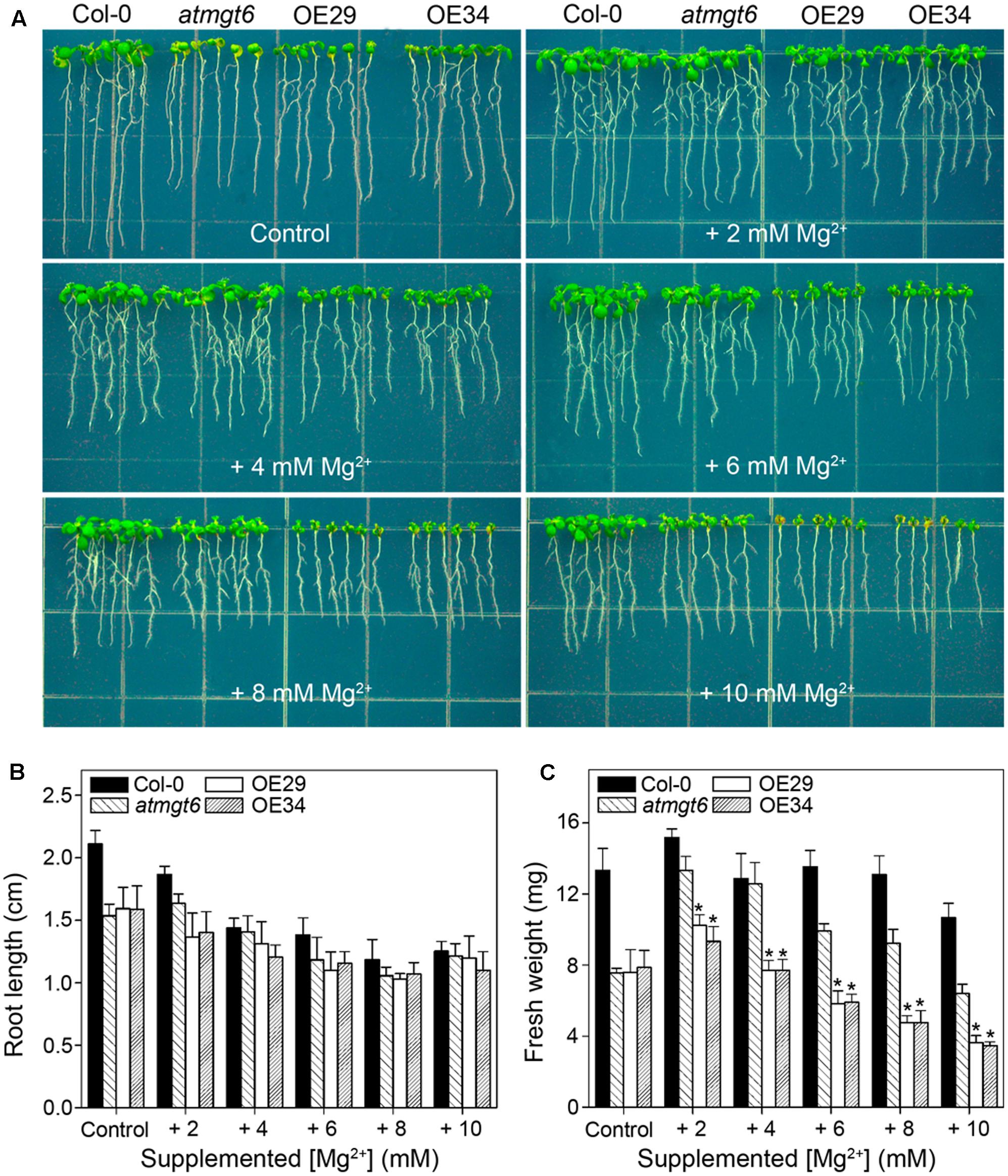
FIGURE 4. Growth phenotype of atmgt6 and transgenic OsHKT2;4-overexpression atmgt6 lines in high-Mg2+ conditions. (A) The growth of Col-0, atmgt6, OE29, and OE34 under the Mg2+-abundant conditions. After planted in half-strength MS (1/2 MS) medium for 3 days, Col-0, atmgt6, OE29, and OE34 were transferred to one-sixth-strength MS (1/6 MS, referred to as “Control” in the figure) containing a basal 0.25 mM Mg2+ and 1/6 MS medium supplemented with extra 2, 4, 6, 8, and 10 mM Mg2+. Plants were photographed after growing for another 7 days. Quantitative analyses of primary root length (B) and whole-plant fresh weight (C) of Col-0, atmgt6, OE29, and OE34 under the Mg2+-abundant conditions described in (A). Six independent 10-day-old seedlings of each genotype were gathered as one biological repeat for root length and fresh weight measurement. Data are represented as the mean ± SD, n = 3, in which n is the number of biological repeat. Asterisks indicate statistically significant differences compared with atmgt6 (Student’s t-test, ∗P < 0.05).
To examine whether the high-Mg2+ toxic phenotype is a consequence of the ectopic expression of OsHKT2;4 in OE lines, we conducted an RT-PCR analysis to verify the expression of OsHKT2;4 under a high-Mg2+ condition supplemented with 6 mM Mg2+. As demonstrated in Supplementary Figure 5, OsHKT2;4 was mainly expressed in shoot tissues under normal growth conditions, consistent with the previous report in rice (Lan et al., 2010). Moreover, the expression of OsHKT2;4 in the shoots was not significantly induced by 6 mM Mg2+, and even decreased after 24 h′ treatment with 6 mM Mg2+. However, expression of OsHKT2;4 in the roots was dramatically induced after 4 h′ treatment with 6 mM Mg2+, and became even stronger after 24 h of this treatment (Supplementary Figure 5). The oppose effect on OsHKT2;4 expression roots and shoots upon high Mg2+ suggested a disturbance on Mg2+ balance between roots and shoots under the high-Mg2+ conditions.
Overexpression of OsHKT2;4 Affected Mg2+ Homeostasis in the atmgt6 Lines
To probe the reason responsible for the increased sensitivity to high external Mg2+ in the OE plants, Mg2+ concentration of the atmgt6 and OsHKT2;4 overexpression lines was determined using ICP-MS. Plants were grown hydroponically for 2 weeks and then transferred to a fresh hydroponic medium containing 0, 0.25 (referred to as “Control” in Figure 5), and 6 mM Mg2+ for another 2 days before the roots and shoots were harvested, respectively, for analysis. As shown in Figure 5A, Mg2+ content was consistently higher in the OE lines than atmgt6 in shoot tissues when plants grown in all Mg2+ regimes tested (0, 0.25, and 6 mM). In analysis of Mg2+ content of root tissues among different plants, although they exhibited similar and incremental Mg2+ content in the Mg2+-deficient and normal medium, the OE lines contained ∼30% less Mg2+ compared with the atmgt6 plants in 6 mM Mg2+ condition (Figure 5B). These results indicated an altered Mg2+ distribution ratio in shoot and root. We thus analyzed the Mg2+ partitioning between shoots and roots in atmgt6 and OE lines, and noticed that as the Mg2+ level elevated, more Mg2+ sequestered in root tissues than in low-Mg2+ condition. In medium containing 6 mM Mg2+, the shoots of atmgt6 accumulated ∼37%, while the OE lines accumulated over ∼50% of the total Mg2+ enclosed in plants (Figure 5C), further confirming the critical role of OsHKT2;4 in the Mg2+ allocation between shoots and roots.
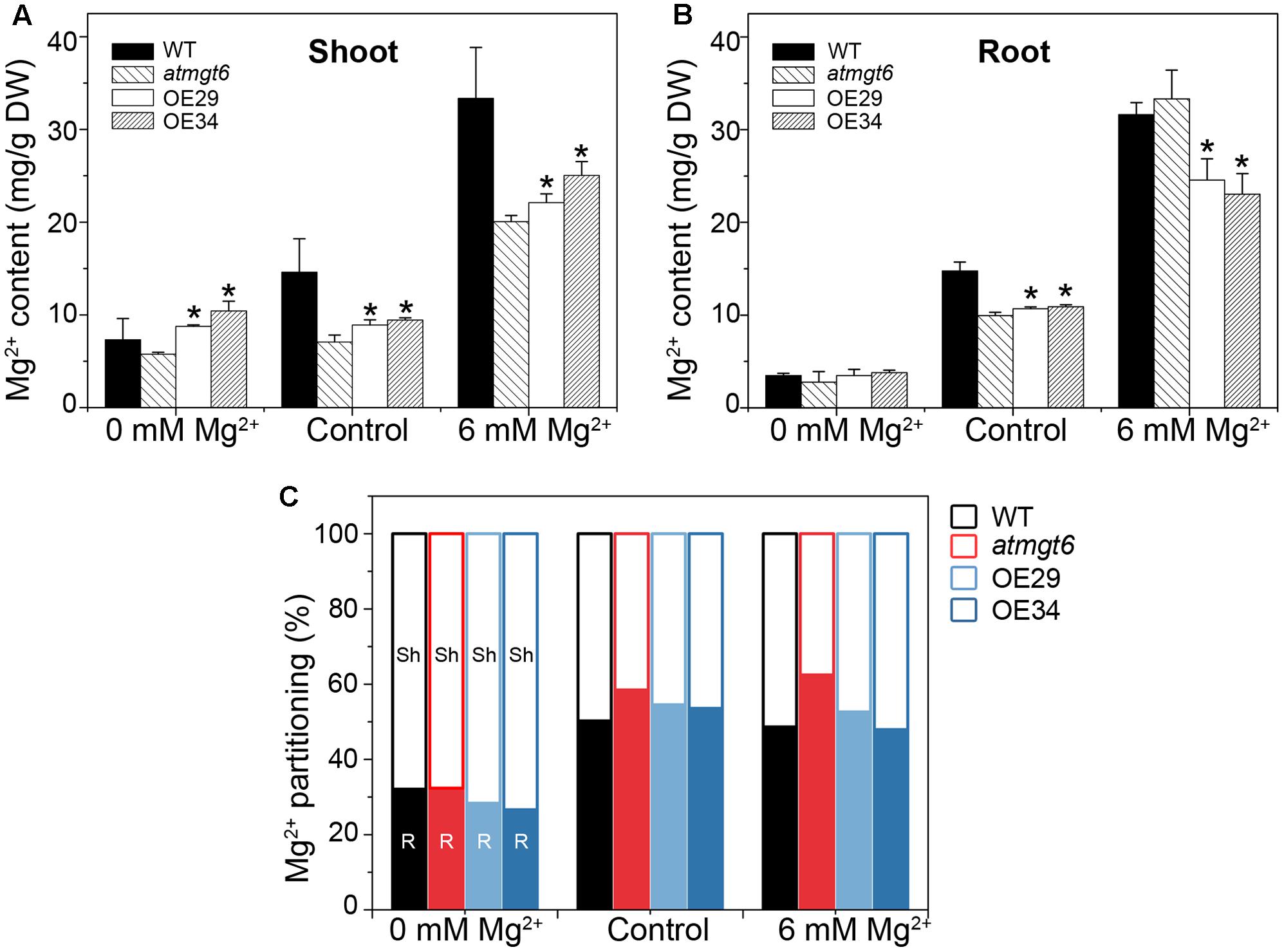
FIGURE 5. Mg2+ content and its partitioning in shoots and roots of atmgt6 and transgenic OsHKT2;4-overexpression atmgt6 lines. Inductively Coupled Plasma-Mass Spectrometry (ICP-MS) analysis of Mg2+ contents in shoots (A) and roots (B) of Col-0, atmgt6 and two transgenic OsHKT2;4-overexpression atmgt6 lines, OE29 and OE34. After planted in the hydroponic medium containing 0.25 mM Mg2+ for 2 weeks, Col-0, atmgt6, OE29, and OE34 were transferred to the hydroponic medium containing 0, 0.25 (Control), or 6 mM Mg2+, and were harvested for elemental analysis of roots and shoots after growing for 2 days. Data are represented as the mean ± SD, n = 3. Asterisks indicate statistically significant differences compared with atmgt6 (Student’s t-test, ∗P < 0.05). (C) Altered Mg2+ partitioning between shoot (Sh) and root (R) in Col-0, atmgt6, OE29, and OE34. Values are deduced from (A,B).
Increased Sensitivity of atmgt6 Lines Expressing OsHKT2;4 to Excess Mg2+ Was Alleviated by Adding Ca2+
Due to the similar physical properties, Ca2+ and Mg2+ compete for the same sites of substrates (Yermiyahu et al., 1994), and the balance of Ca2+ and Mg2+ is an important factor for plant growth. Previously, evidence was presented that OsHKT2;4 acts as a channel for the transport of both Ca2+ and Mg2+ (Lan et al., 2010; Horie et al., 2011). To examine whether Ca2+ affects the high Mg2+-sensitive phenotype in OsHKT2;4 overexpressing lines, we assessed the growth of Col-0, atmgt6, atmgt6 overexpressing OsHKT2;4 lines (OE29 and OE34) on Mg2+-abundant 1/6 MS medium supplemented with different concentrations of excess Ca2+.
In normal 1/6 MS medium, OE29 and OE34 exhibited the similar growth as atmgt6. As the concentration of external Mg2+ increased, OE29 and OE34 started to show a more severe growth arrest than atmgt6 (Figure 4A). However, increasing Ca2+ improved the plant growth (Figure 6A) of all genotypes, and the improvement was more obvious in the OE29 and OE34 lines (Supplementary Figure 2). For example, fresh weight of OE lines was ∼50% of that in atmgt6 in 1/6 MS medium with extra 8 mM Mg2+ (“basal medium” in Figure 6), however, when 1 mM Ca2+ was added to this basal medium, the fresh weight of OE lines was restored and reached to ∼80% to that in atmgt6. Moreover, when 3 mM Ca2+ were added to the basal medium, both root length and fresh weight of OE29 and OE34 were recovered to almost an identical level with that of atmgt6 (Figures 6B,C and Supplementary Figure 2), supporting the notion of Ca2+-Mg2+ antagonism.
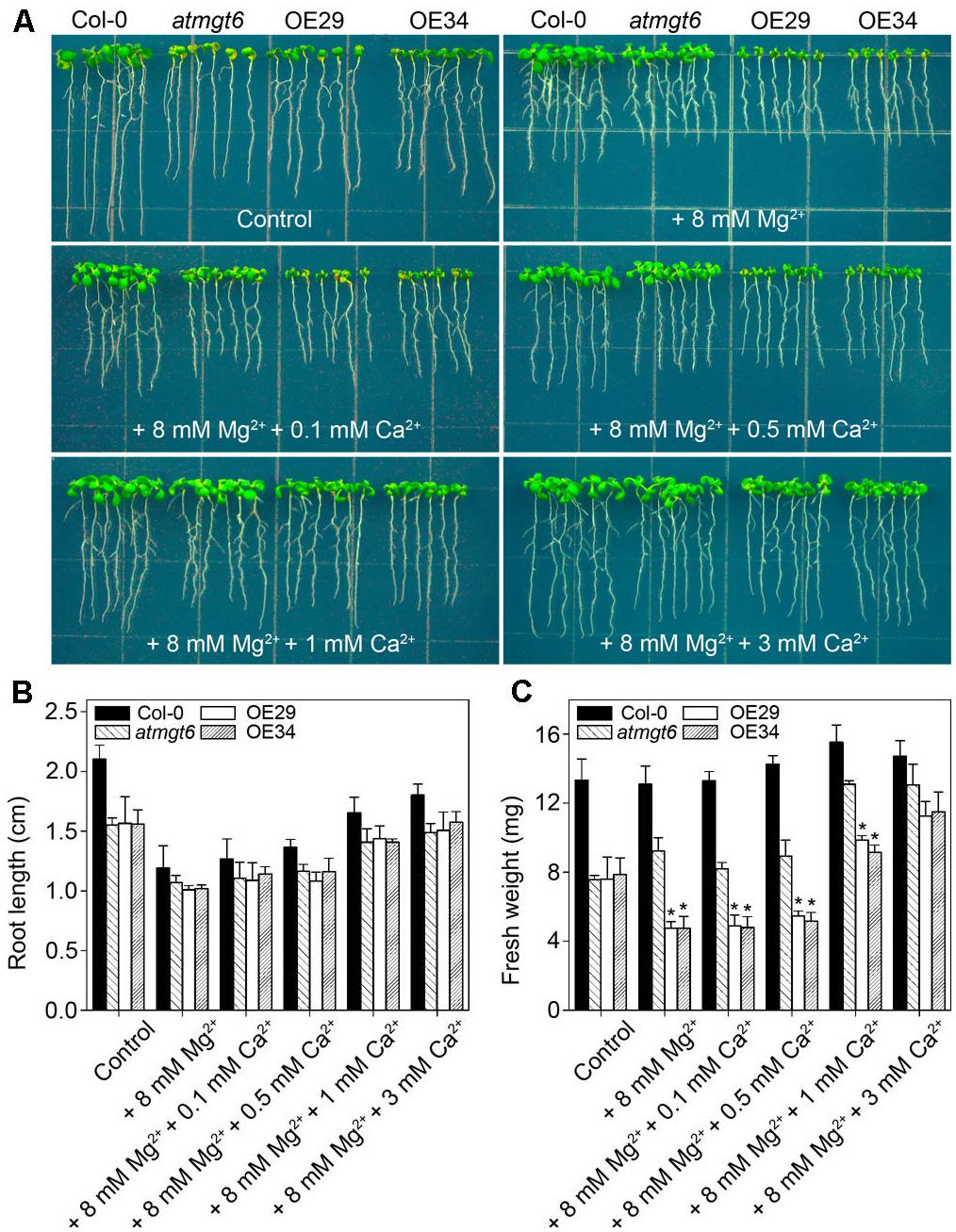
FIGURE 6. Effects of high Ca2+ additions on the growth of atmgt6 and transgenic OsHKT2;4-overexpression atmgt6 lines in the Mg2+-abundant medium. (A) The growth of Col-0, atmgt6 and two transgenic OsHKT2;4 overexpression atmgt6 lines (OE29 and OE34) in 1/6 MS (Control) and the Mg2+-abundant medium (1/6 MS with extra 8 mM Mg2+, referred to as “+8 mM Mg2+” in the figure) containing different extra Ca2+ concentrations. After planted in 1/2 MS medium for 3 days, Col-0, atmgt6, OE29, and OE34 were transferred to the 1/6 MS medium (containing a basal 0.25 mM Mg2+ and 0.5 mM Ca2+), or the Mg2+-abundant medium with 0, 0.1, 0.5, 1, or 3 mM extra Ca2+. Plants were photographed after growing for another 7 days. Quantitative analyses of primary root length (B) and whole-plant fresh weight (C) of Col-0, atmgt6, OE29, and OE34 under the conditions described in (A). Six independent 10-day-old seedlings of each genotype were gathered as one biological repeat for root length and fresh weight measurement. Data are represented as the mean ± SD, n = 3, in which n is the number of biological repeat. Asterisks indicate statistically significant differences compared with atmgt6 (Student’s t-test, ∗P < 0.05).
It has been reported that low Ca2+ in the medium triggered the increase of Mg2+ concentration, mimicking high-Mg2+ conditions (Rios et al., 2012). To analyze further whether Ca2+ deficiency is also responsible for the phenotype induced by high Mg2+, we thus tested the sensitivity among different plants to low-Ca2+ conditions. In Ca2+-depleted 1/6 MS medium (“basal medium” in Figure 7), similar phenotype was observed in atmgt6 and OE lines, suggesting that OsHKT2;4 is not responsible for Ca2+ deficiency. On the contrary, as the increasing content of extra Mg2+ (2, 4, 6, and 8 mM) was added to the basal medium, differences of fresh weight between atmgt6 and OE lines started to occur (Figure 7 and Supplementary Figure 3). These results demonstrated that high Mg2+, rather than Ca2+ deficiency, is the primary factor that caused growth defects in the OE lines than atmgt6.
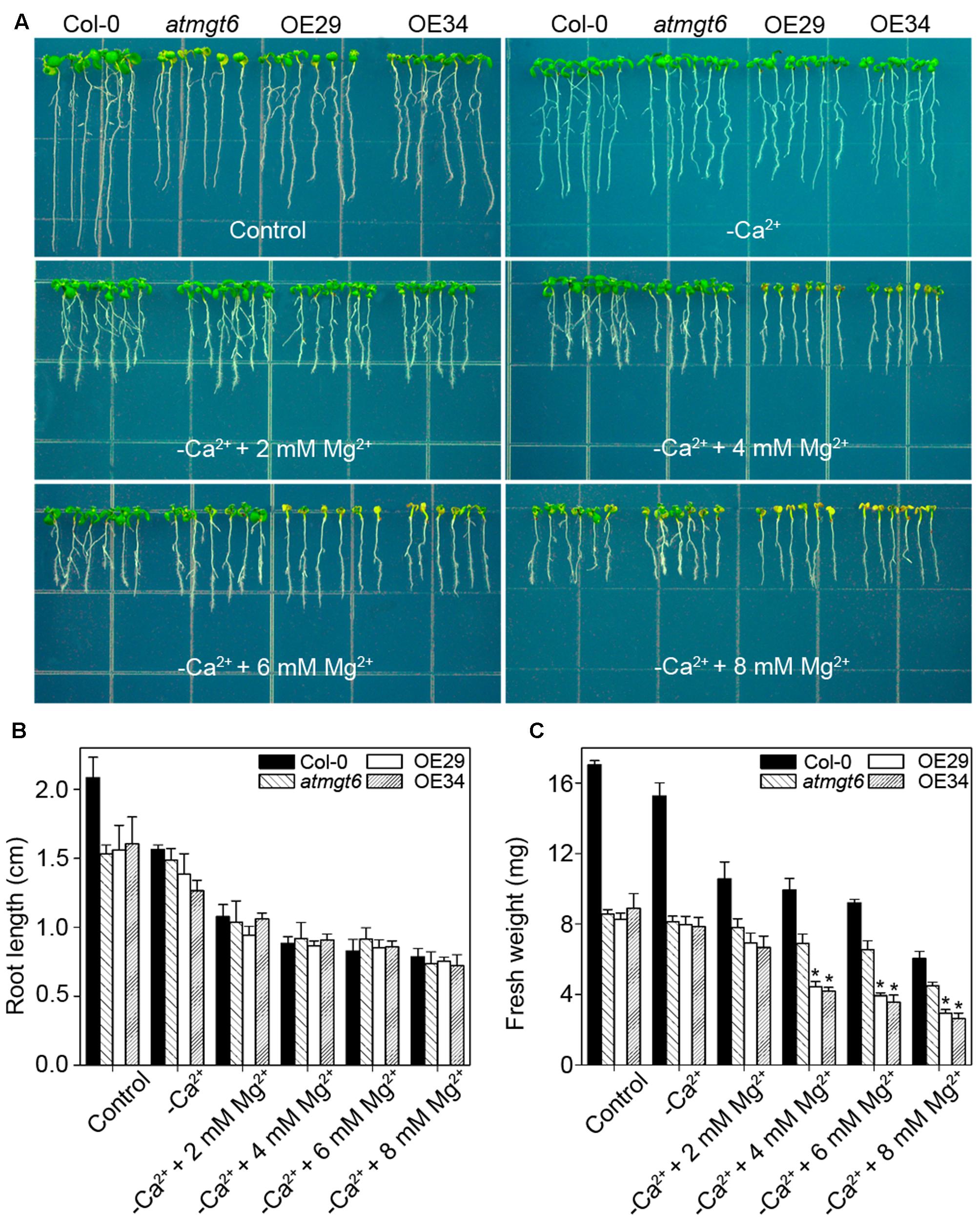
FIGURE 7. Effects of the Ca2+ depletion on the growth of atmgt6 and transgenic OsHKT2;4-overexpression atmgt6 lines in the Mg2+-abundant medium. (A) The growth of Col-0, atmgt6 and transgenic two OsHKT2;4 overexpression atmgt6 lines (OE29 and OE34) in 1/6 MS (Control) and the 1/6 MS medium depleted of Ca2+ (–Ca2+). After planted in 1/2 MS medium for 3 days, Col-0, atmgt6, OE29, and OE34 were transferred to the 1/6 MS medium (containing a basal 0.25 mM Mg2+ and 0.5 mM Ca2+), or the 1/6 MS medium depleted of Ca2+ and supplemented with extra 2, 4, 6, or 8 mM Mg2+. Plants were photographed after growing for another 7 days. Quantitative analyses of primary root length (B) and whole-plant fresh weight (C) of Col-0, atmgt6, OE29, and OE34 under the conditions described in (A). Six independent 10-day-old seedlings of each genotype were gathered as one biological repeat for root length and fresh weight measurement. Data are represented as the mean ± SD, n = 3, in which n is the number of biological repeat. Asterisks indicate statistically significant differences compared with atmgt6 (Student’s t-test, ∗P < 0.05).
Discussion
Mg2+ is an essential macronutrient for plant growth, development and reproductive success (Li et al., 2001; Hermans et al., 2013; Mao et al., 2014), while it could be detrimental at high concentrations (Visscher et al., 2010). Plants possess specific Mg2+ transport systems that can function under a wide range of concentrations to secure intracellular Mg2+ homeostasis. Despite several transporters have been shown to function in Mg2+ uptake and distribution in Arabidopsis, including the AtMGT/AtMRS2-type transporters (Li et al., 2001) and Mg2+/H+ antiporter AtMHX (Shaul et al., 1999), little is known about the transporters responsible for Mg2+ homeostasis in rice. OsHKT2;4 has been reported to function as a non-selective transporter for diverse cations, including Mg2+ and Ca2+. Our study here provided further evidence that OsHKT2;4 exhibits characteristics of low-affinity transport of Mg2+, and plays a key role in Mg2+ homeostasis for plant’s adaptation to high-Mg2+ conditions.
Rice contains up to nine HKT genes (depending on variety), and OsHKT2;4 is the member of class II HKTs with the conserved Gly residues at the four P-loop filter positions (Mäser et al., 2002b). OsHKT2;4 is localized at the plasma membrane of rice cells, and its exogenous expression caused X. laevis oocytes to produce large currents when the extracellular Mg2+ concentrations were at the range of millimolar levels (Lan et al., 2010; Horie et al., 2011). Triticum aestivum HKT2;1 (TaHKT2;1) was also found to result in robust Mg2+ permeability of the oocytes, although to a lesser degree (Horie et al., 2011). OsHKT2;4-mediated currents exhibited the shifts to positive reversal potentials upon increased Mg2+ concentration from 5 to 50 mM (Horie et al., 2011). The present study further analyzed the capability of Mg2+-uptake of OsHKT2;4 in three systems, the oocytes, bacteria, and Arabidopsis under the conditions containing high-Mg2+ concentrations. The current amplitudes and reversal potentials in the oocytes expressing OsHKT2;4 were not changed when the extracellular Mg2+ concentration was less than 1.2 mM, until its concentration reached 6 mM (Figure 2). Similarly, the expression of OsHKT2;4 rescued growth defects of MM281 bacteria cells that are deficient in Mg2+ uptake in the presence of relatively high-Mg2+ concentration, but the rescuing effect was much less than MGT10, the high-affinity Mg2+ transporter (Figure 1). Complementary to the observations in the oocytes and bacteria, the phenotype relating to Mg2+ stress in transgenic OsHKT2;4-overexpressed atmgt6 lines happened at the Mg2+-abundant (Figure 4), but not at Mg2+-deficient conditions (Figure 4). Taken together, our findings showed that OsHKT2;4 has a distinct low-affinity Mg2+ transportation, and confirm Mg2+ permeability of OsHKT2;4 as reported (Lan et al., 2010; Horie et al., 2011). It is worth mentioning that OsHKT2;4 was reported to be impermeable to Mg2+ when it was expressed in the oocytes by an independent study (Sassi et al., 2012). Due to the genetic diversity in rice during evolution and amino acid variation of HKTs among Oryza accessions (Horie et al., 2001; Ren et al., 2005), the differences might result from the sources of OsHKT2;4 from different rice varieties. In the previous studies (Lan et al., 2010; Horie et al., 2011) and the present study, genetically tractable rice (O. sativa; background Nipponbare) was used.
Mg2+ is taken up from the soil by the plant root system, which is likely to be mediated by AtMGT6/MRS2-4. AtMGT6/MRS2-4 is located in the plasma membrane or the endoplasmic reticulum and highly expressed in the root epidermal cells, and its disruption resulted in growth retardation of Arabidopsis under the low-Mg2+ condition (Mao et al., 2014; Oda et al., 2016). OsHKT2;4 might not be a key factor for roots to uptake Mg2+ from the low-Mg2+ environment as its overexpression did not cause the changed growth phenotype of transgenic lines (Figure 3), fitting the idea of its low-affinity Mg2+ transportation. After satisfying the needs of the roots, the rest of the Mg2+ will be transported to the shoot through the process involving AtMGT6/MRS2-4 activity (Oda et al., 2016). However, plants will display Mg2+ toxicity symptom when Mg2+ is over accumulated in the shoot. To deal with this toxicity, plants might restrain the Mg2+ distribution in shoot or sequester the excess intracellular Mg2+ into the vacuoles (Hermans et al., 2013; Tang et al., 2015). The OE lines had higher Mg2+ content in shoot compared with atmgt6 under both low-Mg2+ and normal conditions (Figure 5), indicating the expression level of OsHKT2;4 was high enough to drive transportation of Mg2+ from root to shoot. However, once the expression level of OsHKT2;4 was further enhanced under high-Mg2+ conditions (Supplementary Figure 5), the Mg2+ transportation to shoot was also strengthened, thus leading to an increased Mg2+ distribution ratio of shoot to root and enhanced sensitivities of OE lines to high-Mg2+ conditions. Therefore, we suggested that OsHKT2;4 plays a key role in Mg2+ homeostasis and might control the Mg2+ translocation between roots and shoots under the high-Mg2+ conditions.
Although roles of Ca2+ and Mg2+ are distinct in diverse physiological and biochemical processes, they may play an antagonistic function in plants. (Tang and Luan, 2017). For example, growth retardations induced by individual knockouts of genes in AtMRS2/AtMGT family under low Mg2+ could be ameliorated when Ca2+ concentrations were concomitantly lowered (Lenz et al., 2013). Mutation of AtCAX1, which encodes a vacuolar Ca2+/H+ exchanger, resulted in reduction of Ca2+ in the vacuole, thus leading to more Ca2+ retaining in the cytosol to counteract with excess Mg2+ (Cheng et al., 2003; Bradshaw, 2005). Consistently, our study demonstrated that addition of Ca2+ to the high-Mg2+ medium could partially rescue the Mg2+-induced growth defect of atmgt6 and the OsHKT2;4-overexpressed lines to a wild type level (Figure 6), which is also a supportive evidence for the antagonistic interaction between Ca2+ and Mg2+ in planta. However, Mg2+ currents through OsHKT2;4 in oocytes were not inhibited and their reversal potentials were not significantly shifted in the presence of 1.8 mM Ca2+ in the perfusion solution (Supplementary Figure 4), indicating that Ca2+ did not inhibit Mg2+ uptake in oocytes expressing OsHKT2;4. Thus, the effects of changes in Ca2+ additions on Mg2+ toxicity might result from the physiological antagonism between Ca2+ and Mg2+ in planta (Bradshaw, 2005; Tang et al., 2015), although further evidence is needed.
In our previous study, we found that OsHKT2;4 had the diverse expression pattern in rice plants, including leaves, stems and primary/lateral roots, and was highly expressed at xylem and phloem of epidermis (Lan et al., 2010). However, the homozygous lines of Tos-tagged oshkt2;4 rice lines behaved similarly to wild-type plants (Lan et al., 2010; Horie et al., 2011), and contained similar content of cations, including Mg2+ and Ca2+ (Lan et al., 2010). The absence of a phenotypic change in these rice lines suggested that OsHKT2;4 is functionally redundant with other transporters. Indeed, we found OsHKT2;4 rendered Arabidopsis Mg2+ sensitivity when atmgt6 was knockout (Figure 4). Rice (O. sativa; background Nipponbare) is predicted to have nine AtMGT orthologs based on the BLAST search (Gebert et al., 2009), and Os10g0545000, the closest one to AtMGT6, is also widely expressed in rice according to the microarray gene expression data collected by Genevestigator1 (Supplementary Figure 1). Elucidation of the functional relationships between MGT-type transporters and OsHKT2;4 will be a critical next step toward assessing their biological functions in rice.
Author Contributions
CZ, BZ, HL, JG, and WL designed the study. CZ, HL, BZ, and WL performed experiments. CZ, HL, JW, BZ, WW, JG, and WL analyzed and interpreted the data. CZ, HL, BZ, and WL wrote the manuscript. CZ, BZ, HL, SL, JG, and WL revised the manuscript critically. All authors read and approved the final manuscript.
Funding
This research was supported by the National Natural Science Foundation of China (31271626 and 31271682).
Conflict of Interest Statement
The authors declare that the research was conducted in the absence of any commercial or financial relationships that could be construed as a potential conflict of interest.
Acknowledgment
We thank Jiangsu Collaborative Innovation Center for Modern Crop Production for technical support.
Supplementary Material
The Supplementary Material for this article can be found online at: https://www.frontiersin.org/articles/10.3389/fpls.2017.01823/full#supplementary-material
Footnotes
References
Ariyarathna, H. A., Oldach, K. H., and Francki, M. G. (2016). A comparative gene analysis with rice identified orthologous group II HKT genes and their association with Na+ concentration in bread wheat. BMC Plant Biol. 16:21. doi: 10.1186/s12870-016-0714-7
Bose, J., Babourina, O., Shabala, S., and Rengel, Z. (2013). Low-pH and aluminum resistance in Arabidopsis correlates with high cytosolic magnesium content and increased magnesium uptake by plant roots. Plant Cell Physiol. 54, 1093–1104. doi: 10.1093/pcp/pct064
Bradshaw, H. D. Jr. (2005). Mutations in CAX1 produce phenotypes characteristic of plants tolerant to serpentine soils. New Phytol. 167, 81–88. doi: 10.1111/j.1469-8137.2005.01408.x
Chen, J., Li, L. G., Liu, Z. H., Yuan, Y. J., Guo, L. L., Mao, D. D., et al. (2009). Magnesium transporter AtMGT9 is essential for pollen development in Arabidopsis. Cell Res. 19, 887–898. doi: 10.1038/cr.2009.58
Cheng, N. H., Pittman, J. K., Barkla, B. J., Shigaki, T., and Hirschi, K. D. (2003). The Arabidopsis cax1 mutant exhibits impaired ion homeostasis, development, and hormonal responses and reveals interplay among vacuolar transporters. Plant Cell 15, 347–364. doi: 10.1105/tpc.007385
Chérel, I., Lefoulon, C., Boeglin, M., and Sentenac, H. (2014). Molecular mechanisms involved in plant adaptation to low K+ availability. J. Exp. Bot. 65, 833–848. doi: 10.1093/jxb/ert402
Clough, S. J., and Bent, A. F. (1998). Floral dip: a simplified method for Agrobacterium-mediated transformation of Arabidopsis thaliana. Plant J. 16, 735–743. doi: 10.1046/j.1365-313x.1998.00343.x
Corratgé-Faillie, C., Jabnoune, M., Zimmermann, S., Véry, A. A., Fizames, C., and Sentenac, H. (2010). Potassium and sodium transport in non-animal cells: the Trk/Ktr/HKT transporter family. Cel. Mol. Life Sci. 67, 2511–2532. doi: 10.1007/s00018-010-0317-7
Davenport, R. J., Munoz-Mayor, A., Jha, D., Essah, P. A., Rus, A., and Tester, M. (2007). The Na+ transporter AtHKT1;1 controls retrieval of Na+ from the xylem in Arabidopsis. Plant Cell Environ. 30, 497–507. doi: 10.1111/j.1365-3040.2007.01637.x
Garciadeblas, B., Senn, M. E., Banuelos, M. A., and Rodriguez-Navarro, A. (2003). Sodium transport and HKT transporters: the rice model. Plant J. 34, 788–801. doi: 10.1046/j.1365-313X.2003.01764.x
Gebert, M., Meschenmoser, K., Svidova, S., Weghuber, J., Schweyen, R., Eifler, K., et al. (2009). A root-expressed magnesium transporter of the MRS2/MGT gene family in Arabidopsis thaliana allows for growth in low-Mg2+ environments. Plant Cell 21, 4018–4030. doi: 10.1105/tpc.109.070557
Hauser, F., and Horie, T. (2010). A conserved primary salt tolerance mechanism mediated by HKT transporters: a mechanism for sodium exclusion and maintenance of high K+/Na+ ratio in leaves during salinity stress. Plant Cell Environ. 33, 552–565. doi: 10.1111/j.1365-3040.2009.02056.x
Hermans, C., Conn, S. J., Chen, J., Xiao, Q., and Verbruggen, N. (2013). An update on magnesium homeostasis mechanisms in plants. Metallomics 5, 1170–1183. doi: 10.1039/c3mt20223b
Hicks, D. B., Wang, Z., Wei, Y., Kent, R., Guffanti, A. A., Banciu, H., et al. (2003). A tenth atp gene and the conserved atpI gene of a Bacillus atp operon have a role in Mg2+ uptake. Proc. Natl. Acad. Sci. U.S.A. 100, 10213–10218. doi: 10.1073/pnas.1832982100
Hirsch, R. E., Lewis, B. D., Spalding, E. P., and Sussman, M. R. (1998). A role for the AKT1 potassium channel in plant nutrition. Science 280, 918–921. doi: 10.1126/science.280.5365.918
Horie, T., Brodsky, D. E., Costa, A., Kaneko, T., Lo Schiavo, F., Katsuhara, M., et al. (2011). K+ transport by the OsHKT2;4 transporter from rice with atypical Na+ transport properties and competition in permeation of K+ over Mg2+ and Ca2+ ions. Plant Physiol. 156, 1493–1507. doi: 10.1104/pp.110.168047
Horie, T., Costa, A., Kim, T. H., Han, M. J., Horie, R., Leung, H. Y., et al. (2007). Rice OsHKT2;1 transporter mediates large Na+ influx component into K+-starved roots for growth. EMBO J. 26, 3003–3014. doi: 10.1038/sj.emboj.7601732
Horie, T., Hauser, F., and Schroeder, J. I. (2009). HKT transporter-mediated salinity resistance mechanisms in Arabidopsis and monocot crop plants. Trends Plant Sci. 14, 660–668. doi: 10.1016/j.tplants.2009.08.009
Horie, T., Yoshida, K., Nakayama, H., Yamada, K., Oiki, S., and Shinmyo, A. (2001). Two types of HKT transporters with different properties of Na+ and K+ transport in Oryza sativa. Plant J. 27, 129–138. doi: 10.1046/j.1365-313x.2001.01077.x
Knoop, V., Groth-Malonek, M., Gebert, M., Eifler, K., and Weyand, K. (2005). Transport of magnesium and other divalent cations: evolution of the 2-TM-GxN proteins in the MIT superfamily. Mol. Genet. Genomics 274, 205–216. doi: 10.1007/s00438-005-0011-x
Lan, W. Z., Wang, W., Wang, S. M., Li, L. G., Buchanan, B. B., Lin, H. X., et al. (2010). A rice high-affinity potassium transporter (HKT) conceals a calcium-permeable cation channel. Proc. Natl. Acad. Sci. U.S.A. 107, 7089–7094. doi: 10.1073/pnas.1000698107
Larkin, R. M. (2016). Tetrapyrrole signaling in plants. Front. Plant Sci. 7:1586. doi: 10.3389/fpls.2016.01586
Lenz, H., Dombinov, V., Dreistein, J., Reinhard, M. R., Gebert, M., and Knoop, V. (2013). Magnesium deficiency phenotypes upon multiple knockout of Arabidopsis thaliana MRS2 clade B genes can be ameliorated by concomitantly reduced calcium supply. Plant Cell Physiol. 54, 1118–1131. doi: 10.1093/pcp/pct062
Li, L., Tutone, A. F., Drummond, R. S., Gardner, R. C., and Luan, S. (2001). A novel family of magnesium transport genes in Arabidopsis. Plant Cell 13, 2761–2775. doi: 10.1105/tpc.010352
Li, L. G., Sokolov, L. N., Yang, Y. H., Li, D. P., Ting, J., Pandy, G. K., et al. (2008). A mitochondrial magnesium transporter functions in Arabidopsis pollen development. Mol. Plant 1, 675–685. doi: 10.1093/mp/ssn031
Mao, D., Chen, J., Tian, L., Liu, Z., Yang, L., Tang, R., et al. (2014). Arabidopsis transporter MGT6 mediates magnesium uptake and is required for growth under magnesium limitation. Plant Cell 26, 2234–2248. doi: 10.1105/tpc.114.124628
Mao, D. D., Tian, L. F., Li, L. G., Chen, J., Deng, P. Y., Li, D. P., et al. (2008). AtMGT7: an Arabidopsis gene encoding a low-affinity magnesium transporter. J. Integr. Plant Biol. 50, 1530–1538. doi: 10.1111/j.1744-7909.2008.00770.x
Mäser, P., Eckelman, B., Vaidyanathan, R., Horie, T., Fairbairn, D. J., Kubo, M., et al. (2002a). Altered shoot/root Na+ distribution and bifurcating salt sensitivity in Arabidopsis by genetic disruption of the Na+ transporter AtHKT1. FEBS Lett. 531, 157–161. doi: 10.1016/S0014-5793(02)03488-9
Mäser, P., Hosoo, Y., Goshima, S., Horie, T., Eckelman, B., Yamada, K., et al. (2002b). Glycine residues in potassium channel-like selectivity filters determine potassium selectivity in four-loop-per-subunit HKT transporters from plants. Proc. Natl. Acad. Sci. U.S.A. 99, 6428–6433. doi: 10.1073/pnas.082123799
Miedema, H., Bothwell, J. H., Brownlee, C., and Davies, J. M. (2001). Calcium uptake by plant cells–channels and pumps acting in concert. Trends Plant Sci. 6, 514–519. doi: 10.1016/S1360-1385(01)02124-0
Møller, I. S., Gilliham, M., Jha, D., Mayo, G. M., Roy, S. J., Coates, J. C., et al. (2009). Shoot Na+ exclusion and increased salinity tolerance engineered by cell type-specific alteration of Na+ transport in Arabidopsis. Plant Cell 21, 2163–2178. doi: 10.1105/tpc.108.064568
Munns, R., and Tester, M. (2008). Mechanisms of salinity tolerance. Annu. Rev. Plant Biol. 59, 651–681. doi: 10.1146/annurev.arplant.59.032607.092911
Nieves-Cordones, M., Martinez, V., Benito, B., and Rubio, F. (2016). Comparison between Arabidopsis and rice for main pathways of K+ and Na+ uptake by roots. Front. Plant Sci. 7:992. doi: 10.3389/fpls.2016.00992
Oda, K., Kamiya, T., Shikanai, Y., Shigenobu, S., Yamaguchi, K., and Fujiwara, T. (2016). The Arabidopsis Mg transporter, MRS2-4, is essential for Mg homeostasis under both low and high Mg conditions. Plant Cell Physiol. 57, 754–763. doi: 10.1093/pcp/pcv196
Platten, J. D., Cotsaftis, O., Berthomieu, P., Bohnert, H., Davenport, R. J., Fairbairn, D. J., et al. (2006). Nomenclature for HKT transporters, key determinants of plant salinity tolerance. Trends Plant Sci. 11, 372–374. doi: 10.1016/j.tplants.2006.06.001
Ren, Z. H., Gao, J. P., Li, L. G., Cai, X. L., Huang, W., Chao, D. Y., et al. (2005). A rice quantitative trait locus for salt tolerance encodes a sodium transporter. Nat. Genet. 37, 1141–1146. doi: 10.1038/ng1643
Rios, J. J., Lochlainn, S. O., Devonshire, J., Graham, N. S., Hammond, J. P., King, G. J., et al. (2012). Distribution of calcium (Ca) and magnesium (Mg) in the leaves of Brassica rapa under varying exogenous Ca and Mg supply. Ann. Bot. 109, 1081–1089. doi: 10.1093/aob/mcs029
Rodriguez-Navarro, A. (2000). Potassium transport in fungi and plants. Biochim. Biophys. Acta. 1469, 1–30. doi: 10.1016/S0304-4157(99)00013-1
Sassi, A., Mieulet, D., Khan, I., Moreau, B., Gaillard, I., Sentenac, H., et al. (2012). The rice monovalent cation transporter OsHKT2;4: revisited ionic selectivity. Plant Physiol. 160, 498–510. doi: 10.1104/pp.112.194936
Shaul, O. (2002). Magnesium transport and function in plants: the tip of the iceberg. Biometals 15, 307–321. doi: 10.1023/A:1016091118585
Shaul, O., Hilgemann, D. W., de-Almeida-Engler, J., Van Montagu, M., Inz, D., and Galili, G. (1999). Cloning and characterization of a novel Mg2+/H+ exchanger. EMBO J. 18, 3973–3980. doi: 10.1093/emboj/18.14.3973
Tang, R. J., and Luan, S. (2017). Regulation of calcium and magnesium homeostasis in plants: from transporters to signaling network. Curr. Opin. Plant Biol. 39, 97–105. doi: 10.1016/j.pbi.2017.06.009
Tang, R. J., Zhao, F. G., Garcia, V. J., Kleist, T. J., Yang, L., Zhang, H. X., et al. (2015). Tonoplast CBL-CIPK calcium signaling network regulates magnesium homeostasis in Arabidopsis. Proc. Natl. Acad. Sci. U.S.A. 112, 3134–3139. doi: 10.1073/pnas.1420944112
Townsend, D. E., Esenwine, A. J., Rd, G. J., Bross, D., Maguire, M. E., and Smith, R. L. (1995). Cloning of the mgtE Mg2+ transporter from Providencia stuartii and the distribution of mgtE in gram-negative and gram-positive bacteria. J. Bacteriol. 177, 5350–5354. doi: 10.1128/jb.177.18.5350-5354.1995
Uozumi, N., Rubio, F., Kim, E. J., Yamaguchi, T., Muto, S., Tsuboi, A., et al. (2000). The Arabidopsis HKT1 gene homolog mediates inward Na+ currents in Xenopus laevis oocytes and Na+ uptake in Saccharomyces cerevisiae. Plant Physiol. 122, 1249–1259. doi: 10.1104/pp.122.4.1249
Visscher, A. M., Paul, A. L., Kirst, M., Guy, C. L., Schuerger, A. C., and Ferl, R. J. (2010). Growth performance and root transcriptome remodeling of Arabidopsis in response to Mars-like levels of magnesium sulfate. PLOS ONE 5:e12348. doi: 10.1371/journal.pone.0012348
Yamaguchi, T., Hamamoto, S., and Uozumi, N. (2013). Sodium transport system in plant cells. Front. Plant Sci. 4:410. doi: 10.3389/fpls.2013.00410
Yao, X., Horie, T., Xue, S., Leung, H. Y., Katsuhara, M., Brodsky, D. E., et al. (2010). Differential sodium and potassium transport selectivities of the rice OsHKT2;1 and OsHKT2;2 transporters in plant cells. Plant Physiol. 152, 341–355. doi: 10.1104/pp.109.145722
Keywords: Arabidopsis, HKT transporter, MGT transporter, Mg2+ permeable, rice
Citation: Zhang C, Li H, Wang J, Zhang B, Wang W, Lin H, Luan S, Gao J and Lan W (2017) The Rice High-Affinity K+ Transporter OsHKT2;4 Mediates Mg2+ Homeostasis under High-Mg2+ Conditions in Transgenic Arabidopsis. Front. Plant Sci. 8:1823. doi: 10.3389/fpls.2017.01823
Received: 27 July 2017; Accepted: 10 October 2017;
Published: 24 October 2017.
Edited by:
Kai He, Lanzhou University, ChinaReviewed by:
Kendal Hirschi, Baylor College of Medicine, United StatesFouad Lemtiri-Chlieh, King Abdullah University of Science and Technology, Saudi Arabia
Tamara Pecenkova, Institute of Experimental Botany (ASCR), Czechia
Copyright © 2017 Zhang, Li, Wang, Zhang, Wang, Lin, Luan, Gao and Lan. This is an open-access article distributed under the terms of the Creative Commons Attribution License (CC BY). The use, distribution or reproduction in other forums is permitted, provided the original author(s) or licensor are credited and that the original publication in this journal is cited, in accordance with accepted academic practice. No use, distribution or reproduction is permitted which does not comply with these terms.
*Correspondence: Jiping Gao, anBnYW9Ac2licy5hYy5jbg== Wenzhi Lan, bGFud0BuanUuZWR1LmNu