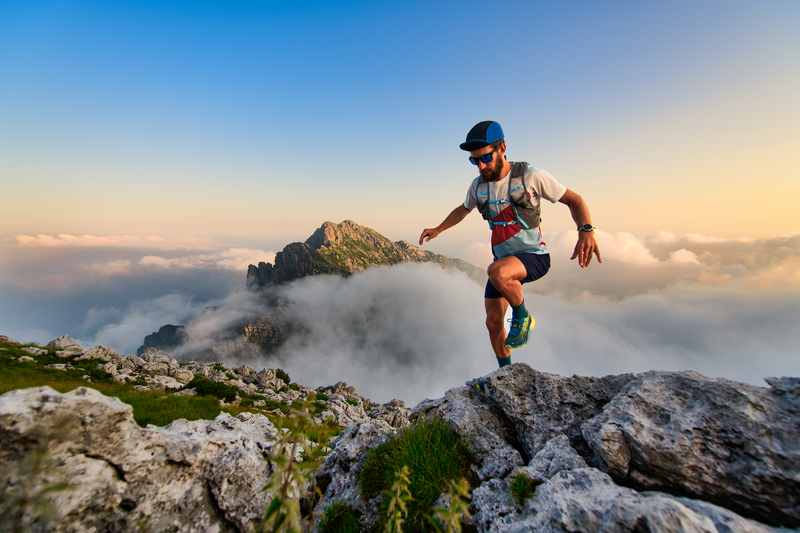
95% of researchers rate our articles as excellent or good
Learn more about the work of our research integrity team to safeguard the quality of each article we publish.
Find out more
OPINION article
Front. Plant Sci. , 12 September 2017
Sec. Plant Breeding
Volume 8 - 2017 | https://doi.org/10.3389/fpls.2017.01587
The future of space missions and extended human presence in space requires the ability to provide proper dietary intake for space travelers with minimal resupply from the Earth, as food and food packaging currently represent a significant burden on space mission consumables (Perchonok et al., 2012). This is critical for sustaining an optimal nutritional status for space travelers and for mitigating stress effects from long-duration space travel, including weight loss, hematological changes, and space radiation-induced oxidative cytotoxic stress, protein oxidation, increased muscle proteolysis, impairment of eye health and changes in the central nervous system (Kennedy et al., 2007; Vergari et al., 2010; Cohu et al., 2014). Such effects are further linked to emotional volatility, psychological stress, and depression among the crew (Rabin et al., 2005). Prevention of deleterious phenomena that accelerate tissue lethality must include targeted intake of whole food-based antioxidants rather than supplements (Wan et al., 2006). These comprise fresh plant sources produced aboard during mission, thereby providing emotional along with nutritional support to space travelers.
For instance, the consumption of carotenoids through whole-food-based diet is a recommendable protective measure since the human body is unable to produce any of the major photoprotective carotenoids considered essential for human vision: β-carotene as precursor of retinal constituent vitamin A, and zeaxanthin and lutein for protecting the eyes by absorbing excess light intensity (Cohu et al., 2014). Production of bioactive and particularly carotenoid-rich vegetables as part of Space Life Support Systems (SSLSs) remains a critical goal for future space missions (Perchonok et al., 2012). Awareness of the importance of fresh functional food in physically and mentally fortifying crews during missions has been growing among space mission participants (Vergari et al., 2010). It is therefore critical to incorporate in SLSSs plant-based fresh functional food production to support human presence during long-distance space travel or extra-planetary habitation.
Plant growth under space conditions is faced with important constraints unknown to Earth-based farming systems and beyond our current understanding of plant physiological responses in terrestrial environments. These include, among others, exposure to high levels of cosmic radiation, lack of a unilateral gravity vector, growth adapted to limited chamber space, reduced nutrient sustainability, and lack of convection (Vandenrbrink and Kiss, 2016). Space farming will be conducted in controlled environment chambers that must infringe minimally on power, volume, and mass resources shared with crews and used for maintaining spacecraft in stable orbit (Poulet et al., 2016). In bioregenerative closed systems, plants are destined to produce food, regenerate the air by removing CO2 and producing O2, and recycle water through transpiration. A major challenge for adapting Earth-based agricultural practices aboard spacecraft or in planetary bases is reduced gravity (or microgravity) that impacts fluid and gas distribution around the plants (Kuang et al., 2000). Reduced mass transport and absence of buoyancy-dependent convective transport are responsible for thick boundary layers forming around plant organs, allowing the build-up of volatile compounds deleterious for plant growth, such as ethylene, and reducing oxygen bioavailability (Monje et al., 2003). Power-assisted forced convection is required as reduced gravity-mediated oxygen transport may result in hypoxic conditions around plant organs whenever the rate of respiration exceeds the rate of oxygen mass transfer to the respiring organs; moreover, transpiration rates tend to increase under hypobaric conditions. Low irradiance levels (≤300 μmol m−2 s−1) is also among the serious constraints imposed on space farming as supplemental lighting is considered a highly energy demanding subsystem of the space farm (Salisbury and Bugbee, 1988).
Gravity is not an absolute requirement for any step in the plant life cycle as complete ontogenesis from seed to seed has been demonstrated in microgravity conditions (Kuang et al., 2000). Tomato seeds formed under simulated microgravity were biologically and functionally complete (Colla et al., 2007). However, seeds formed in space demonstrated retarded deposition of reserves during development (Musgrave et al., 2005), while reduced storage reserve mobilization and hypoxia-induced changes in mitochondrial size and shape, and in starch grain size and distribution was reported during Brassica early seedling growth along (Musgrave, 2002). Seeds of Brassica rapa L. produced in space were smaller, had lower dry weight, were deficient in protein and presented differences in bioactive phytochemicals, such as glucosinolates, compared to ground control seeds (Musgrave et al., 2005). Moreover, morphological and growth characteristics of dwarf tomato plants were modified during microgravity simulation treatment, presenting spreading growth, increased internode length, reduced fruit yield, fruit size, leaf area, leaf dry weight, fruit dry weight, total dry weight, foliar amounts of chlorophylls and carotenoids as well as reduced fruit sugar and dry mater contents (Colla et al., 2007).
The choice of crops may partly hold the answer to several of the challenges facing seed-to-seed production in microgravity. Crop criteria established for plants grown in space include: the ratio of edible mass to total biomass (harvest index), crop efficiency (per unit area, time, and volume), potential yield (edible mass and O2 and H2O production), and the crop's horticultural requirements (planting, harvesting, pollination, processing needs; Berkovich et al., 2004; Yamashita et al., 2009). Salad crops present the highest harvest indices (≈90%) among candidate crops, and low water uptake/transpiration ratio which translates into high humidity input into the space flight environment that can be harnessed, but they cannot be part of a closed system using recycled gray water (Anderson et al., 2015); moreover, they are characterized by low O2 production and CO2 consumption rates, i.e., low biomass fixation. Salad crops are highly suitable for chamber cultivation, they are easy to cultivate, they have short growth cycles, they are low ethylene producers and can be picked and eaten fresh, requiring minimal horticultural input from the crew (Chunxiao and Hong, 2008). Moreover, growing salad crops is easily adaptable to the needs of a diverse and renewed diet while adding a palatable and bioactive aspect to it.
A new class of speciality salad crops valued for their color and flavor enhancing properties but also for their rich phytonutrient content are microgreens (Kyriacou et al., 2016; Bulgari et al., 2017). Produced from the seeds of vegetables, herbs, or grains, including wild or even ornamental species, microgreens have a brief, species-dependent production cycle, of 1–3 weeks from seed germination (Xiao et al., 2012). They are harvested at soil level, when cotyledons are fully expanded and the first pair of true leaves has emerged (Sun et al., 2013). They have recently gained immense popularity as culinary ingredients of novel gastronomic trends (Koppertcress, 2016). Candidate genotypes are expanding based on sensory and health criteria, however, currently exploited are mostly species from the Brassicaceae, Asteraceae, Chenopodiaceae, Lamiaceae, Apiaceae, Amarillydaceae, Amaranthceae, and Cucurbitaceae families (Ebert, 2012). Compared to their mature-leaf counterparts, microgreens contain higher amounts of important phytonutrients (ascorbic acid, β-carotene, α-tocopherol, and phylloquinone) and minerals (Ca, Mg, Fe, Mn, Zn, Se, and Mo) and lower nitrates (Xiao et al., 2012; Pinto et al., 2015). Seeds are demanded in large quantity and high quality, thus constitute a major cost of microgreens production (Di Gioia et al., 2015). Although, foodborne outbreaks have not been associated with the consumption of microgreens, seeds should receive precautionary sanitary treatments for eliminating pathogenic bacteria (Xiao et al., 2014, 2015). Ease of germination varies among microgreens species, with slow germinating species benefiting from brief pre-sowing treatments that help standardize and shorten their production cycle (Lee et al., 2004). Sowing rate depends on average seed weight, estimated germinability and targeted crop density, ranging from 1 seed/cm2 for large-seeded species (e.g., pea, chickpea, sunflower), up to 4 seeds/cm2 for small-seeded species (e.g., arugula, watercress, mustard; Di Gioia and Santamaria, 2015). Microgreens are ideal for space flight environments as they can be harvested directly by crew members, ensuring freshness and high quality. Their production can be implemented on static, shallow substrates with little or no nutrient supplementation, and this alleviates problems of poor crop performance associated with low O2 and nutrient solubility in microgravity hydroponic systems (Perchonok et al., 2012). Synthetic fibrous media can be used and fortified to improve the nutritional value of microgreens (Nyenhuis and Drelich, 2015), furthermore they eliminate the need to prepare and administer complete nutrient solutions and allows transpired water to be recycled to the root module. Bioactive content is usually pronounced in less palatable microgreens species, such as red cabbage (Brassica oleracea L. var. capitata), sorrel (Rumex acetosa L.), peppercress (Lepidium bonariense L.), but also in some species of more agreeable taste such as cilantro (Coriandrum sativum L.) and amaranth (Amaranthus hypochondriacus L.; Xiao et al., 2012).
A major constraint of food production in SLSSs is the high demand for power which antagonizes other space shuttle subsystems (Perchonok et al., 2012). Providing efficient and optimal, in terms of intensity and spectral quality, lighting for crops in spaceflight environments has been increasingly feasible through the introduction of light-emitting diode (LED) technology (Wheeler, 2004; Bourget, 2008). Using LEDs instead of metal halide, fluorescent, incandescent, and high-pressure sodium lamps can reduce power demand per unit of growing area by up to one order of magnitude (Poulet et al., 2014, 2016). Microgreens have a lower demand for photon flux compared to long-cycle crops, thus are ideally adapted to chamber environments. Moreover, modulating the photon flux, photoperiod and especially the wavelength can be an effective means of achieving compound-specific improvements in the functional quality of microgreens and decrease in the levels of anti-nutrients (Kyriacou et al., 2016). For example, supplemental green light improved carotenoid content (β-carotene and lutein/zeaxanthin ratio) in mustard microgreens, while standard blue/red/far red LED illumination increased the levels of carotenoids in red pak and tatsoi (Brazaityté et al., 2015). Even brief (3 d) preharvest application of supplementary red LED was efficacious in improving the antioxidant profiles of several microgreens species (Samuolienė et al., 2012). Red and blue lights, or their mixture, were found more effective than white and yellow in reducing undesirable nitrates in several species (Ohashi-Kaneko et al., 2007; Qi et al., 2007). However, the exact mechanisms behind spectral quality-induced changes on bioactive compounds are far from elucidated and deserve further attention.
In the near future, space exploration will inevitably expand and its food supply system must also continue to evolve. In this perspective, microgreens could be considered a resilient phytochemical factory for the dietary and psychological needs of crew members in orbital flights and platforms. However, as components of bioregenerative life support systems, plants must provide food to the astronauts as well as sufficient photosynthetic CO2 fixation, O2 regeneration and transpirational filtering, and recycling of water. Despite their high harvest index, microgreens are characterized by low biomass fixation and consequently low O2 generation. To a certain extent, this can be maximized by growing microgreens in multi-tiered systems, thereby also increasing space efficiency and water transpiration. Moreover, the need for higher O2 generation can be met by growing microgreens along with larger edible crops characterized by higher biomass fixation but, usually, lower harvest index. Microgreens also provide an easy platform for widening space crop genetic diversity since the diverse cultural needs of long-cycle crops are avoided. Such broad genetic basis is desirable both from a nutritional and sensorial standpoint but also as a means of fending off SLS systems from potential plant disease outbreaks. Although, growing microgreens on artificial media is usually problem-free, food safety, and healthy crop stand necessitate appropriate precautionary measures such as using only high quality certified seed produced and handled under conditions that minimize potential contamination with pathogenic organisms, sanitary maintenance of facilities employed for growing microgreens, pre-sowing treatment of seed with appropriate sanitizers such as calcium hypochlorite, testing, and effective disinfection of irrigation water. Finally, an important factor that warrants further research is the effect of space radiation on seed germinability. Although, this is a horizontal factor influencing both seed-to-seed and short-cycle crops, ensuring seed viability and germinability is even more critical for high seed rate crops such as microgreens.
MK and YR: Had the original idea; MK and YR: Prolonged presence in space and the need for a functional diet; MK, YR, SD: Space farm essentials and constraints; All authors: Ideal candidates for the astronaut's functional salad; All authors: Challenges ahead.
The authors declare that the research was conducted in the absence of any commercial or financial relationships that could be construed as a potential conflict of interest.
Anderson, M. S., Ewert, M. K., Keener, J. F., and Wagner, S. A. (2015). Life Support Baseline Values and Assumptions Document. Available online at https://ntrs.nasa.gov/archive/nasa/casi.ntrs.nasa.gov/20150002905.pdf
Berkovich, Y. A., Chetirkin, P. V., Wheeler, R. M., and Sager, J. C. (2004). Evaluating and optimizing horticultural regimes in space plant growth facilities. Adv. Space Res. 34, 1612–1618. doi: 10.1016/j.asr.2003.08.080
Brazaityté, A., Sakalauskiené, S., Samuoliené, G., Jankauskiené, J., Viršilé, A., Novičkovas, A., et al. (2015). The effects of LED illumination spectra and intensity on carotenoid content in Brassicaceae microgreens. Food Chem. 173, 600–606. doi: 10.1016/j.foodchem.2014.10.077
Bulgari, R., Baldi, A., Ferrante, A., and Lenzi, A. (2017). Yield and quality of basil, Swiss chard, and rocket microgreens grown in a hydroponic system. New Zeal. J. Crop Hortic. Sci. 45, 119–129. doi: 10.1080/01140671.2016.1259642
Chunxiao, X., and Hong, L. (2008). Crop candidates for the bioregenerative life support systems in China. Acta Astron. 63, 1076–1080. doi: 10.1016/j.actaastro.2008.02.003
Cohu, C. M., Lombardi, E., Adams, W. W. III, and Demming-Adams, B. (2014). Increased nutritional quality of plants for long-duration spaceflight missions through choice of plant variety and manipulation of growth conditions. Acta Astonaut. 94, 799–806. doi: 10.1016/j.actaastro.2013.10.009
Colla, G., Rouphael, Y., Cardarelli, M., Mazzucato, A., and Olimpieri, I. (2007). Growth, yield and reproduction of dwarf tomato grown under simulated microgravity conditions. Plant Biosyst. 141, 75–81. doi: 10.1080/11263500601153735
Di Gioia, F., Mininni, C., and Santamaria, P. (2015). “How to grow microgreens,” in Microgreens: Novel Fresh and Functional Food to Explore all the Value of Biodiversity, eds F. Di Gioia and P. Santamaria (Bari: ECO-logica srl), 51–79.
Di Gioia, F., and Santamaria, P. (eds.). (2015). “Microgreens: Microgreens,” in Novel Fresh and Functional Food to Explore all the Value of Biodiversity (Bari: ECO-logica srl), 118.
Ebert, A. (2012). “Sprouts, microgreens, and edible flowers: the potential for high value specialty produce in Asia,” in High Value Vegetables in Southeast Asia: Production, Supply and Demand, Proceedings SEAVEG 2012 (Chiang Mai: Asian Vegetable Research and Development Center), 216–227.
Kennedy, A. R., Guan, J., and Ware, J. H. (2007). Countermeasures against space radiation induced oxidative stress in mice. Radiat. Environ. Biophys. 46, 201–203. doi: 10.1007/s00411-007-0105-4
Koppertcress. (2016). The Standards of a Fair and Objective Review. Available online at http://usa.koppertcress.com (Accessed July 06, 2017).
Kuang, A., Xiao, Y., McClure, G., and Musgrave, M. E. (2000). Influence of microgravity on ultrastructure and storage reserves in seeds of Brassica rapa L. Ann. Bot. 85, 851–859. doi: 10.1006/anbo.2000.1153
Kyriacou, M. C., Rouphael, Y., Di Gioia, F., Kyratzis, A., Serio, F., Renna, M., et al. (2016). Micro-scale vegetable production and the rise of microgreens. Trends Food Sci. Technol. 57, 103–115. doi: 10.1016/j.tifs.2016.09.005
Lee, J. S., Pill, W. G., Cobb, B. B., and Olszewski, M. (2004). Seed treatments to advance greenhouse establishment of beet and chard microgreens. J. Hortic. Sci. Biotech. 79, 565–570. doi: 10.1080/14620316.2004.11511806
Monje, O., Stutte, G. W., Goins, G. D., Porterfield, D. M., and Bingham, G. E. (2003). Farming in space: environmental and biophysical concerns. Adv. Space Res. 31, 151–167. doi: 10.1016/S0273-1177(02)00751-2
Musgrave, M. E., Kuang, A., Tuominen, L. K., Levine, L. H., and Morrow, R. C. (2005). Seed storage reserves and glucosinolates in Brassica rapa L. grown on the international space station. J. Am. Soc. Hortic. Sci. 130, 848–856.
Nyenhuis, J., and Drelich, J. W. (2015). Essential micronutrient biofortification of sprouts grown on mineral fortified fiber mats. Intern. Schol. Sci. Res. Innov. 9, 981–984.
Ohashi-Kaneko, K., Takase, M., Kon, N., Fujiwara, K., and Kurata, K. (2007). Effect of light quality on growth and vegetable quality in leaf lettuce, spinach and komatsuna. Environ. Cont. Biol. 45, 189–198. doi: 10.2525/ecb.45.189
Perchonok, M. H., Cooper, M. R., and Catauro, P. M. (2012). Mission to mars: food production and processing for the final frontier. Ann. Rev. Food Sci. Technol. 3, 311–330. doi: 10.1146/annurev-food-022811-101222
Pinto, E., Almeida, A. A., Aguiar, A. A., and Ferreira, I. M. P. L. V. O. (2015). Comparison between the mineral profile and nitrate content of microgreens and mature lettuces. J. Food Comp. Anal. 37, 38–43. doi: 10.1016/j.jfca.2014.06.018
Poulet, L., Fontaine, J. P., and Dussap, C. G. (2016). Plant's response to space environment: a comprehensive review including mechanistic modelling for future space gardener. Bot. Lett. 163, 337–347. doi: 10.1080/23818107.2016.1194228
Poulet, L., Massa, G. D., Morrow, R. C., Bourget, C. M., Wheeler, R. M., and Mitchell, C. A. (2014). Significant reduction in energy for plant-growth lighting in space using targeted LED lighting and spectral manipulation. Life Sci. Space Res. 2, 43–53. doi: 10.1016/j.lssr.2014.06.002
Qi, L. D., Liu, S. H. Q., Xu, L., Yu, W. Y., Lang, Q. L., and Hao, S. H. Q. (2007). Effects of light qualies on accumulation of oxalate, tannin and nitrate in spinach. Trans. Chin. Soc. Agric. Eng. 4, 201–205. doi: 10.3969/j.issn.1002-6819.2007.4.040
Rabin, B. M., Shukitt Hale, B., Joseph, J., and Todd, P. (2005). Diet as a factor in behavioral radiation protection following exposure to heavy particles. Gravit. Space Biol. Bull. 18, 71–77.
Salisbury, F. B., and Bugbee, B. (1988). Plant productivity in controlled environments. Hortscience 23, 293–299.
Samuolienė, G., Brazaitytė, A., Sirtautas, R., Sakalauskienė, S., Jankauskienė, J., Duchovskis, P., et al. (2012). The impact of supplementary short-term red led lighting on the antioxidant properties of microgreens. Acta Hortic. 956, 649–655. doi: 10.17660/ActaHortic.2012.956.78
Sun, J., Xiao, Z., Lin, L. Z., Lester, G. E., Wang, Q., Harnly, J. M., et al. (2013). Profiling polyphenols in five Brassica species microgreens by UHPLC-PDA-ESI/HRMSn. J. Agric. Food Chem. 61, 10960–10970. doi: 10.1021/jf401802n
Vandenrbrink, J. P., and Kiss, J. Z. (2016). Space, the final frontier: a critical review of recent experiments performed in microgravity. Plant Sci. 243, 115–119. doi: 10.1016/j.plantsci.2015.11.004
Vergari, F., Tibuzzi, A., and Basile, G. (2010). “An overview of the functional food market: from marketing issues and commercial players to future demand from life in space,” in Bio-Farms for Nutraceuticals eds M. T. Giardi, G. Rea, and B. Berra (Boston, MA: Springer), 308–321.
Wan, X. S., Ware, J. H., Zhou, Z., Donahue, J. J., Guan, J., and Kennedy, A. R. (2006). Protection against radiation induced oxidative stress in cultured human epithelial cells by treatment with antioxidant agents. Int. J. Radiat. Oncol. Biol. Phys. 64, 1475–1481. doi: 10.1016/j.ijrobp.2005.11.024
Wheeler, R. M. (2004). Horticulture for mars. Acta Hortic. 642, 201–215. doi: 10.17660/ActaHortic.2004.642.22
Xiao, Z., Bauchan, G., Nichols-Russell, L., Luo, Y., Wang, Q., and Nou, X. (2015). Proliferation of Escherichia coli O157:H7 in soil-substitute and hydroponic microgreen production systems. J. Food Prot. 78, 1785–1790. doi: 10.4315/0362-028X.JFP-15-063
Xiao, Z., Lester, G. E., Luo, Y., and Wang, Q. (2012). Assessment of vitamin and carotenoid concentrations of emerging food products: Edible microgreens. J. Agric. Food Chem. 60, 7644–7651. doi: 10.1021/jf300459b
Xiao, Z., Nou, X., Luo, Y., and Wang, Q. (2014). Comparison of the growth of Escherichia coli O157: H7 and O104: H4 during sprouting and microgreen production from contaminated radish seeds. Food m44, 60–63. doi: 10.1016/j.fm.2014.05.015
Keywords: carotenoids, functional salad, light-emitting diodes (LED), microgravity, space farm
Citation: Kyriacou MC, De Pascale S, Kyratzis A and Rouphael Y (2017) Microgreens as a Component of Space Life Support Systems: A Cornucopia of Functional Food. Front. Plant Sci. 8:1587. doi: 10.3389/fpls.2017.01587
Received: 12 July 2017; Accepted: 29 August 2017;
Published: 12 September 2017.
Edited by:
Marcello Mastrorilli, CREA, ItalyReviewed by:
Yuksel Tuzel, Ege University, TurkeyCopyright © 2017 Kyriacou, De Pascale, Kyratzis and Rouphael. This is an open-access article distributed under the terms of the Creative Commons Attribution License (CC BY). The use, distribution or reproduction in other forums is permitted, provided the original author(s) or licensor are credited and that the original publication in this journal is cited, in accordance with accepted academic practice. No use, distribution or reproduction is permitted which does not comply with these terms.
*Correspondence: Youssef Rouphael, eW91c3NlZi5yb3VwaGFlbEB1bmluYS5pdA==
Disclaimer: All claims expressed in this article are solely those of the authors and do not necessarily represent those of their affiliated organizations, or those of the publisher, the editors and the reviewers. Any product that may be evaluated in this article or claim that may be made by its manufacturer is not guaranteed or endorsed by the publisher.
Research integrity at Frontiers
Learn more about the work of our research integrity team to safeguard the quality of each article we publish.