- 1Agricultural Institute, Centre for Agricultural Research, Hungarian Academy of Sciences, Martonvásár, Hungary
- 2Department of Plant Science, Rothamsted Research, Harpenden, United Kingdom
- 3Institute of Experimental Botany, Centre of the Region Haná for Biotechnological and Agricultural Research, Olomouc, Czechia
Cereal grain fiber is an important health-promoting component in the human diet. One option to improve dietary fiber content and composition in wheat is to introduce genes from its wild relatives Aegilops biuncialis and Aegilops geniculata. This study showed that the addition of chromosomes 2Ug, 4Ug, 5Ug, 7Ug, 2Mg, 5Mg, and 7Mg of Ae. geniculata and 3Ub, 2Mb, 3Mb, and 7Mb of Ae. biuncialis into bread wheat increased the seed protein content. Chromosomes 1Ug and 1Mg increased the proportion of polymeric glutenin proteins, while the addition of chromosomes 1Ub and 6Ub led to its decrease. Both Aegilops species had higher proportions of β-glucan compared to arabinoxylan (AX) than wheat lines, and elevated β-glucan content was also observed in wheat chromosome addition lines 5U, 7U, and 7M. The AX content in wheat was increased by the addition of chromosomes 5Ug, 7Ug, and 1Ub while water-soluble AX was increased by the addition of chromosomes 5U, 5M, and 7M, and to a lesser extent by chromosomes 3, 4, 6Ug, and 2Mb. Chromosomes 5Ug and 7Mb also affected the structure of wheat AX, as shown by the pattern of oligosaccharides released by digestion with endoxylanase. These results will help to map genomic regions responsible for edible fiber content in Aegilops and will contribute to the efficient transfer of wild alleles in introgression breeding programs to obtain wheat varieties with improved health benefits.
Key Message: Addition of Aegilops U- and M-genome chromosomes 5 and 7 improves seed protein and fiber content and composition in wheat.
Introduction
Because of its central role in the human diet, wheat is one of the major sources of dietary fiber (DF). The major DF components in wheat grain are the cell wall polysaccharides, arabinoxylan (AX) and (1-3)(1-4)-β-D-glucan (β-glucan), which account for about 70 and 20%, respectively, of the total cell wall polysaccharides in the starchy endosperm (and hence white flour) (Mares and Stone, 1973). AX and β-glucan occur in soluble and insoluble forms, which may differ in their health benefits. Insoluble DF lowers transit time and increases fecal bulk, defecation frequency, and the binding of carcinogens, while soluble DF reduces the risk of coronary heart disease and type II diabetes. DF components, in particular AX, also affect the processing properties of wheat, with respect to breadmaking, gluten-starch separation, the quality for livestock feed and fermentation to produce alcohol for beverages and biofuel (Courtin and Delcour, 2002; Frederix et al., 2004; Shewry et al., 2010b).
The content and composition of DF polysaccharides varies among cereal species. While wheat and rye are rich in AX, barley and oat have high β-glucan content. AX, the main pentosan component of the wheat grain, has a backbone chain of β-D-xylopyranosyl (Xylp) residues linked through (1-4)-glycosidic linkages. Some of the Xylp residues are monosubstituted with α-L-arabinofuranosyl (Araf) residues at position 3, or disubstituted at positions 2 and 3 of the same Xylp residues (Perlin, 1951; Renard et al., 1990; Hoffmann et al., 1991; Izydorczyk and Biliaderis, 1994). The AX in the secondary walls of the pericarp and seed coat tissues of the bran may also contain 4-O-methyl α-D-glucuronic acid as an additional substituent at position 2 of Xylp units (Schooneveld-Bergmans et al., 1999).
The optimum amount of AX to maintain good breadmaking quality while improving the health benefits in human diets will depend on several factors, including the molecular weight of the AX, the arabinose/xylose (A/X) ratio, the particle size of the fiber and the ferulic acid content (Morales-Ortega et al., 2013). Increased substitution of the xylopyranosyl residues with arabinofuranosyl residues is usually characterized by the ratio of the A/X present in the AX molecule (Ordaz-Ortiz and Saulnier, 2005), with a higher A/X ratio being associated with higher substitution and higher molecular weight. However, a lower A/X ratio of TOT-AX is associated with lower substitutions, lower molecular weight, and better end-use quality. Biliaderis et al. (1995) and Courtin and Delcour (1998) reported that high molecular weight (HMW) AX had greater effects on water absorption and on development time than lower molecular weight WE-AX. A lower amount of HMW polymer reduces the negative effects of fiber on technological properties and breadmaking quality, but the ability of AX to form highly viscous solutions decreases (Buksa et al., 2016). WE-AX could be characterized by lower molecular weight (2–20 kDa) and a lower A/X ratio (0.5–0.6) than insoluble AX (100–120 kDa or 300–600 kDa, 0.3–1.1) (Saulnier et al., 2007), with less negative effects on the quality.
β-Glucan is particularly important as a DF component in barley and oats and our previous studies indicated that this was also true for Aegilops species (unpublished data). The (1–3,1–4)-β-D-glucans are linear, unbranched polymers in which the β-D-glucopyranosyl residues are joined by both (1–3) and (1–4) glucosidic linkages. Single (1–3) linkages are separated by two or more (1–4) linkages, and regions of two or three adjacent (1–4) linkages predominate. The distribution of oligosaccharides in β-glucan differs in different cereal species (Cui et al., 2000; Lazaridou et al., 2004), with the relative proportion of trisaccharide [DP3 (degree of polymerization)] decreasing from wheat (67–72%), to barley (52–69%), and oats (53–61%) and the relative amount of tetrasaccharide (DP4) following the opposite trend. Differences in the ratio of DP3:DP4 may also occur within the same cereal species, which may be attributed to genotypic and environmental factors (Miller et al., 1993; Jiang and Vasanthan, 2000; Storsley et al., 2003; Wood et al., 2003).
Differences in the linkage distribution and molecular weight of β-glucan are likely to affect its solubility and viscosity (Lazaridou and Biliaderis, 2007; Cui and Wood, 2000), which are considered to be key parameters determining health benefits (Wood, 2007). However, high viscosity conferred by β-glucan has negative effects on feed intake, feed conversation rate, and weight gain, and may result in sticky feces when used to feed chickens (Hesselman et al., 1981). In malt and beer production high viscosity causes problems with haze formation and wort filtration (Bamforth, 2010), and reduces yield in starch production. In contrast, high contents of soluble β-glucan are favored for food products as they may reduce serum cholesterol levels and regulate blood glucose level (McIntosh et al., 1991; Cavallero et al., 2002; Wood, 2007). From a processing point of view, HMW β-glucan results in higher water absorption and viscosity (Skendi et al., 2009), lower loaf volume and height (Symons and Brennan, 2004; Izydorczyk and Dexter, 2008; Skendi et al., 2009), and stiffer dough (Cleary et al., 2007) than LMW β-glucan. Therefore it is technologically easier to incorporate LMW barley β-glucan fractions into breads. The flow behavior and gelling properties of β-glucan can also vary with the concentration and molecular weight (Lazaridou et al., 2003; Vaikousi et al., 2004; Skendi et al., 2009).
DF has been studied widely in wheat and other cereals (rye, barley, spelt), primarily focusing on variability in the amount and composition (Saulnier et al., 2007; Andersson et al., 2008; Gebruers et al., 2008; Rakszegi et al., 2008; Shewry et al., 2008; Ward et al., 2008), genetic control (Cyran et al., 1996; Boros et al., 2002; Burton et al., 2006; Mitchell et al., 2007; Charmet et al., 2009; Doblin et al., 2010; Nemeth et al., 2010; Quraishi et al., 2011; Taketa et al., 2012), heritability (Martinant et al., 1999; Li et al., 2009; Gebruers et al., 2010; Shewry et al., 2010b,c), and effects on animal and human health (Bedford and Schulze, 1998; Brouns et al., 2013; Lafiandra et al., 2014; Pirgozliev et al., 2015) and food processing (Courtin and Delcour, 2002; Frederix et al., 2004; Bonnand-Ducasse et al., 2010; Noort et al., 2010; Shewry et al., 2010a; Jones et al., 2015; Heinio et al., 2016). The health benefits of DFs triggered a search for wild alleles suitable to increase the level of DFs in wheat, mainly in the genus Triticum (Marcotuli et al., 2015, 2016). However, to date these efforts have neglected wild relatives of wheat from the genus Aegilops, although these are important donors of new genes and alleles for wheat breeding.
The genus Aegilops is the closest relative of genus Triticum and consists of 11 diploid, 10 tetraploid, and 2 hexaploid species (van Slageren, 1994) with six different genomes (D, S, U, C, N, and M), indicating the great genetic diversity of the genus. Twelve Aegilops species contain the U and/or M genomes, and two of these, the allotetraploid Aegilops biuncialis (2n = 4x = 28, UbUbMbMb) and Aegilops geniculata (2n = 4x = 28, UgUgMgMg), evolved from hybridization between the diploid Aegilops comosa (2n = 2x = 14, MM) and Aegilops umbellulata (2n = 2x = 14, UU) (van Slageren, 1994). Because of their great ecological adaptability, these species are promising sources of genes providing resistance to diseases (Lr9, Lr57, Sr34, Yr8, Yr40, Pm29) and tolerance to abiotic stresses such as salt, drought, frost, and heat stress (Friebe et al., 1996; Rekika et al., 1997; Zaharieva et al., 2001a,b; Molnár et al., 2004; Colmer et al., 2006; Schneider et al., 2008; Dulai et al., 2014).
Besides providing stress tolerance, the U- and M-genomes of Aegilops species are also rich reservoirs of genes for improving the nutritional quality of the wheat grain. Bálint et al. (2001) compared the contents of micro- and macronutrients in the grain of diploid, tetraploid, and hexaploid Triticum and Aegilops species and found that the Cu, Zn, Ca, and Mg contents were significantly higher in Ae. biuncialis and Ae. geniculata than in wheat. Rawat et al. (2009) also reported two- to threefold higher contents of iron and zinc in Ae. geniculata grain than in bread and durum wheats. These results were confirmed by Farkas et al. (2014), who found 1.5- to 2-fold higher contents of K, Zn, Fe, and Mn in Ae. biuncialis than in bread wheat. In wheat, the major determinants of grain processing quality are the gluten storage proteins, which consist of monomeric gliadins (Gli) and polymeric glutenins (Glu), with the gliadins determining dough extensibility and the glutenins its elasticity. Good quality is associated with a high ratio of unextractable polymeric glutenin proteins (UPP; Shewry et al., 1986; Larroque and Békés, 2000) rich in HMW subunits of glutenin. Variation in the HMW subunit composition of Ae. geniculata was reported by Medouri et al. (2015), who identified a total of 27 alleles at the two HMW glutenin loci, Glu-M1 and Glu-U1, resulting in 29 HMW glutenin protein patterns. Other studies on the composition of glutenins and gliadins in Aegilops species have also been reported (Bandou et al., 2009; Kozub et al., 2011; Wang et al., 2012, 2015; Ahmadpoor et al., 2014; Dai et al., 2015; Medouri et al., 2015; Garg et al., 2016).
While information is already available for micronutrients and gluten storage proteins of Aegilops, the content and composition of the grain DF components AX and β-glucan have not been reported yet in species with the U and M genomes.
One strategy to increase genetic variation in bread wheat is to introduce new genes by interspecific hybridization. Several useful agronomic traits have already been transferred from Aegilops into the wheat gene pool by developing wheat–Aegilops hybrids and chromosome addition and translocation lines, as reviewed by Schneider et al. (2008). Wheat-alien disomic chromosome addition lines are excellent genetic resources to study the performance of transferred alien traits in the wheat genetic background and to assign key genes to alien chromosomes. Wheat–Ae. biuncialis addition lines carrying chromosomes 1Ub, 3Ub, 2Mb, 3Mb, and 7Mb were developed by Molnár-Láng et al. (2002) and Schneider et al. (2005), while a complete set of wheat–Ae. geniculata addition lines was developed by Friebe et al. (1999).
The advent of next-generation sequencing technologies (Margulies et al., 2005) and improvements in the flow-cytometric sorting of mitotic chromosomes (Doležel et al., 2014; Rey et al., 2015) allow the identification of the gene repertoire of individual chromosomes and the development of gene-specific markers for large and complex Triticeae genomes, such as barley, rye, bread wheat (Mayer et al., 2011; Martis et al., 2013; The International Wheat Genome Sequencing Consortium [IWGSC], 2014), and their wild relatives (Tiwari et al., 2015). Molnár et al. (2016) reported the flow-sorting of the U- and M-genome chromosomes from the diploid progenitors of Ae. biuncialis and Ae. geniculata, Ae. umbellulata (UU), and Ae. comosa (MM). The high purity of the sorted fractions allowed the sequencing of the U genome based on the individual chromosomes of Ae. umbellulata, thereby producing genomic resources to identify the chromosomal positions in Aegilops of orthologs of the key genes responsible for agronomic traits of interest.
The main goals of the present study were to determine the effects of added Ae. biuncialis and Ae. geniculata chromosomes on the content and composition of the grain storage protein and DF components of hexaploid wheat, by carrying out detailed biochemical analyses of Ae. biuncialis and Ae. geniculata accessions and wheat–Aegilops addition lines. The chromosomal positions of putative orthologs of the key genes determining these components were also identified using Ae. umbellulata chromosome sequences.
Materials and Methods
Plant Material
Bread wheat (cv. Chinese Spring)/Ae. geniculata (TA2899) chromosome addition lines 1Ug, 2Ug, 3Ug, 4Ug, 5Ug, 6Ug, 7Ug, 1Mg, 2Mg, 3Mg, 5Mg, 6Mg, and 7Mg were kindly provided by Dr. Bernd Friebe (Kansas State University, Manhattan, KS, United States) and maintained by the Cereal Genebank, Martonvásár, while a set of bread wheat (line Mv9kr1)/Ae. biuncialis (MvGB642) chromosome addition lines 1Ub, 1Ub6Ub, 3Ub, 2Mb, 3Mb, and 7Mb were produced in Martonvásár (Molnár-Láng et al., 2002; Schneider et al., 2005). The recessive crossability allele kr1 was transferred from bread wheat cv. Chinese Spring (CS) into bread wheat cv. Martonvásári 9 (Mv9) by backcrossing the Mv9 × CS hybrids with Mv9. The Mv9kr1 line carries recessive crossability alleles Kr1 and Kr2, but the genotype is 93.75% Mv9 (Molnár-Láng et al., 1996). Ae. geniculata or Ae. biuncialis accessions were provided by the Cereal Genebank, Martonvásár (MvGB), by the Wheat Genetics Resource Center, Kansas State University, United States (TA) and by the Institute of Plant Genetics and Crop Plant Research, Gatersleben, Germany (AE) and maintained by the Cereal Genebank, Martonvásár.
Growing Conditions
Glasshouse Experiment
Seeds were germinated on wet filter paper in Petri dishes for 3 days at room temperature, and then potted into Jiffy7 pellets1. The 5-day-old seedlings were vernalized at 4°C for 6 weeks under low light intensity (20 μmol m-2 s-1). After vernalization, seedlings were grown in individual pots (one plant/2 l pot) filled with a 3:2:1 mixture of garden soil, compost, and sand, and placed randomly in the greenhouse (Global Glasshouse Venlo) for 12 weeks. Each pot was fertilized every 10 days for four times from the second week after planting with 150 ml of 0.1% g/v % complex fertilizer containing 14% N, 7% P2O5, 21% K2O, 1% Mg, and 1% micronutrients including B, Cu, Mn, Fe, Zn (Volldünger Classic, Gartenhilfe GmbH., Austria). Growth conditions were as follows: the initial 11/7°C day/night temperature and 13 h photoperiod gradually increased to 23/17 °C day/night temperature and 16 h photoperiod (16 h light/8 h dark) at maturity (12 weeks). The seeds of 10 plants per genotype were used for analysis.
Field Trial
The Aegilops accessions, the wheat–Ae. geniculata and wheat–Ae. biuncialis addition lines together with their parental lines were grown in chernozem soil in the experimental field in Martonvásár (2012/2013) characterized by low-input conditions (no fertilizers, herbicides, insecticides, and fungicides applied during the growing seasons). The weather conditions during the growing period (basically between October and July) could be characterized by the total precipitation of 387.5 mm and average temperature of 8.0°C (Mikó et al., 2014).
Each genotype was sown in randomized complete block design with two replications on October 15, 2012. The plots consisted of 5 × 1 m rows with a row distance of 15 cm and 50 seeds per plot. Plots were hand harvested at maturity and grain was stored at 4°C.
Methods
Four grams of seed from each sample was milled using a Retsch Mixer Mill MM 200 ball mill to produce wholemeal samples, which were immediately cooled and stored at -20°C until compositional analysis.
Thousand Kernel Weight
Thousand kernel weights (TKW) were determined by the standard MSZ 6367/4-86 (1987) method. Duplicate analyses were carried out on each sample.
Protein
Crude protein content was determined by the Kjeldahl method, consistent with International Association for Cereal Science and Technology ICC 105/2 (1995), using a Kjeltec 1035 Analyzer instrument. Duplicate analyses were carried out on each sample.
Protein Composition
Size exclusion-high-performance liquid chromatography was used to determine the glutenin, gliadin, and albumin + globulin contents and the UPP (UPP% = insoluble glutenin/soluble + insoluble glutenin) content using a modification of the Batey et al. (1991) method. Ten milligram flour was suspended in 1 ml 0.5% (w/v) SDS in phosphate buffer (pH 6.9) and sonicated for 15 s. After centrifugation, the supernatant was filtered on a 0.45 μm PVDF filter. Analyses were performed on a Phenomenex BIOSEP-SEC 4000 column in acetonitrile buffer [0.05% (v/v) trifluoroacetic acid and 0.05% (v/v) acetonitrile] with a running time of 10 min (2 ml/min flow rate). Proteins were detected by absorption at 214 nm.
Quantitative Determination of Total and Water-Extractable Pentosans
Total and water-extractable pentosans, in which AX is the main component, were determined using a colorimetric method, as described by Douglas (1981) and Finnie et al. (2006). A total of 12.5 ml Milli-Q water was added to 62.5 mg flour and shaken (TOT-pentosan). An aliquot of the suspension (0.5 ml) was diluted to 1.0 ml with water and 5 ml freshly prepared extraction solution was added [93.2% (v/v) acetic acid, 1.69% (v/v) HCl, 0.85% (w/v) phloroglucinol and 0.017% (w/v) glucose]. The tubes were placed in a boiling water bath for 25 min and the absorbance of the samples was measured after cooling at 552 and 510 nm. The remainder of the flour water suspension was shaken for 30 min to determine water-extractable pentosans (WE-pentosan). The suspension was centrifuged at 2500 g for 10 min, 0.5 ml of the supernatant was removed and diluted to 1.0 ml with water, and 5 ml of extraction solution was added. The sample was then boiled for 25 min and the absorbance was measured after cooling at 552 and 510 nm. The pentose concentration was determined by comparing the absorbance values with those of D-(+)-xylose standards. Duplicate analyses were carried out on each sample.
Quantitative Determination of β-Glucan
The total amount of mixed-linkage β-glucan was determined in wholemeal samples using a Megazyme kit (Megazyme, Bray, Ireland) (AACC International, 1995; International Association for Cereal Science and Technology ICC 166, 1998). Duplicate analyses were carried out on each sample.
Enzyme Fingerprinting of AX and β-Glucan
The protocol was adapted from Ordaz-Ortiz et al. (2004, 2005). One milliliter of 80% (v/v) ethanol was added to 100 mg of flour and heated in a 95°C water bath for 5–10 min to inactivate the enzymes. After centrifugation, the residue was washed first with 80% (v/v) ethanol and then with 95% (v/v) ethanol and dried using a Speedvac centrifugal evaporator. The dried powder was resuspended in 1 ml of water containing 16U of endoxylanase (Megazyme, Trichoderma viride, Xylanase M1, Bray, Ireland) and 2U of lichenase (Megazyme) and incubated at 40°C for 16 h with continuous rotation. After centrifugation, 0.6 ml of the supernatant was heated for 10 min in a 95°C hot water bath to inactivate the enzymes. The samples were then centrifuged and filtered using 0.45 μm Millex-HV syringe-driven filters. After water dilution (1:20), samples were injected onto an HPAEC system (high-performance anion-exchange chromatography) using a Carbopac PA1 analytical column (4 mm × 250 mm) (Ordaz-Ortiz et al., 2004, 2005). Duplicate analyses were carried out on each sample. The proportions of unsubstituted, monosubstituted, and disubstituted xylose residues in the AX oligosaccharides (AXOS) and the ratio of the DP3 and DP4 units of β-glucan are calculated from the peak areas (Saulnier et al., 2009; Toole et al., 2010), calculated as
monosubstituted (M) = (XA3XX) + 2(XA3A3XX) + 2(XA3XA3XX) + (XA3A2+3X) + (XA3XA2+3XX), disubstituted (D) = (XA2+3XX) + (XA3A2+3X) + (XA3XA2+3XX), unsubstituted (US) = X + XX + XXX, and total (TOT) = X + XX + XXX + XA3XX + XA3A3XX + XA3XA3XX + (XA2+3XX) + (XA3A2+3XX) + (XA3XA2+3XX) AXOS (Supplementary Figure S1).
DNA Sequence Analysis
Mitotic chromosomes 1U, 2US, 2UL, 3U, 4U, 5U, 6U, 7U, and 7UL of Ae. umbellulata (2n = 2x = 14, UU) accession AE740/03 were purified by flow sorting as described by Molnár et al. (2016). DNA was amplified from three samples of each chromosome using the Illustra GenomiPhi V2 DNA Amplification Kit (Šimková et al., 2008), pooled and sequenced with HiSeq 2000 (Illumina, Inc., San Diego, United States) using standard protocols. The DNA of each chromosome was sequenced on one lane of the instrument, representing nine lanes with ∼130 millions of paired-end reads (∼26 Gb) for each dataset. De novo assembly was done with a MaSuRCA assembler (Zimin et al., 2013) and contigs shorter than 200 bp were removed. The databases of Illumina reads and assembled contigs were made publicly available on the web site of IEB, Olomouc2. Sequences of key genes and alleles determining the composition and quantity of storage proteins, β-glucan, and AX in the grain were downloaded from a publicly available database3,4 (Schreiber et al., 2014) and used as queries for BLASTn searches against Ae. umbellulata chromosome sequences (Supplementary Tables 1–3). The first best hits with at least 75% sequence identity and a minimal alignment length of 200 bp were considered significant and used to obtain the chromosomal positions of key genes in the U genome of Ae. umbellulata.
Statistical Analyses
Two replications were made for the TKW, protein, β-glucan, pentosan, and AX measurements and if the difference between the two replicate samples was higher than 10% the measurement was repeated with two more replications. Three replicate samples were measured for Glu/Gli and UPP%. Least significant difference values between the addition lines, together with the parental wheat and Aegilops genotypes, were calculated at the p = 0.05 probability level using the Microsoft Excel program.
Results
Variation in Composition within Species, Ae. biuncialis and Ae. geniculata
No studies have yet been reported on the composition of DF in Aegilops species with the U and M genomes, but previous results on HMW glutenin subunit alleles showed wide variation within these species (Ahmadpoor et al., 2014; Dai et al., 2015; Medouri et al., 2015; Wang et al., 2015; Garg et al., 2016). In order to determine the extent of variation in the amounts of protein, β-glucan, TOT-pentosan, and WE-pentosan in Ae. geniculata and Ae. biuncialis, five genebank accessions of each species were studied. The Ae. geniculata and Ae. biuncialis accessions had significantly lower TKW than the two wheat genotypes (cv. Chinese Spring and line Mv9kr1) used in the present study (Figure 1A), with values ranging between 17.4 and 22.1 g (accessions AE754/90 and AE274/80, respectively) in Ae. biuncialis, and between 9.3 and 17.0 g (accessions AE1311/00 and AE839/91, respectively) in Ae. geniculata.
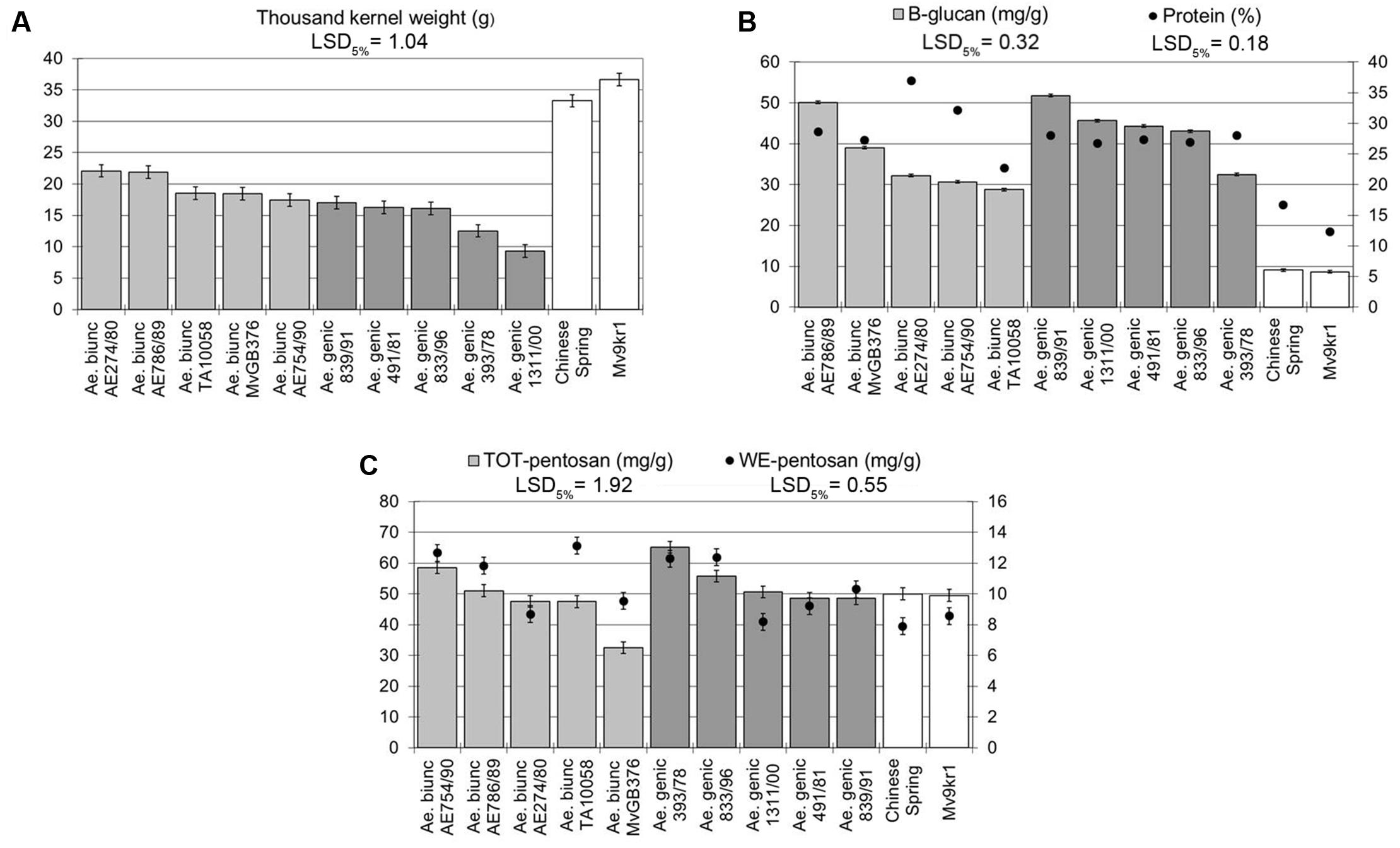
FIGURE 1. Thousand kernel weight and compositional properties of five Aegilops biuncialis and five Aegilops geniculata genebank accessions. (A) Thousand kernel weight, (B) protein and β-glucan content, (C) TOT-pentosan and WE-pentosan content. LSD, least significant difference; TOT, total; WE, water extractable.
The β-glucan and protein content were significantly higher in all Aegilops accessions than in the wheat genotypes (Figure 1B). Within Ae. biuncialis and Ae. geniculata, the β-glucan content varied between ∼30 and 50 mg/g irrespective of the TKW of the accessions. Interestingly, the protein content was more variable among the Ae. biuncialis accessions (∼22–37%) than in Ae. geniculata genotypes (∼26%), where differences were not observed for this parameter. The amounts of TOT-pentosan and WE-pentosan also varied (∼30–60 and ∼8–14 mg/g, respectively), but with the exception of one accession (MvGB376) were similar to or above those in wheat (Figure 1C).
Effect of Aegilops Chromosomes on Thousand Kernel Weight and Storage Protein Content in Wheat
The parental Ae. geniculata TA2899 and Ae. biuncialis MvGB642 genotypes have significantly lower (<50%) TKW than the parental wheat genotypes cv. Chinese Spring and cv. Mv9kr1 (Figure 2A). In the Chinese Spring × Ae. geniculata combination, chromosome addition lines 1Ug, 4-5-6Ug, 1Mg, and 7Mg showed significantly higher TKW than the wheat parent, while addition lines containing chromosomes 2Ug, 2Mg, and 5Mg exhibited significantly lower TKW. Ae. biuncialis chromosomes had no effect on this parameter. In parallel with the lower TKW values, the Aegilops accessions had higher protein content relative to wheat (Figure 2B), which can be attributed to the “yield dilution” effect, i.e., the high grain weight of wheat, resulting from the increased starch content and the consequent relative decrease in grain storage proteins. The addition of chromosomes 2Ug, 4Ug, 5Ug, 7Ug, 2Mg, 5Mg, and 7Mg of Ae. geniculata significantly increased the protein content of wheat cv. Chinese Spring, while the addition of Ae. biuncialis chromosomes 3Ub, 2Mb, 3Mb, and 7Mb significantly increased that of wheat line Mv9kr1 (Figure 2B).
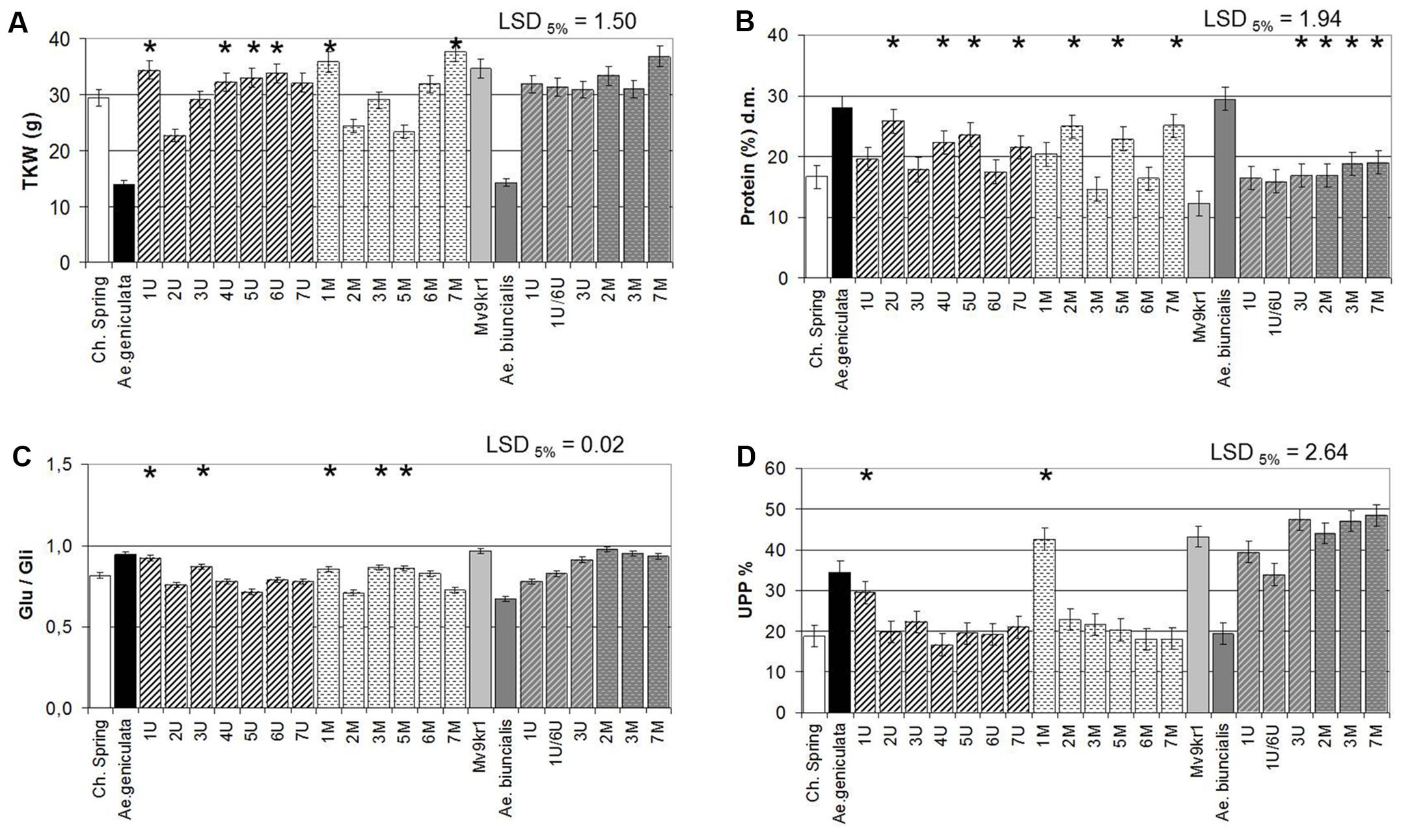
FIGURE 2. Compositional properties of mature grains of two lines of bread wheat (cv. Chinese Spring and Mv9kr1 line), two Aegilops species (Ae. geniculata and Ae. biuncialis), and wheat–Aegilops chromosome addition lines. (A) TKW, (B) protein, (C) Glu/Gli, (D) UPP%. Gli, gliadin; Glu, glutenin; LSD, least significant difference; TKW, thousand kernel weight; UPP, unextractable polymeric protein. ∗Significantly higher than the wheat (Triticum aestivum) control.
Under field conditions, the 6Ug, 3Mg, 6Mg, and 7Mb additions exhibited the most stable TKW similar to wheat, while the TKW of all the other lines were decreased by adding the Aegilops chromosomes (Supplementary Figure S2a). It is probable that the very low TKW resulted the significantly higher protein contents of Aegilops than wheat (with the exception of 2Mb and 3Mb), with 1Ub, 1U/6Ub, 2Ug, 3U, 4Ug, 2Mg, and both 7M having the greatest effects (Supplementary Figure S2b).
The good processing quality of wheat is related to the high content of polymeric glutenins relative to gliadins (Glu/Gli) and to the high ratio of UPP (UPP%). Ae. geniculata accession TA2899 had a higher Glu/Gli ratio and UPP% than cv. Chinese Spring, whereas these two parameters were lower in Ae. biuncialis MvGB642 than in Mv9kr1 (Figures 2C,D). It should be noted that the model wheat genotype cv. Chinese Spring, which has poor processing quality properties, exhibited lower Glu/Gli ratio and UPP% than line Mv9kr1 whose breadmaking quality parameters are good. This is why the effect of Aegilops chromosomes on the quality parameters of wheat was more pronounced in cv. Chinese Spring than in Mv9kr1. In agreement with this, the added chromosomes 1Ug and 1Mg significantly increased the proportion of polymeric glutenin proteins (higher Glu/Gli ratio and UPP%) in the Chinese Spring background, which can be expected to result in improved processing quality (Figures 2C,D). The addition of chromosomes 3Ug, 3Mg, and 5Mg also increased the Glu/Gli ratio, but did not affect the UPP%. In the case of Ae. biuncialis, chromosomes 1Ub and 1Ub/6Ub again had the greatest effects on the protein composition and the proportion of glutenin polymers (Figures 2C,D), but these were negative, with the proportion of monomeric gliadins increasing instead of the glutenins.
Effect of Aegilops Chromosomes on β-Glucan and AX Content
The β-glucan content expressed in mg/g dry weight of wholemeal (measured using the commercial kit for total β-glucan content) was about fivefold higher in Ae. geniculata TA2899 than in cv. Chinese Spring and 2.5-fold higher in Ae. biuncialis MvGB642 than in line Mv9kr1 (Figure 3A). Chromosome addition lines 5Ug, 7Ug, and 7Mg, which had similar or higher TKW than wheat, had a positive effect on β-glucan content in the Chinese Spring genetic background. In the case of Mv9kr1 × Ae. biuncialis addition lines, significantly higher β-glucan content was also observed in the 7Mb addition line.
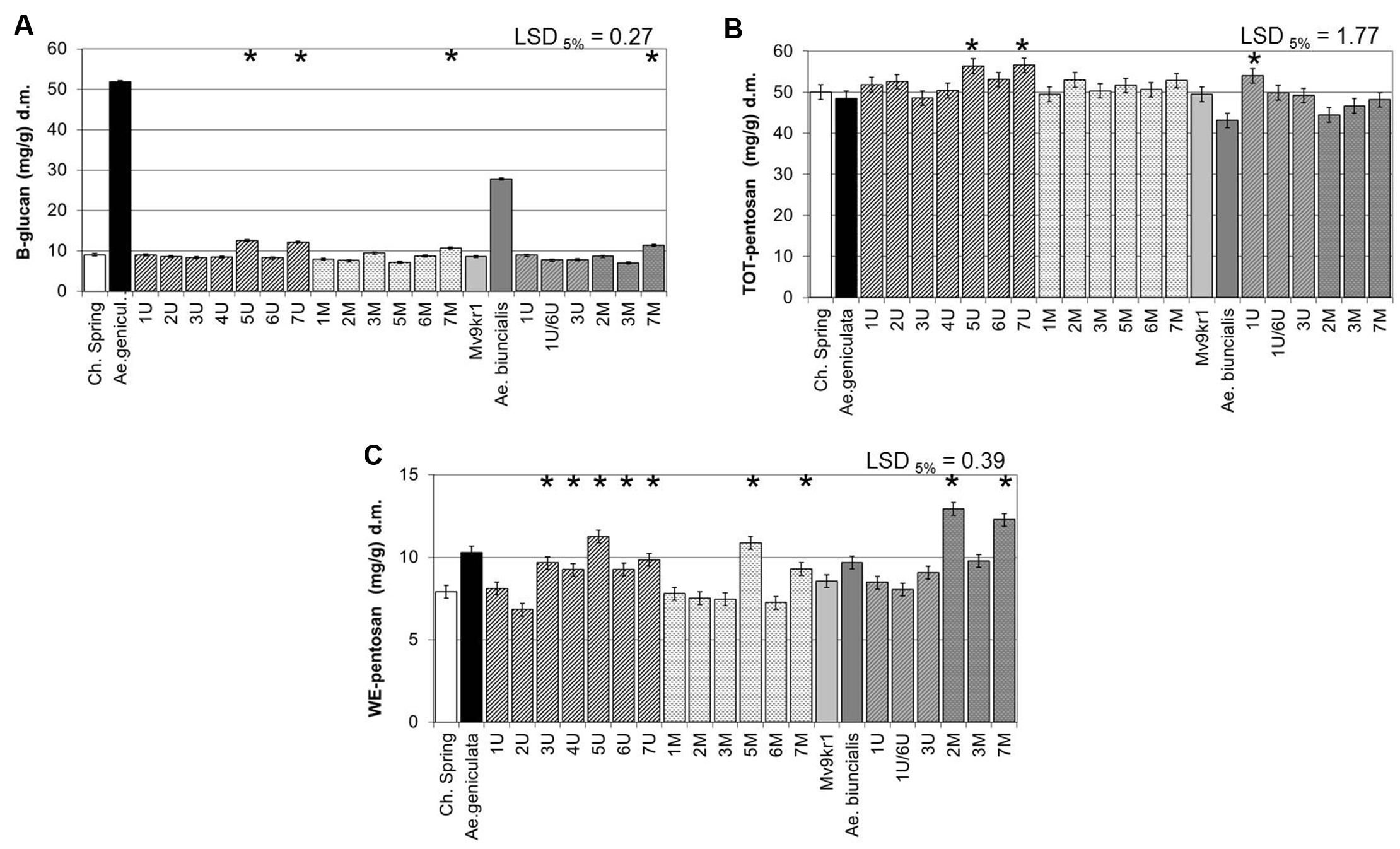
FIGURE 3. Compositional properties of mature grains of two lines of bread wheat (cv. Chinese Spring and Mv9kr1 line), two Aegilops species (Ae. geniculata and Ae. biuncialis), and wheat–Aegilops chromosome addition lines. (A) β-Glucan, (B) TOT-pentosan, and (C) WE-pentosan content. LSD, least significant difference; TOT, total; WE, water-extractable. ∗Significantly higher than the wheat (T. aestivum) control.
Aegilops chromosomes 5Ug, 7Ug, 7Mg, and 7Mb were able to increase the β-glucan content of wheat wholemeal under field conditions, which fully support the results of the glass house experiments (Supplementary Figure S2c). Moreover, the significant effect of chromosome 1Ub was also shown in the field experiment.
The content of total AX (measured as TOT-pentosan) was similar in Ae. geniculata and cv. Chinese Spring and lower in Ae. biuncialis than in line Mv9kr1 (Figure 3B). The effects of chromosome additions were generally not significant, but small increases were observed with the addition of chromosomes 5Ug and 7Ug, which increased the total AX content of wheat (measured as TOT-pentosan in mg/g dry weight of wholemeal), while in the Mv9kr1 genetic background only chromosome 1Ub gave a higher value than the parental wheat genotype (Figure 3B). No significant differences were observed in the contents of TOT-pentosan in the parental lines and the addition lines in the field experiment (Supplementary Figure S2d).
The water-extractable fraction of total pentosans (WE-pentosan) was higher in the Aegilops parents in comparison with the corresponding wheat genotypes (Figure 3C). At the level of single chromosomes, significantly higher WE-pentosan content was observed in chromosome addition lines 3Ug, 4Ug, 5Ug, 6Ug, 7Ug and 5Mg and 7Mg, the highest positive effect being exerted by the group five chromosomes of Ae. geniculata. In the case of Ae. biuncialis, chromosome addition lines 2Mb and 7Mb showed the highest level of WE-pentosan, exceeding those of all the other genotypes investigated (wheat–Ae. geniculata and wheat–Ae. biuncialis additions). The results of the glasshouse experiment were supported by the field experiment. Similar to the glasshouse experiments, chromosome additions 5Ug and 5Mg showed the highest WE-pentosan contents among the Chinese Spring–Ae. geniculata addition lines, although their values were not significantly different from the wheat parent, while the Ae. biuncialis 7Mb chromosome addition had significantly higher WE-pentosan content than the parental wheat Mv9kr1 (Supplementary Figure S2e).
The ratio of TOT-pentosan (mainly AX) to β-glucan reflects the composition of non-starch cell wall polysaccharides. In the present experiment, the parental wheat genotypes had TOT-pentosan to β-glucan ratios of 4.5 and 5.5 (in cv. Chinese Spring and line Mv9kr1, respectively). In contrast, TOT-pentosan to β-glucan ratios of only 1.0 and 1.5 were determined for the Ae. geniculata TA2899 and Ae. biuncialis MvGB642 (Figure 4A), which was attributed to the high β-glucan content of the Aegilops genotypes (Figure 3A). In Chinese Spring–Ae. geniculata chromosome additions, this ratio was higher in lines containing chromosomes 1Ug, 2Ug, 3Ug, 6Ug, and 1Mg, 2Mg, and 5Mg than in the wheat parent, while the chromosome addition line 7Ug showed a lower value for this parameter. Within the set of Ae. biuncialis additions, chromosomes 2Mb and 7Mb significantly decreased the ratio of TOT-pentosan to β-glucan relative to wheat. The ratio of water-extractable to unextractable pentosans (WE/WU pentosan) indicates the relative amounts of the two pentosan fractions, which have different health-promoting effects. The higher WE/WU pentosan ratio showed that the two Aegilops accessions have higher proportions of water-extractable pentosans than the wheat parents (Figure 4B). A relatively higher amount of water-extractable pentosans was also detected in the wheat–Aegilops addition lines 5Mg, 2Mb, and 7Mb (Figure 2C).
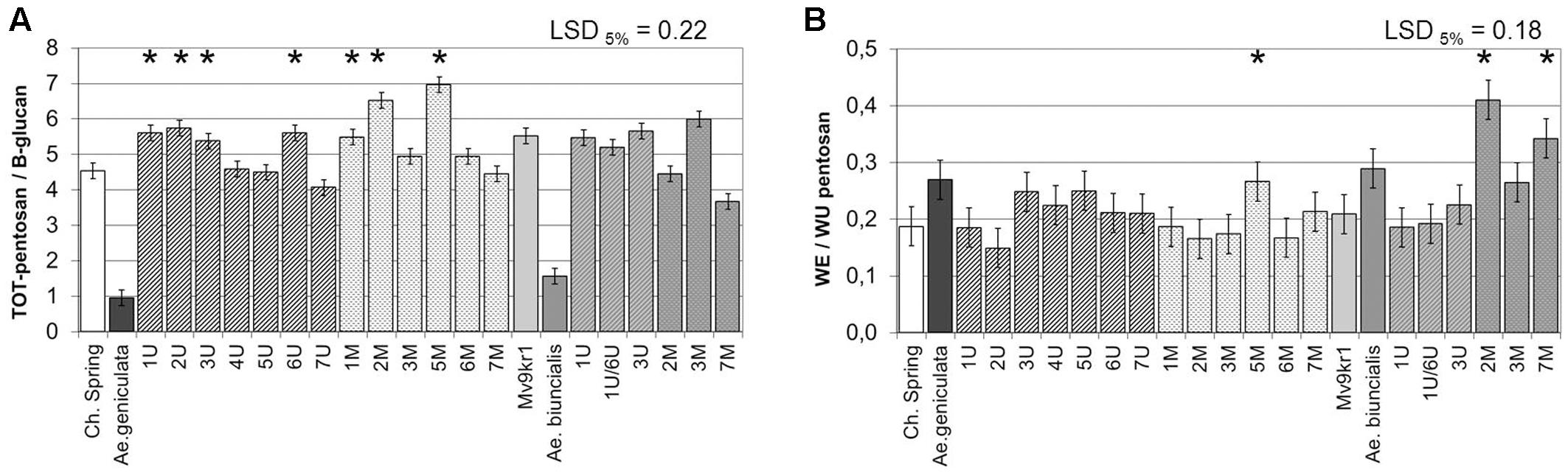
FIGURE 4. Quantitative ratio of TOT-pentosan to β-glucan (A) and WE to WU-pentosan (B) in mature grains of two lines of bread wheat (cv. Chinese Spring and Mv9kr1 line), two Aegilops species (Ae. geniculata and Ae. biuncialis), and wheat–Aegilops chromosome addition lines. LSD, least significant difference; TOT, total; WE, water extractable; WU, water-unextractable. ∗Significantly higher than the wheat (T. aestivum) control.
Effect of Added Aegilops Chromosomes on the Structure of β-Glucan and AX Polymers
Differences in the structure of β-glucan were determined after digestion with lichenase. Lichenase releases glucooligosaccharides (GOS) with degrees of polymerization (DP) of up to 10, with DP3 and DP4 GOS being the major forms. The values for TOTAL-GOS were between 1.6- and 1.3-fold higher in Ae. geniculata TA2899 and Ae. biuncialis MvGB642, respectively, than in wheat (Figure 5A). The ratio of DP3:DP4 glucan units, which represents the ratio of β-(1–3) to β-(1–4) bonds in the polymer, was significantly lower in both Aegilops species than in wheat (Figure 5B). The effect of chromosomes 7Ug and 7Mg on the TOTAL-GOS content, determined using HPAEC (Figure 5A) was similar to that of these chromosomes on the β-glucan content of wheat, determined with a Megazyme kit (Figure 3A). The addition of chromosome 5U reduced the ratio of DP3:DP4 glucan units released by lichenase from TOTAL-GOS. In addition, chromosomes 6Ug and 3Ub had decreasing effects on the DP3:DP4 ratio (Figure 5B), reflecting a higher ratio of larger polymers.
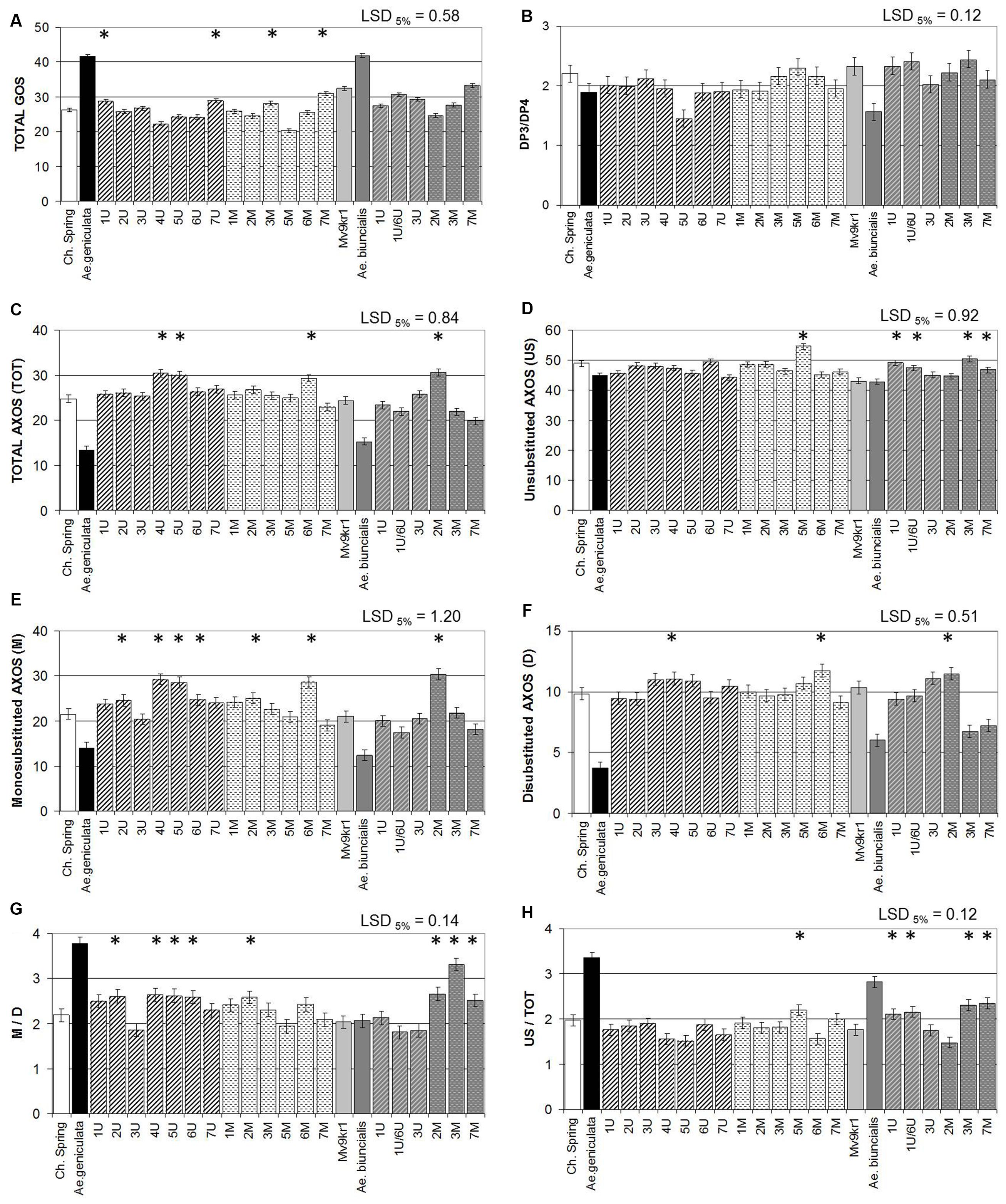
FIGURE 5. Quantity of arabinoxylan units in mature grains of two lines of bread wheat (cv. Chinese Spring and Mv9kr1 line), two Aegilops species (Ae. geniculata and Ae. biuncialis), and wheat–Aegilops chromosome addition lines after enzymatic fingerprinting. (A) Quantity of β-glucan units after enzymatic fingerprinting, (B) ratio of DP3 to DP4 units, (C) TOT-AXOS, (D) unsubstituted AXOS (US), (E) monosubstituted AXOS (M), (F) disubstituted AXOS (D), (G) M/D ratio, (H) US/TOT ratio. The amounts of unsubstituted, monosubstituted, and disubstituted xylose residues in the AXOS are derived from the AXOS peak areas, calculated as (M) = (XA3XX) + 2(XA3A3XX) + 2(XA3XA3XX) + (XA3A2+3X) + (XA3XA2+3XX), (D) = (XA2+3XX) + (XA3A2+3X) + (XA3XA2+3XX), (US) = X+XX+XXX, and (TOT) = X + XX + XXX + XA3XX + XA3A3XX + XA3XA3XX + (XA2+3XX) + (XA3A2+3XX) + (XA3XA2+3XX) AXOS, arabinoxylan oligosaccharide; DP, degree of polymerization; LSD, least significant difference; TOT, total. ∗Significantly higher than the wheat (T. aestivum) control.
Differences in the structure of AX were determined after digestion with endoxylanase. AX molecules have a backbone of xylose residues which may be substituted with arabinose. They vary in structure, in terms of both the proportion and distribution of unsubstituted, monosubstituted, and disubstituted xylose residues. Digestion with a specific endoxylanase releases AXOS which can be separated and quantified by HPAEC, giving “fingerprints” for the samples. As the structures of the separated AXOS have been determined (Ordaz-Ortiz et al., 2005), the peak areas can also be used to compare the proportion of AXOS containing unsubstituted, monosubstituted, and disubstituted xylose residues. This comparison showed that TOTAL-AXOS was ∼50% lower in both Ae. geniculata and Ae. biuncialis than in wheat (Figure 5C). The proportion of substituted xylose residues was also significantly lower in both parental Aegilops accessions than in the wheat genotypes (Figures 5D–F,H). In addition, the ratio of monosubstituted to disubstituted xylose residues was almost twice as high in Ae. geniculata than in wheat or Ae. biuncialis (Figure 5G). The ratio of unsubstituted AXOS to total AXOS (Figures 5C,D) was increased by the addition of chromosomes 5Mg, 1Ub, 1Ub6Ub, 3Mb, and 7Mb, with monosubstituted AXOS being affected by chromosomes 2Ug, 3-5Ug, 2M, and 6Mg (Figure 5E) and disubstituted AXOS by chromosomes 4Ug, 6Mg, and 2Mb (Figure 5F). The ratio of monosubstituted to disubstituted AXOS was increased by the addition of chromosomes 2Ug, 4-6Ug, 2M, 3Mb, and 7Mb (Figure 5G).
Chromosomal Assignment of Genes Involved in the Biosynthesis of Storage Proteins, β-Glucan, or AX in Aegilops
In order to identify Aegilops homologs of the key genes responsible for the biosynthesis of storage proteins, β-glucan, or AX, a comparison was made between wheat or barley and the Aegilops genomes. As complete sequences of the U- and M-genomes of Ae. geniculata and Ae. biuncialis are not available, chromosome survey sequences of the Ae. umbellulata genomes were used for comparative analysis (see text footnote 3). A BLASTn search on the cDNA sequences of the key genes responsible for wheat grain storage proteins (Table 1 and Supplementary Table 1) showed that most of the investigated genes (HMW glutenins, LMW glutenins, γ-gliadins) were assigned to the same homeologous group chromosomes (group 1) in the U-genome of Ae. umbellulata as in bread wheat (Table 1 and Supplementary Table 1). The α-gliadin genes, which are assigned to group 6 chromosomes in bread wheat, were located on the 1U and 3U chromosomes of Aegilops. As for the genes involved in β-glucan biosynthesis (OsCslF1-F2, HvCslF3-4, HvCslF6-10, HvCslF12-13, HvCslH1), the Aegilops homologs were again assigned to the same homeologous group chromosomes (group 1, 2, 5, and 7) as in bread wheat (Table 2 and Supplementary Table 2). This was also true for most of the genes responsible for AX biosynthesis, which were assigned to group 4 or 7 chromosomes (TaGT43 family), group 3 chromosomes (TaGT47 family), and group 2 chromosomes (TaGT75 family) (Table 2 and Supplementary Table 3). However, some Aegilops homologs were found on different chromosomes than the wheat genes. For example, gene TaGT47-12 was assigned to the group 3 chromosomes of hexaploid wheat, while its Aegilops homolog was found on chromosome 6U. Differences in chromosomal assignments were also found for genes HvCslF11, TaGT61-1, TaGT61-2, and TaGT75-4 (Table 2 and Supplementary Tables 2, 3).
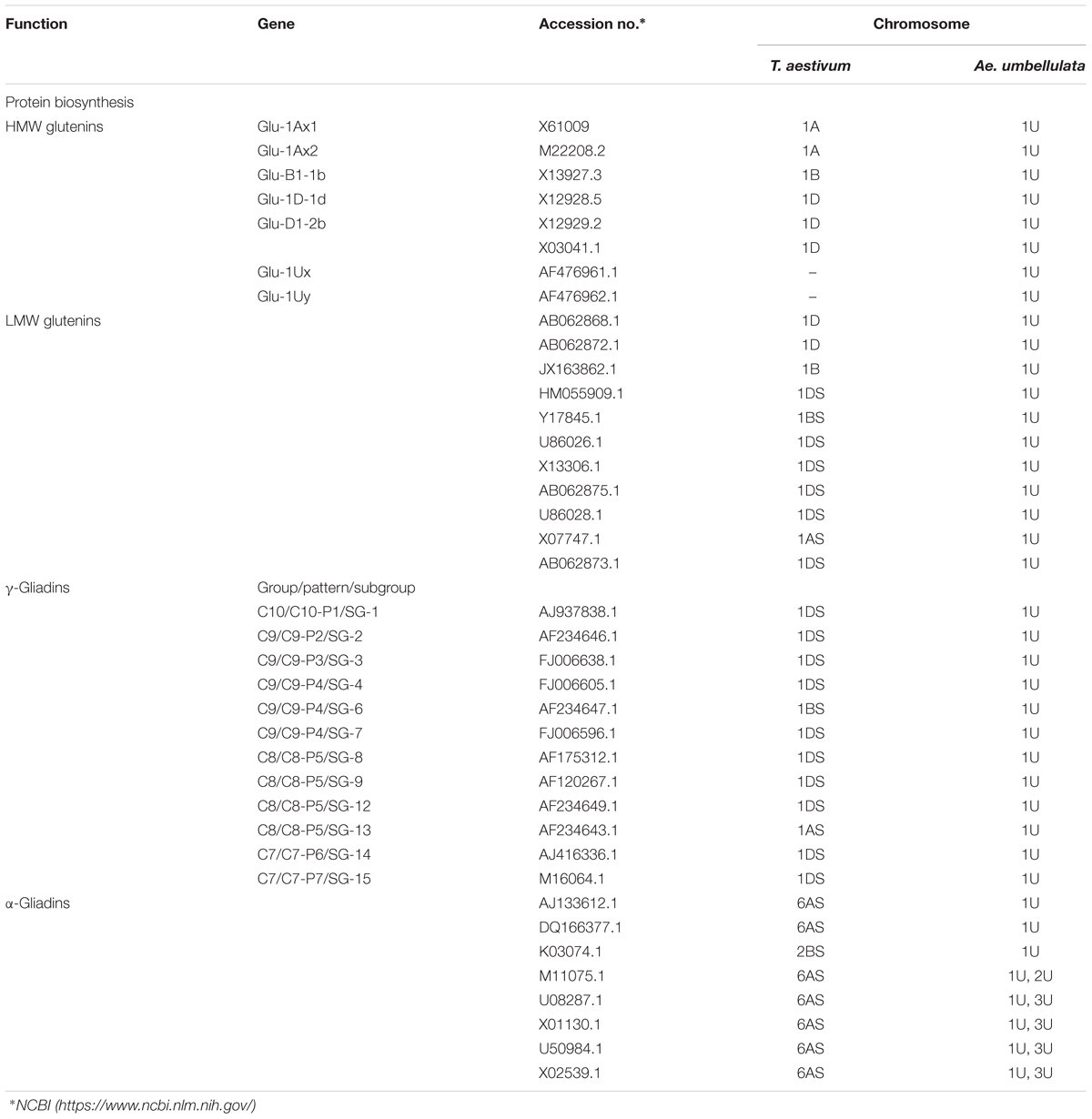
TABLE 1. Chromosomal assignment of genes responsible for grain storage protein biosynthesis in hexaploid wheat and Ae. umbellulata.
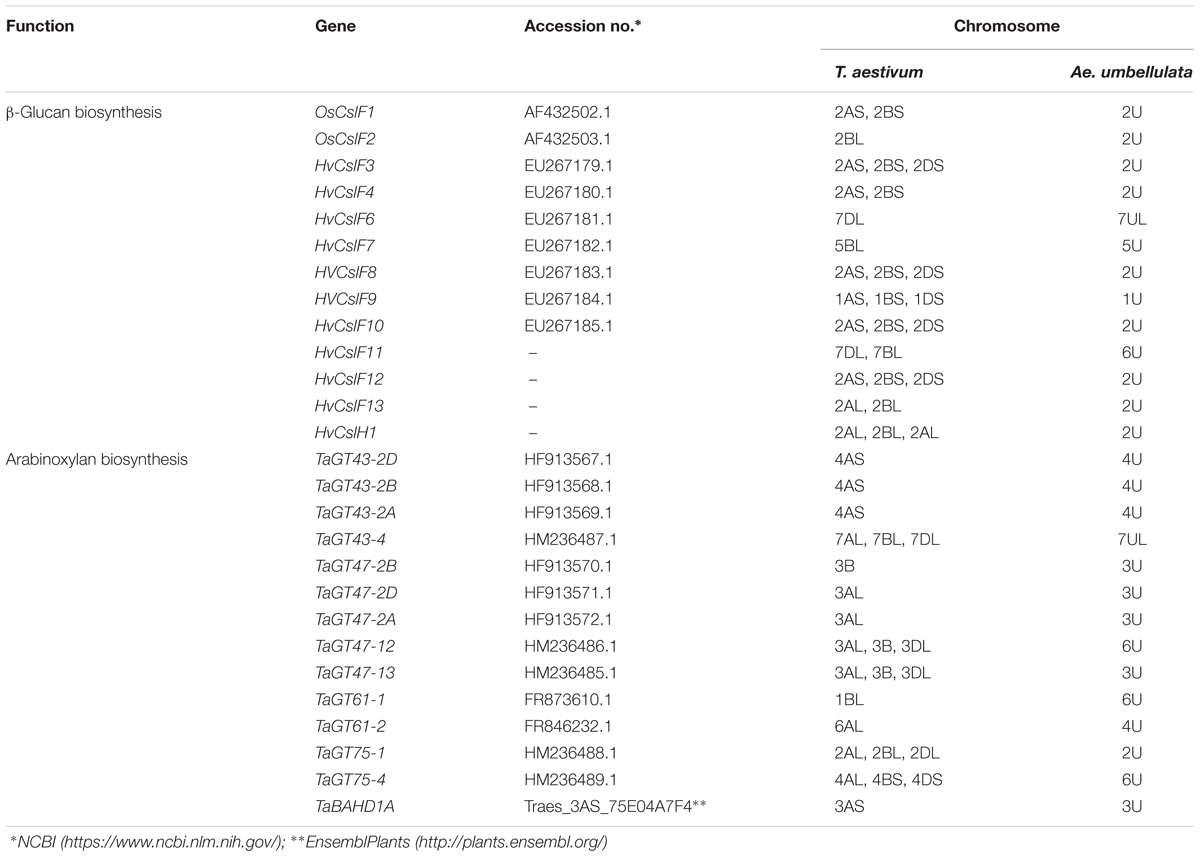
TABLE 2. Chromosomal assignment of genes responsible for β-glucan and arabinoxylan biosynthesis in hexaploid wheat and Ae. umbellulata.
Discussion
Despite their high nutritional value, very few studies have examined the potential of wild genetic resources to improve the content and composition of the edible fiber components in wheat grain (Marcotuli et al., 2015, 2016). Ae. biuncialis and Ae. geniculata exhibited substantial genetic diversity in their protein and fiber fractions relative to the wheat parents used in this study, which indicates that the effect of the chromosome additions on the quality of these components could be reliably studied. Therefore, the present study focuses on the ability of the U- and M-genome chromosomes of Aegilops to modify the amount and composition of storage proteins, AX and β-glucan in bread wheat. Furthermore, this work also provides information on the chromosomal assignment of potential wild alleles of key genes responsible for the biosynthesis of proteins and DFs.
Proteins
The addition of the group 1 chromosomes of Ae. geniculata (1Ug and 1Mg) to bread wheat was found to increase the proportion of insoluble glutenins relative to total glutenins (%UPP). These chromosomes also increased the ratio of glutenins to gliadins (Glu/Gli) (Figures 2C,D). These results are in line with the observations of Garg et al. (2016), who used the same set of Chinese Spring–Ae. geniculata addition lines and found that chromosome 1Mg exhibited greater dough strength than the parental wheat. Wheat gluten protein has been studied in great detail for more than a half century, with the first genetic studies dating back to the 1960s. It has been established that three major groups of wheat gluten proteins (LMW subunits of glutenin, ω-gliadins, and γ-gliadins) are encoded by genes on the short arms of the group 1 chromosomes of all three genomes of wheat (A, B, and D), while the HMW subunits of glutenin are encoded by genes on the long arms of the same chromosomes. A further group of gluten proteins, the α-gliadins, are encoded by genes on the short arms of the group 6 chromosome (Payne, 1987; Shewry et al., 2003a,b, 2009).
Wild homologs of the gluten protein genes were detected on the 1U chromosome sequence contigs of Ae. umbellulata (Table 1 and Supplementary Table 1), consistently with previous studies on Ae. geniculata (Medouri et al., 2015). A recent comparative analysis of individual flow-sorted chromosomes of Ae. umbellulata, Ae. comosa, and wheat indicated that chromosomes 1U and 1M are syntenic with the group 1 chromosomes of hexaploid wheat at the macro level (Molnár et al., 2016), suggesting that the 1Mg chromosome of Ae. geniculata may also contain wild alleles of gluten protein genes.
β-Glucan
The proportion of dietary fiber components in both Aegilops species was more similar to that in oats, barley, and Brachypodium than to that in wheat, with higher proportions of β-glucan than of AX. There is considerable interest in increasing the content of β-glucan in wheat flour due to its known health benefits (Buttriss and Stokes, 2008; Anderson et al., 2009; Tighe et al., 2010). The addition of chromosome 5Ug or group 7 chromosomes from the U and M genomes of Ae. geniculata and Ae. biuncialis were able to significantly increase the β-glucan content of wheat (Figure 3A) across different growth conditions. The addition of the 1Ug, 7Ug (or 7Mg), and 3Mg chromosomes also increased TOTAL-GOS (Figure 5A). Chromosome 5U was also found to reduce the quantitative ratio of DP3:DP4 glucan units (Figure 5B). Earlier studies indicated that the addition of 0–5% β-glucans to bread flour significantly reduced dough extensibility and loaf volume (Brennan and Cleary, 2007), while the solubility of the fibers was decreased by higher β-glucan levels (Izydorczyk and Dexter, 2008), but increased by a lower DP3:DP4 ratio (Izydorczyk and Dexter, 2008). The two opposite effects probably result in the solubility of β-glucan remaining nearly constant in the Aegilops addition lines, while little changes could be expected in their processing properties.
Cellulose synthase-like (Csl) genes are candidates to encode enzymes that synthetize the backbone of various non-cellulosic cell wall polysaccharides (Doblin et al., 2009). They have been classified into nine gene families, designated CslA to CslJ, of which the CslF, CslH, and CslJ families are restricted to cereals, although the CslJ group is not found in rice (Oryza sativa L.) or Brachypodium distachyon L. (Doblin et al., 2010). Expression in transgenic Arabidopsis thaliana L. plants revealed that the barley CslF and CslH families are probably involved in β-glucan synthesis (Burton et al., 2006; Doblin et al., 2009). Comparative genomic studies have shown that barley has 10 CslF family members; HvCslF3, HvCslF4, HvCslF8, HvCslF10, HvCslF12, and HvCslF13 clustered on chromosome 2H, HvCslF9, located on 1H, HvCslF7, located on 5H and HvCslF6 and HvCslF11, located on 7H (Burton et al., 2008; Schreiber et al., 2014). Among the HvCslF genes of barley, HvCslF6 and HvCslF9 have the highest levels of mRNA transcripts in developing barley endosperms (Burton et al., 2008) and map to loci near the centromeres of chromosomes 7H and 1H, respectively, which are close to quantitative trait loci (QTL) for the β-glucan content of barley grain (Igartua et al., 2002; Molina-Cano et al., 2007; Burton et al., 2008). Taketa et al. (2012) analyzed barley mutants lacking β-glucan and showed that the HvCslF6 gene had a unique role and was the key determinant controlling the biosynthesis of β-glucan, while Nemeth et al. (2010) demonstrated the role of the CslF6 gene in wheat β-glucan synthesis by RNAi suppression in grain of transgenic plants.
The β-glucan content of wheat–Aegilops chromosome addition lines was consistent with the locations of putative β-glucan synthase genes in the U genome of Ae. umbellulata. A sequence similarity search on the barley Cellulose-synthase-like F (CslF) gene family showed that the Aegilops orthologs of the CslF family members were located on the same homeologous group chromosomes as in barley. More precisely, homologs of the CslF6 genes of barley were present on chromosome 7U and those of CslF9 on chromosome 1U, while homologs of other CslF genes were located on chromosomes 2U and 5U (Table 2 and Supplementary Table 2). Previous results (Cseh et al., 2011, 2013) indicated that the chromosome 7H-mediated transfer of HvCslF6 gene from barley cv. Manas to wheat significantly increased the level of β-glucan in the grains. While the increase was statistically significant, it should be noted that the β-glucan level content of the 7H addition line was moderate compared to that of the parental barley genotype (Cseh et al., 2011). An explanation for the moderate increase in the β-glucan level of wheat–barley 7H addition line could be that the barley QTL represented on chromosome 7H is only a part of the genomic regions needed for a more efficient synthesis of β-glucan (Houston et al., 2014; Shu and Rasmussen, 2014) and it is not sufficient to drive higher β-glucan levels. This may also apply to the wheat–Aegilops addition lines 5Ug or 7Ug, 7Mg and 7Mb (and for 1Ub under field conditions) where the increase in the β-glucan amount was also moderate.
A homolog of HvCslF6 gene was also identified on chromosome 7U, which further supports the fact that the addition of group 7 chromosomes from Ae. geniculata and Ae. biuncialis to wheat increases the grain β-glucan level. A putative ortholog of the HvCslF7 gene was detected on chromosome 5U, with an increased β-glucan level in the wheat–Ae. geniculata 5Ug addition line. These results suggest that the variant of HvCslF7 may have a role in grain β-glucan synthesis of Aegilops (Burton et al., 2008).
However, further genome-wide association studies on a diverse population of Ae. geniculata, or Ae. biuncialis would help to identify the chromosomal locations of QTL responsible for high β-glucan levels in grain endosperm. The addition lines contain a whole homeologous chromosome pair from Aegilops and, beside the desirable genomic regions, they also contain many other genes which may affect grain development and composition. The elimination of excess alien chromatin by the production of wheat–Aegilops translocation lines containing desirable QTL and their pyramiding into one genotype could be used to increase β-glucan content.
Arabinoxylan
The observation that the addition of chromosomes 5Ug, 7Ug and 1Ub to wheat increases the total pentosan content, while the addition of chromosomes 3Ug, 4Ug, 5Ug, 6Ug, 7Ug, 5Mg, 7Mg, 2Mb and 7Mb increases the water-soluble pentosan fraction (Figures 3B,C) indicates that goatgrasses are a promising source of genes to increase the AX content of wheat. As well as their health benefits, both water-soluble and unsoluble AX result in higher water absorption, dough development time and loaf volume (Biliaderis et al., 1995; Courtin and Delcour, 2002). Soluble AX contributes to gas bubble formation during baking while insoluble AX destabilizes it (Courtin and Delcour, 2002).
Many efforts have been made to identify genes controlling the biosynthesis of AXs in wheat and several mapping populations have been used to identify QTL for AX in wheat. A major QTL was identified on chromosome 1B (Martinant et al., 1999), which explained 59% of the phenotypic variation in WE-AX content and viscosity (Charmet et al., 2009). Quraishi et al. (2011) identified seven loci (chromosomes 1B, 3A, 3D, 5B, 6B, 7A, and 7B) by association genetics, of which three (chromosomes 1B, 3D, and 6B) corresponded to consensus meta-QTL based on data from seven crosses. These authors also identified candidate genes for the future improvement of grain fiber. Using bioinformatic approaches, Mitchell et al. (2007) identified genes for AX synthesis in glycosyltransferase (GT) families 43, 47, and 61, while Zeng et al. (2010) identified GT75 with a combination of proteomics and transcriptomics analyses. In wheat, most GT genes occur in multiple forms, with three homeoalleles of each form being present on the A, B, and D genomes (Mitchell et al., 2007; Wan et al., 2008; Pellny et al., 2012). RNAi suppression of the expression of these homeoalleles demonstrated that GT43 and GT47 encode subunits of β-1,4-xylan synthase and that GT61 encodes a α-(1,3)-arabinosyltransferase (Anders et al., 2012; Lovegrove et al., 2013). Suppression of either TaGT43_2 or TaGT47 resulted in a 40–50% decrease in total AX but increased Araf residues substitution, with a 50% decrease in cell-wall thickness (Lovegrove et al., 2013). Similarly, the suppression of GT61 (renamed TaXAT1) resulted in a 70–80% decrease in the amount of α-(1,3) linked Araf in the AX of mature starchy endosperm (Anders et al., 2012). Decreases in extract viscosity were observed in all transgenic lines, with greater effects in the TaGT43_2 and TaGT47_2 RNAi lines (located on chromosomes 4ABD and 3ABD of wheat, respectively) than in the TaXAT1 RNAi lines (Freeman et al., 2016). These effects were explained by decreases in the amount and chain length of WE-AX.
Putative orthologs of wheat GT43, GT47 and GT61 and GT75 genes were identified on chromosomes 3U (TaGT47-2B, TaGT47-2D, TaGT47-2A, TaGT47-13), 4U (TaGT43-2B, TaGT43-2A, TaGT61-2), 6U (TaGT47-12, TaGT61-1, TaGT75-4), and 7U (TaGT43-4) in the U genome of Ae. umbellulata, which is consistent with the present observation that the addition of chromosome 7Ug increased total pentosan, while the addition of chromosomes 3Ug, 4Ug, 6Ug, and 7Ug increased WE-pentosan (Figures 3B,C, Table 2, and Supplementary Table 3). It should be noted that the addition of Ae. geniculata chromosome 5Ug increased the total- and WE-pentosan content in wheat even if no orthologs of wheat GT genes were identified on chromosome 5U. However, the increased AX content of the 5Ug addition line is consistent with a recent study by Marcotuli et al. (2015), who used GWAS to identify three QTL strongly associated with AX content on chromosome 5A of tetraploid wheat. The possible reason for this discrepancy could be that the chromosome survey sequences do not cover the whole of the Ae. umbellulata genome (data not shown).
The ratio of AXOS released by endoxylanase digestion was affected by Ae. biuncialis chromosomes 2Mb, 3Mb, and 7Mb in wheat, as their addition increased the ratio of monosubstituted to disubstituted AXOS. However, this increase in the M/D ratio was due to an increase in the amount of monosubstituted AXOS in addition line 2Mb, but due to a decrease in the amount of disubstituted AXOS in the addition lines 3Mb and 7Mb. These results indicate that AX biosynthesis could be modified by these Aegilops chromosomes. However, other genes were also observed to have smaller effects (Figure 5G). Interestingly, several enzymes involved in the AX biosynthesis pathway were identified on the group 2, 3, and 7 chromosomes of wheat (Group 2: glucuronosyltransferase, cis-zeatin O-glucosyltransferase 1, glycosyltransferase; group 3: glycosyl hydrolase; group 7: 1,3-β-D-glucan synthase, Glycosyl hydrolase, β-1,4-endoglucanase) (Marcotuli et al., 2015). The fact that the Ae. geniculata and Ae. biuncialis accessions exhibited significant phenotypic variations for the grain β-glucan and AX content supports the notion that these species have considerable genetic variability for improving the edible fiber content of bread wheat.
Conclusion
In conclusion, the present study highlighted the ability of Aegilops chromosomes 5Ug, 7Ug, 7Mg, and 7Mb to increase the β-glucan and 5Ug, 5Mg, and 7Mb to improve WE-AX content of hexaploid wheat grains. After the selection of suitable Aegilops crossing partners, these Aegilops chromosomes could be promising candidates for chromosome-mediated gene transfer and chromosome engineering programs aimed to improve the DF content of wheat. Furthermore, the chromosomal assignment of Aegilops orthologs for genes influencing grain β-glucan and AX content provides a foundation for further genome-wide association studies to identify QTL responsible for the amount and composition of edible fiber in these Aegilops species. The study will thus contribute to the more efficient use of wild wheat relatives in alien introgression breeding programs to obtain wheat varieties with increased fiber content, especially β-glucan and improved health benefits, in general.
Author Contributions
Plant materials were developed by MM-L, IM, ÉD, and AF. Cytogenetic checking was carried out by AF. Experimentation and data evaluation were carried out by MR, AL, and PS. Background for research was established by IM, PS, LL, and ZB. Sequence data of the U genome of Aegilops umbellulata were made available by JD. Blast searches were carried out by IM. Paper was written by MR, IM, AL, ÉD, and PS.
Funding
This research was funded by project No TAMOP-4.2.2.A-11/1/KONV-2012-0008, by the Hungarian National Research Fund [K112226, K116277, K112169], and by a János Bolyai Research Scholarship from the Hungarian Academy of Sciences (for MR and IM). JD received funding from the Ministry of Education, Youth and Sports of the Czech Republic (award LO1204 from the National Program of Sustainability I). Rothamsted Research receives grant-aided support from the Biotechnology and Biological Sciences Research Council (BBSRC) of the United Kingdom. The work at Rothamsted forms part of the Designing Future Wheat strategic program [BB/P016855/1].
Conflict of Interest Statement
The authors declare that the research was conducted in the absence of any commercial or financial relationships that could be construed as a potential conflict of interest.
Abbreviations
Araf, α-L-arabinofuranosyl; A/X, ratio of arabinose to xylose; AXOS, arabinoxylan oligosaccharides; D, (XA2+3XX) + (XA3A2+3X) + (XA3XA2+3XX) sum of disubstituted AXOS; DP, degree of polymerization; GOS, glucooligosaccharides; HPAEC, high-performance anion exchange chromatography; M, (XA3XX) + 2(XA3A3XX) + 2(XA3XA3XX) + (XA3A2+3X) + (XA3XA2+3XX) sum of monosubstituted AXOS; PAD, pulsed amperometric detection; TKW, thousand kernel weight; TOT, X + XX + XXX + XA3XX + XA3A3XX + XA3XA3XX + (XA2+3XX) + (XA3A2+3XX) + (XA3XA2+3XX) sum of all AXOS; TOT-AX, total arabinoxylan; US, X + XX + XXX sum of unsubstituted AXOS; WE-AX, water extractable arabinoxylan.
Acknowledgment
The authors are grateful to Dr. Rowan Mitchell (Rothamsted Research) for his contribution to Supplementary Table 3.
Supplementary Material
The Supplementary Material for this article can be found online at: http://journal.frontiersin.org/article/10.3389/fpls.2017.01529/full#supplementary-material
FIGURE 1 | Degradation products obtained after xylanase and lichenase treatment of flour samples and run on HPAEC-PAD [y-axis: PAD response (μV); x-axis: Time (min)].
FIGURE 2 | Thousand kernel weight and compositional properties of mature grains of two lines of bread wheat (cv. Chinese Spring and Mv9kr1 line), two Aegilops species (Ae. geniculata and Ae. biuncialis), and wheat–Aegilops chromosome addition lines grown on the field in 2012/2013 season. (a) thousand kernel weight, (b) protein, (c) β-glucan content, (d) TOT-pentosan, (e) WE-pentosan content. LSD, least significant difference; TOT, total; WE, water-extractable. ∗Significantly higher than the wheat (T. aestivum) control.
Footnotes
- ^www.jiffygroup.com
- ^http://olomouc.ueb.cas.cz/projects/Aegilops_umbellulata
- ^https://www.ncbi.nlm.nih.gov
- ^http://plants.ensembl.org/
References
AACC International (1995). Approved Methods 32-23.01. Beta-Glucan Content of Barley and Oats – Rapid Enzymatic Procedure. St. Paul, MN: AACC International.
Ahmadpoor, F., Asghari-Zakaria, R., Firoozi, B., and Shahbazi, H. (2014). Investigation of diversity in Aegilops biuncialis and Aegilops umbellulata by A-PAGE. Nat. Prod. Res. 28, 1626–1636. doi: 10.1080/14786419.2014.931392
Anders, N., Wilkinson, M. D., Lovegrove, A., Freeman, J., Tryfona, T., Pellny, T. K., et al. (2012). Glycosyl transferases in family 61 mediate arabinofuranosyl transfer onto xylan in grasses. Proc. Natl. Acad. Sci. U.S.A. 109, 989–993. doi: 10.1073/pnas.1115858109
Anderson, J. W., Baird, P., Davis, R. H. Jr., Ferreri, S., Knudtson, M., Koraym, A., et al. (2009). Health benefits of dietary fiber. Nutr. Rev. 67, 188–205. doi: 10.1111/j.1753-4887.2009.00189.x
Andersson, A. A. M., Kamal-Eldin, A., Fras, A., Boros, D., and Aman, P. (2008). Alkylresorcinols in wheat varieties in the HEALTHGRAIN diversity screen. J. Agric. Food Chem. 56, 9722–9725. doi: 10.1021/jf8011344
Bálint, A., Kovács, G., Erdei, L., and Sutka, J. (2001). Comparison of the Cu, Zn, Fe, Ca and Mg contents of the grains of wild, ancient and cultivated wheat species. Cereal Res. Commun. 29, 375–382.
Bamforth, C. W. (2010). The enzymology of cell wall breakdown during malting and mashing: An Overview. Tech. Q. Mast. Brew. Assoc. Am. 47, 309–321. doi: 10.1094/TQ-47-1-0309-01
Bandou, H., Rodriguez-Quijano, M., Carrillo, J. M., Branlard, G., Zaharieva, M., and Monneveux, P. (2009). Morphological and genetic variation in Aegilops geniculata from Algeria. Plant Syst. Evol. 277, 85–97. doi: 10.1007/s00606-008-0106-z
Batey, I. L., Gupta, R. B., and MacRitchie, F. (1991). Use of size-exclusion high performance liquid chromatography in the study of wheat flour proteins: an improved chromatographic procedure. Cereal Chem. 68, 207–209.
Bedford, M. R., and Schulze, H. (1998). Exogenous enzymes for pigs and poultry. Nutr. Res. Rev. 11, 91–114. doi: 10.1079/NRR19980007
Biliaderis, C. G., Izydorczyk, M. S., and Rattan, O. (1995). Effect of arabinoxylans on bread-making quality of wheat flours. Food Chem. 53, 165–171. doi: 10.1016/0308-8146(95)90783-4
Bonnand-Ducasse, M., Della Valle, G., Lefebvre, J., and Saulnier, L. (2010). Effect of wheat dietary fibres on bread dough development and rheological properties. J. Cereal Sci. 52, 200–206. doi: 10.1016/j.jcs.2010.05.006
Boros, D., Lukaszewski, A. J., Aniol, A., and Ochodzki, P. (2002). Chromosome location of genes controlling the content of dietary fibre and arabinoxylans in rye. Euphytica 128, 1–8. doi: 10.1023/A:1020639601959
Brennan, C. S., and Cleary, L. J. (2007). Utilisation Glucagel®in the β-glucan enrichment of breads A physicochemical and nutritional evaluation. Food Res. Int. 40, 291–296. doi: 10.1016/j.foodres.2006.09.014
Brouns, F. J. P. H., van Buul, V. J., and Shewry, P. R. (2013). Does wheat make us fat and sick? J. Cereal Sci. 58, 209–215. doi: 10.1016/j.jcs.2013.06.002
Buksa, K., Nowotna, A., and Ziobro, R. (2016). Application of cross-linked and hydrolyzed arabinoxylans in baking of model rye bread. Food Chem. 192, 991–996. doi: 10.1016/j.foodchem.2015.07.104
Burton, R. A., Jobling, S. A., Harvey, A. J., Shirley, N. J., Mather, D. E., Bacic, A., et al. (2008). The genetics and transcriptional profiles of the cellulose synthase-like HvCslF gene family in barley. Plant Physiol. 146, 1821–1833. doi: 10.1104/pp.107.114694
Burton, R. A., Wilson, S. M., Hrmova, M., Harvey, A. J., Shirley, N. J., Medhurst, A., et al. (2006). Cellulose synthase-like CslF genes mediate the synthesis of cell wall (1,3;1,4)-beta-D-glucans. Science 311, 1940–1942. doi: 10.1126/science.1122975
Buttriss, J. L., and Stokes, C. S. (2008). Dietary fibre and health: an overview. Nutr. Bull. 33, 186–200. doi: 10.1111/j.1467-3010.2008.00705.x
Cavallero, A., Empilli, S., Brighenti, F., and Stanca, A. M. (2002). High (1-3, 1-4)-β-glucan fractions in bread making and their effect on human glycemic response. J. Cereal Sci. 36, 59–66. doi: 10.1006/jcrs.2002.0454
Charmet, G., Masood-Quraishi, U., Ravel, C., Romeuf, I., Rakszegi, M., Guillon, F., et al. (2009). Genetics of dietary fibre in bread wheat. Euphytica 170, 155–168. doi: 10.1007/s10681-009-0019-0
Cleary, L. J., Andersson, R., and Brennan, C. S. (2007). The behaviour and susceptibility to degradation of high and low molecular weight barley β-glucan in wheat bread during baking and in vitro digestion. Food Chem. 102, 889–897. doi: 10.1016/j.foodchem.2006.06.027
Colmer, T. D., Flowers, T. J., and Munns, R. (2006). Use of wild relatives to improve salt tolerance in wheat. J. Exp. Bot. 57, 1059–1078. doi: 10.1093/jxb/erj124
Courtin, C. M., and Delcour, J. (1998). Physicochemical and bread-making properties of low molecular weight wheat-derived Arabinoxylans. J. Agric. Food Chem. 46, 4066–4073. doi: 10.1021/jf980339t
Courtin, C. M., and Delcour, J. (2002). Arabinoxylans and endoxylanases in wheat flour bread-making. J. Cereal Sci. 35, 225–243. doi: 10.1006/jcrs.2001.0433
Cseh, A., Kruppa, K., Molnár, I., Rakszegi, M., Doležel, J., and Molnár-Láng, M. (2011). Characterization of a new 4BS.7HL wheat/barley translocation line using GISH, FISH and SSR markers and its effect on the β-glucan content of wheat. Genome 54, 795–804. doi: 10.1139/g11-044
Cseh, A., Soós, V., Rakszegi, M., Türkösi, E., Balázs, E., and Molnár-Láng, M. (2013). Expression of HvCslF9 and HvCslF6 barley genes in the genetic background of wheat and their influence on the wheat β-glucan content. Ann. Appl. Biol. 163, 142–150. doi: 10.1111/aab.12043
Cui, W., and Wood, P. J. (2000). “Relationships between structural features, molecular weight and rheological properties of cereal beta-D- glucans,” in Hydrocolloids, PT 1: Physical Chemistry and Industrial Application of Gels, Polysaccharides and Proteins, ed. K. Nishinari (Amsterdam: Elsevier), 159–168.
Cui, W., Wood, P. J., Blackwell, B., and Nikiforuk, J. (2000). Physicochemical properties and structural characterization by two-dimensional NMR spectroscopy of wheat β-D-glucan—comparison with other cereal β-D-glucans. Carbohydr. Polym. 41, 249–258. doi: 10.1016/S0144-8617(99)00143-5
Cyran, M., Rakowska, M., and Miazga, D. (1996). Chromosomal location of factors affecting content and composition of nonstarch polysaccharides in wheat-rye addition lines. Euphytica 89, 153–157. doi: 10.1007/BF00015732
Dai, S. F., Zhao, L., Xue, X. F., Jia, Y. N., Liu, D. C., Pu, Z. J., et al. (2015). Analysis of high-molecular-weight glutenin subunits in five amphidiploids and their parental diploid species Aegilops umbellulata and Aegilops uniaristata. Plant Genet. Resour. 13, 186–189. doi: 10.1017/S1479262114000719
Doblin, M. S., Pettolino, F., and Bacic, A. (2010). Plant cell walls: the skeleton of the plant world. Funct. Plant Biol. 37, 357–381. doi: 10.1071/FP09279
Doblin, M. S., Pettolino, F. A., Wilson, S. M., Campbell, R., Burton, R. A., Fincher, G. B., et al. (2009). A barley cellulose synthase-like CSLH gene mediates (1,3;1,4)-beta-D-glucan synthesis in transgenic Arabidopsis. Proc. Natl. Acad. Sci. U.S.A. 106, 5996–6001. doi: 10.1073/pnas.0902019106
Doležel, J., Vrána, J., Cápal, P., Kubaláková, M., Burešová, V., and Šimková, H. (2014). Advances in plant chromosome genomics. Biotechnol. Adv. 32, 122–136. doi: 10.1016/j.biotechadv.2013.12.011
Douglas, S. G. (1981). A rapid method for the determination of pentosans in wheat flour. Food Chem. 7, 139–145. doi: 10.1016/0308-8146(81)90059-5
Dulai, S., Molnár, I., Szopkó, D., Darkó,É, Vojtkó, A., Sass-Gyarmati, A., et al. (2014). Wheat-Aegilops biuncialis amphiploids have efficient photosynthesis and biomass production during osmotic stress. J. Plant Physiol. 171, 509–517. doi: 10.1016/j.jplph.2013.11.015
Farkas, A., Molnár, I., Dulai, S., Rapi, S., Oldal, V., Cseh, A., et al. (2014). Increased micronutrient content (Zn, Mn) in the 3Mb(4B) wheat-Aegilops biuncialis substitution and 3Mb.4BS translocation identified by GISH and FISH. Genome 57, 61–67. doi: 10.1139/gen-2013-0204
Finnie, S. M., Bettge, A. D., and Morris, C. F. (2006). Influence of cultivar and environment on water-soluble and water-insoluble arabinoxylans in soft wheat. Cereal Chem. 83, 617–623. doi: 10.1094/CC-83-0617
Frederix, S. A., Van Hoeymissen, K., Courtin, C. M., and Delcour, J. A. (2004). Water-extractable and water-unextractable arabinoxylans affect gluten agglomeration behavior during wheat flour gluten-starch separation. J. Agric. Food Chem. 52, 7950–7956. doi: 10.1021/jf049041v
Freeman, J., Lovegrove, A., Wilkinson, M. D., Saulnier, L., Shewry, P. R., and Mitchell, R. A. C. (2016). Effect of suppression of arabinoxylan synthetic genes in wheat endosperm on chain length of arabinoxylan and extract viscosity. Plant Biotechnol. J. 14, 109–116. doi: 10.1111/pbi.12361
Friebe, B., Jiang, J., Raupp, W. J., McIntosh, R. A., and Gill, B. S. (1996). Characterization of wheat alien translocations conferring resistance to diseases and pests: current status. Euphytica 71, 59–87. doi: 10.1007/BF00035277
Friebe, B., Tuleen, N. A., and Gill, B. S. (1999). Development and identification of a complete set of Triticum aestivum–Ae. geniculata chromosome addition lines. Genome 42, 374–380. doi: 10.1139/gen-42-3-374
Garg, M., Tsujimoto, H., Gupta, R. K., Kumar, A., Kaur, N., Kumar, R., et al. (2016). Chromosome specific substitution lines of Aegilops geniculata alter parameters of bread making quality of wheat. PLoS ONE 11:e0162350. doi: 10.1371/journal.pone.0162350
Gebruers, K., Dornez, E., Bedo, Z., Rakszegi, M., Fras, A., Boros, D., et al. (2010). Environment and genotype effects on the content of dietary fiber and its components in wheat in the HEALTHGRAIN diversity screen. J. Agric. Food Chem. 58, 9353–9361. doi: 10.1021/jf100447g
Gebruers, K., Dornez, E., Boros, D., Fras, A., Dynkowska, W., Bedo, Z., et al. (2008). Variation in the content of dietary fiber and components thereof in wheats in the HEALTHGRAIN diversity screen. J. Agric. Food Chem. 56, 9740–9749. doi: 10.1021/jf800975w
Heinio, R. L., Noort, M. W. J., Katina, K., Alam, S. A., Sozer, N., de Kock, H. L., et al. (2016). Sensory characteristics of wholegrain and bran-rich cereal foods - A review. Trends Food Sci. Technol. 47, 25–38. doi: 10.1016/j.tifs.2015.11.002
Hesselman, K., Elwinger, K., Nilsson, M., and Thomke, S. (1981). The effect of beta-glucanase supplementation, stage of ripeness, and storage treatment of barley in diets fed to broiler chickens. Poult. Sci. 60, 2664–2671. doi: 10.3382/ps.0602664
Hoffmann, R. A., Leeflang, B. R., de Barse, M. M. J., Kamerling, J. P., and Vliegenthart, J. F. G. (1991). Characterisation of 1H-N.M.R. spectroscopy of oligosaccharides, derived from arabinoxylans of white endosperm of wheat, that contain the elements -4) [α-L-Araf-(1-3)]-β-D-Xylp-(1-or-4)[α-L-Araf-(1-2)][α-L-Araf-(1-3)]-β-D-Xylp-(1-. Carbohydr. Res. 221, 63–81. doi: 10.1016/0008-6215(91)80049-S
Houston, K., Russell, J., Schreiber, M., Halpin, C., Oakey, H., Washington, J. M., et al. (2014). A genome wide association scan for (1,3;1,4)-β-glucan content in the grain of contemporary 2-row spring and winter barleys. BMC Genomics 15:907. doi: 10.1186/1471-2164-15-907
Igartua, E., Hayes, P. M., Thomas, W. T. B., Meyer, R., and Mather, D. E. (2002). Genetic control of quantitative grain and malt quality traits in barley. J. Crop Prod. 5, 131–164. doi: 10.1300/J144v05n01_06
International Association for Cereal Science and Technology ICC 105/2 (1995). Determination of Crude Protein in Cereals and Cereal Products for Food and for Feed. Vienna: International Association for Cereal Science and Technology.
International Association for Cereal Science and Technology ICC 166 (1998). Determination of β-glucan in Barley, Oat and Rye. Vienna: International Association for Cereal Science and Technology.
Izydorczyk, M. S., and Biliaderis, C. G. (1994). Studies on the structure of wheat-endosperm arabinoxylans. Carbohydr. Polym. 24, 61–71. doi: 10.1016/0144-8617(94)90118-X
Izydorczyk, M. S., and Dexter, J. E. (2008). Barley β-glucans and arabinoxylans: Molecular structure, physicochemical properties, and uses in food products – a Review. Food Res. Int. 41, 850–868. doi: 10.1016/j.foodres.2008.04.001
Jiang, G., and Vasanthan, T. (2000). MALDI-MS and HPLC quantification of oligosaccharides of lichenase-hydrolyzed water-soluble b-glucan from ten barley varieties. J. Agric. Food Chem. 48, 3305–3310. doi: 10.1021/jf0001278
Jones, J. M., Adams, J., Harriman, C., Miller, C., and Van der Kamp, J. W. (2015). Nutritional impacts of different whole grain milling techniques: a review of milling practices and existing data. Cereal Foods World 60, 130–139. doi: 10.1094/CFW-60-3-0130
Kozub, N. A., Sozinov, I. A., Xynias, I. N., and Sozinov, A. A. (2011). Allelic variation at high-molecular-weight glutenin subunit loci in Aegilops biuncialis Vis. Russ. J. Genet. 47, 1078–1083. doi: 10.1134/S1022795411090092
Lafiandra, D., Riccardi, G., and Shewry, P. R. (2014). Improving cereal grain carbohydrates for diet and health. J. Cereal Sci. 59, 312–326. doi: 10.1016/j.jcs.2014.01.001
Larroque, O. R., and Békés, F. (2000). Rapid size-exclusion chromatography analysis of molecular size distribution for wheat endosperm protein. Cereal Chem. 77, 451–453. doi: 10.1094/CCHEM.2000.77.4.451
Lazaridou, A., and Biliaderis, C. G. (2007). Molecular aspects of cereal β-glucan functionality: physical properties, technological applications and physiological effects. J. Cereal Sci. 46, 101–118. doi: 10.1016/j.jcs.2007.05.003
Lazaridou, A., Biliaderis, C. G., and Izydorczyk, M. S. (2003). Molecular size effects on rheological properties of oat beta-glucans in solution and gels. Food Hydrocoll. 17, 693–712. doi: 10.1016/S0268-005X(03)00036-5
Lazaridou, A., Biliaderis, C. G., Micha-Screttas, M., and Steele, B. R. (2004). A comparative study on structure-function relations of mixed linkage (1-3), (1-4) linear β-D-glucans. Food Hydrocoll. 18, 837–855. doi: 10.1016/j.foodhyd.2004.01.002
Li, S., Morris, C. F., and Bettge, A. D. (2009). Genotype and environment variation for arabinoxylans in hard winter and spring wheats of the US Pacific Northwest. Cereal Chem. 86, 88–95. doi: 10.1094/CCHEM-86-1-0088
Lovegrove, A., Wilkinson, M. D., Freeman, J., Pellny, T. K., Tosi, P., Saulnier, L., et al. (2013). RNA interference suppression of genes in glycosyl transferase families 43 and 47 in wheat starchy endosperm causes large decreases in arabinoxylan content. Plant Physiol. 163, 95–107. doi: 10.1104/pp.113.222653
Marcotuli, I., Houston, K., Schwerdt, J. G., Waugh, R., Fincher, G. B., Burton, R. A., et al. (2016). Genetic diversity and genome wide association study of β-glucan content in tetraploid wheat grains. PLoS ONE 11:e0152590. doi: 10.1371/journal.pone.0152590
Marcotuli, I., Houston, K., Waugh, R., Fincher, G. B., Burton, R. A., Blanco, A., et al. (2015). Genome wide association mapping for arabinoxylan content in a collection of tetraploid wheats. PLoS ONE 10:e0132787. doi: 10.1371/journal.pone.0132787
Mares, D. J., and Stone, B. A. (1973). Studies on wheat endosperm. I. Chemical composition and ultrastructure of the cell walls. Aust. J. Biol. Sci. 26, 793–812. doi: 10.1071/BI9730793
Margulies, M., Egholm, M., Altman, W. E., Attiya, S., Bader, J. S., Bemben, L. A., et al. (2005). Genome sequencing in microfabricated high-density picolitre reactors. Nature 437, 376–380. doi: 10.1038/nature03959
Martinant, J. P., Billot, A., Bouguennec, A., Charmet, G., Saulnier, L., and Branlard, G. (1999). Genetic and environmental variations in water-extractable arabinoxylans content and flour extract viscosity. J. Cereal Sci. 30, 45–48. doi: 10.1006/jcrs.1998.0259
Martis, M. M., Zhou, R., Haseneyer, G., Schmutzer, T., Vrána, J., Kubaláková, M., et al. (2013). Reticulate evolution of the rye genome. Plant Cell 25, 3685–3698. doi: 10.1105/tpc.113.114553
Mayer, K. F. X., Martis, M., Hedley, P. E., Šimková, H., Liu, H., Morris, J. A., et al. (2011). Unlocking the barley genome by chromosomal and comparative genomics. Plant Cell 23, 1249–1263. doi: 10.1105/tpc.110.082537
McIntosh, G. H., Whyte, J., McArthur, R., and Nestel, P. J. (1991). Barley and wheat foods – Influence on plasma-cholesterol concentrations in hypercholesterolemic men. Am. J. Clin. Nutr. 53, 1205–1209.
Medouri, A., Bellil, I., and Khelifi, D. (2015). Polymorphism at high molecular weight glutenin subunits and morphological diversity of Aegilops geniculata Roth collected in Algeria. Cereal Res. Commun. 43, 272–283. doi: 10.1556/CRC.2014.0042
Mikó, P., Löschenberger, F., Hiltbrunner, J., Aebi, R., Megyeri, M., Kovács, G., et al. (2014). Comparison of bread wheat varieties with different breeding origin under organic and low input management. Euphytica 199, 69–80. doi: 10.1016/j.dib.2016.04.065
Miller, S. S., Wood, P. J., Pietrzak, L. N., and Fulcher, R. G. (1993). Mixed linkage beta glucans, protein content, and kernel weight in Avena species. Cereal Chem. 70, 231–233.
Mitchell, R. A. C., Dupree, P., and Shewry, P. R. (2007). A novel bioinformatics approach identifies candidate genes for the synthesis and feruloylation of arabinoxylan. Plant Physiol. 144, 43–53. doi: 10.1104/pp.106.094995
Molina-Cano, J. L., Moralejo, M., Elia, M., Munoz, P., Russell, J. R., Perez-Vendrell, A. M., et al. (2007). QTL analysis of a cross between European and North American malting barleys reveals a putative candidate gene for beta-glucan content on chromosome 1H. Mol. Breed. 19, 275–284. doi: 10.1007/s11032-006-9075-5
Molnár, I., Gáspár, L., Sárvári,É, Dulai, S., Hoffmann, B., Molnár-Láng, M., et al. (2004). Physiological and morphological responses to water stress in Aegilops biuncialis and Triticum aestivum genotypes with differing tolerance to drought. Funct. Plant Biol. 31, 1149–1159. doi: 10.1071/FP03143
Molnár, I., Vrána, J., Burešová, V., Cápal, P., Farkas, A., Darkó, É, et al. (2016). Dissecting the U, M, S and C genomes of wild relatives of bread wheat (Aegilops spp.) into chromosomes and exploring their synteny with wheat. Plant J. 88, 452–467. doi: 10.1111/tpj.13266
Molnár-Láng, M., Linc, G., Nagy, E. D., Schneider, A., and Molnár, I. (2002). Molecular cytogenetic analysis of wheat-alien hybrids and derivatives. Acta Agron. Hung. 50, 303–311. doi: 10.1556/AAgr.50.2002.3.8
Molnár-Láng, M., Linc, G., and Sutka, J. (1996). Transfer of the recessive crossability allele kr1 from Chinese Spring into the winter wheat variety Martonvásári 9. Euphytica 90, 301–305. doi: 10.1007/BF00027480
Morales-Ortega, A., Carvajal-Millan, E., Lopez-Franco, Y., Rascon-Chu, A., Lizardi- Mendoza, J., Torres-Chavez, P., et al. (2013). Characterization of water extractable arabinoxylans from a spring wheat flour: rheological properties and microstructure. Molecules 18, 8417–8428. doi: 10.3390/molecules18078417
MSZ 6367/4-86 (1987). Edible, Fodder and Industrial Seeds and Husked Products. Determination of Test Weight, Thousand Kernel Weight and Classification Grade. Budapest: Hungarian Standards Institution. Available at: www.mszt.hu
Nemeth, C., Freeman, J., Jones, H. D., Sparks, C., Pellny, T. K., Wilkinson, M. D., et al. (2010). Downregulation of the CSLF6 gene results in decreased (1,3;1,4)-β-D-glucan in endosperm of wheat. Plant Physiol. 152, 1209–1218. doi: 10.1104/pp.109.151712
Noort, M. W. J., van Haaster, D., Hemery, Y., Schols, H. A., and Hamer, R. J. (2010). The effect of particle size of wheat bran fractions on bread quality - Evidence for fibre protein interactions. J. Cereal Sci. 52, 59–64. doi: 10.1016/j.jcs.2010.03.003
Ordaz-Ortiz, J. J., Devaux, M. F., and Saulnier, L. (2005). Classification of wheat varieties based on structural features of arabinoxylans as revealed by endoxylanase treatment of flour and grain. J. Agric. Food Chem. 53, 8349–8356. doi: 10.1021/jf050755v
Ordaz-Ortiz, J. J., Guillon, F., Tranquet, O., Dervilly-Pinel, G., Tran, V., and Saulnier, L. (2004). Specificity of monoclonal antibodies generated against arabinoxylans of cereal grains. Carbohydr. Polym. 57, 425–433. doi: 10.1016/j.carbpol.2004.05.016
Ordaz-Ortiz, J. J., and Saulnier, L. (2005). Structural variability of arabinoxylans from wheat flour. Comparison of water-extractable and xylanase-extractable arabinoxylans. J. Cereal Sci. 42, 119–125. doi: 10.1016/j.jcs.2004.02.004
Payne, P. I. (1987). Genetics of wheat storage proteins and the effect of allelic variation on bread-making quality. Annu. Rev. Plant Physiol. 38, 141–153. doi: 10.1146/annurev.pp.38.060187.001041
Pellny, T. K., Lovegrove, A., Freeman, J., Tosi, P., Love, C. G., Knox, J. P., et al. (2012). Cell walls of developing wheat starchy endosperm: comparison of composition and RNA-Seq transcriptome. Plant Physiol. 158, 612–627. doi: 10.1104/pp.111.189191
Pirgozliev, V., Rose, S. P., Pellny, T., Amerah, A. M., Wickramasinghe, M., Ulker, M., et al. (2015). Energy utilization and growth performance of chickens fed novel wheat inbred lines selected for different pentosan levels with and without xylanase supplementation. Poult. Sci. 94, 232–239. doi: 10.3382/ps/peu059
Quraishi, U. M., Murat, F., Abrouk, M., Pont, C., Confolent, C., Oury, F. X., et al. (2011). Combined meta-genomics analyses unravel candidate genes for the grain dietary fiber content in bread wheat (Triticum aestivum L.). Funct. Integr. Genomics 11, 71–83. doi: 10.1007/s10142-010-0183-2
Rakszegi, M., Lang, L., Bedo, Z., and Shewry, P. R. (2008). Composition and end-use quality of 150 wheat lines selected for the HEALTHGRAIN diversity screen. J. Agric. Food Chem. 56, 9750–9757. doi: 10.1021/jf8009359
Rawat, N., Tiwari, V. K., Singh, N., Randhawa, G. S., Singh, K., Chhuneja, P., et al. (2009). Evaluation and utilization of Aegilops and wild Triticum species for enhancing iron and zinc content in wheat. Genet. Resour. Crop Evol. 56, 53–64. doi: 10.1007/s10722-008-9344-8
Rekika, D., Monneveux, P., and Havaux, M. (1997). The in vivo tolerance of photosynthetic membranes to high and low temperatures in cultivated and wild wheats of the Triticum and Aegilops genera. J. Plant Physiol. 150, 734–738. doi: 10.1016/S0176-1617(97)80291-X
Renard, C. M. G. C., Rouau, X., and Thibault, J. F. (1990). Structure and properties of water-soluble pentosans from wheat flour. Sci. Aliments 10, 283–292.
Rey, E., Molnár, I., and Doležel, J. (2015). “Genomics of wild relatives and alien introgressions,” in Alien Introgression in Wheat, eds M. Molnár-Láng, C. Ceoloni, and J. Doležel (Cham: Springer International Publishing), 347–381.
Saulnier, L., Robert, P., Grintchenko, M., Jamme, F., Bouchet, B., and Guillon, F. (2009). Wheat endosperm cell walls: spatial heterogeneity of polysaccharide structure and composition using micro-scale enzymatic fingerprinting and FT-IR microspectroscopy. J. Cereal Sci. 50, 312–317. doi: 10.1016/j.jcs.2009.05.003
Saulnier, L., Sado, P. E., Branlard, G., Charmet, G., and Guillon, F. (2007). Wheat arabinoxylans: exploiting variation in amount and composition to develop enhanced varieties. J. Cereal Sci. 46, 261–281. doi: 10.1016/j.jcs.2007.06.014
Schneider, A., Linc, G., Molnár, I., and Molnár-Láng, M. (2005). Molecular cytogenetic characterization of Aegilops biuncialis and its use for the identification of five derived wheat-Aegilops biuncialis disomic addition lines. Genome 48, 1070–1082. doi: 10.1139/g05-062
Schneider, A., Molnár, I., and Molnár-Láng, M. (2008). Utilisation of Aegilops (goatgrass) species to widen the genetic diversity of cultivated wheat. Euphytica 163, 1–19. doi: 10.1007/s10681-007-9624-y
Schooneveld-Bergmans, M. E. F., Beldman, G., and Voragen, A. G. J. (1999). Structural features of (glucurono) arabinoxylans extracted from wheat bran by barium hydroxide. J. Cereal Sci. 29, 63–75. doi: 10.1006/jcrs.1998.0222
Schreiber, M., Wright, F., MacKenzie, K., Hedley, P. E., Schwerdt, J. G., Little, A., et al. (2014). The barley genome sequence assembly reveals three additional members of the CslF (1,3;1,4)-β-Glucan synthase gene family. PLoS ONE 9:e90888. doi: 10.1371/journal.pone.0090888
Shewry, P. R., D’Ovidio, R., Lafiandra, D., Jenkins, J. A., Mills, E. N. C., and Bekes, F. (2009). “Wheat grain proteins,” in Wheat: Chemistry and Technology, eds K. Khan and P. R. Shewry (St. Paul, MN: AACC), 223–298. doi: 10.1094/9781891127557.008
Shewry, P. R., Freeman, J., Wilkinson, M., Pellny, T., and Mitchell, R. A. C. (2010a). “Challenges and opportunities for using wheat for biofuel production,” in Energy Crops, eds N. Halford and A. Karp (London: Royal Society of Chemistry), 13–26.
Shewry, P. R., Piironen, V., Lampi, A. M., Edelmann, M., Kariluoto, S., Nurmi, T., et al. (2010b). The HEALTHGRAIN wheat diversity screen: effects of genotype and environment on phytochemicals and dietary fiber components. J. Agric. Food Chem. 58, 9291–9298. doi: 10.1021/jf100039b
Shewry, P. R., Saulnier, L., Guillon, F., Gebruers, K., Courtin, C., Delcour, J., et al. (2010c). “Improving the benefits of wheat as a source of dietary fibre,” in Dietary Fibre: New Frontiers for Food and Health, eds J. W. van der Kamp, J. Jones, B. McCleary, and D. Topping (Wageningen: Wageningen Academic Publishers), 65–78.
Shewry, P. R., Halford, N. G., and Lafiandra, D. (2003a). “The genetics of wheat gluten proteins,” in Advances in Genetics, Vol. 49, eds J. C. Hall, J. C. Dunlap, and T. Friedman (Cambridge, MA: Academic Press), 111–184.
Shewry, P. R., Halford, N. G., Tatham, A. S., Popineau, Y., Lafiandra, D., and Belton, P. (2003b). The high molecular weight subunits of wheat glutenin and their role in determining wheat processing properties. Adv. Food Nutr. Res. 45, 221–302. doi: 10.1016/S1043-4526(03)45006-7
Shewry, P. R., Li, L., Piironen, V., Lampi, A. M., Nystrom, L., Rakszegi, M., et al. (2008). Phytochemicals and fiber components in oat varieties in the HEALTHGRAIN diversity screen. J. Agric. Food Chem. 56, 9777–9784. doi: 10.1021/jf801880d
Shewry, P. R., Tatham, A. S., Forde, J., Kreis, M., and Miflin, B. J. (1986). The classification and nomenclature of wheat gluten proteins: a reassessment. J. Cereal Sci. 4, 97–106. doi: 10.1016/S0733-5210(86)80012-1
Shu, X. L., and Rasmussen, S. K. (2014). Quantification of amylose, amylopectin, and beta-glucan in search for genes controlling the three major quality traits in barley by genome-wide association studies. Front. Plant Sci. 5:197. doi: 10.3389/fpls.2014.00197
Šimková, H., Svensson, J. T., Condamine, P., Hřibová, E., Suchánková, P., Bhat, P. R., et al. (2008). Coupling amplified DNA from flow-sorted chromosomes to high-density SNP mapping in barley. BMC Genomics 9:294. doi: 10.1186/1471-2164-9-294
Skendi, A., Papageorgiou, M., and Biliaderis, C. G. (2009). Effect of barley β-glucan molecular size and level on wheat dough rheological properties. J. Food Eng. 91, 594–601. doi: 10.1016/j.jfoodeng.2008.10.009
Storsley, J. M., Izydorczyk, M. S., You, S., Biliaderis, C. G., and Rossnagel, B. (2003). Structure and physicochemical properties of b-glucans and arabinoxylans isolated from hull-less barley. Food Hydrocoll. 17, 831–844. doi: 10.1016/S0268-005X(03)00104-8
Symons, L. J., and Brennan, C. S. (2004). The influence of a (1-3, 1-4)-β-D-glucan rich fraction on the physico-chemical properties and in vitro reducing sugar release of white wheat breads. J. Food Sci. 69, 463–467. doi: 10.1111/j.1365-2621.2004.tb10989.x
Taketa, S., Yuo, T., Tonooka, T., Tsumuraya, Y., Inagaki, Y., Haruyama, N., et al. (2012). Functional characterization of barley betaglucanless mutants demonstrates a unique role for CslF6 in (1,3;1,4)-β-D-glucan biosynthesis. J. Exp. Bot. 63, 381–392. doi: 10.1093/jxb/err285
The International Wheat Genome Sequencing Consortium [IWGSC] (2014). A chromosome-based draft sequence of the hexaploid bread wheat (Triticum aestivum) genome. Science 345:1251788. doi: 10.1126/science.1251788
Tighe, P., Duthie, G., Vaughan, N., Brittenden, J., Simpson, W. G., Duthie, S., et al. (2010). Effect of increased consumption of whole-grain foods on blood pressure and other cardiovascular risk markers in healthy middle-aged persons: a randomized controlled trial. Am. J. Clin. Nutr. 92, 733–740. doi: 10.3945/ajcn.2010.29417
Tiwari, V. K., Wang, S., Danilova, T., Koo, D. H., Vrána, J., Kubaláková, M., et al. (2015). Exploring the tertiary gene pool of bread wheat: sequence assembly and analysis of chromosome 5Mg of Aegilops geniculata. Plant J. 84, 733–746. doi: 10.1111/tpj.13036
Toole, G. A., Le Gall, G., Colquhoun, I. J., Nemeth, C., Saulnier, L., Lovegrove, A., et al. (2010). Temporal and spatial changes in cell wall composition in developing grains of wheat cv. Hereward. Planta 232, 677–689. doi: 10.1007/s00425-010-1199-5
Vaikousi, H., Biliaderis, C. G., and Izydorczyk, M. S. (2004). Solution flow behaviour and gelling properties of water- soluble barley (1-3, 1-4)-beta-glucans varying in molecular size. J. Cereal Sci. 39, 119–137. doi: 10.1016/j.jcs.2003.09.001
van Slageren, M. W. (1994). Wild Wheats: a Monograph of Aegilops L. and Amblyopyrum (Jaub and Spach) Eig (Poaceae). Wageningen: Wageningen Agricultural University Papers.
Wan, Y., Poole, R. L., Huttly, A. K., Toscano-Underwood, C., Feeney, K., Welham, S., et al. (2008). Transcriptome analysis of grain development in hexaploid wheat. BMC Genomics 9:121. doi: 10.1186/1471-2164-9-121
Wang, S. L., Chen, D., Guo, G. F., Zhang, T., Jiang, S. S., and Shen, X. X. (2012). Molecular characterization of LMW-GS genes from C, N, U and S-s genomes among Aegilops species. Cereal Res. Commun. 40, 542–551. doi: 10.1007/s00122-010-1354-1
Wang, Y. J., Wang, C. Y., Zhang, H., Li, H., Liu, X. L., and Ji, W. Q. (2015). Identification and evaluation of disease resistance and HMW-GS composition of Aegilops geniculata Roth. Genet. Resour. Crop Evol. 62, 1085–1093. doi: 10.1007/s10722-015-0217-7
Ward, J. L., Poutanen, K., Gebruers, K., Piironen, V., Lampi, A. M., Nystrom, L., et al. (2008). The HEALTHGRAIN cereal diversity screen: concept, results and prospects. J. Agric. Food Chem. 56, 9699–9709. doi: 10.1021/jf8009574
Wood, P. J. (2007). Cereal β-glucans in diet and health. J. Cereal Sci. 46, 230–238. doi: 10.1016/j.jcs.2007.06.012
Wood, P. J., Weisz, J., Beer, M. U., Newman, C. W., and Newman, R. K. (2003). Structure of (1-3)(1-4)-β-D-glucan in waxy and nonwaxy barley. Cereal Chem. 80, 329–332. doi: 10.1094/CCHEM.2003.80.3.329
Zaharieva, M., Gaulin, E., Havaux, M., Acevedo, E., and Monneveux, P. (2001a). Drought and heat responses in the wild wheat relative Aegilops geniculata Roth. Crop Sci. 41, 1321–1329. doi: 10.2135/cropsci2001.4141321x
Zaharieva, M., Monneveux, P., Henry, M., Rivoal, R., Valkoun, J., and Nachit, M. M. (2001b). Evaluation of a collection of wild wheat relative Aegilops geniculata Roth and identification of potential sources for useful traits. Euphytica 119, 33–38. doi: 10.1023/A:1017500728227
Zeng, W., Jiang, N., Nadella, R., Killen, T. L., Nadella, V., and Faik, A. A. (2010). Glucurono(arabino)xylan synthase complex from wheat contains members of the GT43, GT47, and GT75 families and functions cooperatively. Plant Physiol. 154, 78–97. doi: 10.1104/pp.110.159749
Keywords: wheat, Aegilops, dietary fiber, β-glucan, arabinoxylan, U and M genomes
Citation: Rakszegi M, Molnár I, Lovegrove A, Darkó É, Farkas A, Láng L, Bedő Z, Doležel J, Molnár-Láng M and Shewry P (2017) Addition of Aegilops U and M Chromosomes Affects Protein and Dietary Fiber Content of Wholemeal Wheat Flour. Front. Plant Sci. 8:1529. doi: 10.3389/fpls.2017.01529
Received: 27 February 2017; Accepted: 21 August 2017;
Published: 06 September 2017.
Edited by:
Soren K. Rasmussen, University of Copenhagen, DenmarkReviewed by:
Rachel Burton, University of Adelaide, AustraliaHakan Ozkan, Çukurova University, Turkey
Copyright © 2017 Rakszegi, Molnár, Lovegrove, Darkó, Farkas, Láng, Bedő, Doležel, Molnár-Láng and Shewry. This is an open-access article distributed under the terms of the Creative Commons Attribution License (CC BY). The use, distribution or reproduction in other forums is permitted, provided the original author(s) or licensor are credited and that the original publication in this journal is cited, in accordance with accepted academic practice. No use, distribution or reproduction is permitted which does not comply with these terms.
*Correspondence: Marianna Rakszegi, cmFrc3plZ2kubWFyaWFubkBhZ3Jhci5tdGEuaHU=
†These authors are equal first authors.