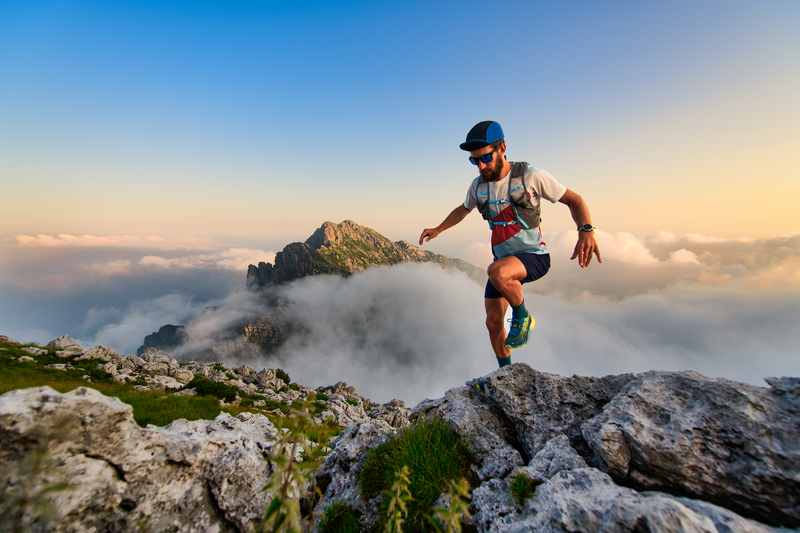
95% of researchers rate our articles as excellent or good
Learn more about the work of our research integrity team to safeguard the quality of each article we publish.
Find out more
MINI REVIEW article
Front. Plant Sci. , 26 July 2017
Sec. Plant Cell Biology
Volume 8 - 2017 | https://doi.org/10.3389/fpls.2017.01313
This article is part of the Research Topic Plastid proteostasis: relevance of transcription, translation and post-translational modifications View all 10 articles
Cytochromes c are hemoproteins, with the prosthetic group covalently linked to the apoprotein, which function as electron carriers. A class of cytochromes c is defined by a CXXCH heme-binding motif where the cysteines form thioether bonds with the vinyl groups of heme. Plastids are known to contain up to three cytochromes c. The membrane-bound cytochrome f and soluble cytochrome c6 operate in photosynthesis while the activity of soluble cytochrome c6A remains unknown. Conversion of apo- to holocytochrome c occurs in the thylakoid lumen and requires the independent transport of apocytochrome and heme across the thylakoid membrane followed by the stereospecific attachment of ferroheme via thioether linkages. Attachment of heme to apoforms of plastid cytochromes c is dependent upon the products of the CCS (for cytochrome c synthesis) genes, first uncovered via genetic analysis of photosynthetic deficient mutants in the green alga Chlamydomonas reinhardtii. The CCS pathway also occurs in cyanobacteria and several bacteria. CcsA and CCS1, the signature components of the CCS pathway are polytopic membrane proteins proposed to operate in the delivery of heme from the stroma to the lumen, and also in the catalysis of the heme ligation reaction. CCDA, CCS4, and CCS5 are components of trans-thylakoid pathways that deliver reducing equivalents in order to maintain the heme-binding cysteines in a reduced form prior to thioether bond formation. While only four CCS components are needed in bacteria, at least eight components are required for plastid cytochrome c assembly, suggesting the biochemistry of thioether formation is more nuanced in the plastid system.
Cytochromes of the c-type, often generically referred to as cytochromes c, are membrane-bound or soluble metalloproteins occurring in energy-transducing membranes in archaea, bacteria, mitochondria, and plastids, where they function as electron carriers in respiration and photosynthesis (Thony-Meyer, 1997; Bonnard et al., 2010; Kletzin et al., 2015). Cytochromes c, on the positive side (or p-side)1 of energy-transducing membranes, carry one or several hemes (ferroprotoporphyrin IX) as a prosthetic group covalently attached via thioether bonds to a heme binding site in the apoprotein. The most common heme binding site consists of a CXXCH motif where the first and second cysteines are, respectively, linked to the vinyl-2 and vinyl-4 groups of heme and the intervening residue X can be any amino-acid except cysteine in naturally occurring cytochromes c (Allen et al., 2004; Bowman and Bren, 2008). The histidine residue serves as the proximal axial ligand of the iron atom. A distant histidine, methionine, or, less commonly, other residues in the apocytochrome provide distal axial ligation of the heme group (Bowman and Bren, 2008).
Variations of the heme binding site are rare and one example is the A/FXXCH motif of mitochondrial cytochromes c in Euglenozoa that bind the vinyl-4 group of heme via a single thioether bond (Priest and Hajduk, 1992; Fülöp et al., 2009). Other non-canonical heme binding sites occur in bacterial cytochromes c and contain three, four, or fifteen intervening residues between the cysteines instead of two (Herbaud et al., 2000; Aragão et al., 2003; Hartshorne et al., 2007) or a lysine instead of a histidine as the proximal heme ligand (Einsle et al., 1999). Another atypical cytochrome c is also the only known example of a cytochrome c on the negative side (n-side)2 of the membrane. This is cytochrome b6 of the b6f complex in plastids and cyanobacteria and cytochrome b of the bc complex in firmicutes, which contain a heme covalently attached via a single thioether bond (de Vitry, 2011). The heme binding cysteine faces the n-side of the membrane and is not found in a motif, unlike other p-side localized cytochromes c. Moreover, there are no amino-acid residues serving as proximal and distal ligands of heme, differentiating this cytochrome c from all other c-type cytochromes occurring on the p-side (de Vitry, 2011).
All p-side localized holocytochromes c are assembled on the p-side of the membrane. This requires the apoform and the heme moiety, both of which are transported independently across at least one biological membrane. Conversion of apocytochrome to its holoform requires free sulfhydryls at the CXXCH motif, provision of heme under the reduced form (ferroheme), and stereospecific attachment of the prosthetic group via catalysis of the thioether bond linkage (Mavridou et al., 2013; Travaglini-Allocatelli, 2013). Extensive genetic and biochemical analyses in bacteria, plants, and fungi revealed that the operation of three distinct assembly pathways called Systems I, II, and III is required for cytochrome c maturation, depending on the location (Kranz et al., 2009; Mavridou et al., 2013; Travaglini-Allocatelli, 2013; Verissimo and Daldal, 2014; Babbitt et al., 2015). The diversity of maturation systems is surprising, considering the biochemical requirements for heme attachment to apocytochrome c are believed to be universal and thioether bond formation appears, a priori, a simple chemical reaction (Bowman and Bren, 2008). Each System can be recognized by prototypical assembly factors but the number of such assembly factors and their features differ considerably among the different Systems (Table 1). An additional layer of complexity is the apparent “mosaic” distribution of Systems I, II, and III among organisms and the different energy transducing membranes (Mavridou et al., 2013; Travaglini-Allocatelli, 2013). Several evolutionary scenarios accounting for the origin and distribution of the different maturation systems have been proposed but the complexity of cytochrome c maturation as a biochemical process still remains mysterious (Bertini et al., 2007; Allen et al., 2008; Giegé et al., 2008; Kranz et al., 2009; Allen, 2011).
Three c-type cytochromes, have been identified within the thylakoid lumen of various plastids: the membrane-bound cytochrome f and the soluble cytochromes c6 and c6A. While cytochrome f and c6 are known to function as electron carriers in photosynthesis, cytochrome c6A function remains enigmatic despite having been discovered 15 years ago (Howe et al., 2006). All plastid cytochromes c contain a single heme attached to a CXXCH motif on the apoprotein. Cytochrome f, a catalytic subunit of the cytochrome b6f complex, is universal in all photosynthetic eukaryotes (and cyanobacteria) and is essential for photosynthesis (Martinez et al., 1994). Cytochrome c6 is found in cyanobacteria and the plastid of eukaryotic algae, where it is widely distributed among green, red and brown algal lineages (Sandmann et al., 1983; Kerfeld and Krogmann, 1998). Cytochrome c6 is involved in the transfer of electrons from cytochrome f of the cytochrome b6f complex to Photosystem I (Merchant and Dreyfuss, 1998). In green algae and cyanobacteria, cytochrome c6 acts as a substitute for plastocyanin in Cu-deficient conditions (Merchant and Bogorad, 1987a,b). Cytochrome c6A occurs in the thylakoid lumen of land plants and green algae but appears absent in red algae and diatoms (Wastl et al., 2004).
Cytochrome c6A was discovered in Arabidopsis as a protein interacting with the lumen-localized immunophilin FKBP13 in a yeast two-hybrid screen (Gupta et al., 2002a,b; Buchanan and Luan, 2005). It was initially postulated that cytochrome c6A acts as a substitute for plastocyanin (Gupta et al., 2002a), as in green algae and cyanobacteria where cytochrome c6 can replace plastocyanin (Merchant and Bogorad, 1987a,b). However, loss of cytochrome c6A in Arabidopsis has no visible phenotype even under Cu deficient conditions (Gupta et al., 2002a). Moreover, an Arabidopsis plastocyanin-deficient mutant is unable to grow photoautotrophically even when cytochrome c6A is overexpressed (Weigel et al., 2003). In vitro, cytochrome c6A is unable to provide electrons to Photosystem I (Molina-Heredia et al., 2003). This observation accounts for the fact that cytochrome c6A cannot act as a functional substitute for plastocyanin in vivo. Hence, cytochrome c6A does not appear to function in the known electron transfer reactions of photosynthesis, which is consistent with its extremely low abundance in the thylakoid. The presence of a disulfide bond in holocytochrome c6A led to the proposal that the molecule acts as an oxidant of luminal proteins dithiols with heme providing electrons for re-oxidation of the cysteine pair (Marcaida et al., 2006). Additional experimental exploration is required to test this hypothesis.
Plastid cytochromes c are matured via System II, also referred to as the CCS pathway, a multicomponent assembly machinery (Hamel et al., 2009; Bonnard et al., 2010; Simon and Hederstedt, 2011). System II first emerged through genetic screens for photosynthesis-impaired ccs mutants (ccs for cytochrome c synthesis) in the green alga Chlamydomonas reinhardtii (Hamel et al., 2009; Simon and Hederstedt, 2011). The Chlamydomonas ccs mutants were isolated on the basis of a dual deficiency in the holoforms of both cytochrome f and cytochrome c6. All ccs mutants are photosynthetic deficient because loss of cytochrome f assembly results in a b6f-minus phenotype (Howe and Merchant, 1992; Inoue et al., 1997; Xie et al., 1998; Dreyfuss and Merchant, 1999; Page et al., 2004). Pulse-chase experiments revealed that both plastid apocytochromes c are synthesized, imported in the thylakoid lumen, and processed by lumen-resident signal peptidase, but they fail to be converted to their respective holoforms (Howe and Merchant, 1993, 1994; Xie et al., 1998). Based on these experiments, it was concluded the ccs mutants exhibit a defect in the heme attachment to apoforms of cytochrome c in the thylakoid lumen (Howe and Merchant, 1993, 1994; Xie et al., 1998). The ccs mutants are also expected to display a defect in cytochrome c6A. However, this could not be tested because holocytochrome c6A could not be detected in a wild-type strain. The defect in the ccs mutants is specific to plastid c-type cytochromes since plastocyanin, another lumen-resident metalloprotein, is normally assembled (Howe and Merchant, 1993, 1994; Xie et al., 1998). The ccs mutants are not affected for the covalent attachment of heme to the single n-side facing cysteine in cytochrome b6, a structural subunit of the b6f complex (Kuras et al., 2007). Catalysis of this thioether bond in cytochrome b6, occurs on the stromal side of the thylakoid membrane and is dependent upon the CCB gene products (de Vitry, 2011).
The first CCS component to be identified is plastid-encoded CcsA (Xie and Merchant, 1996; Hamel et al., 2003), a thylakoid membrane protein belonging to the HHP (Heme Handling Protein) superfamily (Lee et al., 2007), which is defined by the highly conserved tryptophan-rich WWD motif and conserved histidine residues (Figure 1). This feature is also shared by CcmC and CcmF, two HHPs in System I shown to relay heme on the bacterial periplasmic space (Richard-Fogal et al., 2009; Richard-Fogal and Kranz, 2010) (Table 1). The other prototypical component is CCS1, a thylakoid membrane protein with little sequence conservation and lacking domains or structural features speaking to a specific chemical function, with the exception of an invariant histidine (Inoue et al., 1997). Because all photosynthetic plastid genomes (with a few exceptions) encode a CcsA-like protein, the CCS pathway is believed to operate in the plastids of all photosynthetic eukaryotes. System II also occurs in cyanobacteria, a majority of the Gram-positive bacteria, proteobacteria of the β-, δ-, and ε groups, and aquificales (Hamel et al., 2009; Bonnard et al., 2010; Simon and Hederstedt, 2011).
FIGURE 1. A Model for plastid cytochrome c maturation. Plastid cytochrome c maturation involves two different pathways: (1) a trans-thylakoid disulfide reducing route with CCDA (red), CCS5/HCF164 (light orange), and CCS4/HCF153 (orange). The CCDA topology was deduced using PhoA/LacZ topological reporters (Page et al., 2004). CCS5/HCF164 is a membrane anchored, lumen-facing, thioredoxin-like protein (Lennartz et al., 2001). Cysteines (C) are circled and highlighted in yellow. Electron (e-) transfer is indicated by black arrows. Stromal thioredoxin-m is the possible reductant for the CCDA-CCS5/HCF164 pathway, which conveys electrons to reduce disulfide-bonded CXXCH motif in apocytochrome c. CCS4/HCF153 is bound to the thylakoid membrane (Lennartz et al., 2006) and the soluble part of the protein is predicted to be facing the stroma based on the positive-inside rule (Gabilly et al., 2011). The role of CCS4/HCF153 in cytochrome c biogenesis is unclear, but it may be involved in the transport of reductant across the membrane. (2) A heme delivery/cytochrome c synthase pathway composed of CCS1 (dark blue) and CcsA (light blue). CcsA and CCS1 topologies were deduced using PhoA/LacZ topological reporters (Hamel et al., 2003; Dreyfuss et al., 2003). The CCS1/CcsA complex binds and channels the reduced heme from the stroma to the thylakoid lumen and catalyzes thioether bond formation. Two histidines, one from CCS1 and one from CcsA, on the stromal side of the thylakoid membrane could function in relaying the heme across the membrane. Two histidines in CcsA on the luminal side could act by coordinating heme that interacts with the WWD domain. Strictly conserved histidines (H), tryptophans (W), and asparatic acids (D) are circled and highlighted in yellow. The thylakoid membrane is drawn in gray.
Detailed studies, including topological studies of algal CcsA and cyanobacterial Ccs1, site-directed mutagenesis of conserved residues, and molecular analysis of existing ccs1 alleles, established that CcsA and CCS1 are polytopic membrane proteins with functional domains exposed to the lumen and four strictly conserved essential histidine residues on both the lumen and stromal sides (Dreyfuss et al., 2003; Hamel et al., 2003) (Figure 1). Functional domains include the WWD signature motif for CcsA and a large hydrophilic C-terminal extension for CCS1. The C-terminal domain was postulated to chaperone apocytochrome c from studies of cyanobacterial Ccs1 (Tichy and Vermaas, 1999). In Chlamydomonas, a 200 kDa CCS1-containing complex in wild-type no longer accumulated in a ccsA mutant, suggesting that CcsA and CCS1 occur in a complex. The reduced abundance of CCS1 in some ccs mutants suggests the 200 kDa complex may contain other CCS components besides CcsA and CCS1 (Hamel et al., 2003). This led to the proposal that these two proteins act together to relay heme via histidinyl coordination from its site of synthesis, the stroma, to the lumen. In the lumen, heme is relayed to the WWD domain and coordinated by two histidine residues in CcsA (Hamel et al., 2003; Figure 1).
Experimental proof that CcsA and Ccs1 catalyze the heme attachment reaction was provided with the finding that Ccs1-CcsA fusion proteins, naturally occurring in several ε-proteobacteria, could assemble reporter cytochrome(s) c in an Escherichia coli strain lacking its endogenous cytochrome c assembly machinery (Feissner et al., 2006; Frawley and Kranz, 2009; Goddard et al., 2010; Kern et al., 2010; Richard-Fogal et al., 2012). Biochemical evidence supporting a possible role of CcsA and Ccs1 in heme transport from the cytoplasm to the periplasm came from studies of the Ccs1-CcsA fusion from Helicobacter hepaticus. Spectroscopic analysis of the recombinant fusion protein identified the presence of heme. Mutagenesis of the two periplasm-facing histidines highlighted the importance of these residues for the binding of heme and its maintenance in a reduced state (Frawley and Kranz, 2009). This led to the hypothesis that CcsA carries a heme binding site on the periplasmic space, presumably required for the cytochrome c synthase activity. To test the function of the two transmembrane cytoplasm-facing histidines in Ccs1 and CcsA, these residues were mutated in the recombinant protein. Because heme is synthesized in the cytoplasm and was no longer detected in the mutated form of the protein, it was concluded that these histidines provide an entry site for heme through the lipid bilayer on the cytoplasmic side of the membrane.
This implied that Ccs1-CcsA functions in channeling heme from the cytoplasm to the periplasm, but a direct heme transport activity remains to be demonstrated. By analogy, it is plausible that plastid CcsA and CCS1 also function in a heme relay pathway from stroma to lumen and carry the cytochrome c synthase activity but this has not been tested. While candidate components for the chemical reduction of heme were identified in System I, it is unknown how this process is achieved in System II (Table 1).
The operation of a thylakoid transmembrane thiol-disulfide relay in plastid cytochrome c maturation emerged with the description of two thiol-disulfide oxidoreductases at the thylakoid membrane, namely CCDA, a member of the DsbD family, and HCF164, a membrane-anchored, lumen-facing protein that displays similarity to thioredoxin-like CcmG and CcsX (Table 1) (Lennartz et al., 2001; Page et al., 2004; Motohashi and Hisabori, 2006; Motohashi and Hisabori, 2010). In bacteria using Systems I and II, cytochrome c maturation requires the provision of reductants via sequential thiol-disulfide exchanges involving a cytoplasmic thioredoxin, a thiol-disulfide reductase of the DsbD family, and a periplasmic thioredoxin-like protein (CcmG in System I or CcsX in System II) (Table 1) (Mavridou et al., 2013; Travaglini-Allocatelli, 2013). The working model is that the apocytochrome c CXXCH motif is first disulfide bonded by the disulfide bond forming enzymes residing in the periplasm and subsequently reduced by a thioredoxin-like protein (CcmG or CcsX) dedicated to the heme attachment reaction (Mavridou et al., 2013; Travaglini-Allocatelli, 2013). Reverse-genetic analysis in Arabidopsis indicates a function for CCDA and HCF164 in holocytochrome f accumulation, but a possible defect in the heme attachment reaction was not investigated (Lennartz et al., 2001; Page et al., 2004).
The biochemical requirement for thiol-disulfide chemistry in plastid cytochrome c biogenesis was demonstrated with the identification of CCS5, the Chlamydomonas ortholog of thioredoxin-like HCF164 (Gabilly et al., 2010). CCS5 physically interacts with plastid apocytochromes c and a recombinant form of the CCS5 molecule is active as a reductase when apocytochrome c with a disulfide-bonded CXXCH motif is provided as a substrate in an in vitro reaction (Gabilly et al., 2010). Application of exogenous thiols to the ccs5-null mutant rescues the photosynthetic deficiency and holocytochrome f assembly, an indication that CCS5 acts as a disulfide reductase in vivo (Gabilly et al., 2010). By analogy to the bacterial pathway, CCS5/HCF164 is likely to be maintained reduced by the activity of CCDA but this remains to be experimentally tested (Figure 1). The source of reducing equivalents on the stromal side was attributed to thioredoxin-m (Trx-m) (Figure 1) based on the observation that the redox active cysteines in CCDA and HCF164 undergo reduction in isolated thylakoid membranes when Trx-m is added exogenously (Motohashi and Hisabori, 2010). Complete loss of function of CCDA or HCF164 in Arabidopsis and CCS5 in Chlamydomonas does not abolish plastid cytochrome c maturation, an indication that another mechanism for delivery of reductant must exist (Lennartz et al., 2001; Page et al., 2004; Gabilly et al., 2010).
Evidence of an additional pathway for the supply of reducing power was provided with the finding that the ccs4 mutant is restored for cytochrome c assembly by application of exogenous thiols (Gabilly et al., 2011). CCS4 is a small protein with an N-terminal membrane anchor and a C-terminal domain predicted to be exposed to the stromal side of the thylakoid membrane but does not display any motif or residue (such as cysteines) suggesting a role in thiol-based redox chemistry (Gabilly et al., 2011). CCS4 exhibits similarity to Arabidopsis HCF153, a thylakoid membrane anchored protein with a stromal facing C-terminal domain required for cytochrome b6f accumulation (Lennartz et al., 2006). In addition to the thiol-dependent photosynthetic rescue of the ccs4 mutant, the placement of CCS4 in a disulfide-reducing pathway for cytochrome c assembly is further substantiated by the fact that ectopic expression of CCDA, a thiol/disulfide oxidoreductase of the DsbD family, at the thylakoid membrane suppresses the ccs4 mutant (Gabilly et al., 2011). As none of the CCS loci correspond to CCDA (Page et al., 2004), the CCDA-dependent suppression of the ccs4 mutant provides indirect evidence for the function of CCDA in plastid cytochrome c maturation. The suppression can be explained by a compensatory effect due to enhanced expression of the thiol-disulfide oxidoreductase CCDA. The activity of CCS4 in the heme attachment reaction so far remains unclear but one attractive scenario is that it controls the delivery of reducing power through the membrane via transport of a reductant. There is precedence for this in bacterial periplasm where reducing power, in the form of cysteine or glutathione, is transferred from the cytoplasm to the periplasm via specific transporters (Pittman et al., 2005; Ohtsu et al., 2010).
In bacteria using the CCS pathway, CcsA, Ccs1, a thiol-disulfide reductase of the DsbD family, and a thioredoxin-like protein are the only components required to complete holocytochrome c assembly (Beckett et al., 2000; Le Brun et al., 2000). From the genetic analysis of the Chlamydomonas ccs mutants, it appears that cytochrome c maturation in plastids is a more complicated process than in bacteria. This seems counter-intuitive considering that bacteria can assemble numerous mono and multiheme cytochromes c via the CCS pathway, while plastids only need to mature up to three monoheme cytochromes. In addition to CcsA, CCS1, CCDA, and HCF164/CCS5, plastid cytochrome c maturation also requires CCS4 and the products of the CCS2, CCS3, and CCS6 genes (Xie et al., 1998; Page et al., 2004), which remain uncharacterized. The fact that single alleles map to the Chlamydomonas CCS3, CCS4, CCS5, and CCS6 loci suggests that mutant screens for plastid cytochrome c deficient mutants are not saturated and additional CCS loci could still be uncovered (Howe and Merchant, 1992; Xie et al., 1998; Dreyfuss and Merchant, 1999; Page et al., 2004).
SG and PH wrote the manuscript jointly. SG designed the figure.
The authors declare that the research was conducted in the absence of any commercial or financial relationships that could be construed as a potential conflict of interest.
This work was primarily supported by a National Science Foundation grant (MCB-0920062) and a United States Department of Energy (DOE), Office of Science, Basic Energy Sciences (BES) grant (DE-SC0014562) to PH. We thank Dr. B. Alber, Dr. A. Simcox, Dr. S. Cline, M. Webber, and A. Das for critical reading of the manuscript.
Allen, J. W., Ginger, M. L., and Ferguson, S. J. (2004). Maturation of the unusual single-cysteine (XXXCH) mitochondrial c-type cytochromes found in trypanosomatids must occur through a novel biogenesis pathway. Biochem. J. 383(Pt 3), 537–542.
Allen, J. W., Jackson, A. P., Rigden, D. J., Willis, A. C., Ferguson, S. J., and Ginger, M. L. (2008). Order within a mosaic distribution of mitochondrial c-type cytochrome biogenesis systems? FEBS J. 275, 2385–2402. doi: 10.1111/j.1742-4658.2008.06380.x
Allen, J. W. A. (2011). Cytochrome c biogenesis in mitochondria – Systems III and V. FEBS J. 278, 4198–4216. doi: 10.1111/j.1742-4658.2011.08231.x
Aragão, D., Frazão, C., Sieker, L., Sheldrick, G. M., LeGall, J., and Carrondo, M. A. (2003). Structure of dimeric cytochrome c3 from Desulfovibrio gigas at 1.2 A resolution. Acta Crystallogr. D Biol. Crystallogr. 59(Pt 4), 644–653. doi: 10.1107/S090744490300194X
Babbitt, S. E., Sutherland, M. C., Francisco, B. S., Mendez, D. L., and Kranz, R. G. (2015). Mitochondrial cytochrome c biogenesis: no longer an enigma. Trends Biochem. Sci. 40, 446–455. doi: 10.1016/j.tibs.2015.05.006
Beckett, C. S., Loughman, J. A., Karberg, K. A., Donato, G. M., Goldman, W. E., and Kranz, R. G. (2000). Four genes are required for the system II cytochrome c biogenesis pathway in Bordetella pertussis, a unique bacterial model. Mol. Microbiol. 38, 465–481. doi: 10.1046/j.1365-2958.2000.02174.x
Bertini, I., Cavallaro, G., and Rosato, A. (2007). Evolution of mitochondrial-type cytochrome c domains and of the protein machinery for their assembly. J. Inorg. Biochem. 101, 1798–1811. doi: 10.1016/j.jinorgbio.2007.02.001
Bonnard, G., Corvest, V., Meyer, E. H., and Hamel, P. P. (2010). Redox processes controlling the biogenesis of c-type cytochromes. Antioxid. Redox Signal. 13, 1385–1401. doi: 10.1089/ars.2010.3161
Bowman, S. E. J., and Bren, K. L. (2008). The chemistry and biochemistry of heme c: functional bases for covalent attachment. Nat. Prod. Rep. 25, 1118–1130. doi: 10.1039/b717196j
Buchanan, B. B., and Luan, S. (2005). Redox regulation in the chloroplast thylakoid lumen: a new frontier in photosynthesis research. J. Exp. Bot. 56, 1439–1447. doi: 10.1093/jxb/eri158
de Vitry, C. (2011). Cytochrome c maturation system on the negative side of bioenergetic membranes: CCB or System IV. FEBS J. 278, 4189–4197. doi: 10.1111/j.1742-4658.2011.08373.x
Dreyfuss, B. W., Hamel, P. P., Nakamoto, S. S., and Merchant, S. (2003). Functional analysis of a divergent system II protein, Ccs1, involved in c-type cytochrome biogenesis. J. Biol. Chem. 278, 2604–2613. doi: 10.1074/jbc.M208652200
Dreyfuss, B. W., and Merchant, S. (1999). “CCS5, a new locus required for chloroplast c-type synthesis,” in Proceedings of the XIth International Congress on Photosynthesis, eds J. Pusztai and G. Garab (Dordrecht: Kluwer Academic Publishers), 3139–3142.
Einsle, O., Messerschmidt, A., Stach, P., Bourenkov, G. P., Bartunik, H. D., Huber, R., et al. (1999). Structure of cytochrome c nitrite reductase. Nature 400, 476–480. doi: 10.1038/22802
Feissner, R. E., Richard-Fogal, C. L., Frawley, E. R., Loughman, J. A., Earley, K. W., and Kranz, R. G. (2006). Recombinant cytochromes c biogenesis systems I and II and analysis of haem delivery pathways in Escherichia coli. Mol. Microbiol. 60, 563–577. doi: 10.1111/j.1365-2958.2006.05132.x
Frawley, E. R., and Kranz, R. G. (2009). CcsBA is a cytochrome c synthetase that also functions in heme transport. Proc. Natl. Acad. Sci. U.S.A. 106, 10201–10206. doi: 10.1073/pnas.0903132106
Fülöp, V., Sam, K. A., Ferguson, S. J., Ginger, M. L., and Allen, J. W. A. (2009). Structure of a trypanosomatid mitochondrial cytochrome c with heme attached via only one thioether bond and implications for the substrate recognition requirements of heme lyase. FEBS J. 276, 2822–2832. doi: 10.1111/j.1742-4658.2009.07005.x
Gabilly, S. T., Dreyfuss, B. W., Karamoko, M., Corvest, V., Kropat, J., Page, M. D., et al. (2010). CCS5, a thioredoxin-like protein involved in the assembly of plastid c-type cytochromes. J. Biol. Chem. 285, 29738–29749. doi: 10.1074/jbc.M109.099069
Gabilly, S. T., Kropat, J., Karamoko, M., Page, M. D., Nakamoto, S. S., Merchant, S. S., et al. (2011). A novel component of the disulfide-reducing pathway required for cytochrome c assembly in plastids. Genetics 187, 793–802. doi: 10.1534/genetics.110.125369
Giegé, P., Grienenberger, J. M., and Bonnard, G. (2008). Cytochrome c biogenesis in mitochondria. Mitochondrion 8, 61–73. doi: 10.1016/j.mito.2007.10.001
Goddard, A. D., Stevens, J. M., Rondelet, A., Nomerotskaia, E., Allen, J. W., and Ferguson, S. J. (2010). Comparing the substrate specificities of cytochrome c biogenesis Systems I and II: bioenergetics. FEBS J. 277, 726–737. doi: 10.1111/j.1742-4658.2009.07517.x
Gupta, R., He, Z., and Luan, S. (2002a). Functional relationship of cytochrome c6 and plastocyanin in Arabidopsis. Nature 417, 567–571.
Gupta, R., Mould, R. M., He, Z., and Luan, S. (2002b). A chloroplast FKBP interacts with and affects the accumulation of Rieske subunit of cytochrome bf complex. Proc. Natl. Acad. Sci. U.S.A. 99, 15806–15811. doi: 10.1073/pnas.222550399
Hamel, P., Corvest, V., Giege, P., and Bonnard, G. (2009). Biochemical requirements for the maturation of mitochondrial c-type cytochromes. Biochim. Biophys. Acta 1793, 125–138. doi: 10.1016/j.bbamcr.2008.06.017
Hamel, P. P., Dreyfuss, B. W., Xie, Z., Gabilly, S. T., and Merchant, S. (2003). Essential histidine and tryptophan residues in CcsA, a system II polytopic cytochrome c biogenesis protein. J. Biol. Chem. 278, 2593–2603. doi: 10.1074/jbc.M208651200
Hartshorne, R. S., Kern, M., Meyer, B., Clarke, T. A., Karas, M., Richardson, D. J., et al. (2007). A dedicated haem lyase is required for the maturation of a novel bacterial cytochrome c with unconventional covalent haem binding. Mol. Microbiol. 64, 1049–1060. doi: 10.1111/j.1365-2958.2007.05712.x
Herbaud, M. L., Aubert, C., Durand, M. C., Guerlesquin, F., Thöny-Meyer, L., and Dolla, A. (2000). Escherichia coli is able to produce heterologous tetraheme cytochrome c3 when the ccm genes are co-expressed. Biochim. Biophys. Acta 1481, 18–24. doi: 10.1016/S0167-4838(00)00117-5
Howe, C. J., Schlarb-Ridley, B. G., Wastl, J., Purton, S., and Bendall, D. S. (2006). The novel cytochrome c6 of chloroplasts: a case of evolutionary bricolage? J. Exp. Bot. 57, 13–22. doi: 10.1093/jxb/erj023
Howe, G., and Merchant, S. (1992). The biosynthesis of membrane and soluble plastidic c-type cytochromes of Chlamydomonas reinhardtii is dependent on multiple common gene products. EMBO J. 11, 2789–2801.
Howe, G., and Merchant, S. (1993). Maturation of thylakoid lumen proteins proceeds post-translationally through an intermediate in vivo. Proc. Natl. Acad. Sci. U.S.A. 90, 1862–1866. doi: 10.1073/pnas.90.5.1862
Howe, G., and Merchant, S. (1994). Role of heme in the biosynthesis of cytochrome c6. J. Biol. Chem. 269, 5824–5832.
Inoue, K., Dreyfuss, B. W., Kindle, K. L., Stern, D. B., Merchant, S., and Sodeinde, O. A. (1997). CCS1, a nuclear gene required for the post-translational assembly of chloroplast c-type cytochromes. J. Biol. Chem. 272, 31747–31754. doi: 10.1074/jbc.272.50.31747
Kerfeld, C. A., and Krogmann, D. W. (1998). PHOTOSYNTHETIC CYTOCHROMES c IN CYANOBACTERIA, ALGAE, AND PLANTS. Annu. Rev. Plant Physiol. Plant Mol. Biol. 49, 397–425. doi: 10.1146/annurev.arplant.49.1.397
Kern, M., Eisel, F., Scheithauer, J., Kranz, R. G., and Simon, J. (2010). Substrate specificity of three cytochrome c haem lyase isoenzymes from Wolinella succinogenes: unconventional haem c binding motifs are not sufficient for haem c attachment by NrfI and CcsA1. Mol. Microbiol. 75, 122–137. doi: 10.1111/j.1365-2958.2009.06965.x
Kletzin, A., Heimerl, T., Flechsler, J., van Niftrik, L., Rachel, R., and Klingl, A. (2015). Cytochromes c in Archaea: distribution, maturation, cell architecture, and the special case of Ignicoccus hospitalis. Front. Microbiol. 6:439. doi: 10.3389/fmicb.2015.00439
Kranz, R. G., Richard-Fogal, C., Taylor, J.-S., and Frawley, E. R. (2009). Cytochrome c biogenesis: mechanisms for covalent modifications and trafficking of heme and for heme-iron redox control. Microbiol. Mol. Biol. Rev. 73, 510–528. doi: 10.1128/mmbr.00001-09
Kuras, R., Saint-Marcoux, D., Wollman, F. A., and de Vitry, C. (2007). A specific c-type cytochrome maturation system is required for oxygenic photosynthesis. Proc. Natl. Acad. Sci. U.S.A. 104, 9906–9910. doi: 10.1073/pnas.0702340104
Le Brun, N. E., Bengtsson, J., and Hederstedt, L. (2000). Genes required for cytochrome c synthesis in Bacillus subtilis. Mol. Microbiol. 36, 638–650. doi: 10.1046/j.1365-2958.2000.01883.x
Lee, J. H., Harvat, E. M., Stevens, J. M., Ferguson, S. J., and Saier, M. H. Jr. (2007). Evolutionary origins of members of a superfamily of integral membrane cytochrome c biogenesis proteins. Biochim. Biophys. Acta 1768, 2164–2181. doi: 10.1016/j.bbamem.2007.04.022
Lennartz, K., Bossmann, S., Westhoff, P., Bechtold, N., and Meierhoff, K. (2006). HCF153, a novel nuclear-encoded factor necessary during a post-translational step in biogenesis of the cytochrome bf complex. Plant J. 45, 101–112. doi: 10.1111/j.1365-313X.2005.02605.x
Lennartz, K., Plücken, H., Seidler, A., Westhoff, P., Bechtold, N., and Meierhoff, K. (2001). HCF164 encodes a thioredoxin-like protein involved in the biogenesis of the cytochrome b6f complex in Arabidopsis. Plant Cell 13, 2539–2552. doi: 10.1105/tpc.13.11.2539
Marcaida, M. J., Schlarb-Ridley, B. G., Worrall, J. A., Wastl, J., Evans, T. J., Bendall, D. S., et al. (2006). Structure of cytochrome c6A, a novel dithio-cytochrome of Arabidopsis thaliana, and its reactivity with plastocyanin: implications for function. J. Mol. Biol. 360, 968–977. doi: 10.1016/j.jmb.2006.05.065
Martinez, S. E., Huang, D., Szczepaniak, A., Cramer, W. A., and Smith, J. L. (1994). Crystal structure of chloroplast cytochrome f reveals a novel cytochrome fold and unexpected heme ligation. Structure 2, 95–105. doi: 10.1016/S0969-2126(00)00012-5
Mavridou, D. A. I., Ferguson, S. J., and Stevens, J. M. (2013). Cytochrome c assembly. IUBMB Life 65, 209–216. doi: 10.1002/iub.1123
Merchant, S., and Bogorad, L. (1987a). The Cu(II)-repressible plastidic cytochrome c. Cloning and sequence of a complementary DNA for the pre-apoprotein. J. Biol. Chem. 262, 9062–9067.
Merchant, S., and Bogorad, L. (1987b). Metal ion regulated gene expression: use of a plastocyanin-less mutant of Chlamydomonas reinhardtii to study the Cu(II)-dependent expression of cytochrome c-552. EMBO J. 6, 2531–2535.
Merchant, S., and Dreyfuss, B. W. (1998). Posttranslational assembly of photosynthetic metalloproteins. Annu. Rev. Plant Physiol. Plant Mol. Biol. 49, 25–51. doi: 10.1146/annurev.arplant.49.1.25
Molina-Heredia, F. P., Wastl, J., Navarro, J. A., Bendall, D. S., Hervas, M., Howe, C. J., et al. (2003). Photosynthesis: a new function for an old cytochrome? Nature 424, 33–34. doi: 10.1038/424033b
Motohashi, K., and Hisabori, T. (2006). HCF164 receives reducing equivalents from stromal thioredoxin across the thylakoid membrane and mediates reduction of target proteins in the thylakoid lumen. J. Biol. Chem. 281, 35039–35047. doi: 10.1074/jbc.M605938200
Motohashi, K., and Hisabori, T. (2010). CcdA is a thylakoid membrane protein required for the transfer of reducing equivalents from stroma to thylakoid lumen in the higher plant chloroplast. Antioxid. Redox Signal. 13, 1169–1176. doi: 10.1089/ars.2010.3138
Ohtsu, I., Wiriyathanawudhiwong, N., Morigasaki, S., Nakatani, T., Kadokura, H., and Takagi, H. (2010). The L-cysteine/L-cystine shuttle system provides reducing equivalents to the periplasm in Escherichia coli. J. Biol. Chem. 285, 17479–17487. doi: 10.1074/jbc.M109.081356
Page, M. L. D., Hamel, P. P., Gabilly, S. T., Zegzouti, H., Perea, J. V., Alonso, J. M., et al. (2004). A homolog of prokaryotic thiol disulfide transporter CcdA is required for the assembly of the cytochrome b6f complex in Arabidopsis chloroplasts. J. Biol. Chem. 279, 32474–32482. doi: 10.1074/jbc.M404285200
Pittman, M. S., Robinson, H. C., and Poole, R. K. (2005). A bacterial glutathione transporter (Escherichia coli CydDC) exports reductant to the periplasm. J. Biol. Chem. 280, 32254–32261. doi: 10.1074/jbc.M503075200
Priest, J. W., and Hajduk, S. L. (1992). Cytochrome c reductase purified from Crithidia fasciculata contains an atypical cytochrome c1. J. Biol. Chem. 267, 20188–20195.
Richard-Fogal, C., and Kranz, R. G. (2010). The CcmC:heme:CcmE complex in heme trafficking and cytochrome c biosynthesis. J. Mol. Biol. 401, 350–362. doi: 10.1016/j.jmb.2010.06.041
Richard-Fogal, C. L., Frawley, E. R., Bonner, E. R., Zhu, H., San Francisco, B., and Kranz, R. G. (2009). A conserved haem redox and trafficking pathway for cofactor attachment. EMBO J. 28, 2349–2359. doi: 10.1038/emboj.2009.189
Richard-Fogal, C. L., San Francisco, B., Frawley, E. R., and Kranz, R. G. (2012). Thiol redox requirements and substrate specificities of recombinant cytochrome c assembly systems II and III. Biochim. Biophys. Acta 1817, 911–919. doi: 10.1016/j.bbabio.2011.09.008
Sandmann, G., Reck, H., Kessler, E., and Boger, P. (1983). Distribution of plastocyanin and soluble plastidic cytochrome c in various classes of algae. Arch. Microbiol. 134, 23–27. doi: 10.1007/BF00429401
Simon, J., and Hederstedt, L. (2011). Composition and function of cytochrome c biogenesis System II. FEBS J. 278, 4179–4188. doi: 10.1111/j.1742-4658.2011.08374.x
Thony-Meyer, L. (1997). Biogenesis of respiratory cytochromes in bacteria. Microbiol. Mol. Biol. Rev. 61, 337–376.
Tichy, M., and Vermaas, W. (1999). Accumulation of pre-apocytochrome f in a Synechocystis sp. PCC 6803 mutant impaired in cytochrome c maturation. J. Biol. Chem. 274, 32396–32401. doi: 10.1074/jbc.274.45.32396
Travaglini-Allocatelli, C. (2013). Protein machineries involved in the attachment of heme to cytochrome c: protein structures and molecular mechanisms. Scientifica 2013:505714. doi: 10.1155/2013/505714
Verissimo, A. F., and Daldal, F. (2014). Cytochrome c biogenesis System I: an intricate process catalyzed by a maturase supercomplex? Biochim. Biophys. Acta 1837, 989–998. doi: 10.1016/j.bbabio.2014.03.003
Wastl, J., Purton, S., Bendall, D. S., and Howe, C. J. (2004). Two forms of cytochrome c6 in a single eukaryote. Trends Plant Sci. 9, 474–476. doi: 10.1016/j.tplants.2004.08.005
Weigel, M., Varotto, C., Pesaresi, P., Finazzi, G., Rappaport, F., Salamini, F., et al. (2003). Plastocyanin is indispensable for photosynthetic electron flow in Arabidopsis thaliana. J. Biol. Chem. 278, 31286–31289. doi: 10.1074/jbc.M302876200
Xie, Z., Culler, D., Dreyfuss, B. W., Kuras, R., Wollman, F. A., Girard-Bascou, J., et al. (1998). Genetic analysis of chloroplast c-type cytochrome assembly in Chlamydomonas reinhardtii: one chloroplast locus and at least four nuclear loci are required for heme attachment. Genetics 148, 681–692.
Keywords: thylakoid lumen, photosynthesis, cytochrome c, heme, thioether, cytochrome b6f
Citation: Gabilly ST and Hamel PP (2017) Maturation of Plastid c-type Cytochromes. Front. Plant Sci. 8:1313. doi: 10.3389/fpls.2017.01313
Received: 16 April 2017; Accepted: 12 July 2017;
Published: 26 July 2017.
Edited by:
Dario Leister, Ludwig-Maximilians-Universität München, GermanyReviewed by:
Fevzi Daldal, University of Pennsylvania, United StatesCopyright © 2017 Gabilly and Hamel. This is an open-access article distributed under the terms of the Creative Commons Attribution License (CC BY). The use, distribution or reproduction in other forums is permitted, provided the original author(s) or licensor are credited and that the original publication in this journal is cited, in accordance with accepted academic practice. No use, distribution or reproduction is permitted which does not comply with these terms.
*Correspondence: Patrice P. Hamel, aGFtZWwuMTZAb3N1LmVkdQ==
†Present address: Stéphane T. Gabilly, Department of Plant and Microbial Biology, University of California, Berkeley, Berkeley, CA, United States
Disclaimer: All claims expressed in this article are solely those of the authors and do not necessarily represent those of their affiliated organizations, or those of the publisher, the editors and the reviewers. Any product that may be evaluated in this article or claim that may be made by its manufacturer is not guaranteed or endorsed by the publisher.
Research integrity at Frontiers
Learn more about the work of our research integrity team to safeguard the quality of each article we publish.