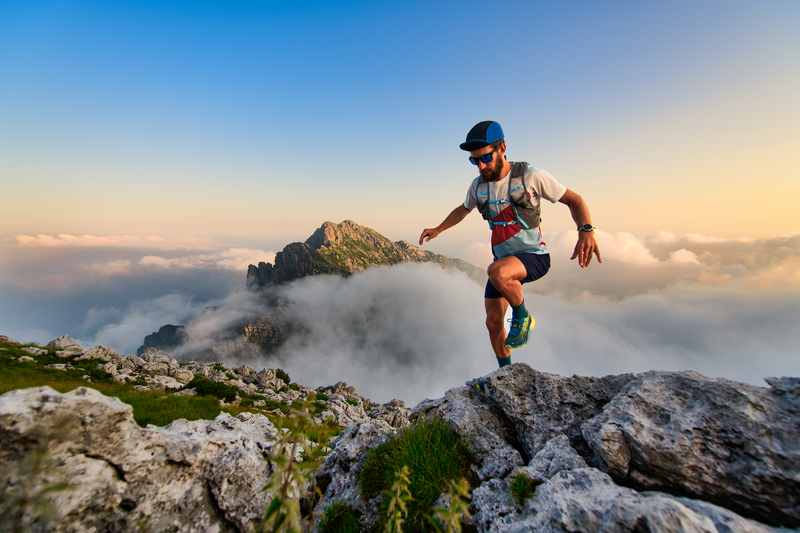
95% of researchers rate our articles as excellent or good
Learn more about the work of our research integrity team to safeguard the quality of each article we publish.
Find out more
ORIGINAL RESEARCH article
Front. Plant Sci. , 23 May 2017
Sec. Plant Development and EvoDevo
Volume 8 - 2017 | https://doi.org/10.3389/fpls.2017.00788
This article is part of the Research Topic Evolution of gene regulatory networks in plant development View all 18 articles
Plant shoot systems give rise to characteristic above-ground plant architectures. Shoots are formed from axillary meristems and buds, whose growth and development is modulated by systemic and local signals. These cues convey information about nutrient and water availability, light quality, sink/source organ activity and other variables that determine the timeliness and competence to maintain development of new shoots. This information is translated into a local response, in meristems and buds, of growth or quiescence. Although some key genes involved in the onset of bud latency have been identified, the gene regulatory networks (GRNs) controlled by these genes are not well defined. Moreover, it has not been determined whether bud dormancy induced by environmental cues, such as a low red-to-far-red light ratio, shares genetic mechanisms with bud latency induced by other causes, such as apical dominance or a short-day photoperiod. Furthermore, the evolution and conservation of these GRNs throughout angiosperms is not well established. We have reanalyzed public transcriptomic datasets that compare quiescent and active axillary buds of Arabidopsis, with datasets of axillary buds of the woody species Vitis vinifera (grapevine) and apical buds of Populus tremula x Populus alba (poplar) during the bud growth-to-dormancy transition. Our aim was to identify potentially common GRNs induced during the process that leads to bud para-, eco- and endodormancy. In Arabidopsis buds that are entering eco- or paradormancy, we have identified four induced interrelated GRNs that correspond to a carbon (C) starvation syndrome, typical of tissues undergoing low C supply. This response is also detectable in poplar and grapevine buds before and during the transition to dormancy. In all eukaryotes, C-limiting conditions are coupled to growth arrest and latency like that observed in dormant axillary buds. Bud dormancy might thus be partly a consequence of the underlying C starvation syndrome triggered by environmental and endogenous cues that anticipate or signal conditions unfavorable for sustained shoot growth.
Shoot branching patterns define overall above-ground plant architecture. In angiosperms, shoots are formed from axillary meristems initiated at the base of leaves. These meristems grow and develop into axillary buds that contain, preformed, most of the elements of adult branches (shoot meristems, leaf primordia, reproductive meristems). Axillary buds can enter a quiescent state, rather than growing out immediately to give a branch. In this latent or dormant state their metabolic activity and cell division are very limited (Shimizu and Mori, 1998; Ruttink et al., 2007). Bud dormancy and bud activation are influenced by environmental signals such as nutrient and water availability, light quality, day-length and temperature, and by endogenous signals such as sink/source organ activity and hormone signaling. Once dormant, buds require changes in specific developmental and/or environmental cues to resume growth and generate an elongated branch. These cues are monitored in different organs, and inform the plant as to when develop new shoots. This information is transduced to the bud and translated into a gene response that leads to quiescence or growth activation (Rameau et al., 2015).
Bud dormancy is therefore an adaptive trait that allows plants to endure adverse situations until conditions are favorable for development of new shoots. It has great impact on plant reproductive success and productivity, and on survival in temperate woody species. Evolution of this trait might have allowed plants to colonize habitats with fluctuating conditions not always suitable for sustained, uninterrupted growth. Depending on the type of stimulus that promotes growth arrest, Lang et al. (1985, 1987) distinguished three types of bud dormancy. When dormancy is induced by environmental factors, it is termed ecodormancy; when promoted by other plant organs it is paradormancy or correlative inhibition, and when it is maintained by signals internal to the bud and can only be reversed under certain conditions it is defined as endodormancy. In woody plants, axillary buds undergo transitions between different dormant states throughout the year. Paradormant buds enter endodormancy in response to changes in daylength and temperature. Chilling promotes transition from endo- to ecodormancy, after which the buds are susceptible to grow in response to mild temperatures (Rohde and Bhalerao, 2007).
Transcriptomic studies have been carried out in several herbaceous and woody species to define expression changes in buds during the transitions into and out of different types of dormancy, in response to changes in daylength, light quality, and apical dominance, and in mutant genotypes in which bud growth is affected (e.g., Tatematsu et al., 2005; Ruttink et al., 2007; González-Grandío et al., 2013; Reddy et al., 2013; Ueno et al., 2013; Porto et al., 2015). The GRNs that act inside the bud to control the stages leading to dormancy nonetheless remain little known. It is also largely unknown whether different types of dormancy share common underlying genetic mechanisms. Even less is known about the degree of conservation and evolution of the genetic control of this process in different plant species. Comparative analyses to identify common themes among different types of dormancy, or across species, are scarce (González-Grandío and Cubas, 2014; Fennell et al., 2015; Howe et al., 2015; Hao et al., 2017). Such comparisons could help us determine whether eco-, para- and endodormancy are variations of a single ancestral genetic program or whether each type is controlled by unrelated GRNs. It also will help elucidate whether GRNs that cause bud growth arrest are conserved in different herbaceous and woody plant species.
The master regulators that locally control the dormancy onset are also largely unknown. The best characterized are the genes that encode the TCP transcription factors (TF) teosinte branched1 (Tb1, Doebley et al., 1997), BRANCHED1 (BRC1, Aguilar-Martínez et al., 2007; Finlayson, 2007) and their orthologs in mono- and dicotyledonous species, respectively. These widely conserved factors play a very important role in the regulation of para- and ecodormancy in herbaceous plants. These genes are expressed in axillary buds and promote bud dormancy in response to fluctuating environmental cues such as light quality and quantity, and endogenous signals such as apical dominance, sugar availability and hormone signaling (reviewed in Nicolas and Cubas, 2015). In Arabidopsis thaliana, BRC1 controls transcription of several GRNs in buds; one, positively controlled by BRC1, leads to abscisic acid (ABA) accumulation and signaling, whereas another two that are downregulated by BRC1 are enriched in ribosomal protein genes in one case, and in cell division and DNA replication genes in the other (González-Grandío et al., 2013, 2017). Additional GRNs controlled by BRC1 remain to be characterized.
In this study our aim was to identify potentially common GRNs induced during the process that leads to bud para-, eco- and endodormancy. For that we compared publicly available transcriptomic data from active para- and ecodormant axillary buds of Arabidopsis, and found, induced in dormant buds, a shared transcriptomic response typical of tissue undergoing C starvation. We then detected this response also in Populus tremula × Populus alba (poplar) apical buds undergoing endodormancy and in Vitis vinifera (grapevine) axillary buds entering para-, endo- and ecodormancy. This C starvation transcriptional response, activated shortly after exposure to conditions leading to bud dormancy, anticipates and underlies the growth-to-dormancy transition in the three species. The C starvation syndrome entails a suite of interconnected transcriptional responses that include sugar signaling, sugar metabolism reprogramming, senescence, autophagy, catabolism, and ABA and ethylene signaling. It also involves downregulation of cytokinin (CK) signaling, inhibition of anabolism, and repression of protein/DNA synthesis and cell division, conditions typical of cells in dormant buds. This conserved starvation response, genetically connected to cell growth arrest, may be one of the underlying forces driving the growth-to-dormancy transition of axillary buds in response to suboptimal conditions in herbaceous and woody species.
Three independent transcriptomic analyses have compared active and dormant buds in Arabidopsis. One study compared the transcriptional profiling of (dormant) buds of intact plants and of (active) buds of decapitated plants at 24 h post-treatment (Tatematsu et al., 2005). Two additional experiments compared the transcripts of active vs. dormant buds of plants exposed to high red-to-far-red light ratio (R:FR, active buds) or low R:FR (dormant buds) (González-Grandío et al., 2013; Reddy et al., 2013). Here we define dormancy as a state in which bud growth is reversibly interrupted, regardless of the requirements to resume development. A search for genes upregulated in dormant buds relative to active buds in the three experiments identified 78 genes termed bud dormancy genes (Supplementary Figure S1 and Dataset S1; González-Grandío and Cubas, 2014). These genes correspond to the least common denominator of the three studies and were induced in para- and ecodormant buds by either correlative inhibition or low R:FR, respectively. They were also differentially expressed at 3 h (Reddy et al., 2013), 8 h (González-Grandío et al., 2013) and 24 h (Tatematsu et al., 2005) after treatment onset.
We evaluated the degree of coregulation of these genes using the most updated co-expression database of ATTED-II (15,275 microarray experiments; Obayashi et al., 2007). Hierarchical clustering analysis revealed four clusters of coregulated genes (14, 20, 13, and 31 genes; Figure 1A and Supplementary Dataset S1). We then searched for additional genes coregulated with each cluster using CoExSearch (ATTED-II, Obayashi et al., 2007) and obtained four lists of highly coregulated genes (Supplementary Dataset S1). Analysis of their fold change (FC) induction in the three active-vs.-dormant bud experiments confirmed that a significant proportion of the genes in each list were induced (FC ≥ 1.2) in dormant buds in at least one experiment (Figure 1B and Supplementary Figure S2). We termed the gene lists that comprised the bud dormancy genes of the original clusters plus their coregulated genes (induced in dormant buds in at least one experiment, red dots in Figure 1B) bud dormancy GRNI-IV, with 297, 283, 271, and 295 genes respectively (Supplementary Dataset S1).
FIGURE 1. Bud dormancy genes and GRN. (A) Hierarchical clustering representation of bud dormancy genes (González-Grandío and Cubas, 2014) based on their degree of coregulation in 15,275 microarray experiments (ATTED-II; Obayashi et al., 2007). The number of coregulated genes and GO terms enriched are indicated. (B) Volcano plots representing pval (–Log10 pval, vertical axis) and relative expression (Log2 fold change, horizontal axis) of all genes in each microarray. Normalized gene intensities in dormant buds vs. normalized gene intensities in active buds were compared in all experiments [3 h low R:FR (N-2 bud) vs. high R:FR (N-2 bud); 8 h low R:FR vs. High R:FR; intact plants vs. 24 h post-decapitation]. Bud dormancy genes and their coregulated genes are highlighted. In red and green, genes induced and repressed in dormant buds, respectively. Genes highlighted in red were attributed to Bud dormancy GRNI-IV (see Supplementary Dataset S1) and were used for subsequent analyses. (C) Venn diagram showing overlap between bud dormancy GRN. Number of common genes is indicated. (D) Model of the relationships between bud dormancy GRN. Line thickness indicates degree of overlap between GRN.
To elucidate the biological processes in which these GRNs were involved, we searched for enrichment in gene ontology (GO) terms using the Panther Classification System (Mi et al., 2017; Supplementary Dataset S2), complemented with a MapMan bin analysis (Thimm et al., 2004; Supplementary Dataset S1). GRNI was significantly enriched in terms related to ethylene, auxin and gibberellin signaling and response; GRNII in terms related to ABA, catabolism and response to abiotic stress; GRNIII in terms related to lipid and amino acid catabolism, senescence, response to starvation and biotic stress; and GRNIV in terms related to protein ubiquitination and response to sucrose starvation.
We evaluated the degree of overlap between these GRNs by seeking common genes. GRNIII and GRNIV shared one-third of their genes; GRNII and GRNIII shared 30%, and GRNI and GRNIV had 26% genes in common (Figure 1C, Supplementary Figure S3 and Dataset S3). This suggested that these GRNs are not strictly independent, but correspond to related aspects of the same syndrome, probably coordinated or maintained by ethylene, auxin and ABA signaling (Figure 1D).
We observed that three robust sugar starvation gene markers, GIBBERELLIN-STIMULATED ARABIDOPSIS 6 (GASA6), DORMANCY-ASSOCIATED PROTEIN-LIKE 1 (DRM1/DYL1) and DARK INDUCIBLE 6 (DIN6) (Contento et al., 2004; Price et al., 2004; Gonzali et al., 2006; Zhong et al., 2015) were members of one or several GRNs (GASA6, GRNI; DRM1, GRNI, III and IV; DIN6, GRNIII and IV; Supplementary Dataset S3). As sugar has a prominent role in the control of shoot outgrowth (Mason et al., 2014; Barbier F. et al., 2015; reviewed in Barbier F.F. et al., 2015) and GRNIV was significantly enriched in terms related to sucrose starvation, we studied this response further. The C starvation syndrome, triggered under C-limiting conditions (e.g., an extended night), helps to obtain an alternative energy source and C skeletons. In Arabidopsis, it comprises a suite of interconnected events that result in changes in C balance and growth. They include reprogramming of sugar sensing, transport, signaling and metabolism, increased protein ubiquitination and degradation, amino acid and lipid catabolism, induction of ABA and ethylene signaling, and recycling of cell components via autophagy and senescence. In addition, CK signaling, ribosomal gene expression, DNA synthesis and cell division are inhibited (Contento et al., 2004; Lin and Wu, 2004; Thimm et al., 2004; Gonzali et al., 2006; Rolland et al., 2006; Rose et al., 2006). Remarkably, many of the GO terms and/or MapMan bins enriched in the four GRNs matched categories induced by C-limiting conditions (Table 1 and Supplementary Datasets S1, S2).
TABLE 1. Bud dormancy genes from categories related to sugar sensing, transport, signaling and metabolism, protein ubiquitination and degradation, as well as amino acid and lipid catabolism, autophagy and senescence.
To test the possibility that these GRNs correspond to a C starvation response, we compared the GRN genes with four lists of genes induced in C-limiting conditions: (i) 26 genes of a robust core of C-signaling response shared by 21 Arabidopsis accessions (Supplementary Dataset S4; Sulpice et al., 2009), (ii) 57 sugar-responsive genes, proposed upstream components of the transcriptional response to sucrose (Supplementary Dataset S4; Osuna et al., 2007), (iii) 429 dark-induced, sugar-repressed genes (Supplementary Dataset S4; Gonzali et al., 2006) and (iv) 507 genes responsive to AKIN10, a catalytic subunit of the SUCROSE-NON-FERMENTING-1-RELATED PROTEIN KINASE (SnRK1), which integrates stress and C signals to coordinate energy balance, metabolism and growth (Supplementary Dataset S4; Baena-González et al., 2007).
Genes from these sets appeared in the GRNs at a much higher frequency than expected in a random list (pval 4.5E-11 to 7.5E-215; Table 2 and Supplementary Figure S4), indicating that the bud dormancy GRNs were very highly enriched in genes typical of a C starvation response.
We assessed this potential C starvation response by performing a Gene Set Enrichment Analysis (GSEA) using all transcribed genes from each experiment, rather than focusing on the bud dormancy GRNs. GSEA is a statistical approach that allows identification of overrepresented gene sets among differentially up- or downregulated genes of a transcriptomic experiment (Subramanian et al., 2005). For each “active-vs.-dormant bud” experiment, we generated a ranked gene list using relative gene expression levels and False Discovery Rate (FDR) values. We then tested whether gene sets related to a potential C starvation syndrome (sugar-, darkness- and AKIN10-responsive genes, ABA, ethylene and CK markers, ribosomal genes, cell cycle and cell division genes; Supplementary Dataset S4) were found toward the top (upregulated) or the bottom (downregulated) of the ranked gene lists. Analyses1 confirmed significant overrepresentation of C-signaling, sugar-repressed, AKIN10-induced and ABA and ethylene marker genes among those upregulated in the three experiments (Figures 2A, 3). In contrast, CK markers, ribosomal genes and S-phase genes were overrepresented among the downregulated genes (Figures 2B, 3). Other cell division markers such as M-phase genes, histones and kinesins were overrepresented only in the 8 and 24 h experiments, which suggests they are downregulated at later stages of the process (Figures 2B, 3).
FIGURE 2. Gene Set Enrichment Analysis (GSEA) analyses of dormant vs. active bud experiments. (A,B) Enrichment Scores (ES; green line) of selected gene sets that illustrate significant overrepresentation among up- (A) or down-regulated genes (B). Barcode-like vertical black lines represent logRatios of genes of each gene set in the ranked ordered data sets. Left (positive logRatios), genes induced in dormant buds; right (negative logRatios), genes repressed in dormant buds.
FIGURE 3. Summary of GSEA analyses of dormant vs. active buds in Arabidopsis, poplar and grapevine. Clustering of GSEA results for all transcriptomic samples and gene sets based on their normalized enrichment score (NES) for each sample. Complete results are in http://bioinfogp.cnb.csic.es/files/projects/tarancon_et_al_2017_supp/. Positive NES values (blue) are for gene sets overrepresented in the “dormant bud” condition. Negative NES values (yellow) are for gene sets overrepresented in the “active bud” condition. White circles indicate gene sets with a significant statistical overrepresentation (FDR < 0.05). Core C-signaling and Brassinolide (BL) markers in poplar, and ethylene and strigolactone (SL) markers in grapevine contained <10 genes each, which may have prevented obtaining significant results.
All these results suggest that a C starvation syndrome is induced early in the growth-to-dormancy transition in para- and ecodormant axillary buds in Arabidopsis.
To find potential master regulators of the C starvation-related bud dormancy GRNs, we searched for overrepresented motifs in the gene promoters (1 kilobase upstream of the transcription start site) of each GRN using Oligo-analysis and Pattern assembly (Rsat; Medina-Rivera et al., 2015). In GRNI and GRNIV, tcTTATCCAc was the most-overrepresented motif (Supplementary Figure S5 and Dataset S5); it contains the sucrose-repressible element TATCCA, bound in rice by the MYB factors OsMYBS1, 2, and 3, which mediate sugar-regulated gene expression (Lu et al., 1998, 2002). We looked for TFs within GRNI and IV that could bind this motif, based on DNA affinity purification sequencing (DAP-Seq) data (O’Malley et al., 2016) or chromatin immunoprecipitation sequencing (ChIP-Seq) data (Song et al., 2016), and that could act as master regulators of the GRNs. The Arabidopsis OsMYBS2 ortholog MYBS2 (At5g08520), which has a role in sugar and ABA signaling (Chen et al., 2016), pertains to GRNI and might bind this motif (Supplementary Figure S6A and Dataset S5). In GRNIV, MYBS2 and three other MYB-related proteins, MYBH/KUA1 (At5g47390), At1g19000 and At1g74840 could bind this motif (Supplementary Figure S6A and Dataset S5). MYBH/KUA1 has a critical role in dark-induced leaf senescence (Huang et al., 2015). These sugar-regulated genes could be instrumental in coordinating gene expression in GRNI and GRNIV.
The most overrepresented motifs in GRNII and III, gaCACGTGtc, tgaCACGT and gACACGT, overlap with the G-box (CACGTG), which is bound by bZIP, bHLH and NAC proteins (Supplementary Figure S5 and Dataset S5). These motifs are overrepresented in the promoters of C starvation response genes (Cookson et al., 2016). In GRNII, the master regulators of ABA signaling GBF2, GBF3, ABF3 and ABF4, and the senescence-inducing NAC factors NAP, NAC6/ORE1 and ATAF1 as well as NAC047 and NAC3 could bind these motifs (Supplementary Figure S6A and Dataset S5; Guo and Gan, 2006; Balazadeh et al., 2010; Garapati et al., 2015; O’Malley et al., 2016; Song et al., 2016). In GRNIII, NAP, NAC6/ORE1, NAC047, NAC3, NAC102, RD26 (Supplementary Figure S6A and Dataset S5) and possibly NAC19, for which there is no available binding information, might regulate these motifs and promote gene expression.
We confirmed significant enrichment of the GRNs in the target genes of these TFs by using DAP-Seq and ChIP-Seq data (O’Malley et al., 2016; Song et al., 2016); their numbers in the GRNs were significantly higher than expected in a random gene list (pval < 0.01). For instance, the number of gene targets for NAC102, RD26 and ABF4 was 6, 3.2, and 3.4 times higher, respectively; for the remainder, this value was between 1.7 and 2.7 times higher than predicted (Supplementary Figure S6B).
All these results indicate that four interrelated GRNs associated to a C starvation response are induced in para- and ecodormant Arabidopsis buds. MYB-related, bZIP and NAC TFs could have a key role in the regulation of these GRNs. A large proportion of the genes in the GRNs are rapidly repressed by sugar and upregulated by AKIN10. They are tightly coregulated with or directly involved in sugar signaling and metabolism, autophagy, senescence, catabolism of lipids and proteins, and ABA and ethylene signaling. This response is also associated with downregulation of CK signaling, protein synthesis and cell division, all conditions that lead to the cell and tissue growth arrest typical of dormant buds.
We investigated whether the GRNs related to a C starvation syndrome identified in Arabidopsis were also induced during the growth-to-dormancy transition in buds of the woody plant species, poplar and grapevine. We studied two public transcriptomic experiments in which apical buds of poplar (Ruttink et al., 2007) or axillary buds of grapevine (Díaz-Riquelme et al., 2012) underwent dormancy. To induce dormancy, shoot apices of poplar plants grown in long days (LD, 16 h light-8 h darkness) were exposed to 1–6 weeks of short days (w SD, 8 h light-16 h darkness) (Ruttink et al., 2007). During treatment, the shoot apices developed into buds (1–3 w SD), grew adapted to dehydration and cold (3–6 w SD), and became dormant (5–6 w SD). Samples were collected weekly. Díaz-Riquelme et al. (2012) collected monthly samples of axillary buds of grapevine plants grown in natural conditions in the northern hemisphere. Grapevine axillary buds are formed between April and May; in July and August they grow, undergo flowering and develop inflorescence meristems, enter endodormancy at the end of September, and exit dormancy by the end of November. They remain ecodormant throughout December, until environmental conditions become benign around March, when they sprout (Martìnez de Toda Fernaàndez, 1991; Díaz-Riquelme et al., 2012).
We analyzed the expression patterns of the poplar and grapevine orthologs of the GRNI-IV genes. Of 838 Arabidopsis genes in these GRNs, we identified 390 poplar and 421 grapevine orthologs (Supplementary Dataset S6). In both species, we studied gene expression relative to levels in the “active bud” sample (LD in poplar, April in grapevine). In general, a large proportion of the bud dormancy gene orthologs were significantly induced at most time points in poplar and grapevine buds (Supplementary Figure S7), which supports a conservation, during the growth-to-dormancy transition in these woody species, of the responses found in Arabidopsis. In poplar, the global induction appeared to increase over the weeks in SD, especially for GRNII and III genes. In contrast, grapevine gene induction was detectable throughout the year (Supplementary Figure S7).
A group of genes showed high expression levels from the earliest stages (1–3 w SD in poplar and July in grapevine), weeks/months before endodormancy onset, and throughout the experiment (Figure 4 and Supplementary Dataset S6). In poplar, these were DRM1, HIS1-3, GID1C, COR413IM1, ALANINE:GLYOXYLATE AMINOTRANSFERASE 3 (AGT3), SUCROSE SYNTHASE3 (SUS3), TEMPRANILLO1 (TEM1) and SEIPIN (Figure 4A). In grapevine, they were DRM1, HIS1-3, DIN6, PIF4, CBP1/MEE14, HSPRO2, EXL4, BCAT2 and ATY13/MYB31, ERF2, ABA receptor PYL9/ABI1, the protein phosphatases 2C HAI1/SAG113 and AIP1/HAI2, involved in ABA signaling and sucrose sensitivity (Lim et al., 2012), DOF5.4, HSFC1, PLANT U-BOX 19, GOLS1, senescence factor NAP, RD26, ALUMINUM SENSITIVE 3 (ALS3), and oxidative stress-related At3g10020 (Figure 4B).
FIGURE 4. Expression profiles of bud dormancy genes in poplar and grapevine. Heatmap of gene expression for poplar (A, Po_GRN) and grapevine (B, Vi_GRN) orthologs of the Arabidopsis GRN genes. Log2 ratios of normalized gene intensities in each time point vs. normalized gene intensities on the active bud sample are indicated. For poplar and grapevine the “active bud” sample are LD and April, respectively. In red and green, genes up- and downregulated in dormant buds respectively. Genes mentioned in the text are indicated. Schematic representations based on information from Ruttink et al. (2007) (A) and Díaz-Riquelme et al. (2012) (B), below indicate the proposed developmental stage of buds in each time point.
Other bud dormancy genes were induced exclusively between 1 and 3 w SD in poplar, and in July in grapevine. Early poplar genes were GASA6, the sugar-responsive gene CBP1/MEE14 (Bi et al., 2005), AKINBETA1 and LSD ONE LIKE 1 (LOL1), a positive regulator of cell death (Epple et al., 2003) (Figure 4A and Supplementary Dataset S6). July-induced grapevine genes were sugar transporters STP14 and STP1, KISS ME DEADLY 4/SKP20, which encodes an F-box protein that negatively regulates the CK response, autophagy factor ATG8I, chaperone DNAJ11, PLASMA-MEMBRANE ASSOCIATED CATION-BINDING PROTEIN 1 (PCaP1), ASD1, involved in cell wall remodeling (Chávez Montes et al., 2008), and ABA-responsive MYB3 (Figure 4B and Supplementary Dataset S6).
In summary, a large proportion of the genes orthologous to Arabidopsis bud dormancy genes are also induced, either early and transiently or early and constantly during the growth-to-dormancy transition in poplar and grapevine, which supports their functional conservation in these woody species.
To obtain a general view of the transcriptomic responses in these experiments, we performed GSEA similar to that for Arabidopsis, using all genes with proposed Arabidopsis orthologs (8023 genes in poplar and 8390 in grapevine) (Ruttink et al., 2007; Díaz-Riquelme et al., 2012).
The sugar- and AKIN10-responsive gene sets were overrepresented among upregulated genes from 1 w SD in poplar, and July in grapevine, and were also induced throughout the treatment/year (Figure 3). This finding confirms that the C starvation response begins early, long before endodormancy onset, and underlies the entire process. The ribosomal gene set was constitutively overrepresented among downregulated genes in all three species, which confirmed that inhibition of protein synthesis is an early and sustained response in buds entering dormancy. General downregulation of cell cycle and cell division genes was also observed in grapevine, whereas in poplar, cell division gene sets were repressed more gradually and reached maximum repression at 5 w SD. In contrast to Arabidopsis, histones were not significantly downregulated in the woody species. Nevertheless, C starvation response gene sets (upregulated) and cell growth-related gene sets (downregulated) clustered together in the three species.
Unlike the gene sets discussed above, hormone responses did not appear to be strongly conserved among species, suggesting more relaxed evolution of these pathways. Whereas ABA-related genes were induced constitutively in grapevine, ABA and ethylene responses were induced from 2 w SD onward in poplar, in accordance with previous observations (Ruttink et al., 2007). CK signaling is repressed in Arabidopsis, but not notably in poplar or grapevine. An early, extended response to the senescence-associated hormone jasmonate (MJ) was repressed in two Arabidopsis experiments, and was induced in most poplar and grapevine samples (Figure 3).
This results indicate that an early and sustained sugar-starvation response associated with downregulation of ribosomal and cell cycle proteins is conserved in buds of Arabidopsis, poplar and grapevine, and might constitute a core response of buds entering dormancy in the angiosperms.
To further analyze the function of the genes induced during the C starvation response in buds, we selected those most highly expressed in Arabidopsis, poplar and grapevine, to determine the cell types in which they are expressed. We used a high-resolution gene expression database of the Arabidopsis shoot apex, which contains the same tissues as axillary buds: meristem and leaf primordia. This database comprises gene expression profiles of different cell populations obtained by fluorescence-activated cell sorting (L1, L2 and L3 layers, central (CZ) and peripheral zone (PZ), leaf primordia, xylem and phloem) (Yadav et al., 2014). As we cannot rule out that the expression levels of these genes change in dormant axillary buds, we used this database for qualitative rather than quantitative analysis, to identify the cell types in which these genes were expressed most abundantly.
Many of the most highly induced genes in buds were expressed preferentially in the vasculature (Figures 5A–D); sugar-related genes SUC2, STP3, TPS11, GOLS1, and TF HB-40, HB-7, HB-12, PAT1 and CDF2 were expressed exclusively in phloem (Figure 5A). Many ABA-related genes (PYL9, HAI1, NAP, NAC055, NAC002, NFYA1, HAT22, RVE6, LEA4-5, DOF5.4, HIS1-3 and TSPO), SCR, the F box-encoding genes MAX2 and KMD2, CBP1/MEE14, AGT3, and CBSX5 were expressed almost exclusively in xylem (Figure 5B). Other ABA-related genes (ABF4, HAB1, HAI2, GBF3, RD26, MYB31, RAP2.3) as well as KING1, TPS10, TRE, XERICO, EXL2, KMD3, VOZ1, SIS and PUB19 were expressed in both xylem and phloem (Figures 5C,D). In the CLV3/WUS expression domain, we found TEM1, protein kinase CIPK14 that interacts with SnRK1 (Yan et al., 2014), SUS3, UDP-GLUCOSYL TRANSFERASE 87A2 (UGT87A2) and 6 PHOSPHOGLUCONOLACTONASE 1 (PGL1) (Figure 5E). The genes PIF1, PIF4, EXL4, ADAGIO (ADO1), ETR2 and COR413 IM1 accumulated preferentially in leaf primordia (Figure 5F). Other strongly expressed genes such as DRM1, PCAP1, KMD1, and AFP3 were found in both vascular tissue and leaf primordia, and GID1C in xylem and the peripheral zone of the meristem. The autophagy genes ATG8F, ATG8C, ATG18A, ATG18F, ATG18H, the senescence gene SAP3, as well as ERF2, GID1B, BYPASS and HISTONE DEACETYLASE 8 were widely expressed throughout the meristem.
FIGURE 5. Cell type-specific expression of bud dormancy genes in the Arabidopsis shoot apical meristem (SAM). Heatmap of bud dormancy gene expression in SAM cell types, normalized for each gene relative to the cell type with the highest expression levels (1.0, red). No expression (0.0), green. Different cell types are indicated on top. Horizontal color lines on the right indicate gene sets to which each gene belongs. (A) Genes expressed almost exclusively in phloem. (B) Genes expressed almost exclusively in xylem. (C,D) Genes expressed preferentially in phloem and xylem. (E) Genes expressed preferentially in layers 1, 2, 3 (L1, L2, L3), meristem (mer) and central zone (CZ). (F) Genes expressed preferentially in peripheral zone (PZ).
In summary, whereas ABA signaling occurs mostly in the xylem, sugar signaling in the phloem, and ethylene in the meristem proper (Yadav et al., 2014), autophagy and arrest of cell growth take place throughout the meristem. This suggests that cell-to-cell communication and movement of signaling molecules, hormones and proteins must take place across different cell types in buds entering dormancy.
It is of great interest to identify robust, universal markers that allow diagnosis of axillary bud status. These markers should be induced early, and their expression be sustained in para-, eco-, and endodormant buds throughout angiosperms. Based on our analysis, several genes met these criteria. We tested further four of them: DRM1, HIS1-3, GID1C, and NAP. DRM1 and HIS1-3 were expressed at high levels in the Arabidopsis, poplar and grapevine experiments. DRM1 is a well-known dormancy marker in herbaceous and woody plants species (Stafstrom et al., 1998; Park and Han, 2003; Tatematsu et al., 2005; Aguilar-Martínez et al., 2007; Kebrom et al., 2010; Wood et al., 2013). It is repressed by sugar, which supports its strong association to low sugar levels and dormancy. ABA-responsive HIS1-3 is upregulated before ABA signaling in poplar and grapevine buds. GID1C, which encodes a gibberellin receptor, is expressed at high levels in the three Arabidopsis experiments and in poplar (Figure 4A). The senescence-promoting gene NAP is expressed at very high levels in Arabidopsis and throughout the year in grapevine (Figure 4B). Both GID1C and NAP belong to the four bud dormancy GRNs (Supplementary Dataset S1).
We tested whether expression of these genes also correlated with bud dormancy in axillary buds of potato (Solanum tuberosum, Solanaceae, Asteridae), a species only distantly related to Arabidopsis, poplar (both Rosidae) and grapevine (basal angiosperms). We identified the potato ortholog genes for the four candidates. In the case of NAP, we found two potato paralogs (NAPa and NAPb). We studied their mRNA levels in buds of plants treated for 10 h with white light (W) or with W supplemented with far red light (W+FR), a treatment that promotes axillary bud dormancy in potato. We also compared mRNA levels in buds of intact and decapitated plants. Whereas DRM1 and HIS1-3 were confirmed as reliable markers of bud dormancy in potato, GID1C, NAPa and NAPb did not respond as anticipated in decapitated plants; GID1C was not upregulated in low R:FR and NAPa/NAPb were highly induced after decapitation (Figure 6).
FIGURE 6. Expression levels of candidate bud dormancy markers in potato. Transcript levels of DRM1, HIS1-3, GID1C and NAP potato ortholog genes in aerial axillary buds were analyzed by quantitative PCR. (A) Intact and decapitated plants, 10 h after treatment. (B) Plants exposed for 10 h to white light or to a low red:far-red light ratio. Values are mean ± SEM (NA = 5, NB = 4). Each biological replicate is a pool of 16 axillary buds. ∗∗pval < 0.01; ∗∗∗pval < 0.001; two-tailed Student’s t-test.
In all eukaryotes, cell proliferation and growth demand high carbohydrate levels for energy generation and macromolecule synthesis. Correspondingly, low C availability promotes a reduction in growth rate in order to retain sufficient C to support essential maintenance functions (Rolland et al., 2006). In Arabidopsis, sugar availability has a great influence on growth and development, both in seedlings and adult plants. C-limiting conditions (e.g., sucrose depletion, night extensions, short-day photoperiods, starchless mutants) trigger a suite of transcriptional responses that lead to growth cessation, and which include repression of genes involved in anabolism, protein synthesis, cell division, cell cycle, and DNA synthesis and repair (Moore et al., 2003; Smith and Stitt, 2007; Wiese et al., 2007).
Regarding shoot branching, it has been shown that sugar availability to buds plays a major role in its control in pea and rose (Mason et al., 2014; Barbier F. et al., 2015). In agreement, we have found that induction of GRNs typical of tissues undergoing C starvation precedes and underlies the bud growth-to-dormancy transition in Arabidopsis, poplar and grapevine. This is concomitant with transcriptional repression of ribosomal and cell-cycle genes, responses typical of buds entering dormancy as well as of tissues undergoing C limitations (e.g., Thimm et al., 2004; Smith and Stitt, 2007; González-Grandío et al., 2013). Indeed it is possible that bud dormancy is a manifestation and a consequence of the observed C starvation syndrome.
How is this C starvation response induced? In apical dominance it has been proposed that the growing shoot apex acting as a sugar sink might limit sugar availability to axillary buds so this can be the direct trigger of the response (Mason et al., 2014). Tre6P, a metabolite that acts as a proxy for C status, may also promote signaling in addition to, or instead of direct sugar sensing (Paul et al., 2008; Lunn et al., 2014). This would be in agreement with the observation that plants that express microbial trehalose-phosphate synthase (TPS) genes show increased shoot branching (Goddijn et al., 1997) and maize plants with a mutation in the trehalose-phosphate phosphatase gene RAMOSA3 have altered inflorescence branching (Satoh-Nagasawa et al., 2006).
Nevertheless, it is likely that the syndrome is not only induced by an actual sugar shortfall, but also by cues that inform of current or future suboptimal conditions which may affect energy availability and/or interfere with respiration and C assimilation (Baena-González et al., 2007; Baena-González and Sheen, 2008). Seasonal environmental changes that perturb these processes (e.g., daylength shortening, light levels, temperature, water availability) may trigger acclimatory signaling pathways that anticipate C limitations (Smith and Stitt, 2007). Those and other stimuli could feed into regulatory networks that economize resources locally, to result in a moderation of growth rate in axillary meristems and buds.
In two of the Arabidopsis experiments examined, the dormancy-inducing stimuli was an exposure to low R:FR light ratio. Low R:FR light is interpreted by plants as a situation with limited light available for photosynthesis. It severely reduces the expression of photosynthesis-related genes (Cagnola et al., 2012) and induces cell-wall remodeling in stem and petioles, which may divert carbohydrates away from axillary buds (Sasidharan et al., 2010). Furthermore, low R:FR promotes ethylene and ABA signaling and CK degradation (Carabelli et al., 2007; Cagnola et al., 2012), hormonal responses tightly linked to the C starvation response (see below).
In the poplar and grapevine studies, the sugar-repressed networks are induced in buds soon after beginning of daylength shortening: in poplar at 1 w SD; in grapevine, in July, when daylength shortening has just begun (June 21), even though buds are still growing. Short-day photoperiod leads to localized flower and seed abortion associated with low levels of C in Arabidopsis (Lauxmann et al., 2016). Likewise, in poplar a measurable shortage of sugar availability is detectable after 1 w SD (Ruttink et al., 2007). Under the natural conditions in which grapevine plants are grown, daylength shortening and C limitations are progressive, but relatively small changes in C balance may trigger the response. Indeed in Arabidopsis even minor alterations in C status, well before C starvation, lead to notable changes in C-related signaling and response (Usadel et al., 2008). In addition, genetic pathways that sense photoperiod might help anticipate and adapt to impending C-limiting conditions in short days. These pathways, controlled by phytochromes, circadian clock, and genes controlling flowering time (Horvath, 2009), may regulate and establish crosstalk with the C starvation response. Indeed, sugars affect the expression of clock genes (Haydon et al., 2013) and conversely, the clock regulates carbohydrate metabolism (Smith and Stitt, 2007). Phytochromes, which monitor changes in R:FR and in day-length, also regulate SD-induced endodormancy in woody species (Johnson et al., 1994; Reed et al., 1994; Olsen et al., 1997; Neff and Chory, 1998; Monte et al., 2003; Ruonala et al., 2008; Franklin and Quail, 2010). Changes in low R:FR light ratio or photoperiod might therefore trigger partially overlapping responses, including potential anticipation of a C-limiting situation.
Although it has not been analyzed in this work, coordination between C and N metabolic pathways probably affect this process as well, as sugar responses depend significantly on the N status of the plant.
The C starvation syndrome comprises a cascade of transcriptomic events that culminate in changes in growth and C balance (Figure 7). These events include induction of genes involved in transcriptional regulation, sugar sensing, transport and signaling, catabolism (i.e., amino acid and lipid degradation), protein ubiquitination and degradation, hormone signaling, autophagy and senescence. Genes required for growth, such as ribosomal, cell cycle and anabolism-related genes become downregulated (Thimm et al., 2004). Buds entering dormancy in Arabidopsis, poplar and grapevine show induction of genes of the former categories and repression of genes of the latter categories.
FIGURE 7. Summary of responses observed in buds entering dormancy in the context of a potential C starvation response. Relationships are based on data obtained in Arabidopsis (see Discussion). Some relevant Bud dormancy genes are indicated.
EXORDIUM-like (EXL)2 and EXL4 are bud dormancy genes potentially involved in sugar sensing. They are induced in extended night treatments in Arabidopsis seedlings, in accordance with a role under C-limiting conditions (Schröder et al., 2012). Their close paralogs, EXORDIUM (EXO) and EXL1, are proposed to integrate apoplastic C status with intracellular responses (EXO) (Lisso et al., 2013) and to control primary and long-term adaptation to C starvation (EXL1) (Schröder et al., 2011, 2012).
Several sugar transporters are also induced in dormant buds. These are STP1, one of the most rapidly and prominently downregulated genes in response to sugars (Price et al., 2004; Cordoba et al., 2015), STP14, which is strongly repressed by sugars (Büttner, 2010) and the sucrose efflux transporters SWEET11 and SWEET12, which act with the sucrose/proton symporter SUT1/SUC2 for phloem loading and long-distance transport (Chen et al., 2012).
Sugar signaling involves Tre6P (Paul et al., 2008; Lunn et al., 2014) and four class-II TPS, TPS8, TPS9, TPS10, and TPS11, are induced in buds entering dormancy. They belong to a core C-signaling response, are usually strongly upregulated in C starvation, and are AKIN10-responsive (Contento et al., 2004; Price et al., 2004; Thimm et al., 2004; Baena-González et al., 2007). Although their proteins may be catalytically inactive, they might modulate other TPSs or act as Tre6-P sensors (Lunn, 2007). In addition, the SnRK1 protein-kinase, a central regulator of growth in response to C availability (Baena-González et al., 2007), is likely to have a key role in the induction of bud dormancy (see below).
Ethylene and ABA signaling are induced during the bud dormancy transition in Arabidopsis, which agrees with studies that associated these hormones with bud dormancy in many other herbaceous and woody species (Suttle, 1998; Ruonala et al., 2006; Rohde and Bhalerao, 2007; Ruttink et al., 2007; Horvath et al., 2008; Díaz-Riquelme et al., 2012; González-Grandío et al., 2013, 2017; Reddy et al., 2013; González-Grandío and Cubas, 2014; Yao and Finlayson, 2015). We propose that ethylene and ABA responses are closely connected to the C starvation syndrome. Indeed, many mutants with altered responses to sugars have impaired ethylene or ABA signaling (Zhou et al., 1998; Arenas-Huertero et al., 2000; Finkelstein and Lynch, 2000; Huijser et al., 2000; Laby et al., 2000; Rook et al., 2001; Cheng et al., 2002; Yuan and Wysocka-Diller, 2006), and there is compelling evidence of crosstalk between sugar sensing and ethylene and ABA response. C starvation leads to induction of ethylene and ABA-related genes, whereas sugar treatment has the opposite effect (Laby et al., 2000; Rook et al., 2001; Brocard et al., 2002; León and Sheen, 2003; Yanagisawa et al., 2003; Thimm et al., 2004; Buchanan-Wollaston et al., 2005; Rolland et al., 2006). Thus C starvation signaling could trigger ethylene and ABA responses in buds. Indeed, the reduction in sugar levels detected in poplar buds during the 1 w SD is suggested to induce ethylene signaling, followed by ABA signaling (Ruttink et al., 2007).
One role of ABA and ethylene in the C starvation syndrome is induction of senescence, a genetically programmed process that promotes degradation of cell components and macromolecules, remobilizes nutrients, and optimizes resources to supply energy and C skeletons. Ethylene and ABA activate senescence-related genes and senescence induces ABA signaling (Abeles et al., 1988; Zeevaart and Creelman, 1988; Zacarias and Reid, 1990; Reid and Wu, 1992; Weaver et al., 1998; Seo et al., 2000; Yang et al., 2003; Buchanan-Wollaston et al., 2005; Lim et al., 2007). It is noteworthy that the potential master regulators of GRNII and III, ATAF1, ORE1/NAC6 and NAP, are ABA-induced factors that control senescence. ATAF1 induces a C starvation transcriptome and ABA biosynthesis (Jensen et al., 2013; Garapati et al., 2015). NAP activates SAG113/HAI1 and controls expression of ABSCISIC ALDEHYDE OXIDASE3 (AAO3), encoding an enzyme that catalyzes the final steps of ABA synthesis (Guo and Gan, 2006; Yang et al., 2014). ORE1 controls the expression of at least 78 SAGs and might also promote DNA degradation (Balazadeh et al., 2010; Matallana-Ramirez et al., 2013; Kim et al., 2014). HAT22, GBF2, GBF3, ABF3 and ABF4 are additional bud dormancy genes related both to senescence and ABA (Lin and Wu, 2004; Rivero et al., 2007; Song et al., 2016). Remarkably, MAX2/ORE9, which encodes an F-box involved directly in strigolactone perception and signaling and has a critical role in the control of shoot branching, also promotes senescence (Woo et al., 2001; Stirnberg et al., 2002). Finally, the MYB genes At1g19000 and At1g74840, proposed to be master regulators of GRNI and IV, are responsive to dark-induced senescence (Lin and Wu, 2004).
In contrast, CK signaling is antagonistic to senescence, and a reduction in CK levels is a key signal for senescence initiation in Arabidopsis (Gan and Amasino, 1995; Kim et al., 2006). Consistent with this, in Arabidopsis dormant buds CK signaling is reduced, and in other species CK levels have also been reported to be reduced relative to active buds (Turnbull et al., 1997; Dun et al., 2012; Roman et al., 2016). Consistently, four genes encoding F-box proteins that promote the ubiquitination and degradation of ARR factors [KISS ME DEADLY (KMD)1-4] are bud dormancy genes.
Autophagy is another process induced by C starvation (Izumi et al., 2013) and whose markers (ATG genes) are upregulated in buds entering dormancy. This is a process by which cytoplasmic components and organelles are transported to the vacuole, where they are broken down and recycled. Under C-limiting conditions it contributes to plant energy availability (Aubert et al., 1996; Rose et al., 2006; Izumi et al., 2013). Autophagy is associated with induction of lipid degradation and upregulation of E2- and E3-ubiquitin ligase components, which promote proteasomal-dependent protein degradation (Thompson and Vierstra, 2005). We have found a remarkable number of bud dormancy genes related to autophagy, ubiquitination, protein degradation and lipid catabolism, many of them controlled by SnRK1 (see below).
SnRK1, a protein-kinase active in low energy conditions, promotes catabolism and represses anabolism, cell division and growth. Our transcriptomic data indicates that it may play an important role during the bud growth-to-dormancy transition. SnRK1 affects expression of robust dormancy markers such HIS1.3 and DRM1, and the potential master regulator of GRNI and GRNIV, MYBH/KUA1. In buds entering dormancy, the SnRK1 β subunit AKINBETA1, whose mRNA levels correlate directly with night duration (Pokhilko et al., 2014), is induced. Most importantly, our GSEA analysis indicates that the transcriptional network downstream of the catalytic SnRK1 α subunit, AKIN10, is significantly induced from the earliest stages of growth-to-dormancy transition in Arabidopsis, poplar and grapevine buds, and is maintained in para-, eco- and endodormant buds. Many of the abovementioned genes involved in sugar sensing, signaling, autophagy and repression of CK signaling are AKIN10-dependent, including EXL4, STP1/14, SWEET11/12, TPS8/9/19/11, AKINBETA, ATG8E/F/G/H, ATG18F/G, and F-box genes KMD1, 3, 4. SnRK1 also causes downregulation of a large number of ribosomal genes, another conserved significant effect detected by our GSEA analysis. SnRK1 could also be responsible for at least part of the observed induction of the ubiquitination machinery and lipid degradation.
Bud dormancy is an adaptive response present in all angiosperms. It prevents shoot development when endogenous or environmental conditions are unfavorable for sustained growth. It has great impact on reproductive success, productivity and survival, and must have been influential in the colonization of habitats with fluctuating conditions.
We have found induction of a conserved C starvation syndrome that precedes and underlies the growth-to-dormancy transition in buds of three distantly-related species, one herbaceous (Arabidopsis) and two woody (poplar and grapevine). This transcriptional response, composed by several interconnected GRNs, comprises ortholog genes in Arabidopsis, poplar and grapevine, as gene sets generated in Arabidopsis were used to detect the response in the woody species. Furthermore, this syndrome has been observed is several unrelated experiments, regardless the stimulus that promoted dormancy, either environmental (low R:FR, short-day photoperiods) or endogenous (apical dominance). This remarkable conservation suggests that a syndrome aimed at adapting to C-limiting situations is deeply rooted in the control of shoot meristem and bud development across angiosperms. Bud dormancy might thus be an ancestral response directly resulting from this C starvation syndrome, coordinated by different pathways that sense and/or anticipate situations on low C availability and feed into this core response to prevent untimely growth and development.
Bud dormancy genes (Supplementary Figure S1) were obtained from González-Grandío and Cubas (2014). Coregulation of the 78 bud dormancy genes was analyzed by hierarchical clustering (Hcluster, ATTED-II, Obayashi et al., 2007). Additional coregulated genes were obtained using CoEx-Search (ATTED-II, Obayashi et al., 2007). The 300 genes most coregulated with each cluster were selected. These genes were validated for induction in dormant buds in the original arrays (Tatematsu et al., 2005; González-Grandío et al., 2013; Reddy et al., 2013). Only genes upregulated (positive fold change FC ≥ 1.2) in at least one experiment in dormant buds were included in the lists of bud dormancy GRNs (Supplementary Figure S8).
Automated function prediction for the GRNs was carried out using GO analyses. The PANTHER classification system (Mi et al., 2017) was used to identify overrepresented biological process ontologies using a statistical overrepresentation test followed by Bonferroni correction for multiple testing. TAIR10 version of Arabidopsis thaliana genome was used as reference. We selected ontologies with a pval < 0.05. In addition, Mapman bins (Thimm et al., 2004) were added to all the genes in Supplementary Dataset S1.
Gene Set Enrichment Analysis (Subramanian et al., 2005) was used to identify gene sets whose genes are overrepresented in different conditions. The GSEA method evaluates whether these genes occur preferentially toward the top or bottom of a ranked list. Enrichment scores are calculated using “weighted” statistics. For each sample, we calculated the log2 ratios of normalized gene intensities vs. normalized gene intensities of the “active bud” sample: white light-treated buds for González-Grandío et al. (2013); High R:FR-treated n-2 buds for Reddy et al. (2013); Buds of decapitated plants for Tatematsu et al. (2005); Buds of LD-grown poplars for Ruttink et al. (2007), April grapevine buds for Díaz-Riquelme et al. (2012). Genes were ranked by their log2 ratios calculated as the difference between normalized log intensity in the “dormant bud” condition minus normalized log2 intensity in the “active bud” condition. Intensity expression values were obtained from the references above. Gene sets for hormone markers were obtained from Nemhauser et al. (2006) and Mashiguchi et al. (2009). Gene sets related to sugar and AKIN10 responses were obtained from Sulpice et al. (2009) (Core C-signaling), Osuna et al. (2007) (Sugar-responsive), Gonzali et al. (2006) (Dark-induced, sugar-repressed), and Baena-González et al. (2007) (AKIN10-responsive). The other gene sets are from González-Grandío et al. (2013). The GSEA Normalized Enrichment Score for all gene sets in all comparisons were clustered with TM4 Multi Experiment Viewer (MeV, Saeed et al., 2003). Tree was generated by the hierarchical clustering method (HCL) using Euclidean distance and average linkage options. Complete results are in http://bioinfogp.cnb.csic.es/files/projects/tarancon_et_al_2017_supp/.
Sequences (1 kb) 5′ of the transcription start site of the bud dormancy GRN genes were retrieved with Sequence Bulk Download2. Overrepresented 6-8mer motifs were identified with Motif discovery (RSAT, Medina-Rivera et al., 2015). The oligo-analysis tool was used to find significantly overrepresented motifs, which were assembled into frequency matrices with pattern-assembly and default parameters. Matrices were converted into consensus motifs with convert-matrix and represented using WebLogo (Crooks et al., 2004).
For each time point we calculated the log2 ratios of normalized gene intensities vs. normalized gene intensities on LD (active buds). Expression data was visualized and clustered with MeV. Tree was generated by HCL, using Euclidean distance and average linkage options.
For each sample, we calculated the log2 ratios of normalized gene intensities vs. normalized gene intensities of the “active bud” sample: LD for poplar, April for grapevine. Expression data for selected bud dormancy genes obtained from Yadav et al. (2014) was visualized and clustered with MeV. Trees were generated by HCL using Euclidean distance and average linkage options.
The putative orthologs of Arabidopsis genes were identified by a tblastn search with protein sequences as query in the Spud DB Potato Genomics Resource website3. cDNAs showing a high similarity e-value with the query were selected. Proteins were aligned with those of Arabidopsis and phylogenetic trees (BioNeighbor joining method, 500 replicates; Gascuel, 1997) were built to identify the most likely orthologs, which were selected for expression studies (Supplementary Figure S9).
Plant growth conditions, experimental design, light treatments, techniques and expression level normalization were as described in Nicolas et al. (2015). For each biological replicate, 8 axillary buds from node 2, and 8 from node 3 were dissected (node 1 = lowest plant node); 4–5 biological replicates were collected for each condition. Primers used are listed in Suplemmentary Table S1.
CT, EG-G, JO, and MN, performed experiments. PC, performed experiments, and wrote the manuscript.
The authors declare that the research was conducted in the absence of any commercial or financial relationships that could be construed as a potential conflict of interest.
We thank Desmond Bradley and Elena Baena for constructive criticisms of the manuscript and Catherine Mark for editorial assistance. PC is supported by a MINECO grant (BIO2014-57011-R). CT is a La Caixa predoctoral fellow. EG-G was a predoctoral fellow of Fundación Ramón Areces and a CSIC JAE-Predoc fellow. MN is a Excelence Severo Ochoa (MINECO) postdoctoral researcher.
The Supplementary Material for this article can be found online at: http://journal.frontiersin.org/article/10.3389/fpls.2017.00788/full#supplementary-material
Abeles, F. B., Dunn, L. J., Morgens, P., Callahan, A., Dinterman, R. E., and Schmidt, J. (1988). Induction of 33-kD and 60-kD Peroxidases during Ethylene-induced senescence of cucumber cotyledons. Plant Physiol. 87, 609–615. doi: 10.1104/pp.87.3.609
Aguilar-Martínez, J. A., Poza-Carrión, C., and Cubas, P. (2007). Arabidopsis BRANCHED1 acts as an integrator of branching signals within axillary buds. Plant Cell 19, 458–472. doi: 10.1105/tpc.106.048934
Arenas-Huertero, F., Arroyo, A., Zhou, L., Sheen, J., and León, P. (2000). Analysis of Arabidopsis glucose insensitive mutants, gin5 and gin6, reveals a central role of the plant hormone ABA in the regulation of plant vegetative development by sugar. Genes Dev. 14, 2085–2096.
Aubert, S., Gout, E., Bligny, R., Marty-Mazars, D., Barrieu, F., Alabouvette, J., et al. (1996). Ultrastructural and biochemical characterization of autophagy in higher plant cells subjected to carbon deprivation: control by the supply of mitochondria with respiratory substrates. J. Cell Biol. 133, 1251–1263. doi: 10.1083/jcb.133.6.1251
Baena-González, E., Rolland, F., Thevelein, J. M., and Sheen, J. (2007). A central integrator of transcription networks in plant stress and energy signalling. Nature 448, 938–942. doi: 10.1038/nature06069
Baena-González, E., and Sheen, J. (2008). Convergent energy and stress signaling. Trends Plant Sci. 13, 474–482. doi: 10.1016/j.tplants.2008.06.006
Balazadeh, S., Siddiqui, H., Allu, A. D., Matallana-Ramirez, L. P., Caldana, C., Mehrnia, M., et al. (2010). A gene regulatory network controlled by the NAC transcription factor ANAC092/AtNAC2/ORE1 during salt-promoted senescence. Plant J. 62, 250–264. doi: 10.1111/j.1365-313X.2010.04151.x
Barbier, F., Péron, T., Lecerf, M., Perez-Garcia, M.-D., Barrière, Q., Rolčík, J., et al. (2015). Sucrose is an early modulator of the key hormonal mechanisms controlling bud outgrowth in Rosa hybrida. J. Exp. Bot. 66, 2569–2582. doi: 10.1093/jxb/erv047
Barbier, F. F., Lunn, J. E., and Beveridge, C. A. (2015). Ready, steady, go! A sugar hit starts the race to shoot branching. Curr. Opin. Plant Biol. 25, 39–45. doi: 10.1016/j.pbi.2015.04.004
Bi, Y.-M., Zhang, Y., Signorelli, T., Zhao, R., Zhu, T., and Rothstein, S. (2005). Genetic analysis of Arabidopsis GATA transcription factor gene family reveals a nitrate-inducible member important for chlorophyll synthesis and glucose sensitivity. Plant J. 44, 680–692. doi: 10.1111/j.1365-313X.2005.02568.x
Brocard, I. M., Lynch, T. J., and Finkelstein, R. R. (2002). Regulation and role of the Arabidopsis abscisic acid-insensitive 5 gene in abscisic acid, sugar, and stress response. Plant Physiol. 129, 1533–1543. doi: 10.1104/pp.005793
Buchanan-Wollaston, V., Page, T., Harrison, E., Breeze, E., Lim, P. O., Nam, H. G., et al. (2005). Comparative transcriptome analysis reveals significant differences in gene expression and signalling pathways between developmental and dark/starvation-induced senescence in Arabidopsis. Plant J. 42, 567–585. doi: 10.1111/j.1365-313X.2005.02399.x
Büttner, M. (2010). The Arabidopsis sugar transporter (AtSTP) family: an update. Plant Biol. 12, 35–41. doi: 10.1111/j.1438-8677.2010.00383.x
Cagnola, J. I., Ploschuk, E., Benech-Arnold, T., Finlayson, S. A., and Casal, J. J. (2012). Stem transcriptome reveals mechanisms to reduce the energetic cost of shade-avoidance responses in tomato. Plant Physiol. 160, 1110–1119. doi: 10.1104/pp.112.201921
Carabelli, M., Possenti, M., Sessa, G., Ciolfi, A., Sassi, M., Morelli, G., et al. (2007). Canopy shade causes a rapid and transient arrest in leaf development through auxin-induced cytokinin oxidase activity. Genes Dev. 21, 1863–1868. doi: 10.1101/gad.432607
Chávez Montes, R. A., Ranocha, P., Martinez, Y., Minic, Z., Jouanin, L., Marquis, M., et al. (2008). Cell wall modifications in Arabidopsis plants with altered alpha-L-arabinofuranosidase activity. Plant Physiol. 147, 63–77. doi: 10.1104/pp.107.110023
Chen, L.-Q., Qu, X.-Q., Hou, B.-H., Sosso, D., Osorio, S., Fernie, A. R., et al. (2012). Sucrose efflux mediated by SWEET proteins as a key step for phloem transport. Science 335, 207–211. doi: 10.1126/science.1213351
Chen, Y.-S., Chao, Y.-C., Tseng, T.-W., Huang, C.-K., Lo, P.-C., and Lu, C.-A. (2016). Two MYB-related transcription factors play opposite roles in sugar signaling in Arabidopsis. Plant Mol. Biol. 93, 299–311. doi: 10.1007/s11103-016-0562-8
Cheng, W.-H., Endo, A., Zhou, L., Penney, J., Chen, H.-C., Arroyo, A., et al. (2002). A unique short-chain dehydrogenase/reductase in Arabidopsis glucose signaling and abscisic acid biosynthesis and functions. Plant Cell 14, 2723–2743. doi: 10.1105/tpc.006494
Contento, A. L., Kim, S.-J., and Bassham, D. C. (2004). Transcriptome profiling of the response of Arabidopsis suspension culture cells to Suc starvation. Plant Physiol. 135, 2330–2347. doi: 10.1104/pp.104.044362
Cookson, S. J., Yadav, U. P., Klie, S., Morcuende, R., Usadel, B., Lunn, J. E., et al. (2016). Temporal kinetics of the transcriptional response to carbon depletion and sucrose readdition in Arabidopsis seedlings. Plant. Cell Environ. 39, 768–786. doi: 10.1111/pce.12642
Cordoba, E., Aceves-Zamudio, D. L., Hernandez-Bernal, A. F., Ramos-Vega, M., and Leon, P. (2015). Sugar regulation of SUGAR TRANSPORTER PROTEIN 1 (STP1) expression in Arabidopsis thaliana. J. Exp. Bot. 66, 147–159. doi: 10.1093/jxb/eru394
Crooks, G. E., Hon, G., Chandonia, J.-M., and Brenner, S. E. (2004). WebLogo: a sequence logo generator. Genome Res. 14, 1188–1190. doi: 10.1101/gr.849004
Díaz-Riquelme, J., Grimplet, J., Martínez-Zapater, J. M., and Carmona, M. J. (2012). Transcriptome variation along bud development in grapevine (Vitis vinifera L.). BMC Plant Biol. 12:181. doi: 10.1186/1471-2229-12-181
Doebley, J., Stec, A., and Hubbard, L. (1997). The evolution of apical dominance in maize. Nature 386, 485–488. doi: 10.1038/386485a0
Dun, E. A., de Saint Germain, A., Rameau, C., and Beveridge, C. A. (2012). Antagonistic action of strigolactone and cytokinin in bud outgrowth control. Plant Physiol. 158, 487–498. doi: 10.1104/pp.111.186783
Epple, P., Mack, A. A., Morris, V. R. F., and Dangl, J. L. (2003). Antagonistic control of oxidative stress-induced cell death in Arabidopsis by two related, plant-specific zinc finger proteins. Proc. Natl. Acad. Sci. U.S.A. 100, 6831–6836. doi: 10.1073/pnas.1130421100
Fennell, A. Y., Schlauch, K. A., Gouthu, S., Deluc, L. G., Khadka, V., Sreekantan, L., et al. (2015). Short day transcriptomic programming during induction of dormancy in grapevine. Front. Plant Sci. 6:834. doi: 10.3389/fpls.2015.00834
Finkelstein, R. R., and Lynch, T. J. (2000). The Arabidopsis abscisic acid response gene ABI5 encodes a basic leucine zipper transcription factor. Plant Cell 12, 599–609. doi: 10.1105/tpc.12.4.599
Finlayson, S. A. (2007). Arabidopsis Teosinte Branched1-like 1 regulates axillary bud outgrowth and is homologous to monocot Teosinte Branched1. Plant Cell Physiol. 48, 667–677. doi: 10.1093/pcp/pcm044
Franklin, K. A., and Quail, P. H. (2010). Phytochrome functions in Arabidopsis development. J. Exp. Bot. 61, 11–24. doi: 10.1093/jxb/erp304
Gan, S., and Amasino, R. M. (1995). Inhibition of leaf senescence by autoregulated production of cytokinin. Science 270, 1986–1988. doi: 10.1126/science.270.5244.1986
Garapati, P., Feil, R., Lunn, J. E., Van Dijck, P., Balazadeh, S., and Mueller-Roeber, B. (2015). Transcription factor Arabidopsis activating factor1 integrates carbon starvation responses with trehalose metabolism. Plant Physiol. 169, 379–390. doi: 10.1104/pp.15.00917
Gascuel, O. (1997). BIONJ: an improved version of the NJ algorithm based on a simple model of sequence data. Mol. Biol. Evol. 14, 685–695. doi: 10.1093/oxfordjournals.molbev.a025808
Goddijn, O. J., Verwoerd, T. C., Voogd, E., Krutwagen, R. W., de Graaf, P. T., van Dun, K., et al. (1997). Inhibition of trehalase activity enhances trehalose accumulation in transgenic plants. Plant Physiol. 113, 181–190. doi: 10.1104/pp.113.1.181
González-Grandío, E., and Cubas, P. (2014). Identification of gene functions associated to active and dormant buds in Arabidopsis. Plant Signal. Behav. 9, 19–21. doi: 10.4161/psb.27994
González-Grandío, E., Pajoro, A., Franco-Zorrilla, J. M., Tarancón, C., Immink, R. G. H., and Cubas, P. (2017). Abscisic acid signaling is controlled by a BRANCHED1/HD-ZIP I cascade in Arabidopsis axillary buds. Proc. Natl. Acad. Sci. U.S.A. 114, E245–E254. doi: 10.1073/pnas.1613199114
González-Grandío, E., Poza-Carrión, C., Sorzano, C. O. S., and Cubas, P. (2013). BRANCHED1 promotes axillary bud dormancy in response to shade in Arabidopsis. Plant Cell 25, 834–850. doi: 10.1105/tpc.112.108480
Gonzali, S., Loreti, E., Solfanelli, C., Novi, G., Alpi, A., and Perata, P. (2006). Identification of sugar-modulated genes and evidence for in vivo sugar sensing in Arabidopsis. J. Plant Res. 119, 115–123. doi: 10.1007/s10265-005-0251-1
Guo, Y., and Gan, S. (2006). AtNAP, a NAC family transcription factor, has an important role in leaf senescence. Plant J. 46, 601–612. doi: 10.1111/j.1365-313X.2006.02723.x
Hao, X., Yang, Y., Yue, C., Wang, L., Horvath, D. P., and Wang, X. (2017). Comprehensive transcriptome analyses reveal differential gene expression profiles of Camellia sinensis axillary buds at Para-, Endo-, ecodormancy, and bud flush stages. Front. Plant Sci. 8:553. doi: 10.3389/fpls.2017.00553
Haydon, M. J., Mielczarek, O., Robertson, F. C., Hubbard, K. E., and Webb, A. A. R. (2013). Photosynthetic entrainment of the Arabidopsis thaliana circadian clock. Nature 502, 689–692. doi: 10.1038/nature12603
Horvath, D. (2009). Common mechanisms regulate flowering and dormancy. Plant Sci. 177, 523–531. doi: 10.1016/j.plantsci.2009.09.002
Horvath, D. P., Chao, W. S., Suttle, J. C., Thimmapuram, J., and Anderson, J. V. (2008). Transcriptome analysis identifies novel responses and potential regulatory genes involved in seasonal dormancy transitions of leafy spurge (Euphorbia esula L.). BMC Genomics 9:536. doi: 10.1186/1471-2164-9-536
Howe, G. T., Horvath, D. P., Dharmawardhana, P., Priest, H. D., Mockler, T. C., and Strauss, S. H. (2015). Extensive transcriptome changes during natural onset and release of vegetative bud dormancy in Populus. Front. Plant Sci. 6:989. doi: 10.3389/fpls.2015.00989
Huang, C.-K., Lo, P.-C., Huang, L.-F., Wu, S.-J., Yeh, C.-H., and Lu, C.-A. (2015). A single-repeat MYB transcription repressor, MYBH, participates in regulation of leaf senescence in Arabidopsis. Plant Mol. Biol. 88, 269–286. doi: 10.1007/s11103-015-0321-2
Huijser, C., Kortstee, A., Pego, J., Weisbeek, P., Wisman, E., and Smeekens, S. (2000). The Arabidopsis SUCROSE UNCOUPLED-6 gene is identical to ABSCISIC ACID INSENSITIVE-4: involvement of abscisic acid in sugar responses. Plant J. 23, 577–585. doi: 10.1046/j.1365-313x.2000.00822.x
Izumi, M., Hidema, J., Makino, A., and Ishida, H. (2013). Autophagy contributes to nighttime energy availability for growth in Arabidopsis. Plant Physiol. 161, 1682–1693. doi: 10.1104/pp.113.215632
Jensen, M. K., Lindemose, S., Masi, F., de Reimer, J. J., Nielsen, M., Perera, V., et al. (2013). ATAF1 transcription factor directly regulates abscisic acid biosynthetic gene NCED3 in Arabidopsis thaliana. FEBS Open Bio 3, 321–327. doi: 10.1016/j.fob.2013.07.006
Johnson, E., Bradley, M., Harberd, N. P., and Whitelam, G. C. (1994). Photoresponses of light-grown phyA mutants of Arabidopsis (phytochrome a is required for the perception of daylength extensions). Plant Physiol. 105, 141–149. doi: 10.1104/pp.105.1.141
Kebrom, T. H., Brutnell, T. P., and Finlayson, S. A. (2010). Suppression of sorghum axillary bud outgrowth by shade, phyB and defoliation signalling pathways. Plant Cell Environ. 33, 48–58. doi: 10.1111/j.1365-3040.2009.02050.x
Kim, H. J., Hong, S. H., Kim, Y. W., Lee, I. H., Jun, J. H., Phee, B.-K., et al. (2014). Gene regulatory cascade of senescence-associated NAC transcription factors activated by ETHYLENE-INSENSITIVE2-mediated leaf senescence signalling in Arabidopsis. J. Exp. Bot. 65, 4023–4036. doi: 10.1093/jxb/eru112
Kim, H. J., Ryu, H., Hong, S. H., Woo, H. R., Lim, P. O., Lee, I. C., et al. (2006). Cytokinin-mediated control of leaf longevity by AHK3 through phosphorylation of ARR2 in Arabidopsis. Proc. Natl. Acad. Sci. U.S.A. 103, 814–819. doi: 10.1073/pnas.0505150103
Laby, R. J., Kincaid, M. S., Kim, D., and Gibson, S. I. (2000). The Arabidopsis sugar-insensitive mutants sis4 and sis5 are defective in abscisic acid synthesis and response. Plant J. 23, 587–596. doi: 10.1046/j.1365-313x.2000.00833.x
Lang, G. A., Early, J. D., Arroyave, N. J., Darnell, R. L., Martin, G. C., and Stutte, G. W. (1985). Dormancy: toward a reduced, universal terminology. HortScience 20, 809–811.
Lang, G. A., Early, J. D., Martin, G. C., and Darnell, R. L. (1987). Endo-, para-, and ecodormancy: physiological terminology and classification for dormancy research. HortScience 22, 371–377.
Lauxmann, M. A., Annunziata, M. G., Brunoud, G., Wahl, V., Koczut, A., Burgos, A., et al. (2016). Reproductive failure in Arabidopsis thaliana under transient carbohydrate limitation: flowers and very young siliques are jettisoned and the meristem is maintained to allow successful resumption of reproductive growth. Plant Cell Environ. 39, 745–767. doi: 10.1111/pce.12634
León, P., and Sheen, J. (2003). Sugar and hormone connections. Trends Plant Sci. 8, 110–116. doi: 10.1016/S1360-1385(03)00011-6
Lim, C. W., Kim, J.-H., Baek, W., Kim, B. S., and Lee, S. C. (2012). Functional roles of the protein phosphatase 2C, AtAIP1, in abscisic acid signaling and sugar tolerance in Arabidopsis. Plant Sci. 187, 83–88. doi: 10.1016/j.plantsci.2012.01.013
Lim, P. O., Kim, H. J., and Gil Nam, H. (2007). Leaf Senescence. Annu. Rev. Plant Biol. 58, 115–136. doi: 10.1146/annurev.arplant.57.032905.105316
Lin, J.-F., and Wu, S.-H. (2004). Molecular events in senescing Arabidopsis leaves. Plant J. 39, 612–628. doi: 10.1111/j.1365-313X.2004.02160.x
Lisso, J., Schröder, F., and Müssig, C. (2013). EXO modifies sucrose and trehalose responses and connects the extracellular carbon status to growth. Front. Plant Sci. 4:219. doi: 10.3389/fpls.2013.00219
Lu, C.-A., Ho, T. D., Ho, S.-L., and Yu, S.-M. (2002). Three novel MYB proteins with one DNA binding repeat mediate sugar and hormone regulation of alpha-amylase gene expression. Plant Cell 14, 1963–1980. doi: 10.1105/tpc.001735
Lu, C. A., Lim, E. K., and Yu, S. M. (1998). Sugar response sequence in the promoter of a rice alpha-amylase gene serves as a transcriptional enhancer. J. Biol. Chem. 273, 10120–10131. doi: 10.1074/jbc.273.17.10120
Lunn, J. E. (2007). Gene families and evolution of trehalose metabolism in plants. Funct. Plant Biol. 34, 550. doi: 10.1071/fp06315
Lunn, J. E., Delorge, I., Figueroa, C. M., Van Dijck, P., and Stitt, M. (2014). Trehalose metabolism in plants. Plant J. 79, 544–567. doi: 10.1111/tpj.12509
Martìnez de Toda Fernaàndez, F. (1991). Biología de la Vid: Fundamentos Biológicos de la Viticultura. Madrid: Mundi-Prensa.
Mashiguchi, K., Sasaki, E., Shimada, Y., Nagae, M., Ueno, K., Nakano, T., et al. (2009). Feedback-regulation of strigolactone biosynthetic genes and strigolactone-regulated genes in Arabidopsis. Biosci. Biotechnol. Biochem. 73, 2460–2465. doi: 10.1271/bbb.90443
Mason, M. G., Ross, J. J., Babst, B. A., Wienclaw, B. N., and Beveridge, C. A. (2014). Sugar demand, not auxin, is the initial regulator of apical dominance. Proc. Natl. Acad. Sci. U.S.A. 111, 6092–6097. doi: 10.1073/pnas.1322045111
Matallana-Ramirez, L. P., Rauf, M., Farage-Barhom, S., Dortay, H., Xue, G. P., Dröge-Laser, W., et al. (2013). NAC transcription factor ORE1 and doi: senescence-induce BIFUNCTIONAL NUCLEASE1 (BFN1) constitute a regulatory cascade in Arabidopsis. Mol. Plant 6, 1438–1452. doi: 10.1093/mp/sst012
Medina-Rivera, A., Defrance, M., Sand, O., Herrmann, C., Castro-Mondragon, J. A., Delerce, J., et al. (2015). RSAT 2015: regulatory sequence analysis tools. Nucleic Acids Res. 43, W50–W56. doi: 10.1093/nar/gkv362
Mi, H., Huang, X., Muruganujan, A., Tang, H., Mills, C., Kang, D., et al. (2017). PANTHER version 11: expanded annotation data from gene ontology and reactome pathways, and data analysis tool enhancements. Nucleic Acids Res. 45, D183–D189. doi: 10.1093/nar/gkw1138
Monte, E., Alonso, J. M., Ecker, J. R., Zhang, Y., Li, X., Young, J., et al. (2003). Isolation and characterization of phyC mutants in Arabidopsis reveals complex crosstalk between phytochrome signaling pathways. Plant Cell 15, 1962–1980. doi: 10.1105/tpc.012971
Moore, B., Zhou, L., Rolland, F., Hall, Q., Cheng, W.-H., Liu, Y.-X., et al. (2003). Role of the Arabidopsis glucose sensor HXK1 in nutrient, light, and hormonal signaling. Science 300, 332–336. doi: 10.1126/science.1080585
Neff, M. M., and Chory, J. (1998). Genetic interactions between phytochrome A, phytochrome B, and cryptochrome 1 during Arabidopsis development. Plant Physiol. 118, 27–35. doi: 10.1104/pp.118.1.27
Nemhauser, J. L., Hong, F., and Chory, J. (2006). Different plant hormones regulate similar processes through largely nonoverlapping transcriptional responses. Cell 126, 467–475. doi: 10.1016/j.cell.2006.05.050
Nicolas, M., and Cubas, P. (2015). “TCP transcription factors: evolution, structure, and biochemical function,” in Plant Transcription Factors, ed. D. H. Gonzalez (Amstredam: Elsevier), 250–267.
Nicolas, M., Rodríguez-Buey, M. L., Franco-Zorrilla, J. M., and Cubas, P. (2015). A recently evolved alternative splice site in the BRANCHED1a gene controls potato plant architecture. Curr. Biol. 25, 1799–1809. doi: 10.1016/j.cub.2015.05.053
Obayashi, T., Kinoshita, K., Nakai, K., Shibaoka, M., Hayashi, S., Saeki, M., et al. (2007). ATTED-II: a database of co-expressed genes and cis elements for identifying co-regulated gene groups in Arabidopsis. Nucleic Acids Res. 35, D863–D869. doi: 10.1093/nar/gkl783
Olsen, J. E., Junttila, O., Nilsen, J., Eriksson, M. E., Martinussen, I., Olsson, O., et al. (1997). Ectopic expression of oat phytochrome A in hybrid aspen changes critical daylength for growth and prevents cold acclimatization. Plant J. 12, 1339–1350. doi: 10.1046/j.1365-313x.1997.12061339.x
O’Malley, R. C., Huang, S. C., Song, L., Lewsey, M. G., Bartlett, A., Nery, J. R., et al. (2016). Cistrome and epicistrome features shape the regulatory DNA landscape. Cell 165, 1280–1292. doi: 10.1016/j.cell.2016.04.038
Osuna, D., Usadel, B., Morcuende, R., Gibon, Y., Bläsing, O. E., Höhne, M., et al. (2007). Temporal responses of transcripts, enzyme activities and metabolites after adding sucrose to carbon-deprived Arabidopsis seedlings. Plant J. 49, 463–491. doi: 10.1111/j.1365-313X.2006.02979.x
Park, S., and Han, K.-H. (2003). An auxin-repressed gene (RpARP) from black locust (Robinia pseudoacacia) is posttranscriptionally regulated and negatively associated with shoot elongation. Tree Physiol. 23, 815–823. doi: 10.1093/treephys/23.12.815
Paul, M. J., Primavesi, L. F., Jhurreea, D., and Zhang, Y. (2008). Trehalose metabolism and signaling. Annu. Rev. Plant Biol. 59, 417–441. doi: 10.1146/annurev.arplant.59.032607.092945
Pokhilko, A., Flis, A., Sulpice, R., Stitt, M., and Ebenhöh, O. (2014). Adjustment of carbon fluxes to light conditions regulates the daily turnover of starch in plants: a computational model. Mol. Biosyst. 10, 613. doi: 10.1039/c3mb70459a
Porto, D. D., Bruneau, M., Perini, P., Anzanello, R., Renou, J.-P., dos Santos, H. P., et al. (2015). Transcription profiling of the chilling requirement for bud break in apples: a putative role for FLC-like genes. J. Exp. Bot. 66, 2659–2672. doi: 10.1093/jxb/erv061
Price, J., Laxmi, A., St Martin, S. K., and Jang, J.-C. (2004). Global transcription profiling reveals multiple sugar signal transduction mechanisms in Arabidopsis. Plant Cell 16, 2128–2150. doi: 10.1105/tpc.104.022616
Rameau, C., Bertheloot, J., Leduc, N., Andrieu, B., Foucher, F., and Sakr, S. (2015). Multiple pathways regulate shoot branching. Front. Plant Sci. 5:741. doi: 10.3389/fpls.2014.00741
Reddy, S. K., Holalu, S. V., Casal, J. J., and Finlayson, S. A. (2013). Abscisic acid regulates axillary bud outgrowth responses to the ratio of red to far-red light. Plant Physiol. 163, 1047–1058. doi: 10.1104/pp.113.221895
Reed, J. W., Nagatani, A., Elich, T. D., Fagan, M., and Chory, J. (1994). Phytochrome A and Phytochrome B have overlapping but distinct functions in arabidopsis development. Plant Physiol. 104, 1139–1149. doi: 10.1104/pp.104.4.1139
Reid, M. S., and Wu, M.-J. (1992). Ethylene and flower senescence. Plant Growth Regul. 11, 37–43. doi: 10.1007/BF00024431
Rivero, R. M., Kojima, M., Gepstein, A., Sakakibara, H., Mittler, R., Gepstein, S., et al. (2007). Delayed leaf senescence induces extreme drought tolerance in a flowering plant. Proc. Natl. Acad. Sci. U.S.A. 104, 19631–19636. doi: 10.1073/pnas.0709453104
Rohde, A., and Bhalerao, R. P. (2007). Plant dormancy in the perennial context. Trends Plant Sci. 12, 217–223. doi: 10.1016/j.tplants.2007.03.012
Rolland, F., Baena-Gonzalez, E., and Sheen, J. (2006). Sugar sensing and signaling in plants:conserved and novel mechanisms. Annu. Rev. Plant Biol. 57, 675–709. doi: 10.1146/annurev.arplant.57.032905.105441
Roman, H., Girault, T., Barbier, F., Péron, T., Brouard, N., Pěnčík, A., et al. (2016). Cytokinins are initial targets of light in the control of bud outgrowth. Plant Physiol. 172, 489–509. doi: 10.1104/pp.16.00530
Rook, F., Corke, F., Card, R., Munz, G., Smith, C., and Bevan, M. W. (2001). Impaired sucrose-induction mutants reveal the modulation of sugar-induced starch biosynthetic gene expression by abscisic acid signalling. Plant J. 26, 421–433. doi: 10.1046/j.1365-313X.2001.2641043.x
Rose, T. L., Bonneau, L., Der, C., Marty-Mazars, D., and Marty, F. (2006). Starvation-induced expression of autophagy-related genes in Arabidopsis. Biol. Cell 98, 53–67. doi: 10.1042/BC20040516
Ruonala, R., Rinne, P. L. H., Baghour, M., Moritz, T., Tuominen, H., and Kangasjärvi, J. (2006). Transitions in the functioning of the shoot apical meristem in birch (Betula pendula) involve ethylene. Plant J. 46, 628–640. doi: 10.1111/j.1365-313X.2006.02722.x
Ruonala, R., Rinne, P. L. H., Kangasjärvi, J., and van der Schoot, C. (2008). CENL1 expression in the rib meristem affects stem elongation and the transition to dormancy in Populus. Plant Cell 20, 59–74. doi: 10.1105/tpc.107.056721
Ruttink, T., Arend, M., Morreel, K., Storme, V., Rombauts, S., Fromm, J., et al. (2007). A molecular timetable for apical bud formation and dormancy induction in poplar. Plant Cell 19, 2370–2390. doi: 10.1105/tpc.107.052811
Saeed, A. I., Sharov, V., White, J., Li, J., Liang, W., Bhagabati, N., et al. (2003). TM4: a free, open-source system for microarray data management and analysis. Biotechniques 34, 374–378.
Sasidharan, R., Chinnappa, C. C., Staal, M., Elzenga, J. T. M., Yokoyama, R., Nishitani, K., et al. (2010). Light quality-mediated petiole elongation in Arabidopsis during shade avoidance involves cell wall modification by Xyloglucan Endotransglucosylase/Hydrolases. Plant Physiol. 154, 978–990. doi: 10.1104/pp.110.162057
Satoh-Nagasawa, N., Nagasawa, N., Malcomber, S., Sakai, H., and Jackson, D. (2006). A trehalose metabolic enzyme controls inflorescence architecture in maize. Nature 441, 227–230. doi: 10.1038/nature04725
Schröder, F., Lisso, J., and Mussig, C. (2011). EXORDIUM-LIKE1 promotes growth during low carbon availability in Arabidopsis. Plant Physiol. 156, 1620–1630. doi: 10.1104/pp.111.177204
Schröder, F., Lisso, J., and Müssig, C. (2012). Expression pattern and putative function of EXL1 and homologous genes in Arabidopsis. Plant Signal. Behav. 7, 22–27. doi: 10.4161/psb.7.1.18369
Seo, M., Koiwai, H., Akaba, S., Komano, T., Oritani, T., Kamiya, Y., et al. (2000). Abscisic aldehyde oxidase in leaves of Arabidopsis thaliana. Plant J. 23, 481–488. doi: 10.1046/j.1365-313x.2000.00812.x
Shimizu, S., and Mori, H. (1998). Analysis of cycles of dormancy and growth in pea axillary buds based on mRNA accumulation patterns of cell cycle-related genes. Plant Cell Physiol. 39, 255–262. doi: 10.1093/oxfordjournals.pcp.a029365
Smith, A. M., and Stitt, M. (2007). Coordination of carbon supply and plant growth. Plant Cell Environ. 30, 1126–1149. doi: 10.1111/j.1365-3040.2007.01708.x
Song, L., Huang, S. S. C., Wise, A., Castanon, R., Nery, J. R., Chen, H., et al. (2016). A transcription factor hierarchy defines an environmental stress response network. Science 354, aag1550. doi: 10.1126/science.aag1550
Stafstrom, J. P., Ripley, B. D., Devitt, M. L., and Drake, B. (1998). Dormancy-associated gene expression in pea axillary buds. Planta 205, 547–552. doi: 10.1007/s004250050354
Stirnberg, P., van de Sande, K., and Leyser, H. M. O. (2002). MAX1 and MAX2 control shoot lateral branching in Arabidopsis. Development 129, 1131–1141.
Subramanian, A., Tamayo, P., Mootha, V. K., Mukherjee, S., Ebert, B. L., Gillette, M. A., et al. (2005). Gene set enrichment analysis: a knowledge-based approach for interpreting genome-wide expression profiles. Proc. Natl. Acad. Sci. U.S.A. 102, 15545–15550. doi: 10.1073/pnas.0506580102
Sulpice, R., Pyl, E.-T., Ishihara, H., Trenkamp, S., Steinfath, M., Witucka-Wall, H., et al. (2009). Starch as a major integrator in the regulation of plant growth. Proc. Natl. Acad. Sci. U.S.A. 106, 10348–10353. doi: 10.1073/pnas.0903478106
Suttle, J. C. (1998). Involvement of ethylene in potato microtuber dormancy. Plant Physiol. 118, 843–848. doi: 10.1104/pp.118.3.843
Tatematsu, K., Ward, S., Leyser, O., Kamiya, Y., and Nambara, E. (2005). Identification of cis-elements that regulate gene expression during initiation of axillary bud outgrowth in Arabidopsis. Plant Physiol. 138, 757–766. doi: 10.1104/pp.104.057984
Thimm, O., Bläsing, O., Gibon, Y., Nagel, A., Meyer, S., Krüger, P., et al. (2004). MAPMAN: a user-driven tool to display genomics data sets onto diagrams of metabolic pathways and other biological processes. Plant J. 37, 914–939. doi: 10.1111/j.1365-313X.2004.02016.x
Thompson, A. R., and Vierstra, R. D. (2005). Autophagic recycling: lessons from yeast help define the process in plants. Curr. Opin. Plant Biol. 8, 165–173. doi: 10.1016/j.pbi.2005.01.013
Turnbull, C. G. N., Raymond, M. A. A., Dodd, I. C., and Morris, S. E. (1997). Rapid increases in cytokinin concentration in lateral buds of chickpea (Cicer arietinum L.) during release of apical dominance. Planta 202, 271–276. doi: 10.1007/s004250050128
Ueno, S., Klopp, C., Leplé, J. C., Derory, J., Noirot, C., Léger, V., et al. (2013). Transcriptional profiling of bud dormancy induction and release in oak by next-generation sequencing. BMC Genomics 14:236. doi: 10.1186/1471-2164-14-236
Usadel, B., Bläsing, O. E., Gibon, Y., Retzlaff, K., Höhne, M., Günther, M., et al. (2008). Global transcript levels respond to small changes of the carbon status during progressive exhaustion of carbohydrates in Arabidopsis rosettes. Plant Physiol. 146, 1834–1861. doi: 10.1104/pp.107.115592
Weaver, L. M., Gan, S., Quirino, B., and Amasino, R. M. (1998). A comparison of the expression patterns of several senescence-associated genes in response to stress and hormone treatment. Plant Mol. Biol. 37, 455–469. doi: 10.1023/A:1005934428906
Wiese, A., Christ, M. M., Virnich, O., Schurr, U., and Walter, A. (2007). Spatio-temporal leaf growth patterns of Arabidopsis thaliana and evidence for sugar control of the diel leaf growth cycle. New Phytol. 174, 752–761. doi: 10.1111/j.1469-8137.2007.02053.x
Woo, H. R., Chung, K. M., Park, J. H., Oh, S. A., Ahn, T., Hong, S. H., et al. (2001). ORE9, an F-box protein that regulates leaf senescence in Arabidopsis. Plant Cell 13, 1779–1790. doi: 10.1105/tpc.13.8.1779
Wood, M., Rae, G. M., Wu, R.-M., Walton, E. F., Xue, B., Hellens, R. P., et al. (2013). Actinidia DRM1–an intrinsically disordered protein whose mRNA expression is inversely correlated with spring budbreak in kiwifruit. PLoS ONE 8:e57354. doi: 10.1371/journal.pone.0057354
Yadav, R. K., Tavakkoli, M., Xie, M., Girke, T., and Reddy, G. V. (2014). A high-resolution gene expression map of the Arabidopsis shoot meristem stem cell niche. Development 141, 2735–2744. doi: 10.1242/dev.106104
Yan, J., Niu, F., Liu, W.-Z., Zhang, H., Wang, B., Yang, B., et al. (2014). Arabidopsis CIPK14 positively regulates glucose response. Biochem. Biophys. Res. Commun. 450, 1679–1683. doi: 10.1016/j.bbrc.2014.07.064
Yanagisawa, S., Yoo, S.-D., and Sheen, J. (2003). Differential regulation of EIN3 stability by glucose and ethylene signalling in plants. Nature 425, 521–525. doi: 10.1038/nature01984
Yang, J., Worley, E., and Udvardi, M. (2014). A NAP-AAO3 regulatory module promotes chlorophyll degradation via ABA biosynthesis in Arabidopsis leaves. Plant Cell 26, 4862–4874. doi: 10.1105/tpc.114.133769
Yang, J. C., Zhang, J. H., Wang, Z. Q., Zhu, Q. S., and Liu, L. J. (2003). Involvement of abscisic acid and cytokinins in the senescence and remobilization of carbon reserves in wheat subjected to water stress during grain filling. Plant Cell Environ. 26, 1621–1631. doi: 10.1046/j.1365-3040.2003.01081.x
Yao, C., and Finlayson, S. A. (2015). Abscisic acid is a general negative regulator of Arabidopsis axillary bud growth. Plant Physiol. 169, 611–626. doi: 10.1104/pp.15.00682
Yuan, K., and Wysocka-Diller, J. (2006). Phytohormone signalling pathways interact with sugars during seed germination and seedling development. J. Exp. Bot. 57, 3359–3367. doi: 10.1093/jxb/erl096
Zacarias, L., and Reid, M. S. (1990). Role of growth regulators in the senescence of Arabidopsis thaliana leaves. Physiol. Plant. 80, 549–554. doi: 10.1111/j.1399-3054.1990.tb05677.x
Zeevaart, J. A. D., and Creelman, R. A. (1988). Metabolism and physiology of abscisic acid. Annu. Rev. Plant Physiol. Plant Mol. Biol. 39, 439–473. doi: 10.1146/annurev.pp.39.060188.002255
Zhong, C., Xu, H., Ye, S., Wang, S., Li, L., Zhang, S., et al. (2015). Gibberellic acid-stimulated Arabidopsis6 Serves as an integrator of gibberellin, abscisic acid, and glucose signaling during seed germination in Arabidopsis. Plant Physiol. 169, 2288–2303. doi: 10.1104/pp.15.00858
Keywords: bud dormancy, carbon starvation, gene regulatory networks, shoot architecture, plant evolution and development
Citation: Tarancón C, González-Grandío E, Oliveros JC, Nicolas M and Cubas P (2017) A Conserved Carbon Starvation Response Underlies Bud Dormancy in Woody and Herbaceous Species. Front. Plant Sci. 8:788. doi: 10.3389/fpls.2017.00788
Received: 26 February 2017; Accepted: 27 April 2017;
Published: 23 May 2017.
Edited by:
José M. Romero, University of Seville, SpainReviewed by:
Nayelli Marsch-Martinez, Centro de Investigación y de Estudios Avanzados del Instituto Politécnico Nacional, MexicoCopyright © 2017 Tarancón, González-Grandío, Oliveros, Nicolas and Cubas. This is an open-access article distributed under the terms of the Creative Commons Attribution License (CC BY). The use, distribution or reproduction in other forums is permitted, provided the original author(s) or licensor are credited and that the original publication in this journal is cited, in accordance with accepted academic practice. No use, distribution or reproduction is permitted which does not comply with these terms.
*Correspondence: Pilar Cubas, cGN1YmFzQGNuYi5jc2ljLmVz
†Present address: Eduardo González-Grandío, Plant Gene Expression Center, 800 Buchanan Street, Albany, CA, USA
Disclaimer: All claims expressed in this article are solely those of the authors and do not necessarily represent those of their affiliated organizations, or those of the publisher, the editors and the reviewers. Any product that may be evaluated in this article or claim that may be made by its manufacturer is not guaranteed or endorsed by the publisher.
Research integrity at Frontiers
Learn more about the work of our research integrity team to safeguard the quality of each article we publish.