- 1Plant-Microbe Interactions, Department of Biology, Faculty of Science, Utrecht University, Utrecht, Netherlands
- 2Plant Physiology, Swammerdam Institute for Life Sciences, University of Amsterdam, Amsterdam, Netherlands
Atmospheric CO2 influences plant growth and stomatal aperture. Effects of high or low CO2 levels on plant disease resistance are less well understood. Here, resistance of Arabidopsis thaliana against the foliar pathogen Pseudomonas syringae pv. tomato DC3000 (Pst) was investigated at three different CO2 levels: high (800 ppm), ambient (450 ppm), and low (150 ppm). Under all conditions tested, infection by Pst resulted in stomatal closure within 1 h after inoculation. However, subsequent stomatal reopening at 4 h, triggered by the virulence factor coronatine (COR), occurred only at ambient and high CO2, but not at low CO2. Moreover, infection by Pst was reduced at low CO2 to the same extent as infection by mutant Pst cor-. Under all CO2 conditions, the ABA mutants aba2-1 and abi1-1 were as resistant to Pst as wild-type plants under low CO2, which contained less ABA. Moreover, stomatal reopening mediated by COR was dependent on ABA. Our results suggest that reduced ABA levels at low CO2 contribute to the observed enhanced resistance to Pst by deregulation of virulence responses. This implies that enhanced ABA levels at increasing CO2 levels may have a role in weakening plant defense.
Introduction
The atmospheric CO2 level has been rising at an accelerating rate since the industrial revolution. According to the Coupled Climate-Carbon Cycle Model Intercomparison Project (C4MIP), atmospheric CO2 is predicted to reach levels varying between 730 and 1020 ppm at the end of 21st century. During recent years, various Free-Air CO2 Enrichment (FACE) studies were conducted to assess the long-term impact of elevated CO2 levels on plant performance. These studies showed that elevated CO2 levels typically result in enhanced plant growth, decreased transpiration, and higher water use efficiency (Coleman et al., 1993; Dermody et al., 2006; Reich et al., 2006; Jain et al., 2007; Leakey et al., 2009; Wang et al., 2012; Schmid et al., 2016). In contrast, studies using reduced CO2 levels revealed an association with decreased photosynthesis and reduced growth (Sage and Coleman, 2001; Temme et al., 2015). Generally, different plants respond similarly to changes in atmospheric CO2 levels, but also variable responses depending on the genotypic differences between plant species and species ecotypes have been reported (Murray, 1995; Li et al., 2006; Temme et al., 2015). For example, levels of the major metabolites fructose, galactose, and glucose decreased significantly under elevated CO2 conditions in Arabidopsis thaliana (Arabidopsis) ecotype Cvi-0, but not in the ecotypes Col-0 and Ws-0 (Li et al., 2006).
The impact of the atmospheric CO2 concentration on the level of plant disease resistance is highly variable (Chakraborty et al., 2000; Garrett et al., 2006; Kobayashi et al., 2006; Yáñez-López et al., 2014). High CO2 concentrations increase the canopy size and leaf humidity, resulting in a microclimate that is favorable for the development of many pathogenic microbes (Manning and Tiedemann, 1995). Nevertheless, at elevated CO2 the infection rate of the anthracnose Colletotrichum gloeosporioides on the pasture Stylosanthes scabra was significantly reduced (Chakraborty and Datta, 2003). Intriguingly, in a FACE study assessing the effects of elevated CO2 on soybean diseases, it was observed that high CO2 increased the susceptibility to brown spot Septoria glycines, whereas the susceptibility to downy mildew Peronospora manshurica was reduced (Eastburn et al., 2010). Moreover, high CO2-induced susceptibility of Arabidopsis to powdery mildew (Erysiphe cichoracearum) was reported to be dependent on the Arabidopsis ecotype (Lake and Wade, 2009). These results indicate that the effect of atmospheric CO2 on disease resistance is influenced by plant genotype, pathogen species, and environmental conditions.
Stomata serve as important passages for many foliar plant pathogenic microbes to access the plant (Melotto et al., 2008; Grimmer et al., 2012). Stomata also control the exchange of gases, such as water vapor and CO2, between the atmosphere and the leaves, hence their formation and aperture is influenced by atmospheric CO2. Elevated atmospheric CO2 levels generally lead to a decrease in stomata density and stomatal aperture (Israelsson et al., 2006). Atmospheric CO2 levels also influence the opening and closure of stomata. Several molecular players have been identified in this process, including the protein kinase HT1 (HIGH LEAF TEMPERATURE1), which is a key regulator of CO2-induced stomatal movement, and the MATE transporter RHC1 (RESISTANCE TO HIGH CO2 1), which represses HT1 (Hashimoto et al., 2006; Tian et al., 2015; Hashimoto-Sugimoto et al., 2016). In addition, carbonic anhydrases and bicarbonate have been identified as early regulators of CO2 signaling in Arabidopsis guard cells, as they enhance the physical interaction between RHC1 and HT1 (Hu et al., 2010; Xue et al., 2011; Tian et al., 2015). The latter process activates OST1 (OPEN STOMATA1) and SLAC1 (SLOW ANION CHANNEL1), resulting in an efflux of anions and subsequent closure of stomata (Xue et al., 2011; Tian et al., 2015), a process which is dependent on ABA signaling (Chater et al., 2015).
The effects of different CO2 conditions on stomata behavior potentially modify pathogen infection. At elevated CO2 levels, red maple leaves showed enhanced resistance to the fungus Phyllosticta minima, which was associated with reduced stomatal aperture (Mcelrone et al., 2005). Also in the tomato-Pseudomonas syringae pv. tomato DC3000 (Pst) interaction, a correlation between increased disease resistance and a reduction of stomatal aperture was observed under elevated CO2 conditions (Li et al., 2014). However, stomata-independent defenses controlled by plant hormones contributed to the observed enhanced resistance as well (Zhang et al., 2015). In addition, the decrease in stomatal aperture of Medicago truncatula by elevated CO2 was demonstrated to improve aphid feeding (Sun et al., 2015).
Control of stomatal aperture is a crucial aspect of the plant defense response to pathogens. Under ambient conditions, the stomata of Arabidopsis and tomato plants close actively within 1 to 2 h after infection with the bacterial pathogen Pst, which restricts entry of this pathogen into the leaf and, hence, limits colonization of the host tissue (Melotto et al., 2006). Nonetheless, a subsequent 2 to 3 h later, Pst suppresses the stomatal closure by producing the virulence factor coronatine (COR), which is a structural mimic of an isoleucine derivative of the plant hormone jasmonic acid (JA), and effectively induces stomata reopening (Melotto et al., 2006). Interestingly, many signaling components that are involved in Pst-induced stomatal responses, particularly the plant hormones abscisic acid (ABA), salicylic acid (SA) and JA, have also been implicated in CO2-induced stomatal responses (Melotto et al., 2008; Neill et al., 2008; Zeng et al., 2010; Montillet et al., 2013). This indicates that stomata act as a key checkpoint of plant defense under changing atmospheric CO2 conditions.
Plant hormones play pivotal roles in gene regulatory networks that control responses to biotic and abiotic stress conditions (Fujita et al., 2006). Besides SA and JA, which are two key players in plant immune signaling, other hormones such as ABA, ethylene, auxins, gibberellins and cytokinins have been implicated in defense signaling, often by modulating the SA–JA backbone of the hormone-regulated immune signaling network (Vlot et al., 2009; Robert-Seilaniantz et al., 2011; Pieterse et al., 2012). ABA can function negatively in the post-invasive defense phase through its antagonism of SA- and JA-controlled pathogen defenses (Ton et al., 2009; Pieterse et al., 2012). For example, tomato and Arabidopsis mutants that are defective in ABA signaling are less susceptible to SA-controlled hemi-biotrophic bacteria like Pst and JA/ethylene-controlled necrotrophic fungi like Botrytis cinerea (Audenaert et al., 2002; Thaler and Bostock, 2004; De Torres-Zabala et al., 2007; Liu et al., 2015). However, ABA can also function positively in plant immunity by co-regulating the pre-invasive defense phase that controls papillae formation at the site of infection and stomatal behavior (Melotto et al., 2008; Ton et al., 2009; Pieterse et al., 2012). For example, Melotto et al. (2006) found that the ABA-deficient mutant aba3-1 was defective in stomatal closure following infection with Pst, suggesting that ABA signaling is required for Pst-induced pre-invasive stomatal defense.
Elevated CO2 has been shown to influence plant hormone levels and signaling. Generally, SA signaling is enhanced and JA signaling is reduced (DeLucia et al., 2012), which was demonstrated in tomato to increase resistance to Pst and reduce resistance to B. cinerea (Zhang et al., 2015). Different results on the effects of elevated CO2 on ABA signaling in Arabidopsis have been reported, showing a reduction in ABA content (Teng et al., 2006), but also an increase in transcript abundance of ABA-responsive genes (Li et al., 2006). It has been demonstrated that ABA signaling interacts with CO2 signaling in guard cells (Leymarie et al., 1998; Israelsson et al., 2006; Kim and Maik, 2010; Hubbard et al., 2012; Merilo et al., 2013, 2015). Whether CO2 and ABA signaling converge in controlling defense responses is unknown.
Despite growing efforts on studying plant disease resistance under high atmospheric CO2, the exact signaling mechanisms underlying the effects of different CO2 levels on plant defense remain elusive. Moreover, up to now studies on the effects of low CO2 on plant immune responses are scarce. Inclusion of low CO2 experiments could reveal effects of the steep incline in CO2 levels that the world has faced since the industrial revolution (Sage and Coleman, 2001). Most plants are expected to still be adapted to lower levels of atmospheric CO2 than the current ambient level. Using Arabidopsis-Pst as a model, we set out to investigate whether and how atmospheric CO2 affects the disease resistance to this bacterial pathogen that gains access to the plant through stomatal openings. We observed that high CO2-grown Arabidopsis plants exhibited enhanced susceptibility to Pst, whereas plants grown under low CO2 conditions were more resistant. The ABA content in low CO2-grown plants was shown to be reduced upon Pst infection. The role of ABA in atmospheric CO2-modulated disease resistance was further investigated using ABA mutants. Both ABA mutants and low CO2-grown wild-type plants showed attenuation of COR-triggered stomatal reopening and displayed an enhanced resistance level to Pst. These data suggest that the historic rise of atmospheric CO2 may have caused enhanced disease susceptibility to certain pathogens due to the ABA-regulated suppression of plant immunity.
Materials and Methods
Plant Materials and Cultivation
Seeds of A. thaliana accessions Col-0 and Landsberg erecta (Ler-0), and mutants aba2-1 [Col-0] (Koornneef et al., 1982), abi1-2 [Col-0] (Gosti et al., 1999), and abi1-1 [Ler-0] (Koornneef et al., 1984) were sown on autoclaved river sand under ambient CO2 conditions (450 ppm). Two weeks later, seedlings were transferred to 60-ml pots containing a sand/potting soil mixture that was autoclaved twice for 20 min. Then, they were placed in high (800 ppm), ambient (450 ppm), or low (150 ppm) atmospheric CO2 conditions, where they remained for the rest of the experiment, except in the experiments shown in Figures 3D,E and Supplementary Figure S3, for which the seedlings continued growing for 2 more weeks under the ambient CO2 condition and were only after inoculation with the Pst bacteria or treatment with ABA placed under the different CO2 conditions for the remainder of the experiment. The technical specifications of the CO2-controlled growth chambers used in this study have been described in detail by Temme et al. (2015). Plants grew at a 10-h day at 20°C and 14-h night at 18°C cycle (350 μmol m-2 s-1) with 70% relative humidity. Plants were watered every other day and received half-strength Hoagland solution (Hoagland and Arnon, 1938) twice a week. Plants were treated when 4 weeks old in all experiments. For dry weight measurements, 10 rosettes per time point were put separately in a paper bag and dried for 3 days at 60°C.
Cultivation of Bacteria and Bioassays
Pst (Whalen et al., 1991) and Pst cor- (strain DB29 of Pst, which is a cmaA cfaA double mutant; Brooks et al., 2004) were grown on KB medium (King et al., 1954) supplemented with 50 μg ml-1 rifampicin. To prepare inoculum, bacteria were streaked from rifampicin-selective KB agar plates and subsequently cultured in liquid KB medium in a shaker at 220 rpm at 28°C for 24 h. Bacteria were collected by centrifugation for 10 min at 1,500 × g, and resuspended in 10 mM MgSO4. For dip inoculation, the bacterial inoculum was diluted to a final concentration of 5 × 107 cfu ml-1 of 10 mM MgSO4 containing 0.015% (v/v) Silwet L-77 (Van Meeuwen Chemicals, Weesp, Netherlands). For pressure infiltration, the bacterial suspension was adjusted to a concentration of 5 × 106 cfu ml-1 (disease assay) or 1 × 108 cfu ml-1 (ABA measurement). The abaxial side of leaves was pressure infiltrated with a needleless syringe.
To determine bacterial growth in planta, leaf disks of infected plants were harvested, weighed, surface sterilized in 70% ethanol for 8 s, and washed with water immediately after. Subsequently, 200 μl of 10 mM MgSO4 was added to the leaf disks after which they were ground thoroughly. Aliquots of 10 μl of different dilutions were plated onto KB plates containing 25 μg ml-1 rifampicin. After 48 h of incubation at room temperature, bacterial colonies were counted and growth of the bacteria was calculated after log-transformation of the cfu data. Eight biological replicates were included for each time point.
Stomata Measurement
Stomatal aperture and density were measured by a modified protocol of dental resin impressions (Geisler et al., 2000). Two components of Present Light Body (Coltène, Altstatten, Switzerland) were mixed thoroughly (v/v, 1:1) and the abaxial side of the leaves was softly pressed onto the dental resin immediately after harvesting. Leaves were removed 10 min later when the mixture had hardened. Transparent nail polish was applied to the dental resin molds to create casts, which were fixed on microscope slides with Anutex modeling wax (Kemdent, Purton, Swindon, Wiltshire, UK) for further observation.
Stomata were examined using an Olympus microscope and Analysis D Olympus Software on the pictures taken. Stomatal aperture was determined by measuring the width and length of the stomata. At least six leaves were harvested for each treatment and 20–30 observations were recorded from each leaf.
ABA Measurement and Treatment
For ABA quantification, 60–250 mg leaf material was harvested 24 h after treatments and ground to a fine powder using liquid nitrogen. ABA was extracted as described (Scala et al., 2013). Briefly, the samples were homogenized in 0.5 ml of 70% methanol using a Precellys24 tissue homogenizer (Bertin Technologies, Berlin) by shaking at 6,000 rpm for 40 s. Subsequently, the homogenates were centrifuged at 10,000 × g for 20 min at 4°C. The supernatants of two extraction steps were pooled together. ABA was quantified by liquid chromatography-mass spectrometry (LC-MS) analysis on a Varian 320 Triple Quad LC-MS/MS. Endogenous ABA levels were quantified by comparing the integrated surface area from each sample with its corresponding internal standard.
To measure stomatal responsiveness to exogenously applied ABA, leaves were dipped in a solution of 15 μM ABA in 0.015% (v/v) Silwet L-77.
Results
Effect of High and Low Atmospheric CO2 Levels on Arabidopsis Growth and Stomatal Behavior
Numerous studies have been conducted to assess the effect of high CO2 levels on plant performance, including plant growth, stomatal behavior, and disease resistance. However, only limited information is available on the effects of low CO2 levels on the plant. Here, we studied the effects of three different CO2 levels on Arabidopsis plants in the absence and presence of pathogens: high (800 ppm), ambient (450 ppm), and low (150 ppm) levels of CO2. Plants were cultivated under ambient CO2 until they were 2 weeks old, after which they were placed under the three respective CO2 conditions. We noticed that plants that had grown under the low CO2 condition for an additional 2 weeks had smaller rosette sizes compared to plants grown under high and ambient CO2 conditions (Figure 1A). Also the dry weight of the rosettes was significantly lower in the low CO2 condition (Figure 1B). In contrast, there were no effects on rosette growth under high CO2 conditions, which was rather unexpected since most previous studies have reported an increase in biomass (Bowes, 1991; Leakey et al., 2009). However, our experimental conditions may not have been optimal for stimulated growth by elevated CO2 (Temme et al., 2015) and moreover, the Col-0 accession that we used may respond differently to high CO2 than other plant species (Li et al., 2006; Leakey et al., 2009; Temme et al., 2015).
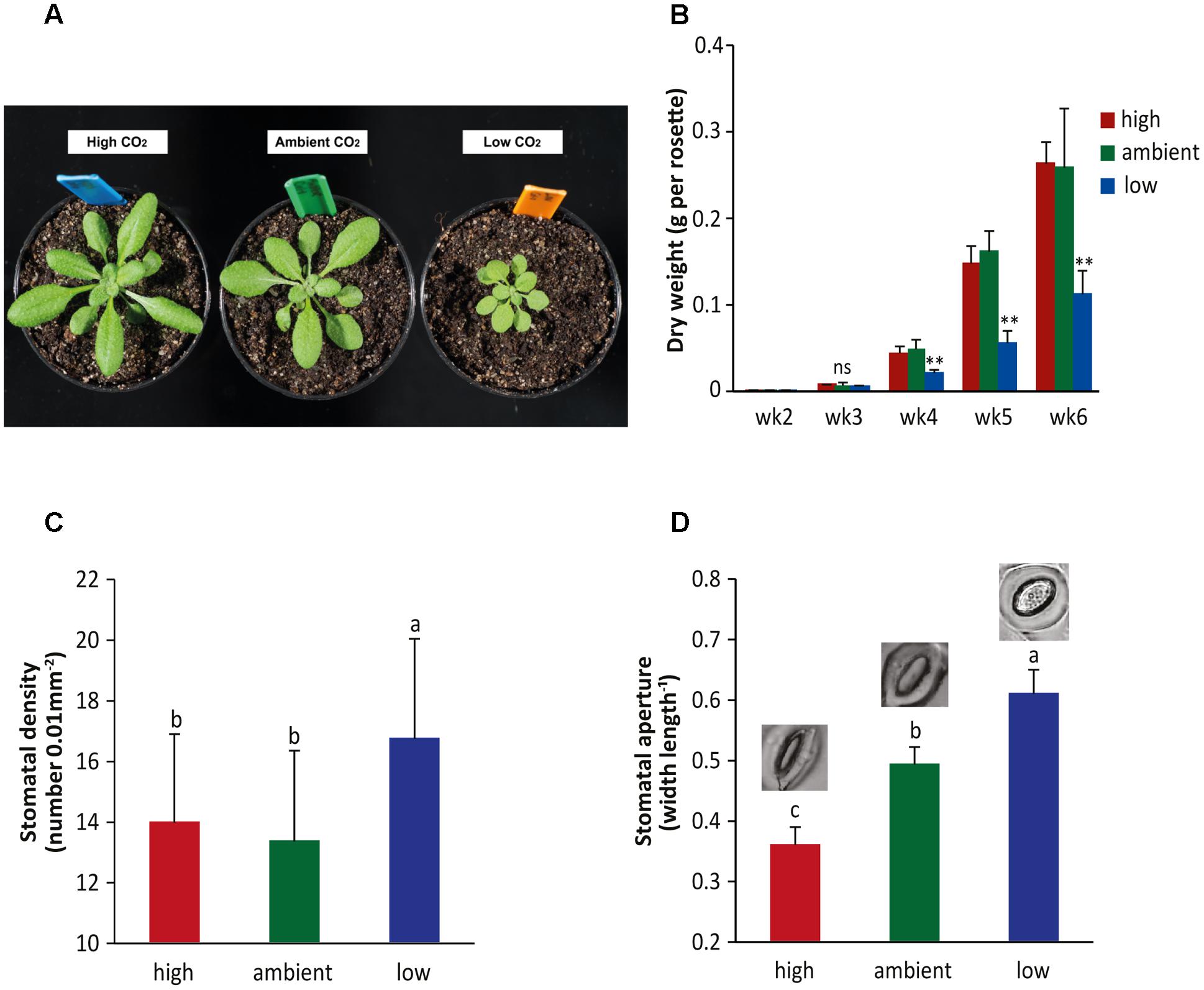
FIGURE 1. Effect of different atmospheric CO2 levels on growth and stomatal behavior of Arabidopsis. (A) Pictures of 4-week-old Arabidopsis plants grown under high (800 ppm), ambient (450 ppm), or low CO2 (150 ppm) conditions. (B) Dry weight of Arabidopsis rosettes at different developmental stages (from week 2 to week 6) under three different CO2 conditions. Asterisks indicate statistically significant differences between the CO2 treatments at the specific time points (one-way ANOVA, Duncan’s multiple range test, ∗∗P < 0.01; ns, no significant difference). Error bars represent SD, n = 10 plants. (C) Stomatal density and (D) stomatal aperture in 4-week-old Arabidopsis plants grown under three different CO2 conditions. Depicted are the averages of stomatal density and aperture (±SD) of six leaves. In (D) examplar pictures of stomatal aperture typical for the CO2 conditions are depicted. Different letters indicate statistically significant differences between the CO2 treatments (one-way ANOVA, Duncan’s multiple range test, P < 0.05). The figures are representative of at least two independent experiments.
Stomatal density and aperture were investigated under the three CO2 conditions as well. At high atmospheric CO2, stomatal density was not influenced, but a significant decrease in stomatal aperture was found (Figures 1C,D). At low atmospheric CO2, an increase in both stomatal density and stomatal aperture was detected. These results are in line with previous studies that found that the inverse relationship between atmospheric CO2 and stomatal behavior was more evident under sub-ambient CO2 conditions than under elevated CO2 conditions (Royer, 2001), which is a phenomenon that is referred to as the CO2 ‘ceiling’ phenomenon. The major effects on plant growth and stomatal behavior observed at especially the low CO2 level prompted us to introduce a pathogen into the system that naturally enters through stomata in order to study the effects of CO2 on plant immunity.
Low Atmospheric CO2 Inhibits COR-Triggered Stomatal Reopening
To explore whether the differential stomatal behavior at the three tested atmospheric CO2 levels affects stomatal defense responses, we examined the stomatal responsiveness of Arabidopsis plants to infection by the bacterial leaf pathogen Pst. Previously, it was shown that at ambient CO2 the stomata close within 1 to 2 h after dip inoculation with Pst, and reopen again at 3 to 4 h due to the action of the virulence factor COR (Melotto et al., 2006). Our results under ambient conditions are in line with this finding, since we found that the stomata closed within 1 h after dip inoculation with wild-type Pst or the COR-deficient mutant Pst cor- (Figure 2). Subsequently, at 4 h after inoculation, the stomatal aperture was significantly greater in leaves infected by the wild-type Pst strain than by the Pst cor- mutant strain. It should be noted that for proper determination of the COR effect one should compare the mutant infection with the wild-type infection, and not with the mock treatment at 4 hpi. The circadian rhythm influences the stomatal aperture (closing in the afternoon), which may explain the somewhat more closed stomata in the mock situation at 4 hpi. Therefore, in comparison to mock, stomata of Pst cor--infected leaves were no longer statistically significantly more closed at 4 hpi and moreover, the stomata of wild-type Pst-challenged leaves were not statistically significantly more open, although a trend, likely due to COR action, was visible.
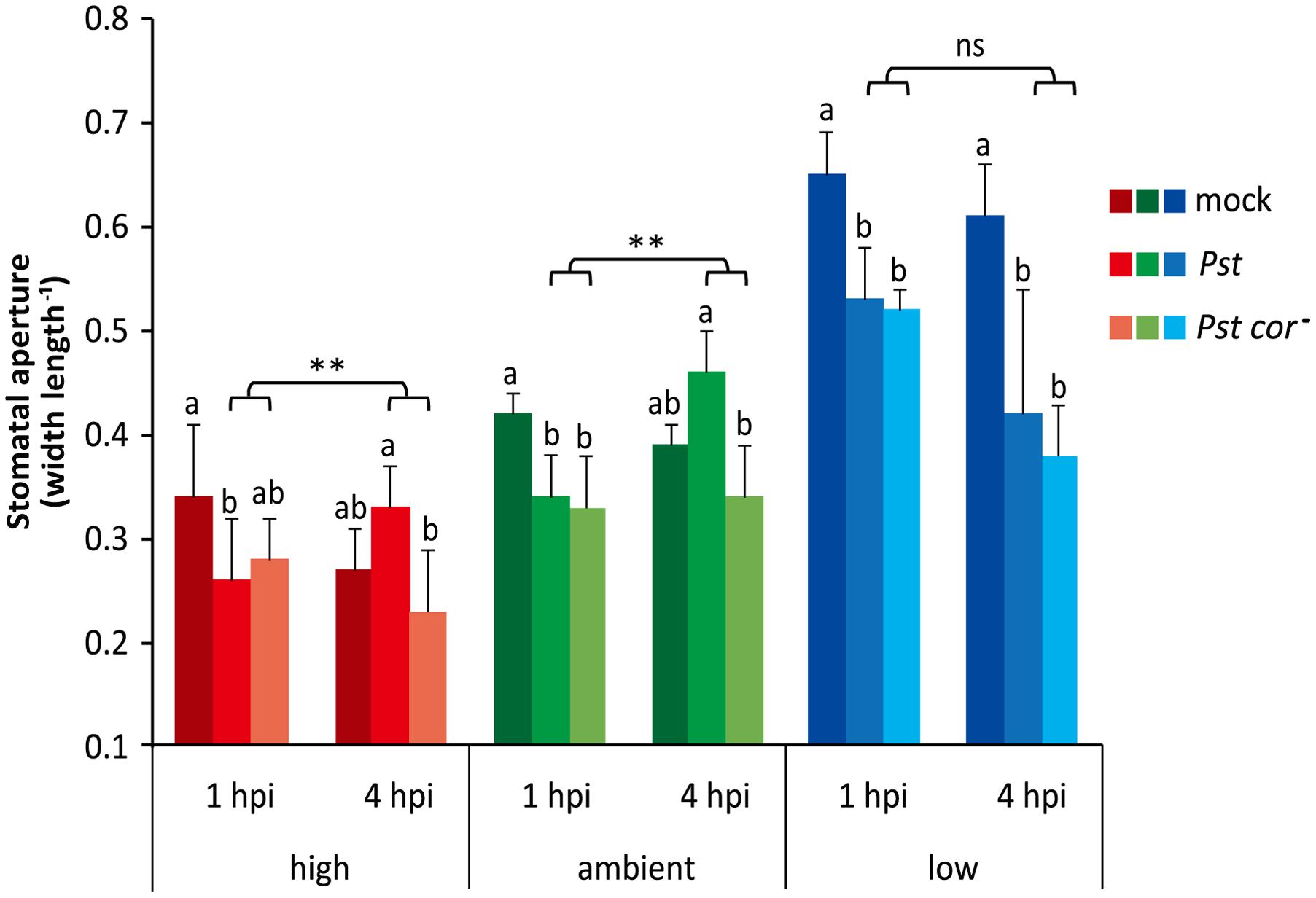
FIGURE 2. Effect of different atmospheric CO2 levels on stomatal aperture upon infection by Pst or Pst cor-. Arabidopsis leaves of 4-week-old plants grown under three different CO2 conditions were dip inoculated with a mock solution, Pst or Pst cor-. Stomatal aperture was determined 1 and 4 h after dip inoculation (hpi, hours post inoculation). Depicted are the averages of stomatal aperture (±SD) of six leaves. Different letters indicate statistically significant differences between the treatments at specific time points within the same atmospheric CO2 level (two-way ANOVA, Fisher’s LSD test, P < 0.05). Indications above the brackets specify the interaction (bacterium genotype × time) between the two Pst genotype treatments (wild type and mutant) and the time (1 and 4 hpi) under the same atmospheric CO2 condition (∗∗P < 0.01; ns, not significant). This figure is representative of three independent experiments.
High CO2-grown plants contained stomata that were generally more closed (Figures 1D, 2). Still, the stomata initially closed further when the leaves were attacked by Pst and near significant closure was also induced by Pst cor- infection. Similar to the ambient condition, under high CO2 the stomata were subsequently reopened by the wild type in comparison to the mutant bacteria. This suggests that stomatal responsiveness to PAMP-triggered closure and COR-triggered opening is intact at the high CO2 level. Under the low CO2 condition, stomata were opened more widely (Figures 1D, 2), but still they closed within 1 h after inoculation with Pst wild type or Pst cor- mutant, which is comparable to the ambient and high CO2 conditions. In contrast, at 4 h after inoculation, the stomata of both the Pst- and the Pst cor--challenged leaves remained closed under the low CO2 condition. These data show that plants grown under high and low CO2 conditions initially respond to Pst infection by closing their stomata, despite their original differences in stomatal aperture. However, the subsequent COR-mediated stomatal reopening occurs only under high and ambient CO2 conditions, whereas it is blocked under the low CO2 condition.
Atmospheric CO2 Alters Resistance to Pst in a COR-Dependent Manner
The resistance of Arabidopsis plants to Pst infection under the different atmospheric CO2 conditions was tested by determining the growth of Pst in plants cultivated at different CO2 levels. Initially, at 4 h after dip inoculation, plants grown at high CO2 levels contained significantly less Pst than plants grown at low CO2 (Figure 3A), which coincided with the lower stomatal density and aperture in leaves of high CO2-grown plants, thereby allowing fewer bacteria to enter the leaves (Figures 1C,D, 2). However, at 4 days after inoculation, the Pst bacterial titer in high CO2-grown plants was significantly higher compared to that in ambient and low CO2-grown plants (Figure 3A). In this particular experiment, the bacterial titer in low CO2-grown plants showed a trend of reduced amounts compared to ambient-grown plants; in other experiments the difference between the two treatments was often found to be statistically significant (Figures 3D,E, 5B and Supplementary Figure S2). Moreover, less-severe chlorotic disease symptoms on plants grown at low CO2 compared to plants grown at ambient and high CO2 were detected (Figure 3B). Given the different plant growth rates when cultivated at different CO2 levels, it is possible that differences in the weight per leaf area caused the detected differences in Pst titer when depicted per leaf area. To rule out this possibility, the bacterial titer was also determined per gram of leaf tissue. The same trend, namely enhanced multiplication of Pst under high, and reduced amplification under low CO2 conditions was found (Figure 3C).
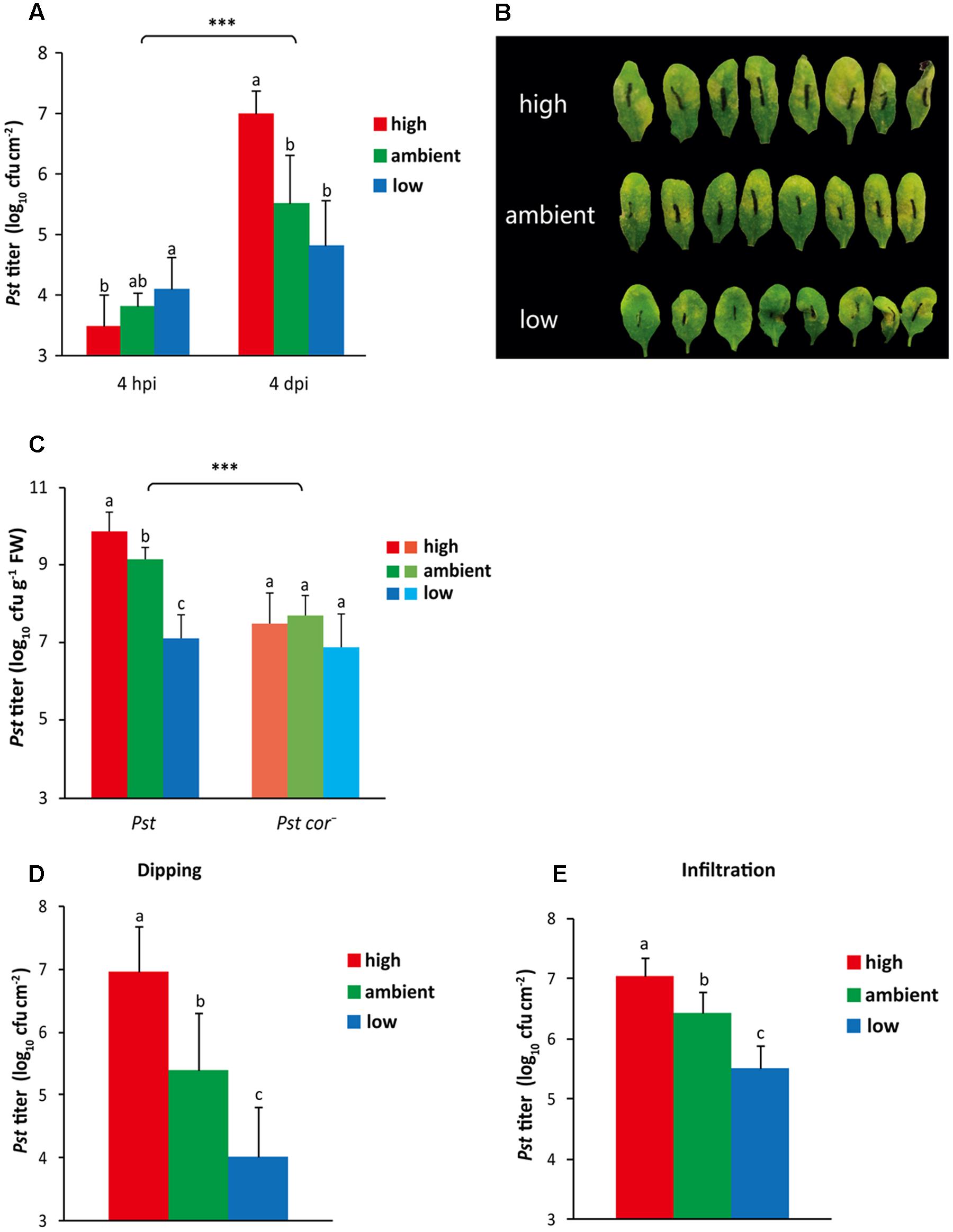
FIGURE 3. Effect of different atmospheric CO2 levels on resistance of Arabidopsis to Pst. (A) Growth of Pst in planta at 4 h and 4 days after dip inoculation of plants grown under three different CO2 conditions. Depicted are the averages of log10-transformed bacterial titer (±SD; per leaf area) from eight biological replicates. Different letters indicate statistically significant differences between the CO2 treatments at the indicated time point (two-way ANOVA, Fisher’s LSD test, P < 0.05). Indications above the brackets specify the interaction (CO2 condition × time) between the three CO2 conditions and the time (4 hpi and 4 dpi) (∗∗∗P < 0.001). (B) Pictures of the disease symptoms of plants grown under three different CO2 conditions at 4 days after dip inoculation with Pst. (C) Growth of Pst or Pst cor- in planta at 4 days after dip inoculation of plants grown under three different CO2 conditions. Indicated are the averages of log10-transformed bacterial titer (±SD; per g of leaf fresh weight) from eight biological replicates. Different letters indicate statistically significant differences between the CO2 treatments within the same bacterial treatment (two-way ANOVA, Fisher’s LSD test, P < 0.05). Indications above the brackets specify the interaction (CO2 condition × bacterium genotype) between the three CO2 conditions and the two Pst genotype treatments (wild type and mutant) (∗∗∗P < 0.001). (D,E) Growth of Pst in planta 4 days after inoculation of plants that had been cultivated under the ambient CO2 condition until inoculation by (D) dipping (5 × 107 cfu ml-1) or (E) pressure infiltration (6 × 105 cfu ml-1), after which plants were transferred to either high, ambient, or low CO2 conditions. Depicted are the averages of log10-transformed bacterial titer (±SD; per g of leaf fresh weight) from eight biological replicates. Different letters indicate statistically significant differences between the CO2 treatments (Fisher’s LSD test, P < 0.05). These figures are representative of at least two independent experiments.
The role of COR in successful infection by Pst through facilitation of stomatal reopening, but also by suppression of SA-mediated defense signaling and disease symptom development, has been well established (Mittal and Davis, 1995; Brooks et al., 2005). The in planta growth of the Pst cor- mutant strain was on average, over all three tested atmospheric CO2 conditions, significantly lower than that of the Pst wild-type strain, as was reported previously (Figure 3C) (Melotto et al., 2006). However, while the bacterial titer of wild-type Pst was significantly higher in high CO2-grown plants and lower in low CO2-grown plants, growth of the mutant Pst cor- was severely limited under all three CO2 conditions, reaching the same low bacterial titer as that of wild-type Pst in low CO2-grown plants (Figure 3C). The statistically significant interaction of atmospheric CO2 with the in planta growth difference between Pst and Pst cor- suggests that atmospheric CO2 regulates the plant’s sensitivity to COR, leading to enhanced responsiveness at high CO2 and impaired responsiveness at low CO2. This differential responsiveness to COR could play a role in the observed differences in resistance levels to Pst under the three tested CO2 conditions.
Whether the inhibition of COR-mediated stomatal reopening under the low CO2 condition could explain the observed high level of resistance to Pst was tested by assaying bacterial growth after pressure infiltration of the Pst bacteria into the leaves. However, due to the small leaf size of low CO2-cultivated plants, a different experimental set-up had to be employed in which all plants were grown at ambient CO2 until they were inoculated with Pst (when the plants were 4 weeks old), after which they were placed under either the high, ambient, or low CO2 condition for the remainder of the experiment. First, we tested in this set-up the effect of different CO2 levels on Pst growth after dip inoculation and found that the bacterial growth was affected similarly to the original set-up in which plants had experienced the different CO2 conditions already 2 weeks preceding the dip inoculation (Figures 3A,D). Also upon pressure infiltration of Pst into the leaves, the effects of CO2 on bacterial growth were very much alike: high CO2 caused enhanced susceptibility whereas low CO2 caused reduced susceptibility (Figure 3E). This suggests that under the low CO2 condition not only COR-mediated stomatal reopening is affected, but also the suppression of post-invasive defense responses is reduced. On the other hand, under the high CO2 condition post-invasive defense appears stronger downregulated.
A Role for ABA Signaling in COR-Mediated Stomatal Reopening
To gain more insight into how the differential responsiveness to COR observed under different atmospheric CO2 levels may alter plant immunity, we assessed the role of the hormone ABA, a known regulator of stomatal aperture, in Pst-triggered stomatal closure and subsequent reopening (Melotto et al., 2006). First, we studied the effect of ABA on stomatal behavior under our ambient CO2 condition. It has previously been shown that in non-induced situations the stomata of the Arabidopsis ABA-deficient mutant aba2-1 and the ABA-insensitive mutant abi1-1 can be more open than that of wild-type plants, but this effect is not always evident (Figures 4, 5A, and Supplementary Figure S1) (Merlot et al., 2002; Melotto et al., 2006), which may be related to difference in stomatal aperture of wild-type plants at different times of the day on which the experiments were executed (Somers et al., 1998; Dodd et al., 2004). Nevertheless, in all experiments challenge with Pst triggered initially stomatal closure in wild type as well as aba2-1 and abi1-1 plants within 1 h after dip inoculation (Figure 4 and Supplementary Figure S1a). Moreover, while stomata subsequently reopened in a COR-dependent manner in wild-type plants as detected at 4 h after inoculation, stomata did not reopen in the aba2-1 and abi1-1 mutants when treated with either Pst or Pst cor- (Figure 4 and Supplementary Figure S1a). These findings are in contrast with those described by Melotto et al. (2006) who showed that neither the ABA-deficient mutant aba3-1, nor the ABA-insensitive mutant ost1-2, responded with stomatal closure to infection by Pst or treatment with flg22, the active epitope of bacterial flagellin. However, in accordance with our results, Montillet et al. (2013) reported that mutants aba2-1 and ost1-2 did close their stomata upon flg22 treatment. These results suggest the existence of an ABA-independent pathway in guard cells involving pathogen-induced stomatal closure. Furthermore, our data reveal a role for ABA signaling in COR-mediated stomatal reopening.
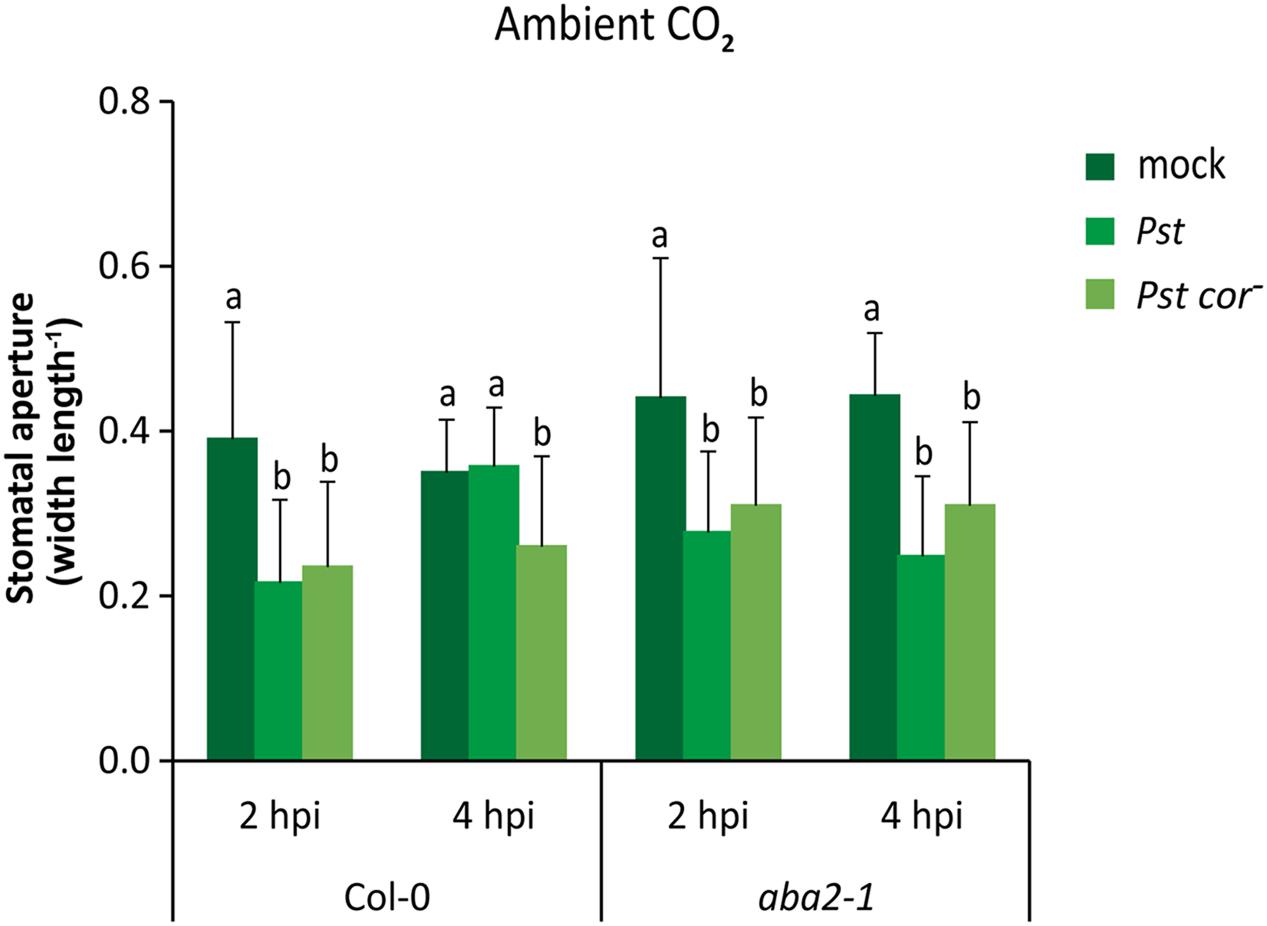
FIGURE 4. Effect of ABA signaling on stomatal aperture in response to Pst and Pst cor- under ambient CO2 conditions. Stomatal aperture in wild-type Col-0 and the ABA deficient mutant aba2-1 at 2 and 4 h after dip inoculation with Pst or Pst cor-. Indicated are the averages of the stomatal aperture (±SD) of six leaves. Different letters indicate statistically significant differences between treatments within one plant genotype at the indicated time point (two-way ANOVA, Fisher’s LSD test, P < 0.05). The interaction (bacterium genotype × time) between the two Pst genotype treatments (wild type and mutant) and the time (1 and 4 hpi) in the same plant genotype was 0.26 for wild-type Col-0 and 0.95 for aba2-1. This figure is representative of two independent experiments.
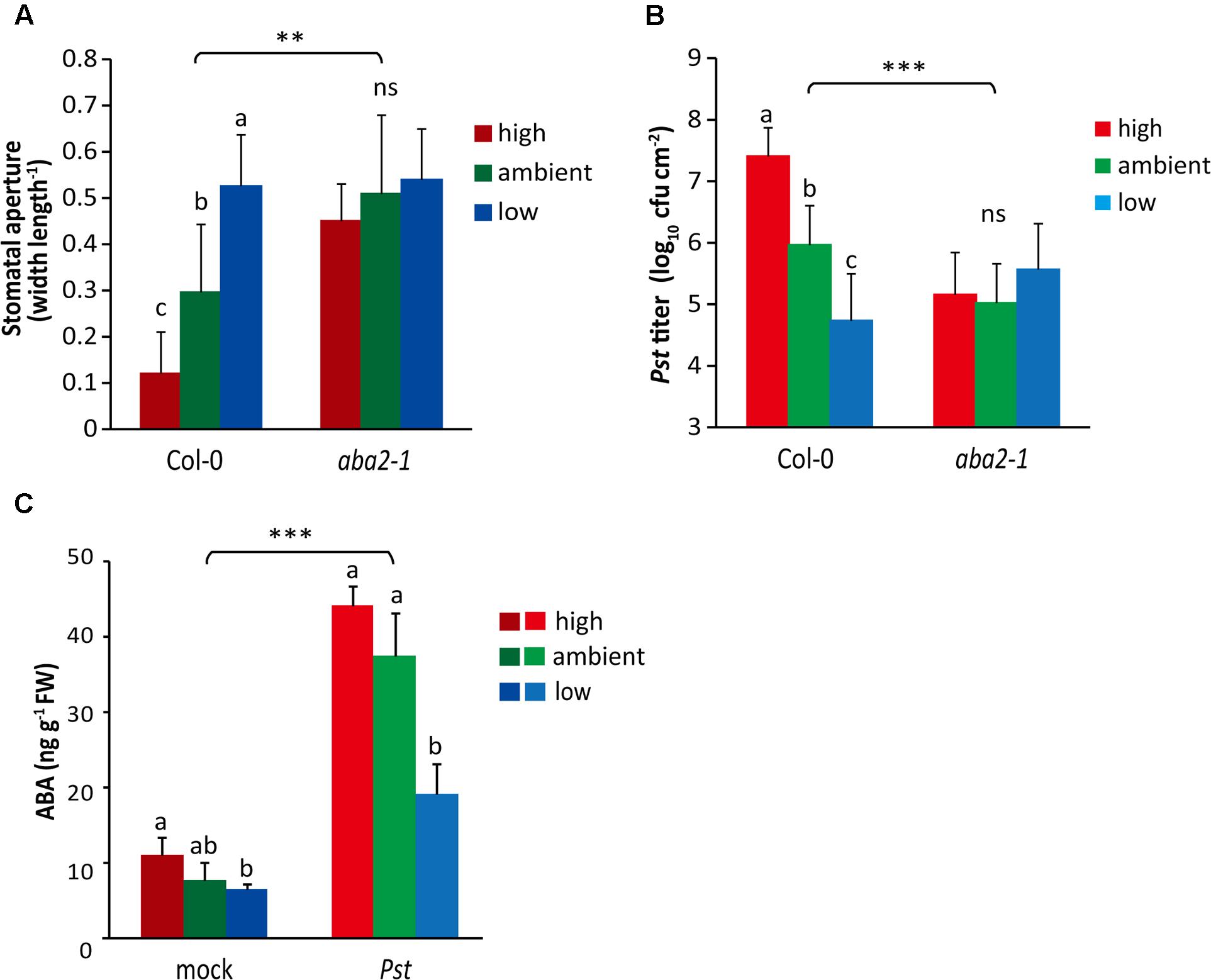
FIGURE 5. The effect of ABA signaling on atmospheric CO2-altered stomatal aperture and resistance to Pst. (A) Stomatal aperture of Arabidopsis wild-type Col-0 and the ABA deficient mutant aba2-1 grown under different atmospheric CO2 conditions. Depicted are the averages of the stomatal aperture (±SD) of six leaves. Different letters indicate statistically significant differences between the CO2 treatments within the same genotype (two-way ANOVA, Fisher’s LSD test, P < 0.05; ns, not significant). Indications above the brackets specify the interaction (CO2 condition × Arabidopsis genotype) between the three CO2 conditions and the two Arabidopsis genotype (wild-type Col-0 and mutant aba2-1) (∗∗P < 0.01). This experiment has not been repeated. (B) Growth of Pst in wild-type Col-0 and the mutant aba2-1 at 4 days after dip inoculation. Indicated are the averages of the log10-transformed bacterial titer (±SD; per leaf area) from eight biological replicates. Different letters indicate a statistically significant difference between the CO2 treatments within the same genotype (two-way ANOVA, Fisher’s LSD test, P < 0.05; ns, not significant). Indications above the brackets specify the interaction (CO2 condition × Arabidopsis genotype) between the three CO2 conditions and the two Arabidopsis genotypes (wild-type Col-0 and mutant aba2-1) (∗∗∗P < 0.001). (C) Levels of ABA in leaves of wild-type Col-0 plants grown under three different atmospheric CO2 conditions. Leaves of 4-week-old plants were pressure infiltrated with Pst (1 × 108 cfu ml-1) or mock (10 mM MgSO4) solution and after 24 h assayed for ABA content. Indicated are the averages of ABA levels (±SD) from five biological replicates. Different letters indicate a statistically significant difference in ABA levels between the CO2 conditions within the same treatment (two-way ANOVA, Fisher’s LSD test, P < 0.05). Indications above the brackets specify the interaction (CO2 condition × bacterium treatment) between the three CO2 conditions and the treatments (Pst and mock) (∗∗∗P < 0.0001). The part labels (B) and (C) are representative of two independent experiments.
ABA-Dependency of Atmospheric CO2-Controlled Stomatal Aperture and Disease Resistance against Pst
Based on our finding that under ambient CO2 conditions ABA mutants show a stomatal response pattern to Pst infection that is similar to that of wild-type Arabidopsis plants grown under low CO2 conditions, we hypothesized that there could be a role for ABA signaling in atmospheric CO2-altered disease resistance to Pst. To test this, we first measured stomatal aperture of the mutant aba2-1 when cultivated at different levels of atmospheric CO2 without Pst infection. As expected, stomata of wild-type Col-0 plants were more closed at high CO2 and more opened at low CO2 (Figure 5A). However, stomata of the aba2-1 mutant did not change their aperture under different CO2 conditions. In fact, their stomata were relatively open under all three CO2 conditions, to the same high level as that of low CO2-grown wild-type plants (Figure 5A). This statistical interaction between ABA and CO2 levels suggests that ABA can be involved in stomatal responsiveness to different atmospheric CO2 conditions.
Subsequently, we tested whether atmospheric CO2 can alter disease resistance to Pst in ABA mutants. Under ambient and high CO2 conditions, both aba2-1 and abi1-1 exhibited reduced in planta growth of Pst compared with wild-type plants (Figure 5B and Supplementary Figure S2), supporting a negative role for ABA signaling in the defense response against Pst, as shown previously (Melotto et al., 2006; De Torres-Zabala et al., 2007). More importantly, under all three CO2 conditions Pst growth in both ABA mutants was as low as in the wild-type plants grown at low CO2 (Figure 5B and Supplementary Figure S2). Together, these results suggest that ABA signaling plays an important role in atmospheric CO2-regulated plant defense responses against Pst.
Previously, ABA has been reported to accumulate upon Pst infection (De Torres-Zabala et al., 2007). Moreover, enrichment in atmospheric CO2 can also change ABA levels or ABA signaling, although variable effects in Arabidopsis have been described (Li et al., 2006; Teng et al., 2006). We assayed the ABA content in leaves infected with Pst under different atmospheric CO2 conditions. In the absence of Pst, ABA accumulation under the low CO2 condition was significantly reduced compared to that under the high CO2 condition, but no statistically significant differences with the ambient CO2 condition were detected (Figure 5C). In Pst-challenged leaves the ABA concentrations rose significantly compared with mock-treated leaves under all three CO2 conditions. However, in the low CO2-grown plants the ABA levels were significantly lower than those in the ambient and high CO2-grown plants upon infection by Pst. These results suggest that reduced ABA levels in low CO2-grown plants may be responsible for enhanced resistance to Pst.
Discussion
As one of the major characteristics of global climate change, the continuously rising atmospheric CO2 concentration has received extensive attention during the past decades. Here, we investigated the interplay between atmospheric CO2 and Arabidopsis defense mechanisms to infection by Pst. Plants are likely still evolutionary adapted to pre-industrial CO2 levels that are lower than the current global CO2 concentration. Therefore, by comparing three conditions, namely low (150 ppm), ambient (450 ppm), and high (800 ppm) CO2 levels, we compared the effect of the historic and future incline in CO2 level. Up to now studies on low CO2 effects on plant performance and plant disease resistance have been scarce (Tissue and Lewis, 2012). We show that effects of atmospheric CO2 on ABA signaling may account for the observed differential stomatal responsiveness as part of the altered resistance level to pathogen infection, and that post-invasive defenses are modulated by atmospheric CO2 as well.
Plant and Stomata Performance under Low and High CO2 Conditions
Previous studies, focusing mainly on the effects of elevated atmospheric CO2, showed that growth of various plant species was promoted by high CO2 levels and inhibited by low CO2 levels (Leakey et al., 2009; Eastburn et al., 2010; Temme et al., 2015). In our study, we show that the low CO2 condition significantly reduced growth of Arabidopsis and caused the stomata to be opened more widely than under ambient CO2 (Figure 1). Reduced ABA content and sensitivity in low CO2-grown plants (Figure 5C and Supplementary Figure S3) may be causal for the enhanced stomatal opening phenotype, and although a reduced plant stature has been reported for ABA mutants as well (Chatfield et al., 2000; LeNoble et al., 2004), the growth reduction at low CO2 is more likely caused by reduced photosynthesis. We found that Arabidopsis grown at high CO2 displayed a reduced opening of their stomata, as has been reported previously (Araújo et al., 2011). However, we found no reduction in stomatal density, nor an increase in rosette dry weight under the high CO2 condition. No or small effects of high CO2 on growth enhancement has previously been reported (Li et al., 2006; Temme et al., 2015). In our experimental set-up light intensity and nutrient constraints may have limited the stimulating effect of high CO2 on plant growth. Our findings on stomatal density are in line with the previously described CO2 ‘ceiling’ phenomenon, which refers to reaching a maximum stomatal density at a CO2 level of 400 ppm and that stomata respond more strongly to sub-ambient than to elevated CO2 concentrations (Kürschner et al., 1997; Royer, 2001).
Pst-Induced Stomatal Closure Can Be Independent of ABA and Occurs Independently of the CO2 Condition
Besides CO2, ABA determines the stomatal aperture. Here, we provide evidence for a role of ABA signaling in the regulation of stomatal aperture by different CO2 levels in un-infected plants. Unlike the wild type, the stomatal aperture of the ABA biosynthesis mutant aba2-1 was not influenced by the CO2 conditions (Figure 5A). Moreover, the ABA signaling mutant abi1-1 was unresponsive to high CO2-induced stomatal closure, albeit sensitivity regarding low CO2-induced opening was observed (Supplementary Figure S1). In general, our data corroborate previous findings on the interrelationship of ABA with elevated CO2-regulated signaling in guard cells (Leymarie et al., 1999; Nishimura et al., 2010; Xue et al., 2011; McLachlan et al., 2014).
Activation of stomatal closure has been demonstrated to be an essential pre-invasive defense response to foliar pathogens in various plant species (Melotto et al., 2006; Lee et al., 2013; Li et al., 2013; Du et al., 2014). The ABA-deficient mutant aba3-1 was previously shown to be compromised in its ability to close its stomata in response to Pst infection, suggesting a requirement for ABA biosynthesis in Pst-induced stomatal closure (Melotto et al., 2006). However, in our experiments, at all CO2 levels tested, both wild-type plants and the ABA mutants aba2-1 and abi1-1 responded to Pst infection with closure of their stomata (Figure 4 and Supplementary Figure S1). This indicates that the Pst-induced stomatal closure occurs at least partly independently of ABA and that atmospheric CO2 does not influence this mechanism. This is in line with a recent finding that an ABA-independent oxylipin pathway is responsible for flg22- and Pst-induced stomatal closure (Montillet et al., 2013). Moreover, a genetic screen of Arabidopsis mutants that are impaired in Pst-induced stomatal closure generated multiple mutants that still exhibited ABA-induced stomatal closure (Zeng et al., 2011). In addition, Lim et al. (2014) demonstrated that ABA hyposensitive PP2CA1 overexpressors closed their stomata in response to Pst infection or flg22 treatment. Taken together, these results support the notion that besides ABA signaling, additional mechanisms that are independent of ABA and independent of atmospheric CO2 play a role in the stomatal closure response upon Pst infection.
COR-Induced Stomatal Reopening Is Blocked at Low CO2 and Depends on ABA
The phytotoxin COR that is produced by Pst induces stomatal opening to stimulate infection. We found that under both ambient and high CO2 conditions, initial Pst-induced stomatal closure was followed by COR-dependent stomatal reopening (Figure 2). Interestingly, while stomata in low CO2-grown plants still responded to Pst with closing within 1 hpi, they did not reopen at 4 hpi, resulting in a stomatal aperture very similar to that of Pst cor--infected plants (Figure 2). Thus, if COR production by Pst is not affected by the low CO2 level, sensitivity to COR in terms of stomata reopening seems compromised under the low CO2 condition.
Previous reports demonstrated that under ambient CO2 conditions, COR and ABA signaling can influence each other’s activity either negatively or positively. For instance, ABA-induced stomatal closure is inhibited by COR (Melotto et al., 2006; Zheng et al., 2012), while both COR and ABA repress SA-regulated defense signaling (Brooks et al., 2005; De Torres-Zabala et al., 2007). Also, ABA and COR both activate gene expression of three NAC transcription factors that suppress Pst-induced SA biosynthesis and stomatal closure (Zheng et al., 2012). Here, we show that the mutants aba2-1 and abi1-1 closed their stomata upon inoculation with Pst and were unable to reopen them in response to COR production by Pst at 4 hpi under all three CO2 conditions tested (Figure 4 and Supplementary Figure S1). This pointed to an unexpected role for ABA signaling in COR-mediated stomatal reopening, which is independent of CO2 levels. As a follow-up experiment, expression of the COR-inducible, ABA-dependent NAC transcription factor genes ANAC019, ANAC055, and ANAC072 (Zheng et al., 2012) could be assessed in aba2-1 and abi1-1; their reduced expression may contribute to the ABA-dependency of COR-mediated stomatal reopening. It has been demonstrated that reactive oxygen species (ROS) act as essential second messengers in Arabidopsis guard cells, functioning in CO2- and ABA-induced stomatal closure (Pei et al., 2000; Mustilli et al., 2002; Chater et al., 2015). Interestingly, it has recently been reported that COR inhibited ROS production in guard cells, thereby aiding the inhibition of stomatal closure (Toum et al., 2016). Whether ROS production may act as a point of convergence between CO2, ABA, and COR signaling and in doing so determines the outcome of COR responsiveness in ABA mutants and under different CO2 conditions is an important question.
Low CO2 and Defective ABA Signaling Enhance Resistance to Pst, While High CO2 Reduces Resistance
In accordance with the blocked COR-induced stomatal reopening, low CO2-grown plants exhibited significantly reduced growth of Pst at 4 dpi compared with ambient CO2-grown plants (Figures 3C–E, 5B and Supplementary Figure S2). However, this decrease in susceptibility to Pst was apparent both in dip-inoculated and pressure-infiltrated leaves (Figures 3D,E), suggesting that the CO2 effect on Pst infection is beyond the interference of CO2 with stomatal defenses. The in planta growth of Pst under the low CO2 condition was arrested to the same level as that of Pst cor- under low, ambient or high CO2 conditions (Figure 3C). This demonstrates that impairment of COR-mediated defense suppression that is apparent under low CO2 conditions severely reduces the virulence of Pst. It is known that SA plays an essential role in the defense response of Arabidopsis against Pst. By acting as a structural JA mimic, COR triggers a signaling cascade that counteracts SA-dependent defenses, thus promoting susceptibility to Pst infection (Zheng et al., 2012). It is possible that under low CO2 conditions the function of COR is impaired, which alleviates the suppression of downstream SA signaling, resulting in enhanced resistance to Pst.
The inability of the mutants aba2-1 and abi1-1 to respond with stomatal reopening to COR, was associated with enhanced resistance to Pst (Figure 5B and Supplementary Figure S2), indicating the important role of ABA signaling in suppression of defenses by COR. The link with defective responsiveness to COR and enhanced resistance has been shown previously, albeit the role of ABA herein was in closing instead of reopening of the stomata (Lim et al., 2014). Interestingly, the aba2-1 and abi1-1 mutants were under all three CO2 conditions as resistant to Pst as wild-type plants grown at low CO2 (Figure 5B and Supplementary Figure S2) and Pst and Pst cor- grew to a similar level in the aba2-1 mutant (data not shown). Thus, the resistance phenotype of low CO2-grown wild-type plants resembles that of the ABA mutants. Remarkably, also plant growth is inhibited by both low CO2 and ABA-related mutations (Figures 1A,B) (Chatfield et al., 2000; LeNoble et al., 2004). Moreover, in low CO2-grown plants the ABA levels were induced to a lower extent by Pst infection than in ambient or high CO2-grown plants and a trend of reduced ABA content was already visible in the non-infected situation (Figure 5C). In addition, responsiveness to ABA was affected under low CO2, shown by a significantly smaller effect on stomatal closure induced by exogenous application of ABA than under the high and ambient CO2 conditions (Supplementary Figure S3). Altogether, these results suggest that the enhanced resistance to Pst that is evident under the low CO2 condition is related to a decrease in ABA content and signaling.
In addition to a role in COR-triggered stomatal reopening that we demonstrated, ABA is also known to suppress SA defense signaling, possibly in part by activation of the three above-mentioned NAC transcription factors (De Torres-Zabala et al., 2007; Zheng et al., 2012). Modulation of ABA signaling by atmospheric CO2 may affect expression of SA-mediated defense responses. Not only did we show that the ABA mutants aba2-1 and abi1-1 were more resistant to Pst infection, we also demonstrated that the ABA hypersensitive mutant abi1-2 is more susceptible to Pst (Supplementary Figure S4). At high CO2 levels, wild-type Arabidopsis plants were also more susceptible to Pst (Figures 3, 5B and Supplementary Figure S2). We did not detect a significant increase in ABA content under high CO2, although a trend was visible (Figure 5C). Under high CO2 conditions, stomatal aperture was decreased, causing fewer Pst bacteria to enter the leaves, but still at 4 dpi higher bacterial titers were measured, and also upon pressure infiltration enhanced Pst in planta growth was detected (Figures 1C, 3). It is unclear how high CO2 interferes with plant defense responsiveness. Besides an effect on plant defense, the enhanced Pst growth may be caused by a favorable endophytic environment for the bacteria in terms of nutrition and water availability in high CO2-grown plants (Lake and Wade, 2009; Pangga et al., 2011), but this enriched condition was unlikely established within the 4 days time frame in which the plants were transferred from ambient to high CO2 condition, which was used for some of the experiments (Figures 5D,E). Moreover, in contrast to our findings, tomato plants were reported to exhibit reduced infection by Pst at elevated CO2 levels (Li et al., 2014). Thus, enrichment of the endophytic environment alone unlikely explains the full effect of high CO2 on enhanced Pst growth in Arabidopsis that we demonstrated. Possibly, the difference in genetic make-up between Arabidopsis and tomato plants can explain the difference in effect of high CO2 on susceptibility to Pst.
Conclusion
Our results show that atmospheric CO2 influences resistance of Arabidopsis to Pst, whereby pre-industrial, low CO2 levels lead to an increase in resistance. ABA signaling is demonstrated to be a regulator of COR-mediated stomatal reopening and susceptibility to Pst. Under low CO2 conditions ABA levels are reduced, which could explain the defect in COR-mediated stomatal reopening and the enhanced resistance to Pst. The global rise in atmospheric CO2 may be causal for the detected increase in ABA content of plants grown under ambient compared to the low CO2 condition when infected by the Pst pathogen. Further research could aid in developing efficient strategies to maintain agricultural production.
Author Contributions
YZ, CP, and SVW planned and designed the research. YZ conducted the laboratory work. YZ, CP, and SVW analyzed and interpreted the data and wrote the manuscript. IV-V contributed to data analysis and improved the manuscript. RS provided hormone analysis.
Funding
This work was supported by a Chinese Scholarship Council (CSC) Ph.D. scholarship (to YZ), VIDI grant no. 11281 of the Netherlands Organization for Scientific Research (NWO/STW; to SVW), and ERC Advanced Investigator grant no. 269072 of the European Research Council (to CP).
Conflict of Interest Statement
The authors declare that the research was conducted in the absence of any commercial or financial relationships that could be construed as a potential conflict of interest.
Acknowledgment
The authors thank Michel de Vries for running the LC-MS/MS samples and Merel Steenbergen for performing some of the statistical analyses.
Supplementary Material
The Supplementary Material for this article can be found online at: http://journal.frontiersin.org/article/10.3389/fpls.2017.00700/full#supplementary-material
References
Araújo, W. L., Fernie, A. R., and Nunes-Nesi, A. (2011). Control of stomatal aperture: a renaissance of the old guard. Plant Signal. Behav. 6, 1305–1311. doi: 10.4161/psb.6.9.16425
Audenaert, K., De Meyer, G. B., and Höfte, M. M. (2002). Abscisic acid determines basal susceptibility of tomato to Botrytis cinerea and suppresses salicylic acid-dependent signaling mechanisms. Plant Physiol. 128, 491–501. doi: 10.1104/pp.010605
Bowes, G. (1991). Growth at elevated CO2: photosynthetic responses mediated through Rubisco. Plant Cell Environ. 14, 795–806. doi: 10.1093/jxb/err133
Brooks, D. M., Bender, C. L., and Kunkel, B. N. (2005). The Pseudomonas syringae phytotoxin coronatine promotes virulence by overcoming salicylic acid-dependent defences in Arabidopsis thaliana. Mol. Plant Pathol. 6, 629–639. doi: 10.1111/j.1364-3703.2005.00311.x
Brooks, D. M., Hernández-Guzmán, G., Kloek, A. P., Alarcón-Chaidez, F., Sreedharan, A., Rangaswamy, V., et al. (2004). Identification and characterization of a well-defined series of coronatine biosynthetic mutants of Pseudomonas syringae pv. tomato DC3000. Mol. Plant Microbe Interact. 17, 162–174. doi: 10.1094/MPMI.2004.17.2.162
Chakraborty, S., and Datta, S. (2003). How will plant pathogens adapt to host plant resistance at elevated CO2 under a changing climate? New Phytol. 159, 733–742. doi: 10.1046/j.1469-8137.2003.00842.x
Chakraborty, S., Tiedemann, A., and Teng, P. (2000). Climate change: potential impact on plant diseases. Environ. Pollut. 108, 317–326. doi: 10.1016/S0269-7491(99)00210-9
Chater, C., Peng, K., Movahedi, M., Dunn, J. A., Walker, H. J., Liang, Y.-K., et al. (2015). Elevated CO2-induced responses in stomata require ABA and ABA signaling. Curr. Biol. 25, 2709–2716. doi: 10.1016/j.cub.2015.09.013
Chatfield, S. P., Stirnberg, P., Forde, B. G., and Leyser, O. (2000). The hormonal regulation of axillary bud growth in Arabidopsis. Plant J. 24, 159–169. doi: 10.1046/j.1365-313x.2000.00862.x
Coleman, J. S., Mcconnaughay, K. D. M., and Bazzaz, F. A. (1993). Elevated CO2 and plant nitrogen-use: is reduced tissue nitrogen concentration size-dependent? Oecologia 93, 195–200. doi: 10.1007/BF00317671
De Torres-Zabala, M., Truman, W., Bennett, M. H., Lafforgue, G., Mansfield, J. W., Egea, P. R., et al. (2007). Pseudomonas syringae pv. tomato hijacks the Arabidopsis abscisic acid signalling pathway to cause disease. EMBO J. 26, 1434–1443. doi: 10.1038/sj.emboj.7601575
DeLucia, E. H., Nabity, P. D., Zavala, J. A., and Berenbaum, M. R. (2012). Climate change: resetting plant-insect interactions. Plant Physiol. 160, 1677–1685. doi: 10.1104/pp.112.204750
Dermody, O., Long, S. P., and Delucia, E. H. (2006). How does elevated CO2 or ozone affect the leaf-area index of soybean when applied independently? New Phytol. 169, 145–155.
Dodd, A. N., Parkinson, K., and Webb, A. A. R. (2004). Independent circadian regulation of assimilation and stomatal conductance in the ztl-1 mutant of Arabidopsis. New Phytol. 162, 63–70. doi: 10.1111/j.1469-8137.2004.01005.x
Du, M. M., Zhai, Q. Z., Deng, L., Li, S. Y., Li, H. S., Yan, L. H., et al. (2014). Closely related NAC transcription factors of tomato differentially regulate stomatal closure and reopening during pathogen attack. Plant Cell 26, 3167–3184. doi: 10.1105/tpc.114.128272
Eastburn, D. M., Degennaro, M. M., Delucia, E. H., Dermody, O., and Mcelrone, A. J. (2010). Elevated atmospheric carbon dioxide and ozone alter soybean diseases at SoyFACE. Glob. Change Biol. 16, 320–330. doi: 10.1111/j.1365-2486.2009.01978.x
Fujita, M., Fujita, Y., Noutoshi, Y., Takahashi, F., Narusaka, Y., Yamaguchi-Shinozaki, K., et al. (2006). Crosstalk between abiotic and biotic stress responses: a current view from the points of convergence in the stress signaling networks. Curr. Opin. Plant Biol. 9, 436–442. doi: 10.1016/j.pbi.2006.05.014
Garrett, K. A., Dendy, S. P., Frank, E. E., Rouse, M. N., and Travers, S. E. (2006). Climate change effects on plant disease: genomes to ecosystems. Annu. Rev. Phytopathol. 44, 489–509. doi: 10.1146/annurev.phyto.44.070505.143420
Geisler, M., Nadeau, J., and Sack, F. D. (2000). Oriented asymmetric divisions that generate the stomatal spacing pattern in Arabidopsis are disrupted by the too many mouths mutation. Plant Cell 12, 2075–2086. doi: 10.1105/tpc.12.11.2075
Gosti, F., Beaudoin, N., Serizet, C., Webb, A. A., Vartanian, N., and Giraudat, J. (1999). ABI1 protein phosphatase 2C is a negative regulator of abscisic acid signaling. Plant Cell 11, 1897–1909. doi: 10.1105/tpc.11.10.1897
Grimmer, M. K., Foulkes, M. J., and Paveley, N. D. (2012). Foliar pathogenesis and plant water relations: a review. J. Exp. Bot. 63, 4321–4331. doi: 10.1093/jxb/ers143
Hashimoto, M., Negi, J., Young, J., Israelsson, M., Schroeder, J. I., and Iba, K. (2006). Arabidopsis HT1 kinase controls stomatal movements in response to CO2. Nat. Cell Biol. 8, 391–397. doi: 10.1038/ncb1387
Hashimoto-Sugimoto, M., Negi, J., Monda, K., Higaki, T., Isogai, Y., Nakano, T., et al. (2016). Dominant and recessive mutations in the Raf-like kinase HT1 gene completely disrupt stomatal responses to CO2 in Arabidopsis. J. Exp. Bot. 67, 3251–3261. doi: 10.1093/jxb/erw134
Hoagland, D. R., and Arnon, D. I. (1938). The water-culture method for growing plants without soil. Calif. Agric. Exp. Stn. 347, 36–39.
Hu, H. H., Boisson-Dernier, A., Israelsson-Nordström, M., Böhmer, M., Xue, S., Ries, A., et al. (2010). Carbonic anhydrases are upstream regulators of CO2-controlled stomatal movements in guard cells. Nat. Cell Biol. 12, 87–93. doi: 10.1038/ncb2009
Hubbard, K. E., Siegel, R. S., Valerio, G., Brandt, B., and Schroeder, J. I. (2012). Abscisic acid and CO2 signalling via calcium sensitivity priming in guard cells, new CDPK mutant phenotypes and a method for improved resolution of stomatal stimulus–response analyses. Ann. Bot. 109, 5–17. doi: 10.1093/aob/mcr252
Israelsson, M., Siegel, R. S., Young, J., Hashimoto, M., Iba, K., and Schroeder, J. I. (2006). Guard cell ABA and CO2 signaling network updates and Ca2+ sensor priming hypothesis. Curr. Opin. Plant Biol. 9, 654–663. doi: 10.1016/j.pbi.2006.09.006
Jain, V., Pal, M., Raj, A., and Khetarpal, S. (2007). Photosynthesis and nutrient composition of spinach and fenugreek grown under elevated carbon dioxide concentration. Biol. Plant. 51, 559–562. doi: 10.1007/s10535-007-0122-9
Kim, T.-H., and Maik, B. (2010). Guard cell signal transduction network: advances in understanding abscisic acid, CO2, and Ca2+ signaling. Annu. Rev. Plant Biol. 61, 561–591. doi: 10.1146/annurev-arplant-042809-112226
King, E. O., Ward, M. K., and Raney, D. E. (1954). Two simple media for the demonstration of pyocyanin and fluorescin. J. Lab. Clin. Med. 44, 301–307.
Kobayashi, T., Ishiguro, K., Nakajima, T., Kim, H., Okada, M., and Kobayashi, K. (2006). Effects of elevated atmospheric CO2 concentration on the infection of rice blast and sheath blight. Phytopathology 96, 425–431. doi: 10.1094/PHYTO-96-0425
Koornneef, M., Jorna, M., Brinkhorst-Van Der Swan, D., and Karssen, C. (1982). The isolation of abscisic acid (ABA) deficient mutants by selection of induced revertants in non-germinating gibberellin sensitive lines of Arabidopsis thaliana (L.) Heynh. Theor. Appl. Genet. 61, 385–393. doi: 10.1007/BF00272861
Koornneef, M., Reuling, G., and Karssen, C. (1984). The isolation and characterization of abscisic acid-insensitive mutants of Arabidopsis thaliana. Physiol. Plant. 61, 377–383. doi: 10.1111/j.1399-3054.1984.tb06343.x
Kürschner, W., Wagner, F., Visscher, E., and Visscher, H. (1997). Predicting the response of leaf stomatal frequency to a future CO2-enriched atmosphere: constraints from historical observations. Geol. Rundsch. 86, 512–517. doi: 10.1007/s005310050158
Lake, J. A., and Wade, R. N. (2009). Plant–pathogen interactions and elevated CO2: morphological changes in favour of pathogens. J. Exp. Bot. 60, 3123–3131. doi: 10.1093/jxb/erp147
Leakey, A. D. B., Ainsworth, E. A., Bernacchi, C. J., Rogers, A., Long, S. P., and Ort, D. R. (2009). Elevated CO2 effects on plant carbon, nitrogen, and water relations: six important lessons from FACE. J. Exp. Bot. 60, 2859–2876. doi: 10.1093/jxb/erp096
Lee, S., Yang, D. S., Uppalapati, S. R., Sumner, L. W., and Mysore, K. S. (2013). Suppression of plant defense responses by extracellular metabolites from Pseudomonas syringae pv. tabaci in Nicotiana benthamiana. BMC Plant Biol. 13:65. doi: 10.1186/1471-2229-13-65
LeNoble, M. E., Spollen, W. G., and Sharp, R. E. (2004). Maintenance of shoot growth by endogenous ABA: genetic assessment of the involvement of ethylene suppression. J. Exp. Bot. 55, 237–245. doi: 10.1093/jxb/erh031
Leymarie, J., Lascève, G., and Vavasseur, A. (1998). Interaction of stomatal responses to ABA and CO2 in Arabidopsis thaliana. Funct. Plant Biol. 25, 785–791. doi: 10.1093/jxb/erw134
Leymarie, J., Lasceve, G., and Vavasseur, A. (1999). Elevated CO2 enhances stomatal responses to osmotic stress and abscisic acid in Arabidopsis thaliana. Plant Cell Environ. 22, 301–308. doi: 10.1046/j.1365-3040.1999.00403.x
Li, J., Besseau, S., Törönen, P., Sipari, N., Kollist, H., Holm, L., et al. (2013). Defense-related transcription factors WRKY70 and WRKY54 modulate osmotic stress tolerance by regulating stomatal aperture in Arabidopsis. New Phytol. 200, 457–472. doi: 10.1111/nph.12378
Li, P. H., Sioson, A., Mane, S. P., Ulanov, A., Grothaus, G., Heath, L. S., et al. (2006). Response diversity of Arabidopsis thaliana ecotypes in elevated CO2 in the field. Plant Mol. Biol. 62, 593–609. doi: 10.1007/s11103-006-9041-y
Li, X., Sun, Z., Shao, S., Zhang, S., Ahammed, G. J., Zhang, G., et al. (2014). Tomato–Pseudomonas syringae interactions under elevated CO2 concentration: the role of stomata. J. Exp. Bot. 66, 307–316. doi: 10.1093/jxb/eru420
Lim, C. W., Luan, S., and Lee, S. C. (2014). A prominent role for RCAR3-mediated ABA signaling in response to Pseudomonas syringae pv. tomato DC3000 infection in Arabidopsis. Plant Cell Physiol. 55, 1691–1703. doi: 10.1093/pcp/pcu100
Liu, S. A., Kracher, B., Ziegler, J., Birkenbihl, R. P., and Somssich, I. E. (2015). Negative regulation of ABA signaling by WRKY33 is critical for Arabidopsis immunity towards Botrytis cinerea 2100. eLife 4:e07295. doi: 10.7554/eLife.07295
Manning, W. J., and Tiedemann, A. V. (1995). Climate change: potential effects of increased atmospheric carbon dioxide (CO2), ozone (O3), and ultraviolet-B (UV-B) radiation on plant diseases. Environ. Pollut. 88, 219–245. doi: 10.1016/0269-7491(95)91446-R
Mcelrone, A. J., Reid, C. D., Hoye, K. A., Hart, E., and Jackson, R. B. (2005). Elevated CO2 reduces disease incidence and severity of a red maple fungal pathogen via changes in host physiology and leaf chemistry. Glob. Change Biol. 11, 1828–1836. doi: 10.1111/j.1365-2486.2005.001015.x
McLachlan, D. H., Kopischke, M., and Robatzek, S. (2014). Gate control: guard cell regulation by microbial stress. New Phytol. 203, 1049–1063. doi: 10.1111/nph.12916
Melotto, M., Underwood, W., and He, S. Y. (2008). Role of stomata in plant innate immunity and foliar bacterial diseases. Annu. Rev. Phytopathol. 46, 101–122. doi: 10.1146/annurev.phyto.121107.104959
Melotto, M., Underwood, W., Koczan, J., Nomura, K., and He, S. Y. (2006). Plant stomata function in innate immunity against bacterial invasion. Cell 126, 969–980. doi: 10.1016/j.cell.2006.06.054
Merilo, E., Jalakas, P., Kollist, H., and Brosché, M. (2015). The role of ABA recycling and transporter proteins in rapid stomatal responses to reduced air humidity, elevated CO2 and exogenous ABA. Mol. Plant 8, 657–659. doi: 10.1016/j.molp.2015.01.014
Merilo, E., Laanemets, K., Hu, H., Xue, S., Jakobson, L., Tulva, I., et al. (2013). PYR/RCAR receptors contribute to ozone-, reduced air humidity-, darkness-and CO2-induced stomatal regulation. Plant Physiol. 162, 1652–1668. doi: 10.1104/pp.113.220608
Merlot, S., Mustilli, A.-C., Genty, B., North, H., Lefebvre, V., Sotta, B., et al. (2002). Use of infrared thermal imaging to isolate Arabidopsis mutants defective in stomatal regulation. Plant J. 30, 601–609. doi: 10.1046/j.1365-313X.2002.01322.x
Mittal, S., and Davis, K. R. (1995). Role of the phytotoxin coronatine in the infection of Arabidopsis thaliana by Pseudomonas syringae pv. tomato. Mol. Plant Microbe Interact. 8, 165–171. doi: 10.1094/MPMI-8-0165
Montillet, J.-L., Leonhardt, N., Mondy, S., Tranchimand, S., Rumeau, D., Boudsocq, M., et al. (2013). An abscisic acid-independent oxylipin pathway controls stomatal closure and immune defense in Arabidopsis. PLoS Biol. 11:e1001513. doi: 10.1371/journal.pbio.1001513
Murray, D. R. (1995). Plant responses to carbon dioxide. Am. J. Bot. 82, 690–697. doi: 10.2307/2445426
Mustilli, A.-C., Merlot, S., Vavasseur, A., Fenzi, F., and Jérôme, G. (2002). Arabidopsis OST1 protein kinase mediates the regulation of stomatal aperture by abscisic acid and acts upstream of reactive oxygen species production. Plant Cell 14, 3089–3099. doi: 10.1105/tpc.007906
Neill, S., Barros, R., Bright, J., Desikan, R., Hancock, J., Harrison, J., et al. (2008). Nitric oxide, stomatal closure, and abiotic stress. J. Exp. Bot. 59, 165–176. doi: 10.1093/jxb/erm293
Nishimura, N., Sarkeshik, A., Nito, K., Park, S.-Y., Wang, A., Carvalho, P. C., et al. (2010). PYR/PYL/RCAR family members are major in-vivo ABI1 protein phosphatase 2C-interacting proteins in Arabidopsis. Plant J. 61, 290–299. doi: 10.1111/j.1365-313X.2009.04054.x
Pangga, I. B., Hanan, J., and Chakraborty, S. (2011). Pathogen dynamics in a crop canopy and their evolution under changing climate. Plant Pathol. 60, 70–81. doi: 10.1111/j.1365-3059.2010.02408.x
Pei, Z.-M., Murata, Y., Benning, G., Thomine, S., Klusener, B., Allen, G. J., et al. (2000). Calcium channels activated by hydrogen peroxide mediate abscisic acid signaling in guard cells. Nature 406, 731–734. doi: 10.1038/35021067
Pieterse, C. M. J., Van Der Does, D., Zamioudis, C., Leon-Reyes, A., and Van Wees, S. C. M. (2012). Hormonal modulation of plant immunity. Annu. Rev. Cell Dev. Biol. 28, 489–521. doi: 10.1146/annurev-cellbio-092910-154055
Reich, P. B., Hungate, B. A., and Luo, Y. (2006). Carbon-nitrogen interactions in terrestrial ecosystems in response to rising atmospheric carbon dioxide. Annu. Rev. Ecol. Evol. Syst. 37, 611–636. doi: 10.1146/annurev.ecolsys.37.091305.110039
Robert-Seilaniantz, A., Grant, M., and Jones, J. D. G. (2011). Hormone crosstalk in plant disease and defense: more than just jasmonate-salicylate antagonism. Annu. Rev. Phytopathol. 49, 317–343. doi: 10.1146/annurev-phyto-073009-114447
Royer, D. (2001). Stomatal density and stomatal index as indicators of paleoatmospheric CO2 concentration. Rev. Palaeobot. Palynol. 114, 1–28. doi: 10.1016/S0034-6667(00)00074-9
Sage, R. F., and Coleman, J. R. (2001). Effects of low atmospheric CO2 on plants: more than a thing of the past. Trends Plant Sci. 6, 18–24. doi: 10.1016/S1360-1385(00)01813-6
Scala, A., Mirabella, R., Mugo, C., Matsui, K., Haring, M. A., and Schuurink, R. C. (2013). E-2-hexenal promotes susceptibility to Pseudomonas syringae by activating jasmonic acid pathways in Arabidopsis. Front. Plant Sci. 4:74. doi: 10.3389/fpls.2013.00074
Schmid, I., Franzaring, J., Müller, M., Brohon, N., Calvo, O., Högy, P., et al. (2016). Effects of CO2 enrichment and drought on photosynthesis, growth and yield of an old and a modern barley cultivar. J. Agron. Crop Sci. 202, 81–95. doi: 10.1111/jac.12127
Somers, D. E., Webb, A. A. R., Pearson, M., and Kay, S. A. (1998). The short-period mutant, toc1-1, alters circadian clock regulation of multiple outputs throughout development in Arabidopsis thaliana. Development 125, 485–494.
Sun, Y., Guo, H., Yuan, L., Wei, J., Zhang, W., and Ge, F. (2015). Plant stomatal closure improves aphid feeding under elevated CO2. Glob. Change Biol. 21, 2739–2748. doi: 10.1111/gcb.12858
Temme, A. A., Liu, J. C., Cornwell, W. K., Cornelissen, J. H., and Aerts, R. (2015). Winners always win: growth of a wide range of plant species from low to future high CO2. Ecol. Evol. 5, 4949–4961. doi: 10.1002/ece3.1687
Teng, N., Wang, J., Chen, T., Wu, X., Wang, Y., and Lin, J. (2006). Elevated CO2 induces physiological, biochemical and structural changes in leaves of Arabidopsis thaliana. New Phytol. 172, 92–103. doi: 10.1111/j.1469-8137.2006.01818.x
Thaler, J. S., and Bostock, R. M. (2004). Interactions between abscisic-acid-mediated responses and plant resistance to pathogens and insects. Ecology 85, 48–58. doi: 10.1890/02-0710
Tian, W., Hou, C., Ren, Z., Pan, Y., Jia, J., Zhang, H., et al. (2015). A molecular pathway for CO2 response in Arabidopsis guard cells. Nat. Commun. 6:6057. doi: 10.1038/ncomms7057
Tissue, D. T., and Lewis, J. D. (2012). Learning from the past: how low CO2 studies inform plant and ecosystem response to future climate change. New Phytol. 194, 4–6. doi: 10.1111/j.1469-8137.2012.04081.x
Ton, J., Flors, V., and Mauch-Mani, B. (2009). The multifaceted role of ABA in disease resistance. Trends Plant Sci. 14, 310–317. doi: 10.1016/j.tplants.2009.03.006
Toum, L., Torres, P. S., Gallego, S. M., Benavídes, M. P., Vojnov, A. A., and Gudesblat, G. E. (2016). Coronatine inhibits stomatal closure through guard cell-specific inhibition of NADPH oxidase-dependent ROS production. Front. Plant Sci. 7:1851. doi: 10.3389/fpls.2016.01851
Vlot, A. C., Dempsey, D. M. A., and Klessig, D. F. (2009). Salicylic acid, a multifaceted hormone to combat disease. Annu. Rev. Phytopathol. 47, 177–206. doi: 10.1146/annurev.phyto.050908.135202
Wang, D., Heckathorn, S. A., Wang, X., and Philpott, S. M. (2012). A meta-analysis of plant physiological and growth responses to temperature and elevated CO2. Oecologia 169, 1–13. doi: 10.1007/s00442-011-2172-0
Whalen, M. C., Innes, R. W., Bent, A. F., and Staskawicz, B. J. (1991). Identification of Pseudomonas syringae pathogens of Arabidopsis and a bacterial locus determining avirulence on both Arabidopsis and soybean. Plant Cell 3, 49–59. doi: 10.1105/tpc.3.1.49
Xue, S. W., Hu, H. H., Ries, A., Merilo, E., Kollist, H., and Schroeder, J. I. (2011). Central functions of bicarbonate in S-type anion channel activation and OST1 protein kinase in CO2 signal transduction in guard cell. EMBO J. 30, 1645–1658. doi: 10.1038/emboj.2011.68
Yáñez-López, R., Torres-Pacheco, I., Guevara-González, R. G., Hernández-Zul, M. I., Quijano-Carranza, J. A., and Rico-García, E. (2014). The effect of climate change on plant diseases. Afr. J. Biotechnol. 11, 2417–2428.
Zeng, W., Brutus, A., Kremer, J. M., Withers, J. C., Gao, X., Jones, A. D., et al. (2011). A genetic screen reveals Arabidopsis stomatal and/or apoplastic defenses against Pseudomonas syringae pv. tomato DC3000. PLoS Pathog. 7:10. doi: 10.1371/journal.ppat.1002291
Zeng, W., Melotto, M., and He, S. (2010). Plant stomata: a checkpoint of host immunity and pathogen virulence. Curr. Opin. Biotechnol. 21, 599–603. doi: 10.1016/j.copbio.2010.05.006
Zhang, S., Li, X., Sun, Z., Shao, S., Hu, L., Ye, M., et al. (2015). Antagonism between phytohormone signalling underlies the variation in disease susceptibility of tomato plants under elevated CO2. J. Exp. Bot. 66, 1951–1963. doi: 10.1093/jxb/eru538
Keywords: atmospheric CO2, Arabidopsis resistance, ABA signaling, coronatine, stomata
Citation: Zhou Y, Vroegop-Vos I, Schuurink RC, Pieterse CMJ and Van Wees SCM (2017) Atmospheric CO2 Alters Resistance of Arabidopsis to Pseudomonas syringae by Affecting Abscisic Acid Accumulation and Stomatal Responsiveness to Coronatine. Front. Plant Sci. 8:700. doi: 10.3389/fpls.2017.00700
Received: 15 December 2016; Accepted: 18 April 2017;
Published: 16 May 2017.
Edited by:
Hua Lu, University of Maryland, Baltimore County, USAReviewed by:
Harsh Bais, University of Delaware, USAGustavo Eduardo Gudesblat, National Scientific and Technical Research Council, Argentina
Murray Grant, University of Warwick, UK
Copyright © 2017 Zhou, Vroegop-Vos, Schuurink, Pieterse and Van Wees. This is an open-access article distributed under the terms of the Creative Commons Attribution License (CC BY). The use, distribution or reproduction in other forums is permitted, provided the original author(s) or licensor are credited and that the original publication in this journal is cited, in accordance with accepted academic practice. No use, distribution or reproduction is permitted which does not comply with these terms.
*Correspondence: Saskia C. M. Van Wees, cy52YW53ZWVzQHV1Lm5s