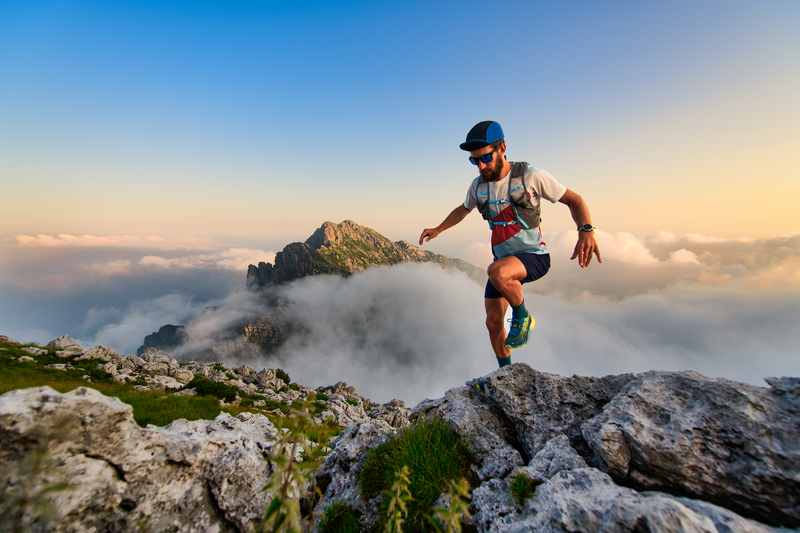
95% of researchers rate our articles as excellent or good
Learn more about the work of our research integrity team to safeguard the quality of each article we publish.
Find out more
ORIGINAL RESEARCH article
Front. Plant Sci. , 02 May 2017
Sec. Plant Breeding
Volume 8 - 2017 | https://doi.org/10.3389/fpls.2017.00666
This article is part of the Research Topic Tailoring crops for higher water productivity View all 12 articles
Increasing water use efficiency and reducing nitrogen pollutant discharge are important tasks for modern agriculture. To evaluate the effect of alternate partial root-zone irrigation (APRI) on tomato plant growth, water use efficiency and nitrate-15N uptake, an experiment was conducted from June to December in 2014 under greenhouse condition in northern China. The experiment contained two irrigation patterns [APRI and conventional irrigation (CI)], two 15N labeled depths in soil (10 and 50 cm) and two transplant time (early and late summer). Results showed that, compared to CI, APRI did not significantly (p > 0.05) impact the growth and biomass accumulation in aboveground part of tomato, while it enhanced the root, reflecting by greater length density, and more dry mass. APRI produced marginally lower yields, but saved 34.9% of irrigation water, and gave a 37.6–49.9% higher water use efficiency relative to CI. In addition, APRI improved fruit quality, mainly through increasing the contents of soluble solid (by 12.8–21.6%), and vitamin C (2.8–12.7%), and the sugar/acid ratio (3.5–8.5%). The 15N utilization efficiency (15NUE) in APRI was higher than that in CI, which was more evident when 15N was labeled at 50 cm depth. Significant (p < 0.05) 15N recovery increase of 10.2–13.2% and 15N loss decrease of 35.4–54.6% were found for APRI compared to CI. The overall results suggest that APRI under greenhouse could benefit the nitrate-N recovery and increase the water use efficiency in tomato.
Greenhouse agriculture achieves great success in many countries like Netherlands (Korthals Altes and van Rij, 2013), Israel (Teitel and Zhao, 1992; Elad et al., 2014), Japan (Kinoshita et al., 2016), and the United States (Burnett et al., 2016). China has the world's largest area of greenhouse agriculture, however, more than 90% of the greenhouses use primitive facilities, and soil culture is still the main method for crop production (Du, 2007). In a long time, the purpose of China's vegetable production is to acquire high yield, the energy, water and fertilizer resources are seriously overused, leading to a serious waste of agricultural inputs. A survey has shown that in China, average inorganic N input for one season vegetable under greenhouse is 569–2,000 kg/ha, which is several times or even dozen times over that applied to field crop, quantities of the applied fertilizer nitrogen are residual in the soil (Dorais et al., 2005).
The residual inorganic N in dryland soil is mainly existed in nitrate nitrogen (-N) form, which is difficult to be absorbed by soil particles (Wang X. et al., 2015), and is easy to migrate downward along with the irrigation water (Kanthle et al., 2016). Due to the weak denitrification effect, -N in the deep soil layer is hard to transform to other N forms, it will move to the deeper soil layer and pose a threat to the underground water environment (Stefanelli et al., 2010). -N leaching is influenced by various factors, the vertical movement of soil water is one of the most important factors that affecting the distribution of -N in profile soil (Wallis et al., 2011). Some studies have employed innovative irrigation methods to change the -N location and the crop -N uptake (Sepaskhah and Tafteh, 2012; Liu et al., 2015; Wang X. et al., 2015).
In recent years, alternate partial root-zone irrigation (APRI) has been shown to be an effective irrigation technique in many regions of the world (Wei et al., 2016). APRI irrigates only part of the root zone leaving the other part to dry to a predetermined level before the next irrigation, it is a further development of deficit irrigation (Wang et al., 2012). APRI is found to improve water productivity of crop production without much yield reduction (Dodd, 2009; Du et al., 2015) including in tomato (Sarker et al., 2016; Wei et al., 2016). The APRI-induced plant responses include reduced leaf initiation and expansion rate and decreased inefficient transpiration but without significant reduction in photosynthesis, thus increasing the intrinsic water use efficiency (WUE) (Wei et al., 2016). Sezen et al. (2011) conducted a 2-year experiment in the eastern Mediterranean region of Turkey and revealed that partial root-zone irrigation is acceptable for sunflower production under a water scarcity situation, which received about 36% less irrigation water, reduced the yield by 15%, but resulted in a dramatically high WUE of 1.0 kg m−3. Topak et al. (2016) study in semi-arid area demonstrated that, APRI with 50% full irrigation water increased the root WUE of sugar beet by 19.8% compared to full irrigation, and by 8.5% compared to conventional deficit irrigation with a same amount of applied water. Moreover, the root dry biomass is found higher in APRI plants than that in conventional deficit irrigation and full irrigation plants (Wang et al., 2012). One reason is that plants may effectively forage for water in APRI treatment by proliferating their roots into the wetted root-zones (Du et al., 2008), and the other reason is that alternating the wet and dry parts of the root-zone stimulates growth of the previously dry root system (Mingo et al., 2004). In addition, Sun (Sun et al., 2013) reported that APRI significantly increases plant N content in relation to the conventional deficit irrigation practice. However, when using 50% of the full irrigation amount, APRI showed significant yield decrease (by 52%) in processing tomato, according to Casa and Rouphael's research (Casa and Rouphael, 2014). Although, many positive or negative effects of APRI have been reported in many earlier literatures, these researches focused mainly on the roles played by APRI on the water saving or the crop performance, few studies have looked into the effect of APRI on the soil nutrient, particularly on the soil -N.
Crop yield formation is greatly influenced by the environmental factors such as light and temperature under different growth seasons (Tijskens et al., 2016). In recent years, tomato has quickly become one of the major vegetables grown in solar greenhouses of China because of its high potential yield, water productivity and profitability (Maomao et al., 2014). For greenhouse tomato, it is of great importance to select suitable transplant season. Excessive irradiance and temperature facilitate the occurrence of the blossom-end rot incidence and increase the yield loss in tomato (Kanechi et al., 2013). On the contrary, under a relatively lower temperatures (day and night temperature of 16/14°C), the early yield is delayed when compared to the conventional ones (day and night temperature of 20/18°C) although will be balanced out by higher yields in later harvests (Kläring et al., 2015).
Modern agriculture is now faced with two tasks: (1) to maintain crop yield and quality while increasing water use efficiency, and (2) to reduce agricultural pollutant outputs in greatest degree when irrigating (Djurović et al., 2016; Tang et al., 2016). In this study, it is hypothesized that the yield, quality, water saving in tomato (Solanum lycopersicum L) can reach a compromise under APRI. We also hypothesized that the lower water supply in APRI may keep nitrate-N in shallower soil layer, thus may increase the plant N uptake compared to conventional irrigation. Therefore, we conducted an experiment in northeastern China that using 15N tracing technique as research method, to investigate: (1) the effect of APRI on the tomato growth, biomass accumulation, quality, yield and WUE at different transplant time, and (2) the effect of APRI on the recovery and loss of soil -N. The results are expected to provide useful information for the application of APRI under greenhouse condition, and for the reutilization of soil residual fertilizer nitrogen.
The experiments were carried out in the Production Base of Greenhouse Vegetables (longitude 126°22′E, latitude 46°12′N) of Lanxi county, Suihua city, Heilongjiang province (Experiments were permitted by the owner of the fields named Jiahui Hou). Suihua city is located in the northern hemisphere temperate zone. Suihua has four distinct seasons, with snow covering in winter season, while the climate of summer season is warm and humid. Moreover, the maximum average annual temperature from 2000 to 2013 is in a range of 18.4° to 26.6°C, while the minimum is from −13.2° to −24.8°C. The frost-free season is in a period of 120–140 days, and the sunshine duration are 2,600–2,900 h. The annual average amount of rainfall is 483 mm. The rainfalls occur intensively in summer, particularly in July and August.
The experiments were conducted in a solar greenhouse with span of 10 m, length of 80 m and back wall height of 3 m. Several vents were installed for ventilating and cooling when intensely high temperature occurred, and the height from the ground to the vent was 1 m (Figure 1). The crops in this study were transplanted at different dates respectively in early summer and late summer. During the whole growth stage of crop, the day/night average temperature was 24/20°C in early summer, and 20/18°C in late summer. The soil in the greenhouse was classified as silt loam, its physicochemical properties, measured prior to the early-summer experiment, were listed in Table 1.
Figure 1. Solar greenhouse for the experiment (During whole growth stage of crop, the day/night average temperature was 24/20°C in early summer, and 20/18°C in late summer).
The experiment included two irrigation patterns, two 15N labeled depths in soil, and two transplant dates, thus there were 2 × 2 × 2 = 8 treatments in total. The 15N was labeled at 10 cm and 50 cm soil depth, respectively. The irrigation patterns contained APRI and conventional irrigation (CI). The transplant dates were June 18, 2014 and August 22, 2014, respectively (differed by 9 weeks), corresponding to early and late summer. Detailed experimental design was also shown in Table 2.
The tomato cultivar used was “Red Ruby.” The experiment under solar greenhouse in northern China showed that controlling the lower limit of soil moisture at 70%θf (field capacity in 0–20 cm soil layer, 32.6%) and upper limit at 90%θf could reach an optimal compromise among WUE, yield and quality of tomato (Lv, 2013). Based on the results of previous study, the soil moisture of CI in this study was controlled at a lower limit of 70%θf, and an upper limit of 90%θf, during the whole growth stage of tomato. Early studies proved that APRI could save 40% irrigation water while not significantly reduce the crop yield (Du et al., 2006; Wang, 2014). Thus, in our study, total irrigation amount of APRI was designed as 60% of the amount of CI. Once the soil moisture (0–20 cm layer) in CI was close to 70%θf, the irrigation started and the amount was recorded, then 60% of the recorded irrigation amount was provided to one-side of root-zone in APRI, and next time changing to the other side. For both seasons, tomatoes were irrigated by the different patterns from 28 days after transplant (DAT), the same amount of 62 mm irrigation water among the treatments was applied for seedling survival during 0–28 DAT. The total irrigation amount of CI and APRI was recorded as 498 and 324 mm, respectively, at the transplant time of early summer (TES). Similarly, the total irrigation amount of CI and APRI was recorded as 476 and 310 mm, respectively, at the transplant time of late summer (TLS). The soil moisture was measured using the Delta-T ML2X soil moisture meter, and the irrigation was conducted by hand. Each irrigation and its impact on the volumetric soil water content for CI treatments were shown in Figure 2.
Figure 2. Each irrigation and its impact on volumetric soil water content for the conventional treatments at transplant time of early summer (A) and later summer (B).
The experiment was conducted in several soil columns that pre-buried in the fields. The soil column was prepared using PVC cylindrical mold with a height of 1 m and a diameter of 40 cm, and the bottom of mold was not sealed. Plastic films were used and kept closely to the inner side of the mold. The soils were digged out as 20 cm depth per layer and were filled into the mold as original layers of the field. To avoid the deflecting of mold, the backfill soils were kept the same height for inside and outside the mold during the filling process. Soils in 0–20 cm layer were mixed with NH4NO3, Ca(H2PO4)2 and K2SO4 to provide nutrients needed by tomato, and the dosage was 100 mg/kg N (3.5 g per column), 150 mg/kg P2O5 (5.3 g per column) and 150 mg/kg K2O (5.3 g per column). The K15NO3 with the abundance of 10.57% was adopted as the labeling material, and the 15N labeled layer was 10 cm in thickness, as was shown in Figure 2. For each soil column, the dosage of 15N was 466 mg. It should be noticed that, since K15NO3was used to make the 15N labeling, an K2O amount of 1.5 g was added into each column along with the 15N. After the soil backfilling and the 15N labeling, the mold was taken out from the field, leaving the plastic film separating the soils inside and outside the column. The dissolved urea was used as additional fertilizer, and was applied two times, for each time the N application amount was 60 mg/kg. The additional fertilizer was applied at 55 and 76 DAT respectively, according to local practice. Besides, a film separator with 20 cm height was buried in the middle of each soil column for APRI treatment, 5 cm height of the separator was left out of the soil surface (Figure 3). The separator opened a gap in the center for the tomato planting.
Figure 3. Diagrammatic sketch of 15N labeling in soil column [Soil columns of (1) and (3) are for the plants with alternate partial root-zone irrigation, (2) and (4) are for the plants with conventional irrigation].
To avoid the interaction in leached 15 between treatments with different 15N labeled locations, those treatments with the same labeled location were arranged together. The detailed soil column arrangement was displayed in Figure 4. The distance between two adjacent columns was 20 cm. The distance between the two plots for different transplant time was 40 cm.
Figure 4. Arrangement of soil columns (In block 1 and block 5, the plants are treated with alternate partial root-zone irrigation, and 15N is labeled at 10 cm depth in the soil; in block 3 and block 7, the plants are treated with alternate partial root-zone irrigation, and 15N is labeled at 50 cm depth in the soil; in block 2 and block 6, the plants are treated with conventional irrigation, and 15N is labeled at 10 cm depth in the soil; in block 4 and block 8, the plants are treated with conventional irrigation, and 15N is labeled at 50 cm depth in the soil).
The tomato fruits were harvested in batches during the maturity stage. The first harvest were done at 56–76 DAT (Table 3), and the harvest duration were from 41 to 53 days. The fallen leaves were collected during the whole growth stage of tomato. The tomato plants were collected separately as root, stem, leaf and fruit after the experiment. A 10 cm-diameter root drill (KHT-016, produced by Kanghua Electronic Instrument co., LTD, Jintan City, China) was used to collect root samples, respectively from 0–20, 20–40, 40–60, 60–80 to 80–100 cm soil layer. The root components were carefully seeked out from the soil using tweezers.
After harvest, soil samples were collected using a soil auger as 10 cm per layer. Ten samples in a total depth of 1 m were collected for each soil column.
At each harvest time, the number and weight of tomatoes were recorded, and the tomato yield was calculated after the last harvest.
The tomatoes in the first and third layer of the plant were used for the quality determination. In each treatment, twelve ripening (when the fruits turned red) fruits (6 from first layer and 6 from the third) with similar appearance were randomly collected from the six plants. For each fruit, 10 g tomato flesh was taken along the longitudinal axis and homogenized for quality measurements. The following components contributed greatly to the tomato quality: volume, density, soluble solids, total acid, vitamin C and sugar/acid ratio. The fruit volume was measured by the displacement method. The soluble solids were measured using a ACT-1E digital refractometer (ATAGO company, Japan). The total sugar was measured by Fehling reagent titration method. The total acid was measured by the sodium hydroxide titration method. The vitamin C content was measured by 2, 6-dichloroindophenol titrimetric method (Zhai et al., 2015).
The plant samples were placed into an oven and were dried firstly at 105°C for 30 min, then at 70°C until achieving the constant weight. The soil samples were air dried naturally in open space. Dried soil samples were ground and sieved through 0.15 mm mesh for 15N measuring. The 15N atom percent excess in plant and soil samples was measured using the mass spectrometer (Finniga-Mat-251, Finnigan, Germany) at Nanjing Institute of Soil Science, CAS.
The root samples of tomato were cleaned and scanned by the EPSON EXPRESSION 1680 scanner, then analyzed using the WinRHIZO software to get the data of root length density.
Tomato LAI was simulated using the model proposed by Qin et al. (2008):
Where, LAI and t is leaf area index and the days after transplanted, respectively. LAIM, LAI0, α, β, and τ are the parameters that will be determined according to the measured values of LAI and t (LAI were measured during 10–90 DAT, in 10-day intervals). LAIM is the theoretical upper limit of LAI, LAI0 is the theoretical lower limit of LAI, α, β is the constants, τ is the days that needed to reach 1/2 LAIM.
The WUE (kg m−3) is calculated by the following equation:
where Y is the tomato yield (t ha−1), ET is the evapotranspiration (mm). ET is calculated using the water balance equation of the farm land (Hou and Shao, 2016):
where, P is the valid rainfall (mm), I is the irrigation amount (mm), U is the groundwater recharge (mm), R is the runoff (mm), D is the deep percolation (mm), and ΔW is the variation of soil moisture before and after the experiment (mm). For this study, P, U (the ground water level is below 9 m), R and D can be ignored. The equation can thus be simplified as:
The 15N use efficiency (15NUE, %) was calculated as (Liang et al., 2013):
Where, Ndff is the 15N amount in tomato organ (mg), Cs is the total N amount in tomato organ (mg), Es is the 15N atom percentage excess of tomato organ, Ef is the 15N atom percentage excess of the 15N labeled fertilizer, Mf is the amount of 15N labeled fertilizer (mg).
The amount of15N recovery amount (mg per soil column) contains the 15N in 0–100 cm soil layer and the 15N absorbed by tomato plant. The15N recovery rate is the ratio of 15N recovery amount and 15N application amount (Liang et al., 2013).
The 15N loss (mg per soil column) is calculated using the 15N application amount minus the 15N recovery amount. The 15N loss rate is the ratio of 15N loss and 15N application amount (Hou et al., 2017).
The data were compared statistically using Duncan's multiple range test in SPSS software Version 17.0. Data from the same treatment but different planting seasons were also statistically analyzed.
As was shown in Table 3, both plant height and stem diameter were significantly affected by 15N labeled depth (p < 0.01) or transplant time (p < 0.05), but were not significantly (p > 0.05) affected by irrigation pattern. The insignificant difference of plant growth between two irrigation patterns might be that: (1) APRI reduced the ineffective water consumption for crop (Centritto et al., 2005), thus the growth of tomato have not been obviously affected. (2) APRI was proved by the early study to maintain the optimal aeration and moisture condition in soil and enhance the activities of soil microorganisms (Wang, 2008), which might have benefited the plant N uptake and the plant growth, although the irrigation amount in APRI was lower. At 30 DAT, compared to the labeled depth of 50 cm, 10 cm labeled depth significantly (p < 0.05) increased the plant height by 16.8–28.3%, and the stem diameter by 4.8–15.3%. At 60 DAT, the plant height, as well as stem diameter, showed no significant (p > 0.05) difference between APRI and CI treatments, while they were significantly (p < 0.05) increased by the shallower labeled depth of 15N. CI10 registered greatest plant height (90.3 cm) and stem diameter (1.22 cm) at TES, while the lowest (74.3 cm and 0.94 cm) were in APRI50 at TLS. Generally, the tomatoes with TES obtained higher plant height and stem diameter than that with TLS, when the irrigation pattern and labeled depth were the same. Compared to CI, APRI delayed the time of first harvesting, which was particularly obvious at TES. Moreover, the duration needed for the first harvesting was noticeably influenced by the transplant time. TLS delayed 11.8 days, on average, in the first harvest of tomato, when compared to TES. Similar result obtained by Kläring (Kläring et al., 2015) showed that one Kelvin reduction in temperature would result in a 3.5-day delay of the first harvest.
The response of LAI to the treatments varying with DAT is shown in Figure 5. Under the same DAT, the labeled depth of 10 cm significantly (p < 0.05) increased the LAI compared to that of 50 cm. Nevertheless, no obvious difference of LAI was found between APRI and CI except the slight decrease found in APRI. There were differences between TES and TLS in the dynamics of LAI. Compared to TES, TLS achieved a greater increase rate of LAI after 60 DAT, although LAI at TLS was smaller than that at TES at any time point during 0–60 DAT. At 90 DAT, the greatest LAI were found in CI10, and were 8.8 and 8.5 for TES and TLS, respectively.
Figure 5. Tomato leaf area index varying with the days after transplanted (APRI10 and APRI50 represent that 15N is labeled at 10 and 50 cm soil depths respectively under alternate partial root-zone irrigation, while CI10 and CI50 represent that 15N is labeled at 10 and 50 cm soil depths respectively under conventional irrigation). Tomato leaf area index varying with the days after transplanted at different transplant times of early summer (A) and late summer (B).
The simulation model well reflected the dynamic of LAI (Table 4), with correlation coefficient of 0.978–0.999. Compared to CI, APRI reduced the time needed by the tomato to obtain 1/2 LAIM, this was more obvious at TES. As was calculated by the model, APRI slightly decrease the LAIM by 2.3–3.8%. However, LAIM was noticeably increased by the shallower labeled depth of 15N, 10 cm labeled depth increased which by 12.0–14.3% and 13.0–13.2%, respectively under APRI and CI. The model also showed that under the same irrigation pattern and labeled depth of 15N, LAIM showed almost no difference between TES and TLS.
For both transplant times, APRI had no significant (p > 0.05) effect on the biomass of leaf, stem, fruit, as well as their sum, when the labeled depths were the same. While under the same irrigation patterns, the biomass of the plant parts were significantly (p < 0.01) influenced by the labeled depth of 15N (Table 5). Compared to 50 cm, the 10 cm labeled depth significantly (p < 0.05) increased the leaf biomass by 11.3–12.6%, the stem by 11.2–24.2% and the fruit by 9.5–13.0%, for both transplant times. The applied 15 could have contributed to a higher dry matter accumulation of tomato (Wang, 2014; Badr et al., 2016). An insignificant decrease in biomass of leaf, stem and fruit was found in TLS when compared to TES. The tomato with CI10 at TES obtained highest fruit biomass of 249.8 g plant−1, although it had not significant (p > 0.05) difference compared to that with APRI10. The total biomass aboveground were ranged from 458.1 to 539.1 g plant−1, the highest was registered by CI10 at TES while the lowest was in APRI50 at TLS.
Overall, the root dry weight and length density at all soil layers were significantly affected by the irrigation pattern (Table 6). Compared to CI, APRI promoted the root growth in varying degrees, agreeing with Wang et al. (2012) findings that the crop root was enlarged by APRI compared to full irrigation. This mainly due to the fact that APRI distributed more photosynthetic products to the root, moreover, the dry-wet condition under APRI stimulated the compensatory growth for root (Li et al., 2011). Oppositely, Abrisqueta et al. (2008) reported a 42% reduction in root length density under APRI, possibly because he adopted a lower irrigation amount (50% relative to the full irrigated treatment). Besides, the root attributes were also significantly influenced by 15N labeling, this influence was more evident in the labeling layer, proving that the tomato root was sensitive to nitrate 15N supply. Early study also showed that -N could induce the growth and development of crop lateral roots effectively, thus increase root dry weight and length density (Centritto et al., 2005). In this study, the greatest root dry weight and length density were both found in APRI10 at TES, and were 0.352 mg cm−3 and 0.672 cm cm−3, respectively. Although, the transplant time had no obvious impact on root dry weight, as well as root length density, both of them were found slightly lower at TLS compared to that at TES.
Compared to CI, APRI slightly decreased the yield of tomato (Figure 6). APRI10 reduced tomato yield by 6.1 and 5.4%, and APRI50 reduced it by 4.4 and 7.4%, respectively corresponding to TES and TLS, but the yield reduction by APRI was not significant (p > 0.05). The 15N labeled depth had obvious effects on the yield of tomato. APRI10 significantly (p < 0.05) increased the yield by 7.0 and 11.4%, respectively at TES and TLS, when compared to APRI50. Similarly, CI10 significantly (p < 0.05) increased the yield by 8.7 and 9.3% compared to CI50. With regards to the significant difference in yield and growth of the two 15N labeled depth treatments, it could be explained by that, when 15N was at 10 cm layer, equivalent to 13.3% higher amount of basal N was applied for the early growth of plant; while if at 50 cm layer, when the tomato root was able to capture 15N, great amount of the 15N have been leached below the main root zone and remained unavailable for utilization by the tomato root. In addition, although TES increased the tomato yield relative to TLS, the yield increase by TES was not significant (p > 0.05).
Figure 6. Yield and water use efficiency of tomato with different treatments (APRI10 and APRI50 represent that 15N is labeled at 10 and 50 cm soil depths respectively under alternate partial root-zone irrigation, CI10, and CI50 represent that 15N is labeled at 10 and 50 cm soil depths respectively under conventional irrigation. The means followed by the same letter (a, b, c, d) do not differ significantly at 5% level according to Duncan's multiple range test, and the eight means were compared together. Each value is the mean ± SD).
In comparison to CI, the APRI treatments significantly (p < 0.05) increased tomato WUE by 37.6–49.9%. However, the WUE was basically unaffected by the 15N labeled depth. Besides, the WUE of tomato at TES were slightly higher relative to that at TLS, while the difference of WUE between TES and TLS was not significant (p > 0.05) except that found in APRI10.
To find the compromise between yield producing and water saving, is the key task for developing an optimal irrigation scheme (Wang et al., 2011). The insignificant decrease in yield but significant increase in WUE by this study confirmed the previous findings by Kirda et al. (2004). Accumulated results on that APRI allowed considerable water savings while maintained yield were not only found in tomato (Wei et al., 2016), but in many other crops such as potato (Sun et al., 2013), maize (Wang et al., 2012), apple (Du et al., 2017), grape (Du et al., 2008), peach (Abrisqueta et al., 2008), and so on.
The indicators that contributed greatly to fruit quality were shown in Table 7 for the treatments. Compared to CI, APRI had no significant effect (p > 0.05) on the fruit density and volume, while it increased the contents of soluble solid, total acid, vitamin C and sugar/acid ratio in various degrees. The highest soluble solid, vitamin C contents and sugar/acid ratio were all found in APRI10 at TLS, and were 6.3%, 14.2 mg 100g−1 and 10.2, respectively. This indicated that APRI contributed to a higher nutrient accumulation and a better taste of fruit compared to CI. Our result confirmed the result of Yang (Yang et al., 2012) who reported that APRI increased contents of vitamin C and soluble sugar in tomato by 12.6 and 4.5% in comparison to CI. Zegbe (Zegbe et al., 2004) noted that APRI could advance the maturity of tomato fruit early, make fruit redder, increase the soluble solid content, and promote the sugar transfer from the vegetative organs to fruits to ensure fruit development thus improve the fruit quality. Similar research by Du (Du et al., 2008) showed that APRI increased vitamin C content in the fruit of grape by 15.3–42.2% and the ratio of total soluble solids/titrated acid as compared to conventional drip irrigation. The previous study revealed that when reducing irrigation water supply, the water consumption used for osmotic regulation in peel was reduced, leading to an increase in vitamin C; meanwhile, the content of sugar that entering from phloem to fruit was increased, this contributed to a higher soluble solid content in tomato (Mahajan and Singh, 2006).
Otherwise, although the density, volume, contents of soluble solid and total acid were not obviously influenced by the 15N labeled depth, the vitamin C content and sugar/acid ratio were significantly (p < 0.05) increased by the labeled depth of 10 cm. Compared to APRI50, APRI10 increased the vitamin C content and sugar/acid ratio by 17.0 and 11.4% at TES, and 11.8 and 14.6% at TLS. Similarly, compared to CI50, CI10 increased the vitamin C content and sugar/acid ratio by 11.9 and 8.2% at TES, and 10.5 and 14.6% at TLS.
Overall, the transplant time had no significant (p > 0.05) effect on the soluble solid, total acid, vitamin C contents and sugar/acid ratio. However, the volume of tomato at TLS were significantly (p < 0.05) greater than that at TES, when the irrigation pattern and the labeled depth were the same, this has proved the Kläring's (Kläring et al., 2015) findings. The greatest tomato volume (142.6 cm3) was registered by CI10 at TLS, while the lowest (118.4 cm3) was in APRI50 at TES.
Controlling nitrate outputs from arable land has become an arduous task for modern agriculture in China (Ju et al., 2006; Lenka et al., 2013; Wang Z. H. et al., 2015). Taking into account the likely contribution of the water-saving irrigation to crop nitrate utilization, is an alternative opinion for increasing nitrate nitrogen recovery. In this study, the 15N uptake in all organs were significantly (p < 0.05) affected by irrigation pattern (Table 8). Overall, when the 15N was labeled at 50 cm soil depth, the 15N amount in each plant part under APRI was significantly (p < 0.05) higher than that under CI. In comparison to CI50, APRI50 significantly (p < 0.05) increased the 15N amount of the whole plant by 13.5 and 11.2%, respectively at TES and TLS, indicating that APRI contributed to a higher uptake of 15N that in deeper soil layer. While when the 15N was labeled at 10 cm soil depth, there were no significant (p > 0.05) difference of plant 15N between APRI and CI treatments. The significantly (p < 0.05) higher 15NUE by APRI when 15N was labeled at 50 cm depth might be explained two reasons: (1) An enlarged root system. Greater dry weight and length density of root were observed under APRI (Table 6). In addition, the root surface area of tomato for nitrogen uptake could be enhanced by APRI as has been indicated in earlier study (Mingo et al., 2004). (2) A high 15N availability. APRI maintained the 15N in a shallower soil layer relative to CI (Figure 6). Similar result was also reported by Skinner (Skinner et al., 1999) that the alternate furrow irrigation successfully reduced the potential of -N leaching. Moreover, the soil under APRI was proved to have higher microbial biomass (Liu et al., 2015) and accelerated mineralization rate of organic nitrogen (Wang et al., 2010) thus increasing mineral nitrogen available to plants. However, it should be noticed that variation in plant 15N could also ascribe to nitrogen isotope fractionation within the plant; for instance, volatilization of NH3through stomata preferring 14N might result in an increase of plant 15N (Wang et al., 2012).
Under the same irrigation pattern, 15N labeled at 10 cm depth significantly (p < 0.05) increased the 15N amount in the plant parts than that at 50 cm, for both transplant time. Besides, the whole plant under TES had a significantly (p < 0.05) higher 15N amount than under TLS, when the 15N was labeled at 10 cm depth. However, when labeled at 50 cm, the 15N difference of the whole plant between two transplant times was not significant (p > 0.05). These indicated that the tomato transplanted in early summer could absorb more N from the shallow soil layer. The greatest 15N amount for the whole plant (130.2 mg plant−1) in this study was detected in APRI10 at TES, and the corresponding 15NUE (27.9%) was also the highest.
Figure 7 displayed the 15N distribution in soil layers. The 15N labeled at 10 cm depth obviously moved downward with irrigation water, while those labeled at 50 cm depth mostly remained in situ or moved upward, only small amount distributed below 60 cm. The peak value of 15N under APRI was found in shallower soil layer relative to CI, and this was particularly evident at TES. APRI10 reserved 36.1 and 29.8% of the applied 15N in its labeled layer, respectively at TES and TLS, while CI10 reserved only 20.3% and 15.2%. The similar advantage of APRI in reserving 15N in corresponding labeled layer could also be obtained through comparisons between APRI50 and CI50. Besides, it was found that TES and TLS differed little on the 15N distribution in soil layers.
Figure 7. The accumulation amount of 15N in soil layers as influenced by different treatments (APRI10 and APRI50 represent that 15N is labeled at 10 and 50 cm soil depths respectively under alternate partial root-zone irrigation, CI10, and CI50 represent that 15N is labeled at 10 and 50 cm soil depths respectively under conventional irrigation. Here, the accumulation amount of 15N refers to the amount in each soil column).
The amount of 15N recovery for the treatments were ranged from 339.9 to 432.5 mg per soil column, with recovery rate of 72.9–92.8% (Table 9). Correspondingly, the 15N loss were from 33.8 to 126.4 mg per soil column, and the loss rate were 7.2–27.1%. The amount of 15N recovery in APRI was significantly (p < 0.05) higher than in CI when the labeled depth was the same. APRI10 increased the amount of 15N recovery by 10.8% (average of TES and TLS) compared to CI10, and APRI50 increased it by 11.7% compared to CI50. Under the same irrigation pattern, the recovery of 15N with 10 cm labeled depth were significantly (p < 0.05) higher than with 50 cm depth (except CI10 and CI50). Among the different treatments, APRI10 at TES had the highest recovery rate (92.8%) and the lowest loss rate (7.2%) of 15N. On the contrary, the lowest recovery rate (72.9%) and the highest loss rate (27.1%) were in CI50, at TLS.
The 4-year case study showed that a 33.3% decrease in irrigation amount led to a 11.0–15.1 kg/ha more recovery of 15N (15N was originated from double labeled NH4NO3 with abundance of 10.3%) (Hou et al., 2017). In our study, APRI significantly (p < 0.05) increased the 15N recovery by 10.2–13.2% but decreased the 15N loss by 35.4–54.6% in comparison to CI. The reason might be that APRI could reduce 15N leaching, promote 15N to move upwards, therefore increase the opportunity for root to capture the 15N (Wang, 2014).
Collectively, our results suggested that APRI improved WUE and fruit quality, and noticeably increased the 15N recovery, indicating a great potential in reusing the residual fertilizer nitrogen in the soil. In practice, to apply N fertilizer to 50 cm below the ground is difficult, in relation to this, fertigation by using APRI and CI could be an option to evaluate the synergistic effect of spatial and temporal water and N supply to the root zone. This study has three aspects that needed to be noticed or improved: (1) During the experiment process, we have not observed the soil water content continuously using the buried type moisture sensor, this might limit the mechanism analysis of plant water use under APRI. (2) Since we used K15NO3 as labeling material, the K that added into the soil column might influence the plant growth, yield, particularly the quality. The Na15NO3 fertilizer, could be considered in similar experiment. (3) In future study, the height of the plastic film used to separate the root system in each soil column should be deeper, in order to achieve better partial root zone drying effect in the deeper soil layers.
Compared to CI, APRI did not significantly impact the growth and biomass accumulation in the aboveground part of tomato, but it enhanced the root, reflecting by greater length density and more dry mass. APRI produced marginally lower yields, while saved 34.9% of the total irrigation water, and gave a 37.6–49.9% higher WUE relative to CI. In addition, APRI improved the fruit quality, mainly through increasing the contents of soluble solid (by 12.8–21.6% over that of CI) and vitamin C (2.8–12.7%), and the ratio of sugar/acid (3.5–8.5%). The 15NUE of tomato in APRI was higher than that in CI, which was more evident when the 15N was labeled at the soil depth of 50 cm. The significant (p < 0.05) 15N recovery increase of 10.2–13.2% and 15N loss decrease of 35.4–54.6% were found for APRI compared to CI. Surprisingly, in our study, different transplant time showed little differences in growth, yield and quality of tomato, except that transplanting in late summer caused a delay in the first harvest of tomato and increased the volume of single fruit significantly (p < 0.05). We concluded that an enlarged root system and a high 15N bioavailability under APRI might have contributed to the higher 15NUE of tomato.
In this study, author MH wrote the main manuscript. QJ gave the valuable guidance for the experiments. XL, JL, and HZ analyzed the data. In addition, YG helped to modify the manuscript.
The authors declare that the research was conducted in the absence of any commercial or financial relationships that could be construed as a potential conflict of interest.
This work was financed by Natural Science Foundation of Fujian Province (2016J05069), Young Talent Project of Horticultural College of Fujian A&F University (61201400705), Postdoctoral Funds of Fujian A&F University (132300198), Key Project on Poor Supporting of Fujian Province (K1516035A) and New Technology Project on Irrigation and Drainage of Tiaozini (SF-201724).
Abrisqueta, J. M., Mounzer, O., Alvarez, S., Conejero, W., Garcia-Orellana, Y., Tapia, L. M., et al. (2008). Root dynamics of peach trees submitted to partial rootzone drying and continuous deficit irrigation. Agric. Water Manage. 95, 959–967. doi: 10.1016/j.agwat.2008.03.003
Badr, M. A., Abou-Hussein, S. D., and El-Tohamy, W. A. (2016). Tomato yield, nitrogen uptake and water use efficiency as affected by planting geometry and level of nitrogen in an arid region. Agric. Water Manage. 169, 90–97. doi: 10.1016/j.agwat.2016.02.012
Burnett, S. E., Mattson, N. S., and Williams, K. A. (2016). Substrates and fertilizers for organic container production of herbs, vegetables, and herbaceous ornamental plants grown in greenhouses in the United States. Sci. Hortic. 208, 111–119. doi: 10.1016/j.scienta.2016.01.001
Casa, R., and Rouphael, Y. (2014). Effects of partial root-zone drying irrigation on yield, fruit quality, and water-use efficiency in processing tomato. J. Hortic. Sci. Biotechnol. 89, 389–396. doi: 10.1080/14620316.2014.11513097
Centritto, M., Wahbi, S., Serraj, R., and Chaves, M. M. (2005). Effects of partial rootzone drying (PRD) on adult olive tree (Olea europaea) in field conditions under arid climate II. Photosynthetic responses. Agric. Ecosyst. Environ. 106, 303–311. doi: 10.1016/j.agee.2004.10.016
Djurović, N., Ćosić, M., Stričević, R., Savić, S., and Domazet, M. (2016). Effect of irrigation regime and application of kaolin on yield, quality and water use efficiency of tomato. Sci. Hortic. 201, 271–278. doi: 10.1016/j.scienta.2016.02.017
Dodd, I. C. (2009). Rhizosphere manipulations to maximize ‘crop per drop’ during deficit irrigation. J. Exp. Bot. 60, 2454–2459. doi: 10.1093/jxb/erp192
Dorais, M., Costa, J. M., Heuvelink, E., and Botden, N. (2005). Greenhouse Horticulture in China: Situation and Prospects, Horticultural Production Chains Group, Wageningen University, Wageningen, The Netherlands, 2004. Sci. Hortic. 104, 121–124. doi: 10.1016/j.scienta.2004.11.002
Du, H. (2007). Nitrogen Use, Soil Nutrient, Salt Accumulation of Vegetables in Protected Agriculture. Doctor. thesis, Chinese Academy of Sciences.
Du, S., Kang, S., Li, F., and Du, T. (2017). Water use efficiency is improved by alternate partial root-zone irrigation of apple in arid northwest China. Agric. Water Manag. 179, 184–192. doi: 10.1016/j.agwat.2016.05.011
Du, T., Kang, S., Zhang, J., Li, F., and Yan, B. (2008). Water use efficiency and fruit quality of table grape under alternate partial root-zone drip irrigation. Agric. Water Manage. 95, 659–668. doi: 10.1016/j.agwat.2008.01.017
Du, T. S., Kang, S. Z., Zhang, J. H., and Davies, W. J. (2015). Deficit irrigation and sustainable water-resource strategies in agriculture for China's food security. J. Exp. Bot. 66, 2253–2269. doi: 10.1093/jxb/erv034
Du, T. S., Kang, S. Z., Zhang, J. H., Li, F. S., and Hu, X. T. (2006). Yield and physiological responses of cotton to partial root-zone irrigation in the oasis field of northwest China. Agric. Water Manage. 84, 41–52. doi: 10.1016/j.agwat.2006.01.010
Elad, Y., Israeli, L., Fogel, M., David, R. D., Kenigsbuch, D., Chalupowicz, D., et al. (2014). Conditions influencing the development of sweet basil grey mould and cultural measures for disease management. Crop Protect. 64, 67–77. doi: 10.1016/j.cropro.2014.06.006
Hou, M., and Shao, X. (2016). Optimization of irrigation-drainage scheme for tomato crop based on multi-index analysis and projection pursuit model. Zemdirbyste-Agric. 103, 221–228. doi: 10.13080/z-a.2016.103.029
Hou, M., Shao, X., Jin, Q., and Gao, X. (2017). A 15N tracing technique-based analysis of the fate of fertilizer N: a 4-year case study in eastern China. Arch. Agron. Soil Sci. 63, 74–83. doi: 10.1080/03650340.2016.1274825
Ju, X. T., Kou, C. L., Zhang, F. S., and Christie, P. (2006). Nitrogen balance and groundwater nitrate contamination: comparison among three intensive cropping systems on the North China plain. Environ. Pollut. 143, 117–125. doi: 10.1016/j.envpol.2005.11.005
Kanechi, M., Hikosaka, Y., and Uno, Y. (2013). Application of sugarbeet pure and crude extracts containing glycinebetaine affects root growth, yield, and photosynthesis of tomato grown during summer. Sci. Hortic. 152, 9–15. doi: 10.1016/j.scienta.2013.01.003
Kanthle, A. K., Lenka, N. K., Lenka, S., and Tedia, K. (2016). Biochar impact on nitrate leaching as influenced by native soil organic carbon in an Inceptisol of central India. Soil Tillage Res. 157, 65–72. doi: 10.1016/j.still.2015.11.009
Kinoshita, T., Yamazaki, H., Inamoto, K., and Yamazaki, H. (2016). Analysis of yield components and dry matter production in a simplified soilless tomato culture system by using controlled-release fertilizers during summer–winter greenhouse production. Sci. Hortic. 202, 17–24. doi: 10.1016/j.scienta.2016.02.019
Kirda, C., Cetin, M., Dasgan, Y., Topcu, S., Kaman, H., Ekici, B., et al. (2004). Yield response of greenhouse grown tomato to partial root drying and conventional deficit irrigation. Agric. Water Manage. 69, 191–201. doi: 10.1016/j.agwat.2004.04.008
Kläring, H.-P., Klopotek, Y., Krumbein, A., and Schwarz, D. (2015). The effect of reducing the heating set point on the photosynthesis, growth, yield and fruit quality in greenhouse tomato production. Agric. Forest Meteorol. 214–215, 178–188. doi: 10.1016/j.agrformet.2015.08.250
Korthals Altes, W. K., and van Rij, E. (2013). Planning the horticultural sector: managing greenhouse sprawl in the Netherlands. Land Use Policy 31, 486–497. doi: 10.1016/j.landusepol.2012.08.012
Lenka, S., Singh, A. K., and Lenka, N. K. (2013). Soil water and nitrogen interaction effect on residual soil nitrate and crop nitrogen recovery under maize–wheat cropping system in the semi-arid region of northern India. Agric. Ecosyst. Environ. 179, 108–115. doi: 10.1016/j.agee.2013.08.001
Li, C., Sun, J., and Zhou, X. (2011). Root morphology characteristics under alternate furrow irrigation. Acta Ecol. Sin. 31, 3956–3963.
Liang, B., Zhao, W., Yang, X., and Zhou, J. (2013). Fate of nitrogen-15 as influenced by soil and nutrient management history in a 19-year wheat–maize experiment. Field Crops Res. 144, 126–134. doi: 10.1016/j.fcr.2012.12.007
Liu, C., Rubæk, G. H., Liu, F., and Andersen, M. N. (2015). Effect of partial root zone drying and deficit irrigation on nitrogen and phosphorus uptake in potato. Agric. Water Manage. 159, 66–76. doi: 10.1016/j.agwat.2015.05.021
Lv, J. (2013). Effects of irrigation lower limit on growth, utilization efficiency of water and quality of tomato. J. Gansu Agric. Univ. 2, 37–41.
Mahajan, G., and Singh, K. G. (2006). Response of Greenhouse tomato to irrigation and fertigation. Agric. Water Manage. 84, 202–206. doi: 10.1016/j.agwat.2006.03.003
Maomao, H., Xiaohou, S., and Yaming, Z. (2014). Effects of different regulatory methods on improvement of greenhouse saline soils, tomato quality, and yield. Sci. World J. 2014:953675. doi: 10.1155/2014/953675
Mingo, D. M., Theobald, J. C., Bacon, M. A., Davies, W. J., and Dodd, I. C. (2004). Biomass allocation in tomato (Lycopersicon esculentum) plants grown under partial rootzone drying: enhancement of root growth. Funct. Plant Biol. 31, 971–978. doi: 10.1071/FP04020
Qin, F. F., Xu, H. L., and Ma, G. (2008). Garlic sprouts grown indoors at kitchen sites. Med. Aromatic Plant Sci. Biotechnol. 2, 117–122.
Sarker, K. K., Akanda, M. A. R., Biswas, S. K., Roy, D. K., Khatun, A., and Goffar, M. A. (2016). Field performance of alternate wetting and drying furrow irrigation on tomato crop growth, yield, water use efficiency, quality and profitability. J. Integr. Agric. 15, 2380–2392. doi: 10.1016/S2095-3119(16)61370-9
Sepaskhah, A. R., and Tafteh, A. (2012). Yield and nitrogen leaching in rapeseed field under different nitrogen rates and water saving irrigation. Agric. Water Manage. 112, 55–62. doi: 10.1016/j.agwat.2012.06.005
Sezen, S. M., Yazar, A., and Tekin, S. (2011). Effects of partial root zone drying and deficit irrigation on yield and oil quality of sunflower in a mediterranean environment. Irrigat. Drainage 60, 499–508. doi: 10.1002/ird.607
Skinner, R. H., Hanson, J. D., and Benjamin, J. G. (1999). Nitrogen uptake and partitioning under alternate- and every-furrow irrigation. Plant Soil 210, 21–30. doi: 10.1023/A:1004695301778
Stefanelli, D., Goodwin, I., and Jones, R. (2010). Minimal nitrogen and water use in horticulture: effects on quality and content of selected nutrients. Food Res. Int. 43, 1833–1843. doi: 10.1016/j.foodres.2010.04.022
Sun, Y., Yan, F., and Liu, F. (2013). Drying/rewetting cycles of the soil under alternate partial root-zone drying irrigation reduce carbon and nitrogen retention in the soil–plant systems of potato. Agric. Water Manage. 128, 85–91. doi: 10.1016/j.agwat.2013.06.015
Tang, K., Gong, C., and Wang, D. (2016). Reduction potential, shadow prices, and pollution costs of agricultural pollutants in China. Sci. Total Environ. 541, 42–50. doi: 10.1016/j.scitotenv.2015.09.013
Teitel, M., and Zhao, Y. (1992). International Society for Horticultural Science, ISHS. Sci. Hortic. 49, 341–349.
Tijskens, L. M. M., et al. (2016). From fruitlet to harvest: modelling and predicting size and its distributions for tomato, apple and pepper fruit. Sci. Hortic. 204, 54–64. doi: 10.1016/j.scienta.2016.03.036
Topak, R., Acar, B., Uyanöz, R., and Ceyhan, E. (2016). Performance of partial root-zone drip irrigation for sugar beet production in a semi-arid area. Agric. Water Manage. 176, 180–190. doi: 10.1016/j.agwat.2016.06.004
Wallis, K. J., Candela, L., Mateos, R. M., and Tamoh, K. (2011). Simulation of nitrate leaching under potato crops in a Mediterranean area. Influence of frost prevention irrigation on nitrogen transport. Agric. Water Manage. 98, 1629–1640. doi: 10.1016/j.agwat.2011.06.001
Wang, C., Zhu, P., Shu, L., Zhu, J., Yu, H., Zhan, Y., et al. (2014). Effects of alternate partial root-zone irrigation and nitrogen forms on utilization and movement of nitrate in soil. Nongye Gongcheng Xuebao/Trans. Chin. Soc. Agric. Eng. 30, 92–101.
Wang, F., Kang, S., Du, T., Li, F., and Qiu, R. (2011). Determination of comprehensive quality index for tomato and its response to different irrigation treatments. Agric. Water Manage. 98, 1228–1238. doi: 10.1016/j.agwat.2011.03.004
Wang, J., Kang, S., Li, F., Zhang, F., Li, Z., and Zhang, J. (2008). Effects of alternate partial root-zone irrigation on soil microorganism and maize growth. Plant Soil 302, 45–52. doi: 10.1007/s11104-007-9453-8
Wang, X., Shi, Y., Guo, Z., Zhang, Y., and Yu, Z. (2015). Water use and soil nitrate nitrogen changes under supplemental irrigation with nitrogen application rate in wheat field. Field Crops Res. 183, 117–125. doi: 10.1016/j.fcr.2015.07.021
Wang, Y., Liu, F., de Neergaard, A., Jensen, L. S., Luxhoi, J., and Jensen, C. R. (2010). Alternate partial root-zone irrigation induced dry/wet cycles of soils stimulate N mineralization and improve N nutrition in tomatoes. Plant Soil 337, 167–177. doi: 10.1007/s11104-010-0513-0
Wang, Z. H., Miao, Y. F., and Li, S. X. (2015). Effect of ammonium and nitrate nitrogen fertilizers on wheat yield in relation to accumulated nitrate at different depths of soil in drylands of China. Field Crops Res. 183, 211–224. doi: 10.1016/j.fcr.2015.07.019
Wang, Z., Liu, F., Kang, S., and Jensen, C. R. (2012). Alternate partial root-zone drying irrigation improves nitrogen nutrition in maize (Zea mays L.) leaves. Environ. Exp. Bot. 75, 36–40. doi: 10.1016/j.envexpbot.2011.08.015
Wei, Z., Du, T., Zhang, J., Shujun, X., Paul, J. C., and Davies, W. J. (2016). Carbon isotope discrimination shows a higher water use efficiency under alternate partial root-zone irrigation of field-grown tomato. Agric. Water Manage. 165, 33–43. doi: 10.1016/j.agwat.2015.11.009
Yang, L., Qu, H., Zhang, Y., and Li, F. (2012). Effects of partial root-zone irrigation on physiology, fruit yield and quality and water use efficiency of tomato under different calcium levels. Agric. Water Manage. 104, 89–94. doi: 10.1016/j.agwat.2011.12.001
Zegbe, J. A., Behboudiana, M. H., and Clothier, B. E. (2004). Partial rootzone drying is a feasible option for irrigating processing tomatoes. Agric. Water Manage. 68, 195–206. doi: 10.1016/j.agwat.2004.04.002
Keywords: Solanum lycopersicum L, partial root-zone irrigation, water use efficiency, N recovery, N loss, quality, yield
Citation: Hou M, Jin Q, Lu X, Li J, Zhong H and Gao Y (2017) Growth, Water Use, and Nitrate-15N Uptake of Greenhouse Tomato as Influenced by Different Irrigation Patterns, 15N Labeled Depths, and Transplant Times. Front. Plant Sci. 8:666. doi: 10.3389/fpls.2017.00666
Received: 08 January 2017; Accepted: 11 April 2017;
Published: 02 May 2017.
Edited by:
Michel Edmond Ghanem, International Center for Agricultural Research in the Dry Areas, MoroccoReviewed by:
Fulai Liu, University of Copenhagen, DenmarkCopyright © 2017 Hou, Jin, Lu, Li, Zhong and Gao. This is an open-access article distributed under the terms of the Creative Commons Attribution License (CC BY). The use, distribution or reproduction in other forums is permitted, provided the original author(s) or licensor are credited and that the original publication in this journal is cited, in accordance with accepted academic practice. No use, distribution or reproduction is permitted which does not comply with these terms.
*Correspondence: Maomao Hou, bmpob3VtYW9tYW9AMTI2LmNvbQ==
Disclaimer: All claims expressed in this article are solely those of the authors and do not necessarily represent those of their affiliated organizations, or those of the publisher, the editors and the reviewers. Any product that may be evaluated in this article or claim that may be made by its manufacturer is not guaranteed or endorsed by the publisher.
Research integrity at Frontiers
Learn more about the work of our research integrity team to safeguard the quality of each article we publish.