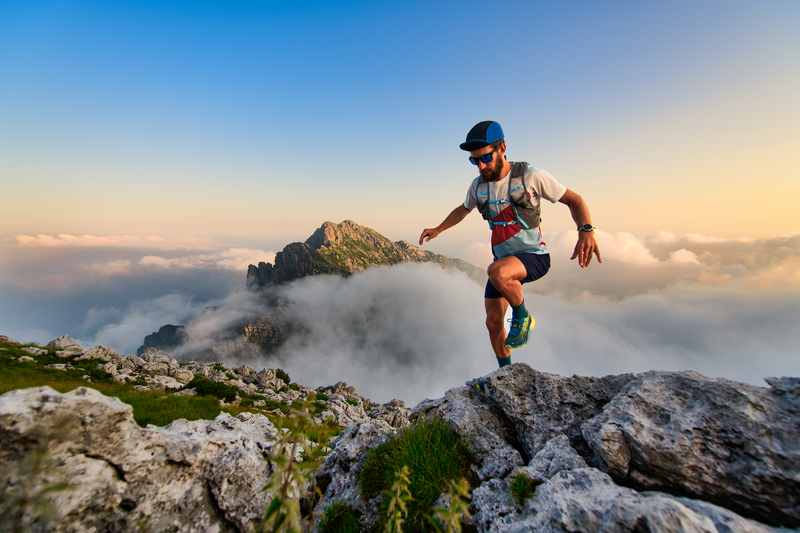
95% of researchers rate our articles as excellent or good
Learn more about the work of our research integrity team to safeguard the quality of each article we publish.
Find out more
ORIGINAL RESEARCH article
Front. Plant Sci. , 17 March 2017
Sec. Plant Physiology
Volume 8 - 2017 | https://doi.org/10.3389/fpls.2017.00371
This article is part of the Research Topic Phytohormones and the Regulation of Stress Tolerance in Plants: Current Status and Future Directions View all 28 articles
Cytokinins (CTKs) regulate panicle size and mediate heat tolerance in crops. To investigate the effect of high temperature on panicle CTK expression and the role of such expression in panicle differentiation in rice, four rice varieties (Nagina22, N22; Huanghuazhan, HHZ; Liangyoupeijiu, LYPJ; and Shanyou63, SY63) were grown under normal conditions and subjected to three high temperature treatments and one control treatment in temperature-controlled greenhouses for 15 days during the early reproductive stage. The high temperature treatments significantly reduced panicle CTK abundance in heat-susceptible LYPJ, HHZ, and N22 varieties, which showed fewer spikelets per panicle in comparison with control plants. Exogenous 6-benzylaminopurine application mitigated the effect of heat injury on the number of spikelets per panicle. The high temperature treatments significantly decreased the xylem sap flow rate and CTK transportation rate, but enhanced cytokinin oxidase/dehydrogenase (CKX) activity in heat-susceptible varieties. In comparison with the heat-susceptible varieties, heat-tolerant variety SY63 showed less reduction in panicle CTK abundance, an enhanced xylem sap flow rate, an improved CTK transport rate, and stable CKX activity under the high temperature treatments. Enzymes involved in CTK synthesis (isopentenyltransferase, LONELY GUY, and cytochrome P450 monooxygenase) were inhibited by the high temperature treatments. Heat-induced changes in CTK transportation from root to shoot through xylem sap flow and panicle CTK degradation via CKX were closely associated with the effects of heat on panicle CTK abundance and panicle size. Heat-tolerant variety SY63 showed stable panicle size under the high temperature treatments because of enhanced transport of root-derived CTKs and stable panicle CKX activity. Our results provide insight into rice heat tolerance that will facilitate the development of rice varieties with tolerance to high temperature.
The global mean surface temperature increased rapidly and considerably during the 20th century, and a further increase of 0.3–4.8°C is predicted by the end of the 21st century (Pachauri et al., 2014). Notably, nighttime temperature has increased more rapidly than has daytime temperature (Peng et al., 2004; Elagib, 2010). High temperature extremes and warmer nights are likely to become more frequent and intense in the near future (Mika, 2013; Wang et al., 2014).
Rice plants are highly susceptible to high temperature stress, especially during the reproductive stage (Moldenhauer et al., 2001; Jagadish et al., 2015), during which high temperature may severely reduce grain yield. An increase of 1–4°C reduced rice grain yield by 0–49%; grain yield decreased 14% for every 1°C increase in temperature (Singh et al., 2009). Another study showed that an increase of 1°C in nighttime temperature reduced rice grain yield by 10% (Peng et al., 2004). Heat-induced yield reduction is largely attributed to adverse effects on yield components (Jagadish et al., 2015).
During the early reproductive phase of rice, which includes the processes of panicle initiation and development, high temperature events, including relatively warm nights, reduced the number of spikelets (Wei et al., 2010; Wu et al., 2016). During the middle and late reproductive phases, during which heading and grain filling occur, high temperature events reduce the grain filling rate and grain weight in rice (Jagadish et al., 2015). Several studies have assessed the agronomic, physiological, and molecular aspects of the negative impact of heat stress on rice production, especially at the flowering and grain filling stages (Shi et al., 2013; Jagadish et al., 2015). However, understanding of the physiological aspects of the impact of high temperature on panicle differentiation and spikelet formation during the early reproductive phase is limited (Wang et al., 2015).
In higher plants, cytokinins (CTKs) regulate numerous biological processes, including shoot growth and development, differentiation, and responses to environmental stresses (Ha et al., 2012; Zwack and Rashotte, 2015). In rice, CTKs also regulate the number of spikelets (Kyozuka, 2007). Rice varieties with small panicles had correspondingly low panicle CTK abundance, but exogenous CTK application to plants gradually increased the number of spikelets (Ashikari et al., 2005; Ding et al., 2014). These results show that CTKs play crucial roles in panicle formation and spikelet differentiation in rice.
Cytokinin accumulation in the aerial organs of plants is determined by the rate of importation of root-synthesized CTKs, local CTK synthesis, and local CTK catabolism (Kudo et al., 2010). Root-derived CTKs are the main source of CTKs for aerial organs (Aloni et al., 2005), in which most CTKs are derived from the roots and transported to shoot via xylem sap (Yong et al., 2014). In Betula pubescens, CTK abundance in xylem sap was positively correlated with the growth rate of shoot and number of branches (Rinne and Saarelainen, 1994). CTK synthesis involves several enzymes, but isopentenylation of adenosine phosphate by isopentenyltransferases (IPT) to produce N6-(Δ2-isopentenyl) adenosine (iP) phosphate is the key step in CTK biosynthesis (Miyawaki et al., 2006). Trans-hydroxylation of the side-chain of iP-riboside to form trans-zeatin (tZ) riboside is catalyzed by cytochrome P450 mono-oxygenase CYP735A (Kiba et al., 2013). Additionally, LONELY GUY (LOG) proteins with phosphoribohydrolase activity are involved in the conversion of riboside 5′-monophosphate CTKs with low activity to high-activity forms such as iP (Kurakawa et al., 2007; Tokunaga et al., 2012). Degradation of CTKs is primarily catalyzed by cytokinin oxidase/dehydrogenase (CKX) (Kudo et al., 2010).
Suppressed CTK degradation and enhanced CTK biosynthesis contribute to local CTK accumulation in plant organs (Kudo et al., 2010). CTK metabolism influences panicle size in rice; relatively low CKX activity was associated with reduced panicle CTK degradation and a greater number of spikelets per panicle (Ashikari et al., 2005). And, others have reported that enhanced CTK biosynthesis is associated with large panicle size in rice. For example, expression levels of IPT and LOG, which are involved in local CTK synthesis in shoot meristem, were directly associated with the number of panicle spikelets (Kurakawa et al., 2007; Ding et al., 2014). In Arabidopsis, shoot development and growth were promoted by overexpression of CYP735A and retarded in loss-of-function CYP735A mutants (Kiba et al., 2013). However, few studies have simultaneously evaluated enzymes involved in CTK synthesis and catabolism, especially in rice, and it is not yet clear which processes and enzymes involved in CTK metabolism influence panicle CTK accumulation and panicle differentiation.
Cytokinins mediate plant responses to abiotic stresses, including heat stress (Ha et al., 2012). In rice, Arabidopsis and passion fruit, reduced CTK abundance in shoots in response to heat caused floret abortion, whereas exogenous CTK application to shoots mitigated the effects of heat injury on branches and florets (Sobol et al., 2014; Wu et al., 2016). CTK transportation via xylem sap is involved in heat stress tolerance (Ha et al., 2012; Zwack and Rashotte, 2015). In creeping bentgrass, application of zeatin riboside (ZR) to the root zone increased the abundance of shoot CTKs and alleviated heat stress injury following exposure to high soil temperature and high air temperature (Liu et al., 2002). However, Udompraset et al. (1995) found that root tZR was not involved in the development of heat tolerance in Phaseolus vulgaris. These findings show that the relationship between CTK transportation from root to shoot and heat tolerance is not well understood.
Changes in CTK metabolism are thought to be involved in adaptation by plants to various environmental stresses. Enzymes involved in CTK synthesis play roles in stress responses in several plant species. IPT, the key enzyme in the process of CTK synthesis, is involved in adaption to heat, drought, osmotic stress, and salt stress in maize, peanut, and Arabidopsis (Vyroubalová et al., 2009; Qin et al., 2011; Skalák et al., 2016). In rice, LOG expression is altered by various abiotic stress conditions, including heat stress, cold stress, drought, and salinity (Tripathi et al., 2012). CYP735A expression in rice was altered by exposure to cold and dehydration (Maruyama et al., 2014). Regulation of CTK abundance by CKX occurs in response to heat, cold, drought, and salinity in rice, maize, tobacco, and pea, respectively (Vaseva-Gemisheva et al., 2004; Vaseva et al., 2009; Vyroubalová et al., 2009; Tripathi et al., 2012; Lubovská et al., 2014). Accumulation of CTKs as a result of impaired degradation, enhanced local biosynthesis, and/or enhanced CTK transportation from roots, is favorable for adaption to abiotic stress (Ha et al., 2012). Therefore, assessing the effects of heat on CTK transportation from root to shoot via xylem sap and the activity levels of enzymes involves in CTK metabolism, such as IPT, LOG, CYP735A, and CKX, should illuminate the mechanisms underlying phytohormonal regulation of heat tolerance in rice.
Rice varieties display wide genotypic variation in the physiological response to heat stress (Tao et al., 2009; Shi et al., 2013). In this study, we investigated genotypic variation in physiological processes associated with CTK homeostasis in rice plants exposed to high temperature, as well as the relationship between these processes and spikelet number, with the goal of revealing the mechanisms underlying hormonal regulation of panicle size under heat stress during the early reproductive phase.
Pot experiments were conducted during the rice growth season of 2013 at Huazhong Agricultural University, Wuhan, China (30°29′ N, 114°22′ E). Four varieties were used in this study: N22, HHZ, LYPJ, and SY63. The methods for crop husbandry, temperature treatments, and phytohormone determination were described as previous study (Wu et al., 2016).
After breaking dormancy at 50°C for 5 days, seeds were sown in plastic seeding trays with loam soil. Four three-leaf seedlings were transplanted into a 14 L plastic pot (28.5 cm height × 30 cm top diameter × 25 cm bottom circumference) containing a mixture of 17 kg soil (loam:sand, 2:1) and 12.5 g compound fertilizer (N:P2O5:K2O, 16%:16%:16%). Seedlings were thinned to three plants per pot 8 days after transplanting, and the main tillers were tagged. A total of 1.0 g urea was topdressed per pot 10 days after transplanting. The plants were flooded with water staying approximately 2 cm above soil surface from sowing to maturity. Each pot was manually rotated by 90° clockwise every 7 days to avoid positional effects. Pests, diseases, birds, and weeds were intensively controlled.
The rice plants were randomly arranged with four replications. All plants were carefully cultivated under natural ambient conditions, after which they were moved to four temperature-controlled greenhouses at the start of panicle initiation (panicle emergence was observed visually). The greenhouses were equipped with a wetting machine, an air conditioner, two ventilators, and two sensors for monitoring relative humidity (RH) and air temperature.
The four temperature treatments included a high nighttime temperature treatment (HNT) that imposed high temperature from 19.00 to 07.00 h, high daytime temperature treatment (HDT) that imposed high temperature from 07.00 to 19.00 h, high daytime plus nighttime temperature treatment (ADT) that imposed high temperature during the entire day, and control (CK) treatment that imposed a favorable temperature for rice plant growth during the entire day. RH was set at 80%. The air temperature and RH in the greenhouse were controlled by a central auto-controller (Auto-Greenhouse Monitoring and Data Management System, Version 3.00, Auto, China). Air temperature and RH% were recorded 5 cm above the rice canopy using a standalone sensor (HOBO, H08-003-02, Onset Computer Corporation, Bourne, MA, USA). The mean nighttime and daytime temperatures were 27.2 and 31.9°C under the CK treatment. The mean daytime temperature was 36.1°C under the HDT treatment (4.2°C higher than that of the CK treatment). The mean nighttime temperature was 31.9°C under HNT treatment (4.7°C higher than that of the CK treatment). The mean nighttime and daytime temperatures under the ADT treatment were 31.5 and 38.3°C, respectively (4.3 and 6.4°C higher than those of the CK treatment, respectively) (Figure 1).
FIGURE 1. Dynamic temperature records for the three high-temperature treatments and one control treatment.
The plants were exposed to the high temperature treatments for 15 days, after which the plants were removed and grown continuously under normal conditions until maturity, at which point panicle length was approximately 1.5–2.0 cm.
The solution of BAP (60 mg L-1), a synthetic CTK, was prepared by dissolving 60 mg BAP (Sigma-Aldrich, USA) in 1 mL of 1% (w/v) NaOH solution, following by dilution to a final volume of 1 L with double-distilled H2O. Two drops of 0.01% (v/v) Tween 20 was added as a surfactant, after which the solution was mixed thoroughly. The BAP solution was sprayed onto the stems of LYPJ (heat-sensitive) and SY63 (heat-tolerant) varieties under the ADT treatment (20 mL per plant per application). The BAP solution was applied twice: 1 day before the high temperature treatments and on the second day after the high temperature treatments.
To determine the number of spikelets per panicle and grain yield, three panicles of three main tillers were harvested at maturity from three plants grown in three pots for each replication. All spikelets were threshed from the panicles manually, and then collected to determine grain yield and yield components. Grain yield and other yield components were also calculated. The number of spikelets per panicle was referred to as the number of spikelets in the main tiller.
For each replication, three young panicles of the main tillers were collected from three plants grown in three pots at the last day of exposure to the high temperature treatments. The panicles were frozen in liquid nitrogen and stored at -80°C. Next, xylem sap was collected from the same three plants during the night (19.00–07.00 h) as described by Arai-Sanoh et al. (2010). The number of tillers per plant was counted, after which the plants were cut approximately 8 cm above the soil level. Dead leaf sheathes in stubbles were cleared artificially, and the first droplet of exudation was wiped to avoid contamination. Polyethylene bags containing cotton wool (6–7 g) were attached to the cut ends and fixed with rubber bands, and collected root xylem sap for 12 h. The difference in weight of the cotton wool was considered as the weight of the collected root exudates. The root xylem sap flow rate (mg tiller-1 h-1) was calculated as the amount of collected sap per tiller divided by 12. Finally, roots were sampled and washed to allow collection of fresh white roots. The collected roots and exudates were stored at -80°C and used for measurements of CTK abundance.
Cytokinins in the roots, xylem sap, and panicles were extracted and purified according to methods reported by Xie and Zhang (2001) and Hoyerová et al. (2006) and quantified according to the methods of Chou et al. (2000) using high performance liquid chromatography (HPLC) with some minor modifications.
For CTKs in the panicles and roots, frozen samples were cut into pieces and mixed completely. Next, 1 g of tissue was ground with 8 mL of cold extraction buffer (methanol:double-distilled H2O:formic acid, 15:4:1). The homogenates were transferred to 10-mL centrifuge tubes, incubated at 4°C for 12 h, and centrifuged at 12,000 × g for 20 min at 4°C, after which supernatants were collected. The pellets were subjected to phytohormone extraction twice as described above (e.g., 8 mL cold extraction buffer and centrifugation). All supernatants were pooled and condensed to 2 mL using a freeze-dryer (ALPHA 1-4 LD plus, Marin Christ, Osterode, Germany), after which 2 mL of petroleum ether was added to extract pigments and phenolics. The extraction was repeated three times. After removing the petroleum ether containing pigments and phenolics, the lower aqueous phase was freeze dried, and 3 mL of sodium acetate (1 mol/L, pH 8.0) was used to resuspend the samples as crude extracts for determination of various CTKs.
For CTK extraction in root xylem sap, 10 mL of xylem sap was collected in a centrifuge tube by extruding the cotton wool in which the xylem sap was collected. The xylem sap was centrifuged at 12,000 × g for 20 min at 4°C, after which 8 mL of the supernatant was collected and freeze-dried. Next, the sample was resuspended in 3 mL of sodium acetate (1 mol/L, pH 8.0). These solutions were designated as the crude extracts for determination of various CTKs.
For further CTK extraction, the crude extract (3 mL) was extracted with 1-butanol (3 mL) three times, after which the upper organic phase (1-butanol) containing CTKs was pooled together for CTK measurement. The lower aqueous phase was pooled, adjusted to pH 3.0, and extracted three times with ethyl acetate. The upper organic phase (ethyl acetate) was pooled as the mixture of IAA, GAs, and ABA.
All collected upper organic phases were pooled together for determining CTKs, IAA, GAs, and ABA. After freeze-drying, the residues were dissolved in 5 mL of methyl alcohol and purified using a C18-SepPak cartridge (Waters Corporation, Milford, MA, USA). The purified samples were freeze-dried and dissolved in 0.8 mL of methyl alcohol for phytohormone determination. The elution procedure, flow rate, column temperature, and detection wavelength for HPLC analysis were optimized. Based the optimized conditions, abundance of CTKs was measured using HPLC equipped with a C18 column (WondaCract ODS-2 C18 column; 4.6 mm × 250 mm, 5 μm) by a multi-step linear gradient elution (45 min) at a flow rate of 1.6 mL min-1. The column temperature was maintained at 45°C. The UV detection wavelength was 269 nm. The solutions for eluting according to the methods of Chou et al. (2000) with modification. The solutions for eluting include methanol (A), double-distilled H2O (B), and 4.5% acetic acid solution (C). The following protocol was used for gradient elution: 0 min, 0% A and 100% B; 17 min, 30% A and 70% B; 18 min, 40% A and 60% B; 22 min, 40% A and 60% B; 24 min, 35% A and 65% B; 25min, 35% A and 65% C; 35min, 100% B; 45min, 100% B.
The calibration standards were mixed in a CTK standard solution containing isopentenyladenine riboside-5’-monophosphate (iPMP), iP, N6-(Δ2-isopentenyl) adenosine riboside (iPA), tZ, and tZR (OlChemIm Ltd., Czechia). The calibration standards were prepared at concentrations of 5.7, 8.5, 11.4, 45.6, 91.1, and 182.3 ng mL-1 for each hormone standard in the mixed standard solution of five compounds. Calibration standard curves were repeated four times, after which a standard curve was calculated for each compound.
To evaluate recovery of extracted CTKs, a 1-g sample of panicle tissue was ground with 150 ng of each CTK standard mentioned above. The percentage recovery of tZ, tZR, iPMP, iP, and iPA was 109.4 ± 5.4, 97.8 ± 4.4, 62.1 ± 2.2, 96.7 ± 4.1, 97.2 ± 5.0%, respectively.
The concentration of each CTK in the roots and panicles was expressed as ng g-1 based on fresh weight, whereas the concentration in xylem sap was expressed as pg mL-1. The transport rate of each CTK via xylem sap (pg tiller-1 hr-1) was calculated by multiplying the xylem sap flow rate by the concentration of the corresponding CTK in xylem sap.
According to the methods described by Zalewski et al. (2010), a 0.5-g sample of young panicles was cut into pieces, powdered with liquid nitrogen using a hand mortar, and extracted with 6 mL of TRIS-HCl buffer (0.2 M, pH 8.0, containing 1 mM phenylmethylsulfonyl fluoride and 0.3% Triton X-100).
All debris was removed by centrifugation at 12,000 × g for 15 min at 4°C. The supernatants were collected and used for determinations of enzyme activity. The soluble protein concentration was evaluated using the method of Bradford (1976), with bovine serum albumin as the standard.
The assay of CKX activity based on iP degradation was performed according to the methods of Frébort et al. (2002) with minor changes. A reaction mixture containing 0.2 mL of the enzyme extract, 0.2 mL iP (0.15 mM), 0.5 mL 2,6-dichlorophentolindophenol (0.5 mM), and 0.2 mL Tris/HCl buffer (75 mM, pH 8.5) was incubated at 37°C for 60 min, after which the reaction was stopped by the addition of 0.3 mL trichloroacetic acid (40%). The mixture was centrifuged at 18,000 × g for 30 min. HPLC analysis was used to quantify iP by measuring absorbance at 269 nm as described previously. CKX activity (nmol mg-1 protein h-1) was defined as the amount of iP (nmol) degraded by 1 mg protein per hour under the selected reaction conditions.
The assay of IPT activity was performed according to the method of Takei et al. (2001a) with minor changes. The enzyme extract (0.2 mL) was incubated in 0.2 mL of the reaction mixture (1 M betaine, 20 mM triethanolamine, 50 mM KCl, 10 mM MgCl2, 1 mM dithiothreitol, 1 mg/mL bovine serum albumin, pH 8.0) with 0.2 mL adenosine monophosphate (1 mM) and 0.3 mL dimethylallylpyrophosphate (340 μM) at 25°C for 2 h, after which the reaction was stopped by the addition of 0.2 mL acetate (10%). When optimizing the conditions for the IPT activity determination, we had set six incubation times, i.e., 20, 30, 50, 60, 90, and 120 min, respectively, and found that the activity of IPT was highest when incubating for 2 h, and the activity did not detected when incubating for 20, 30, 50, 60. The mixture was centrifuged at 18,000 × g for 20 min. The supernatant was subjected to HPLC, and iPMP was quantified by measuring absorbance at 269 nm as described previously. The activity of IPT (nmol mg-1 protein h-1) was defined as the amount of produced iPMP (nmol) per 1 mg protein per hour under the selected reaction conditions.
The assay of LOG activity was performed according to the method of Kurakawa et al. (2007) with minor changes. The enzyme extract (0.2 mL) was incubated in 0.2 mL of the reaction mixture (50 mM Tris-HCl, 1 mM MgCl2, 1 mM dithiothreitol, pH 6.5) with 0.08 mL iPMP (10 mM) at 30°C for 2 h. The reaction was terminated using 0.3 mL of cold acetone. The mixture was stored at -80°C for 30 min and centrifuged at 18,000 × g for 20 min. The supernatant was subjected to HPLC. The content of synthetic iP was quantified by measuring absorbance at 269 nm as described previously. LOG activity (nmol mg-1 protein h-1) was defined as the amount of iP produced per hour per mg protein under the selected reaction conditions.
The assay of CYP735A activity was performed according to the method of Sasaki et al. (2013) with minor changes. The enzyme extract (0.2 mL) was incubated with 0.2 mL of the reaction mixture (100 mM sodium phosphate, 10% sucrose, 3 mM triphosphopyridine nucleotide, 1 mg/mL bovine serum albumin, pH 7.5) and 0.08 mL iPMP (10 mM) at 20°C for 2 h. The reaction was terminated by the addition of 0.2 mL of termination buffer (50 mM CHES-NaOH, 0.5 mM MgCl2, pH 10.0). The mixture was incubated with 0.01 mL of calf-intestine alkaline phosphatase (1 u/μL, Sigma) at 37°C for 40 min and centrifuged at 18,000 × g for 20 min. The supernatant was subjected to HPLC. The content of tZR was quantified by measuring absorbance at 269 nm and comparison with the standard curve for tZR. CYP735A activity (nmol mg-1 protein h-1) was defined as the amount of tZR produced per hour per mg protein under the selected reaction conditions.
In this study, tZ-type and iP-type CTKs were referred to as biologically active CTKs (aCTKs), including tZ, tZR, iPMP, iP, and iPA. Relative values for traits are presented in this study as a means of evaluating the responses of rice plants to high temperature treatments. A relative value was defined as the ratio of the value under a high temperature treatment to that under the control treatment for the same trait in the same variety. A relative value less or more than 1.0 indicated a decrease or increase in a trait under a high temperature treatment compared with that under the control condition, respectively. The absolute values of CTK contents, xylem sap flow rate, enzyme activities are presented as Supplementary Tables S1–S3, respectively. The absolute concentrations of CTKs in young panicle have already presented in our previous paper (Wu et al., 2016), in which we focused the relationship panicle differentiation and panicle CTK contents under high temperature treatments.
The mean of the relative value across the four replicates was used for analysis of variance and determination of significant differences by the least significant difference (LSD) test at P < 0.05 using Statistix 8.0 (Analytical Software, Tallahassee, FL, USA). Regression analysis was used to estimate the relationship among the investigated traits across four varieties and three high temperature treatments (n = 12) using Sigmaplot software (version 12.5; SPSS Inc., Chicago, IL, USA).
The absolute numbers of spikelets per panicle in N22, HHZ, LYPJ, and SY63 were 103, 203, 196, and 127 under control temperature treatment, respectively. As shown in Figure 2, the three high temperature treatments significantly reduced the number of spikelets per panicle in the HHZ and LYPJ varieties, but the largest reductions in the number of spikelets per panicle were observed under the ADT treatment. The number of spikelets per panicle was reduced by 19.9–32.3% in the HHZ variety and 15.6–32.0% in the LYPJ variety under the three high temperature treatments. For the N22 variety, the number of spikelets per panicle was reduced significantly only under the ADT treatment. For the SY63 variety, the number of spikelets per panicle was not affected significantly by the three high temperature treatments. The SY63 variety had more spikelets per panicle in comparison with the LYP9 and HHZ varieties under the three high temperature treatments, as well as more spikelets per panicle in comparison with the N22 variety under the ADT treatment.
FIGURE 2. Relative number of spikelets per panicle of rice varieties under high temperature treatments. Data are presented as mean ± SD (n = 4). Different letters indicate significant differences among varieties under the same temperature treatment by a least significant difference (LSD) test at P < 0.05. Asterisks indicate significant differences when comparing the absolute mean of a trait under a given high temperature treatment with that under the control by LSD test at P < 0.05.
Application of exogenous BAP increased the number of spikelets per panicle by 11% in the LYPJ variety and by 5% in the SY63 variety under the ADT treatment, in comparison with the same varieties under the ADT treatment without BAP application (Figure 2).
In rice panicles, absolute concentrations of tZ+tZR, iPMP+iP+iPA, and aCTKs were 114, 223, and 336 ng/g in N22, 169, 208, and 376 ng/g in HHZ, 184, 257, and 441 ng/g in LYPJ, and 114, 238, and 353 ng/g in SY63 under control temperature treatment, respectively (Supplementary Table S1).
The HNT treatment significantly decreased the abundance of tZ+tZR, iPMP+iP+iPA, and aCTKs, with the exception of tZ+tZR in the N22 and SY63 varieties (Table 1). Similarly, the HDT and ADT treatments significantly reduced the abundance of the tested CTKs, with the exception of tZ+tZR in the SY63 variety. Generally, the largest reductions were found under the ADT treatment; on average across the four varieties, iP-type CTKs, tZ-type CTKs, and aCTKs in the panicles were reduced in abundance under ADT treatment by 57% (17–73%), 25.3% (0–39%), and 45.8% (16–57%), respectively. In general, the abundance of iP-type CTKs was reduced more than was the abundance of tZ-type CTKs. On average, iP-type CTKs and tZ-type CTKs in the panicles were reduced in abundance by 40.4% (15–73%) and 17.0% (0–39%), respectively, across the four varieties and three high temperature treatments (Table 1).
TABLE 1. Relative cytokinin (CTK) concentrations in panicles and roots under high temperature treatments.
Generally, the three high temperature treatments had no effects on panicle tZ+tZR content in the SY63 variety, which showed the highest relative abundance of panicle CTKs among the four tested varieties (Table 1).
The absolute concentrations of tZ+tZR, iPMP+iP+iPA, and aCTKs in roots were 364, 232, and 596 ng/g in N22, 726, 201, and 926 ng/g in HHZ, 748, 253, and 1001 ng/g in LYPJ, and 622, 193, and 815 ng/g in SY63 under control temperature treatment, respectively (Supplementary Table S1).
There was no significant reduction in the abundance of tZ+tZR, iPMP+iP+iPA, or aCTKs in the roots of the N22, HHZ, and SY63 varieties under the three high temperature treatments. However, in the LYPJ variety, the abundance of tZ+tZR, iPMP+iP+iPA, and aCTKs was reduced significantly by the HDT and ADT treatments (Table 1).
The absolute rates of xylem sap flow were 86, 151, 143, and 85 mg/tiller/h in N22, HHZ, LYPJ, and SY63 under control temperature treatment, respectively (Supplementary Table S2).
The high temperature treatments significantly reduced xylem sap flow in the HHZ, LYPJ, and N22 varieties; xylem sap flow was reduced, on average, by 17% (9–23%) in HHZ, 21% (8–27%) in LYPJ, and 19% (8–27%) in the N22 variety across the three high temperature treatments. However, the xylem sap flow of the SY63 variety was significantly increased, on average, by 18% (11–22%) across the three high temperature treatments. The relative xylem sap flow in the SY63 variety was significantly higher than that of the HHZ, LYPJ, and N22 varieties (Table 2).
TABLE 2. Relative CTK concentrations and transport rates of CTKs in xylem sap under high temperature treatments.
The absolute concentrations of tZ+tZR, iPMP+iP+iPA, and aCTKs in xylem sap were 1.30, 0.19, and 1.49 pg/ml in N22, 1.70, 0.26, and 1.96 pg/ml in HHZ, 1.66, 0.26, and 1.92 pg/ml in LYPJ, and 1.56, 0.22, and 1.78 pg/ml in SY63 under control temperature treatment, respectively (Supplementary Table S2).
The HNT treatment did not substantially decrease the abundance of xylem sap CTKs in the four varieties. Similar effects were observed under the HDT treatment, but iP-type CTKs were reduced in abundance in the HHZ and LYPJ varieties. The three temperature treatments had no effects on tZ+tZR; however, the high temperature treatments decreased the abundance of iP-type CTKs in the N22, HHZ, and LYPJ varieties, as well as the abundance of aCTKs in the HHZ and LYPJ varieties. Generally, the three high temperature treatments imposed small effects on CTK concentrations in xylem sap; most of the effects of high temperature were not significant, with the exception of the significant effects of the ADT treatment on the LYPJ, HHZ, and N22 varieties (Table 2 and Supplementary Table S2).
The absolute transport rates of tZ+tZR, iPMP+iP+iPA, and aCTKs were 0.11, 0.02, and 0.13 pg/tiller/h in N22, 0.26, 0.04, and 0.30 pg/tiller/h in HHZ, 0.24, 0.04, and 0.27 pg/tiller/h in LYPJ, and 0.13, 0.02, and 0.15 pg/tiller/h in SY63 under control temperature treatment, respectively (Supplementary Table S2).
The transport rate of CTKs changed in a manner similar to that of xylem sap flow in the four rice varieties (Table 2 and Supplementary Table S2). The transport rates of tZ+tZR, iPMP+iP+iPA, and aCTKs were reduced significantly in the HHZ, LYPJ, and N22 varieties in response to the three high temperature treatments, while these transport rates were increased or not affected by the treatments in the SY63 variety. The relative transport rate of CTKs in xylem sap in the SY63 variety was significantly greater than the corresponding rates in the HHZ, LYPJ, and N22 varieties.
Absolute activity levels of CKX were 22.0, 8.0, 17.9, and 23.8 nmol iP/mg protein/h in N22, HHZ, LYPJ, and SY63 under control temperature, respectively (Supplementary Table S3). The three high temperature treatments significantly increased the activity level of CKX in the HHZ, LYPJ, and N22 varieties; however, the treatments had no substantial effects in the SY63 variety (Table 3 and Supplementary Table S3). Additionally, the relative activity level of CKX in the SY63 variety was significantly lower than that of the HHZ, LYPJ, and N22 varieties (Table 3).
Absolute activity levels of IPT, LOG, and CYP735A were 0.17 nmol iPMP/mg protein/h, 1.63 nmol iP/mg protein/h, and 0.22 nmol tZR/mg protein/h in N22, 0.04, 1.93, and 0.13 in HHZ, 0.11, 1.65, and 0.28 in LYPJ, and 0.09, 1.67, and 0.23 in SY63, respectively (Supplementary Table S3).
Generally, the activity levels of IPT, LOG, and CYP735A were slightly or significantly reduced by HNT in the four tested rice varieties; however, the activity levels of IPT, LOG, and CYP735A were reduced significantly under the HDT and ADT treatments. The relative activity levels of IPT, LOG, and CYP735A under the HNT treatment were higher than their activity levels under the HDT and ADT treatments. Additionally, the SY63 and N22 varieties generally showed high relative activity levels of IPT, LOG, and CYP735A in comparison with those of the HHZ and LYPJ varieties (Table 3).
As shown in Figure 3A, the relative concentrations of tZ-type CTKs, iP-type CTKs, and aCTKs in panicles were positively and significantly correlated with their relative transport rates via xylem sap. It was also observed that concentrations of panicle CTKs were significantly and positively correlated with the xylem sap flow rate (Figure 3B) and CTK concentrations in xylem sap (Figure 3C). Additionally, panicle CTK concentrations showed positive correlations with root CTK concentrations, with the exception of iP-type CTKs (Figure 3D).
FIGURE 3. Regression analysis for the relative transport rate of CTKs (A), relative xylem sap flow rate (B), relative root xylem sap CTK concentration (C), and relative root CTK concentration (D) with relative panicle CTK concentration across four varieties and three high temperature treatments.
Generally, CTK concentrations in xylem sap were not correlated significantly with root CTK concentrations, with the exception of iP-type CTKs (r = 0.77, P < 0.01 for tZ-type; r = 0.74, P < 0.01 for iP-type; r = 0.80, P < 0.01 for aCTKs, n = 12). The CTK transport rate via xylem sap was poorly correlated with root CTK concentrations, with the exception of iP-type CTKs (r = 0.56, P = 0.06 for tZ-type; r = 0.51, P = 0.09 for iP-type; r = 0.59, P < 0.05 for aCTKs, n = 12).
The relative contents of tZ-type CTKs, iP-type CTKs, and aCTKs in panicles were significantly and negatively correlated with the relative CKX activity level in panicles (r = -0.81 for tZ-type, -0.68 for iP-type, and -0.75 for aCTKs) (Figure 4A). The relative contents of iP-type CTKs and aCTKs were significantly and positively correlated with the relative activities of IPT (r = 0.63 for iP-type and 0.62 for aCTKs, Figure 4B), and CYP735A (r = 0.57 for iP-type, and 0.57 for aCTKs, Figure 4D); however, tZ-type CTK abundance was poorly correlated with the activity levels of IPT, LOG, and CYP735A.
FIGURE 4. Regression analysis for the relative activity levels of CKX (A), IPT (B), LOG (C), and CYP735A (D) with the relative concentration of panicle CTKs across four varieties and three high temperature treatments.
The relative number of spikelets per panicle was positively and significantly correlated with the relative concentrations of tZ-type CTKs (r = 0.93), iP-type CTKs (r = 0.82), and aCTKs (r = 0.87) in the panicles (Figure 5A), as well as with the relative transport rates of tZ-type CTKs (r = 0.69), iP-type CTKs (r = 0.81), and aCTKs (r = 0.75) (Figure 5B). The relative number of spikelets per panicle was negatively and significantly correlated with the relative activity level of CKX (r = -0.69, Figure 5C). The relative activity levels of IPT, LOG, and CYP735A were poorly correlated with the relative number of spikelets per panicle (Figure 5D).
FIGURE 5. Regression analysis for the relative concentrations of panicle CTKs (A), relative transport rate of xylem CTKs (B), and relative activity levels of CKX (C), IPT, LOG, and CYP735A (D) with the relative number of spikelets per panicle across four varieties and three high temperature treatments.
Panicle size was reduced in response to the three high temperature treatments, especially in the HHZ, LYPJ, and N22 varieties (Figure 2). Previous studies also reported reduced panicle size in rice plants subjected to high temperature conditions (Wei et al., 2010; Wang et al., 2015). Heat-induced reduction in panicle size was associated with disruption of differentiation and induction of degradation of secondary branches and attached florets (Wang et al., 2015). The SY63 variety showed relatively stable panicle size under the three high temperature treatments. Moreover, the relative number of spikelets per SY63 panicle was significantly higher than that of the panicles of the HHZ and LYPJ varieties under the HNT, HDT, and ADT treatments (Figure 2). Therefore, the results presented in this study show that the effect of high temperature on panicle size is dependent on the variety of rice subjected to high temperature conditions. In addition, the SY63 variety has heat tolerance greater than that of the HHZ variety or LYPJ variety. Similarly, Wang et al. (2015) also reported genotypic variation in the effect of high temperature on panicle size during the early reproductive phase in rice. It is noteworthy that the N22 variety showed heat tolerance under the HNT and HDT conditions (Figure 2). However, the N22 variety suffered from heat stress injury under the ADT treatment (a combination of the HNT and HDT treatments), in contrast to the SY63 variety and similar to the heat-susceptible HHZ and LYPJ varieties (Figure 2). The increased intensity of high temperature conditions aggravates injury in rice during the reproductive stage (Jagadish et al., 2007). Therefore, our data suggest that the N22 variety can likely withstand only low intensity of heat stress, whereas the SY63 variety may be able to withstand high intensity of heat stress (a combination of the HNT and HDT treatments).
Panicle CTK abundance was reduced in response to high temperature during the early reproductive stage, especially in the heat-susceptible LYPJ and HHZ varieties (Table 1). This result was in agreement with previous observations that high temperature reduced the abundance of active CTKs (aCTKs) in Arabidopsis and Phalaenopsis (Chou et al., 2000; Skalák et al., 2016).
Under the HNT and HDT treatments, the N22 variety, which showed a stable number of spikelets per panicle, had relatively stable/high aCTK abundance in panicles; however, the N22 variety showed large reductions in the number of spikelets per panicle and the abundance of iP-type CTKs and aCTKs under the ADT treatment (combination of the HDT and HNT treatments), and the relative aCTK abundance of the N22 variety under the ADT treatment was similar to that of the heat-susceptible HHZ and LYPJ varieties (Table 1). In tobacco leaves, the abundance of aCTKs was reduced continuously as the duration of high temperature treatment was prolonged (Macková et al., 2013). However, in heat-tolerant variety SY63, which had a stable number of spikelets per panicle, the abundance of panicle tZ-type CTKs was not affected by the three high temperature treatments. Although panicle iP-type CTKs and aCTKs were reduced significantly in abundance by the high temperature treatments in the SY63 variety, their abundance remained relatively stable in comparison with that of the same CTKs in the other three varieties (Table 1). Heat-induced reduction in the number of spikelets per panicle was alleviated by application of exogenous BAP to heat-susceptible variety Liangyoupeijiu, which showed reduced CTK abundance under the high temperature treatments (Figure 1). In rice, exogenous BAP application increased the abundance of tZ-type CTKs (Liu et al., 2011) and the number of spikelets per panicle (Ding et al., 2014). Similarly, several passion fruit species and Arabidopsis ecotypes that showed stable abundance of aCTKs had less severe reductions in floret number in comparison with species and ecotypes that showed reduced CTK abundance in response to high temperature when exogenous CTKs were applied to mitigate the effect of heat injury on floret growth (Sobol et al., 2014). Additionally, we observed that relative panicle CTK concentrations were positively correlated with the relative number of spikelets per panicle (Figure 5A). In our study, reductions in the number of spikelets per panicle were associated with reduced panicle CTK abundance under high temperature treatments (Figure 6). The stability of panicle CTKs may be involved in maintaining panicle size under high temperature conditions in heat-tolerant variety SY63.
FIGURE 6. Proposed mechanism by which cytokinins mediate changes in the number of spikelets per panicle in response to high temperature treatments during the early reproductive phase in rice. -, +, and 0 indicate a decrease, an increase, and relative stability in a certain trait under high temperature treatment, respectively. → indicates a significant correlation between two traits. ⤑ indicates no significant correlation between two traits. The value of the trait with a box was decreased in heat-susceptible varieties while increased in heat-tolerant variety SY63 under the three high temperature treatments. The other traits without a box showed similar responses to the high temperature treatments, which showed larger changes in heat-susceptible varieties than that in heat-tolerant variety.
This study assessed the stability of tZ-type CTKs in SY63 panicles under high temperature treatments (Table 1). tZ-type CTKs are thought to act as messengers from root to shoot, while iP-type CTKs act as messengers from shoot to shoot (Kudo et al., 2010). Root-synthesized CTKs are transported to the aerial organs via xylem sap flow (Mader et al., 2003; Aloni et al., 2005; Yong et al., 2014). In SY63 variety, stable tZ-type CTKs in panicles may be partially attributed to stable import of root CTKs via enhanced xylem sap flow under the high temperature treatments (Table 2).
We observed that xylem sap flow showed genotypic variation in response to high temperature. In heat-susceptible varieties, xylem sap flow was decreased significantly by exposure to high temperature, while heat-tolerant variety SY63 showed significantly increased xylem sap flow after 15 days of high temperature treatment during the early reproductive phase (Table 2). Tao et al. (2009) found that heat-tolerant rice varieties showed enhanced exudation of xylem sap during 5 weeks of high temperature treatment; however, xylem sap exudation was inhibited significantly after 2 weeks of high temperature treatment in heat-susceptible rice varieties. Xylem sap flow is closely associated with transpiration flow (Boonman et al., 2007; Kudo et al., 2010). Under high temperature stress, the increased transpiration flow from roots may be importance for the decrease in leaf surface temperature via evaporative cooling, and for transport of root-derived messengers (such as ABA) transport to shoot to relief heat injury (Zandalinas et al., 2016). Therefore, our results suggest that heat-tolerant rice varieties may relieve heat stress via persistent enhancement of xylem sap flow in comparison that of heat-sensitive varieties.
In the present study, root CTKs and xylem sap CTKs showed similar responses to high temperature treatments; their abundance was slightly reduced in comparison with that of panicle CTKs (Tables 1, 2), indicating that the effects of high temperature treatments on panicle CTKs were more severe than their effects on root CTKs. Similarly, Takei et al. (2001b) reported that root CTKs showed changes in abundance that corresponded with changes in CTKs transport from root to shoot via xylem sap. In apple trees, exposure of roots to high temperature treatment produced negligible effects on xylem sap CTKs (Tromp and Ovaa, 1994). Additionally, high air temperature produced minor effects on CTKs in roots, which were less severe than the effects of high air temperature on CTKs in the aerial organs of pea plants (Vaseva et al., 2009). These studies demonstrate that CTKs in roots and xylem sap were less sensitive than CTKs in aerial organs, such as panicles, to high temperature.
In this study, the transport rate of CTKs changed in response to high temperature in a manner similar to that of xylem sap flow (r = 0.98, P < 0.01, n = 12). The transport rate of CTKs was decreased significantly by high temperature treatments in heat-susceptible varieties (LYPJ, HHZ, and N22), which showed decreased xylem sap flow rate in comparison with that of heat-tolerant variety SY63. However, the transport rate of CTKs in heat-tolerant variety SY63 increased as the xylem sap flow rate increased (Table 2). CTK transport is associated with xylem sap flow, which is closely associated with transpiration flow (Boonman et al., 2007; Kudo et al., 2010). In shade, reduced CTK transport leads to decreased CTK abundance in aerial organs (Boonman et al., 2007). In our study, the relative transport rate of CTKs was significantly correlated with relative CTK abundance in young panicles (Figure 3) and the relative number of spikelets per panicle (Figure 5). Root-derived CTKs translocated via xylem sap mediate apical shoot development (Aloni et al., 2005). Changes in xylem sap CTK abundance in response to environmental conditions regulate plant adaptation to adverse stresses (Ambler et al., 1992; Takei et al., 2001b; Alvarez et al., 2008; Kudoyarova et al., 2014). Taken together, our results suggest that transport of CTKs via xylem sap influences the number of spikelets per panicle by adjusting panicle CTK abundance under high temperature conditions (Figure 6). A relatively high CTK transport rate and relatively good panicle CTK stability likely contribute to the minimal reduction in the number of spikelets per panicle observed in heat-tolerant variety SY63 in response to high temperature.
In this study, CTK metabolism-related enzymes CKX, IPT, LOG, and CYP735A were regulated to different extents by high temperature treatments in a manner dependent on rice variety. The activity level of CKX increased significantly in heat-susceptible varieties LYPJ, HHZ, and N22; however, heat-tolerant variety SY63 showed no substantial changes in CKX activity under any of the three tested high temperature treatments. The activity levels of LOG, IPT, and CYP735A were reduced by high temperature in all four tested varieties (Table 3). Similar results were obtained in previous studies, in which the activity level of CKX was increased in Nicotiana tabacum and Pisum sativum under high temperature conditions (Vaseva-Gemisheva et al., 2004; Lubovská et al., 2014), whereas the activity levels of IPT, CYP735A, and LOG were suppressed by various abiotic stresses such as heat, cold, and drought (Maruyama et al., 2014; Skalák et al., 2016). These results suggest that stress conditions, including heat stress, disrupt the balance between synthesis and catabolism of CTKs via regulation of the activity levels of related enzymes.
Most previous studies have separately assessed the roles of IPT (Ding et al., 2014), CYP735A (Takei et al., 2004), LOG (Kurakawa et al., 2007), and CKX (Ashikari et al., 2005) in regulating CTK abundance and panicle size in rice and Arabidopsis; however, it is not yet clear which processes or enzymes play important roles in heat tolerance. CKX activity was stable under each high temperature treatment in heat-tolerant variety SY63, which showed stable panicle CTK abundance and a consistent number of spikelets per panicle. Correlation analysis also revealed that the relative CKX activity level was significantly and negatively correlated with relative panicle CTK abundance and the relative number of spikelets per panicle (Figures 4A, 5C). In rice and Nicotiana, CKX is involved in the effect of high temperature on plant growth (Tripathi et al., 2012; Lubovská et al., 2014). Although certain, but not always significant, relationships were found between the activity levels of CTK biosynthetic enzymes (IPT and CYP735A) and panicle CTK abundance (Figure 4), none of these enzymes had an activity level that was correlated with the relative number of spikelets per panicle (Figure 5D). However, it is noteworthy that the activity levels of IPT, LOG, and CYP735A were reduced significantly by the HDT and ADT treatments. Moreover, slight correlations were found between the activity levels of IPT and CYP735A and the concentrations of iP-type CTK and aCTK in panicles (Figures 4B,D). Therefore, the relationship between reductions in the activity levels of IPT and CYP735A and panicle size merit further investigation. Our study demonstrates that CKX activity seems to influence panicle size under high temperature treatments by regulating panicle CTK abundance (Figure 6). The stable CKX activity of heat-tolerant variety SY63 may underlie its stable panicle CTK abundance and consistent number of spikelets per panicle under high temperature conditions.
The three high temperature treatments significantly decreased the number of spikelets per panicle in heat-susceptible rice varieties (HHZ, LYPJ, and N22), whereas heat-tolerant variety SY63 showed a relatively stable number of spikelets per panicle. Application of exogenous BAP increased the number of spikelets per panicle in the LYPJ variety under the ADT treatment, indicating that CTKs are involved in panicle differentiation under high temperature conditions.
The three high temperature treatments significantly decreased panicle CTK abundance, xylem sap flow rate, and the transport rate of CTKs via xylem sap flow, whereas the treatments increased the activity level of CKX in three heat-sensitive rice varieties (HHZ, LYPJ, and N22). In comparison with the heat-susceptible varieties, heat-tolerant variety SY63 showed more stable panicle CTK abundance, an enhanced xylem sap flow rate, a greater CTK transport rate, and more stable CKX activity under the three high temperature treatments. The activity levels of enzymes involved in CTK synthesis (IPT, LOG, and CYP735A) were decreased by the high temperature treatments, especially by the HDT and ADT treatments.
Generally, panicle CTK concentrations were significantly and positively correlated with the xylem sap flow rate, transport rate of CTK via xylem sap, and CTK concentrations in xylem sap and roots, whereas panicle CTK concentrations were negatively correlated with CKX activity and slightly and positively correlated with the activity levels of IPT, and CYP735A.
According to our results, reduced panicle CTK abundance in heat-susceptible rice varieties under the high temperature treatments was associated with: (i) the reduced transport rate of xylem sap CTKs, which was resulted from the reduced xylem sap flow and the decreased xylem sap CTK concentration; (ii) increased activity of CKX in young panicles, which promoted degradation of panicle CTKs; (iii) decreased activity levels of IPT, and CYP735A, regardless of the insignificant correlations between the relative activity levels of these enzymes and relative panicle size. Therefore, CTK transport from root to shoot and CTK degradation via CKX are the key processes that determine panicle CTK abundance and thus regulate panicle size under high temperature conditions.
Generally, stable CTK concentrations under high temperature conditions inhibited the reduction in the number of spikelets per panicle in heat-tolerant variety SY63 in comparison with that which occurred in the heat-susceptible varieties; this effect was attributed to enhanced transportation of root-derived CTKs due mainly to strong xylem sap flow and impaired CTK degradation in panicles as a result of a relatively low level of panicle CKX activity. Therefore, a high transport rate of CTKs and low CKX activity are required to stabilize panicle size under heat stress (Figure 6). From the viewpoint of plant hormone metabolism, our results provide insight into the mechanisms underlying rice heat tolerance, laying a foundation for effective breeding and selection of rice varieties with high temperature tolerance and maximal grain yield.
CW and KC designed the experiments. CW, QL, WW, SF, and QH performed the experiments, KC, JH, LN, and SP performed parts of the experiments. CW and KC analyzed the data and wrote the manuscript. SF, JH, LN, PM, and SP revised the manuscript. All authors have read and approved the final manuscript.
The authors declare that the research was conducted in the absence of any commercial or financial relationships that could be construed as a potential conflict of interest.
This study was a part of the Ph.D. thesis research of CW (the first author) and was jointly supported by the Fundamental Research Funds for the Central Universities (No. 2011SC05), the National Natural Science Foundation of China (30971707, 31361140368), and the National Science & Technology Pillar Program (2013BAD07B10). We thank Professor Jianchang Yang’s group from Yangzhou University for training, technical support, and guidance on phytohormone determination.
The Supplementary Material for this article can be found online at: http://journal.frontiersin.org/article/10.3389/fpls.2017.00371/full#supplementary-material
Aloni, R., Langhans, M., Aloni, E., Dreieicher, E., and Ullrich, C. I. (2005). Root-synthesized cytokinin in Arabidopsis is distributed in the shoot by the transpiration stream. J. Exp. Bot. 56, 1535–1544. doi: 10.1093/jxb/eri148
Alvarez, S., Marsh, E. L., Schroeder, S. G., and Schachtman, D. P. (2008). Metabolomic and proteomic changes in the xylem sap of maize under drought. Plant Cell Environ. 31, 325–340. doi: 10.1111/j.1365-3040.2007.01770.x
Ambler, J. R., Morgan, P. W., and Jordan, W. R. (1992). Amounts of zeatin and zeatin riboside in xylem sap of senscent and nonsenescent sorghum. Crop Sci. 32, 411–419. doi: 10.2135/cropsci1992.0011183X003200020027x
Arai-Sanoh, Y., Ishimaru, T., Ohsumi, A., and Kondo, M. (2010). Effects of soil temperature on growth and root function in rice. Plant Prod. Sci. 13, 235–242. doi: 10.1626/pps.13.235
Ashikari, M., Sakakibara, H., Lin, S., Yamamoto, T., Takashi, T., Nishimura, A., et al. (2005). Cytokinin oxidase regulates rice grain production. Science 309, 741–745. doi: 10.1126/science.1113373
Boonman, A., Prinsen, E., Gilmer, F., Schurr, U., Peeters, A. J., Voesenek, L. A., et al. (2007). Cytokinin import rate as a signal for photosynthetic acclimation to canopy light gradients. Plant Physiol. 143, 1841–1852. doi: 10.1104/pp.106.094631
Bradford, M. M. (1976). A rapid and sensitive method for the quantitation of microgram quantities of protein utilizing the principle of protein-dye binding. Anal. Biochem. 72, 248–254. doi: 10.1016/0003-2697(76)90527-3
Chou, C. C., Chen, W. S., Huang, K. L., Yu, H. C., and Liao, L. J. (2000). Changes in cytokinin levels of Phalaenopsis leaves at high temperature. Plant Physiol. Biochem. 38, 309–314. doi: 10.1016/S0981-9428(00)00746-4
Ding, C. Q., You, J., Chen, L., Wang, S. H., and Ding, Y. F. (2014). Nitrogen fertilizer increases spikelet number per panicle by enhancing cytokinin synthesis in rice. Plant Cell Rep. 33, 363–371. doi: 10.1007/s00299-013-1536-9
Elagib, N. A. (2010). Trends in intra- and inter-annual temperature variabilities across Sudan. Ambio 39, 413–429. doi: 10.1007/s13280-010-0042-3
Frébort, I., Sebela, M., Galuszka, P., Werner, T., Schmülling, T., and Pec, P. (2002). Cytokinin oxidase/cytokinin dehydrogenase assay: optimized procedures and applications. Anal. Biochem. 306, 1–7. doi: 10.1006/abio.2002.5670
Ha, S., Vankova, R., Yamaguchi-Shinozaki, K., Shinozaki, K., and Tran, L. (2012). Cytokinins: metabolism and function in plant adaptation to environmental stresses. Trends Plant Sci. 17, 172–179. doi: 10.1016/j.tplants.2011.12.005
Hoyerová, K., Gaudinová, A., Malbeck, J., Dobrev, P. I., Kocábek, T., Solcová, B., et al. (2006). Efficiency of different methods of extraction and purification of cytokinins. Phytochemistry 67, 1151–1159. doi: 10.1016/j.phytochem.2006.03.010
Jagadish, S. V. K., Craufurd, P. Q., and Wheeler, T. R. (2007). High temperature stress and spikelet fertility in rice (Oryza sativa L.). J. Exp. Bot. 58, 1627–1635. doi: 10.1093/jxb/erm003
Jagadish, S. V. K., Murty, M. V. R., and Quick, W. P. (2015). Rice responses to rising temperatures-challenges, perspectives and future directions. Plant Cell Environ. 38, 1686–1698. doi: 10.1111/pce.12430
Kiba, T., Takei, K., Kojima, M., and Sakakibara, H. (2013). Side-chain modification of cytokinins controls shoot growth in Arabidopsis. Dev. Cell 27, 452–461. doi: 10.1016/j.devcel.2013.10.004
Kudo, T., Kiba, T., and Sakakibara, H. (2010). Metabolism and long-distance translocation of cytokinins. J. Integr. Plant Biol. 52, 53–60. doi: 10.1111/j.1744-7909.2010.00898.x
Kudoyarova, G. R., Korobova, A. V., Akhiyarova, G. R., Arkhipova, T. N., Zaytsev, D. Y., Prinsen, E., et al. (2014). Accumulation of cytokinins in roots and their export to the shoots of durum wheat plants treated with the protonophore carbonyl cyanide m-chlorophenylhydrazone (CCCP). J. Exp. Bot. 65, 2287–2294. doi: 10.1093/jxb/eru113
Kurakawa, T., Ueda, N., Maekawa, M., Kobayashi, K., Kojima, M., Nagato, Y., et al. (2007). Direct control of shoot meristem activity by a cytokinin-activating enzyme. Nature 445, 652–655. doi: 10.1038/nature05504
Kyozuka, J. (2007). Control of shoot and root meristem function by cytokinin. Curr. Opin. Plant Biol. 10, 442–446. doi: 10.1016/j.pbi.2007.08.010
Liu, X., Huang, B., and Banowetz, G. (2002). Cytokinin effects on creeping bentgrass responses to heat stress: I. Shoot and root growth. Crop Sci. 42, 457–465. doi: 10.2135/cropsci2002.4660
Liu, Y., Ding, Y., Wang, Q., Meng, D., and Wang, S. (2011). Effects of nitrogen and 6-benzylaminopurine on rice tiller bud growth and changes in endogenous hormones and nitrogen. Crop Sci. 51, 786–792. doi: 10.2135/cropsci2010.04.0217
Lubovská, Z., Dobrá, J., Storchová, H., Wilhelmová, N., and Vanková, R. (2014). Cytokinin oxidase/dehydrogenase overexpression modifies antioxidant defense against heat, drought and their combination in Nicotiana tabacum plants. J. Plant Physiol. 171, 1625–1633. doi: 10.1016/j.jplph.2014.06.021
Macková, H., Hronková, M., Dobrá, J., Turečková, V., Novák, O., Lubovská, Z., et al. (2013). Enhanced drought and heat stress tolerance of tobacco plants with ectopically enhanced cytokinin oxidase/dehydrogenase gene expression. J. Exp. Bot. 64, 2805–2815. doi: 10.1093/jxb/ert131
Mader, J. C., Turnbull, C. G. N., and Emery, R. J. N. (2003). Transport and metabolism of xylem cytokinins during lateral bud release in decapitated chickpea (Cicer arietinum) seedlings. Physiol. Plant. 117, 118–129. doi: 10.1034/j.1399-3054.2003.1170115.x
Maruyama, K., Urano, K., Yoshiwara, K., Morishita, Y., Sakurai, N., Suzuki, H., et al. (2014). Integrated analysis of the effects of cold and dehydration on rice metabolites, phytohormones, and gene transcripts. Plant Physiol. 164, 1759–1771. doi: 10.1104/pp.113.231720
Mika, J. (2013). Changes in weather and climate extremes: phenomenology and empirical approaches. Clim. Change 121, 15–26. doi: 10.1007/s10584-013-0914-1
Miyawaki, K., Tarkowski, P., Matsumoto-Kitano, M., Kato, T., Sato, S., Tarkowska, D., et al. (2006). Roles of Arabidopsis ATP/ADP isopentenyltransferases and tRNA isopentenyltransferases in cytokinin biosynthesis. Proc. Natl. Acad. Sci. U.S.A. 103, 16598–16603. doi: 10.1073/pnas.0603522103
Moldenhauer, K., Wilson, C. E., Counce, P., and Hardke, J. (2001). “Rice growth and development,” in Rice Production Handbook, ed. J. T. Hardke (Little Rock, AR: University of Arkansas Division of Agriculture Cooperative Extension Service), 7–14.
Pachauri, R. K., Allen, M. R., Barros, V. R., Broome, J., Cramer, W., Christ, R., et al. (2014). Climate Change 2014: Synthesis Report. Contribution of Working Groups I, II and III to the Fifth Assessment Report of the Intergovernmental Panel on Climate Change. Geneva: IPCC.
Peng, S., Huang, J., Sheehy, J. E., Laza, R. C., Visperas, R. M., Zhong, X., et al. (2004). Rice yields decline with higher night temperature from global warming. Proc. Natl. Acad. Sci. U.S.A. 101, 9971–9975. doi: 10.1073/pnas.0403720101
Qin, H., Gu, Q., Zhang, J., Sun, L., Kuppu, S., Zhang, Y., et al. (2011). Regulated expression of an isopentenyltransferase gene (IPT) in peanut significantly improves drought tolerance and increases yield under field conditions. Plant Cell Physiol. 52, 1904–1914. doi: 10.1093/pcp/pcr125
Rinne, P., and Saarelainen, A. (1994). Root produced DHZR-, ZR- and IPA-like cytokinins in xylem sap in relation to coppice shoot initiation and growth in cloned trees of Betula pubescens. Tree Physiol. 14, 1149–1161. doi: 10.1093/treephys/14.10.1149
Sasaki, E., Ogura, T., Takei, K., Kojima, M., Kitahata, N., Sakakibara, H., et al. (2013). Uniconazole, a cytochrome P450 inhibitor, inhibits trans-zeatin biosynthesis in Arabidopsis. Phytochemistry 87, 30–38. doi: 10.1016/j.phytochem.2012.11.023
Shi, W., Muthurajan, R., Rahman, H., Selvam, J., Peng, S., Zou, Y., et al. (2013). Source–sink dynamics and proteomic reprogramming under elevated night temperature and their impact on rice yield and grain quality. New Phytol. 197, 825–837. doi: 10.1111/nph.12088
Skalák, J., Černý, M., Jedelský, P., Dobrá, J., Ge, E., Novák, J., et al. (2016). Stimulation of ipt overexpression as a tool to elucidate the role of cytokinins in high temperature responses of Arabidopsis thaliana. J. Exp. Bot. 67, 2861–2873. doi: 10.1093/jxb/erw129
Singh, S. D., Chakrabarti B., and Aggarwal P. K. (2009). “Impact of elevated temperature on growth and yield of some field crops,” in Global Climate Change and Indian Agriculture, ed. P. K. Aggarwal (New Delhi: M/s Chandu Press), 45–47.
Sobol, S., Chayut, N., Nave, N., Kafle, D., Hegele, M., Kaminetsky, R., et al. (2014). Genetic variation in yield under hot ambient temperatures spotlights a role for cytokinin in protection of developing floral primordia. Plant Cell Environ. 37, 643–657. doi: 10.1111/pce.12184
Takei, K., Sakakibara, H., and Sugiyama, T. (2001a). Identification of genes encoding adenylate isopentenyltransferase, a cytokinin biosynthesis enzyme, in Arabidopsis thaliana. J. Biol. Chem. 276, 26405–26410. doi: 10.1074/jbc.M102130200
Takei, K., Sakakibara, H., Taniguchi, M., and Sugiyama, T. (2001b). Nitrogen-dependent accumulation of cytokinins in root and the translocation to leaf: implication of cytokinin species that induces gene expression of maize response regulator. Plant Cell Physiol. 42, 85–93. doi: 10.1093/pcp/pce009
Takei, K., Yamaya, T., and Sakakibara, H. (2004). Arabidopsis CYP735A1 and CYP735A2 encode cytokinin hydroxylases that catalyze the biosynthesis of trans-zeatin. J. Biol. Chem. 279, 41866–41872. doi: 10.1074/jbc.M406337200
Tao, L. X., Tan, H. J., Wang, X., Cao, L. Y., Song, J., and Cheng, S.-H. (2009). Physiological effects of high temperature stress on grain-setting for Guodao 6 during flowering and filling stage. Acta Agron. Sin. 35, 110–117. doi: 10.3724/SP.J.1006.2009.00110
Tokunaga, H., Kojima, M., Kuroha, T., Ishida, T., Sugimoto, K., Kiba, T., et al. (2012). Arabidopsis lonely guy (LOG) multiple mutants reveal a central role of the LOG-dependent pathway in cytokinin activation. Plant J. 69, 355–365. doi: 10.1111/j.1365-313X.2011.04795.x
Tripathi, A. K., Pareek, A., Sopory, S. K., and Singla-Pareek, S. L. (2012). Narrowing down the targets for yield improvement in rice under normal and abiotic stress conditions via expression profiling of yield-related genes. Rice 5, 1–12. doi: 10.1186/1939-8433-5-37
Tromp, J., and Ovaa, J. C. (1994). Spring cytokinin composition of xylem sap of apple at two root temperatures. Sci. Hortic. 57, 1–6. doi: 10.1016/0304-4238(94)90029-9
Udompraset, N., Li, P. H., Davis, D. W., and Markhart, A. H. (1995). Root cytokinin level in relation to heat tolerance of Phaseolus acutifolius and Phaseolus vulgaris. Crop Sci. 35, 486–490. doi: 10.2135/cropsci1995.0011183X003500020034x
Vaseva, I., Todorova, D., Malbeck, J., Travnièkova, A., and Machaèkova, I. (2009). Mild temperature stress modulates cytokinin content and cytokinin oxidase/dehydrogenase activity in young pea plants. Acta Agron. Hungarica 57, 33–40. doi: 10.1556/AAgr.57.2009.1.4
Vaseva-Gemisheva, I., Lee, D., Alexieva, V., and Karanov, E. (2004). Cytokinin oxidase/dehydrogenase in Pisum sativum plants during vegetative development. Influence of UV-B irradiation and high temperature on enzymatic activity. Plant Growth Regul. 42, 1–5. doi: 10.1023/B:GROW.0000014888.45592.4d
Vyroubalová,Š., Václavíková, K., Turecková, V., Novák, O., Smehilová, M., Hluska, T., et al. (2009). Characterization of new maize genes putatively involved in cytokinin metabolism and their expression during osmotic stress in relation to cytokinin levels. Plant Physiol. 151, 433–447. doi: 10.1104/pp.109.142489
Wang, Y. J., Ren, F. M., and Zhang, X. B. (2014). Spatial and temporal variations of regional high temperature events in China. Int. J. Climatol. 34, 3054–3065. doi: 10.1002/joc.3893
Wang, Y. L., Zhang, Y. P., Zeng, Y. H., Wu, H., Xiang, J., Chen, H.-Z., et al. (2015). Effect of high temperature stress on rice spikelet differentiation and degeneration during panicle initiation stage. Chin. J. Agrometeorol. 36, 724–731. doi: 10.3969/j.issn.1000-6362.2015.06.009
Wei, J. L., Pan, X. H., and Deng, Q. H. (2010). Effects of nighttime temperature increase at different growth stages on double season rice grain yield. Chin. J. Appl. Ecol. 21, 331–337.
Wu, C., Cui, K., Wang, W., Li, Q., Fahad, S., Hu, Q., et al. (2016). Heat-induced phytohormone changes are associated with disrupted early reproductive development and reduced yield in rice. Sci. Rep. 6:34978. doi: 10.1038/srep34978
Xie, J., and Zhang, Y. Z. (2001). Determination of plant intrinsic hormones by reversed phase high performance liquid chromatography. J. Instr. Anal. 20, 61–62. doi: 10.3969/j.issn.1004-4957.2001.01.019
Yong, J. W. H., Letham, D. S., Wong, S. C., and Farquhar, G. D. (2014). Rhizobium-induced elevation in xylem cytokinin delivery in pigeonpea induces changes in shoot development and leaf physiology. Funct. Plant. Biol. 41, 1323–1335. doi: 10.1071/FP14066
Zalewski, W., Galuszka, P., Gasparis, S., Orczyk, W., and Nadolska-Orczyk, A. (2010). Silencing of the HvCKX1 gene decreases the cytokinin oxidase/dehydrogenase level in barley and leads to higher plant productivity. J. Exp. Bot. 61, 1839–1851. doi: 10.1093/jxb/erq052
Zandalinas, S. I., Rivero, R. M., Martínez, V., Gómez-Cadenas, A., and Arbona, V. (2016). Tolerance of citrus plants to the combination of high temperatures and drought is associated to the increase in transpiration modulated by a reduction in abscisic acid levels. BMC Plant Biol. 16:105. doi: 10.1186/s12870-016-0791-7
Keywords: cytokinin oxidase/dehydrogenase, cytokinin synthetic enzymes, heat stress, panicle cytokinins, panicle size, rice (Oryza sativa), root xylem sap
Citation: Wu C, Cui K, Wang W, Li Q, Fahad S, Hu Q, Huang J, Nie L, Mohapatra PK and Peng S (2017) Heat-Induced Cytokinin Transportation and Degradation Are Associated with Reduced Panicle Cytokinin Expression and Fewer Spikelets per Panicle in Rice. Front. Plant Sci. 8:371. doi: 10.3389/fpls.2017.00371
Received: 10 September 2016; Accepted: 02 March 2017;
Published: 17 March 2017.
Edited by:
Sheo Mohan Prasad, Allahabad University, IndiaReviewed by:
Guzel Kudoyarova, Ufa Institute of Biology (RAS), RussiaCopyright © 2017 Wu, Cui, Wang, Li, Fahad, Hu, Huang, Nie, Mohapatra and Peng. This is an open-access article distributed under the terms of the Creative Commons Attribution License (CC BY). The use, distribution or reproduction in other forums is permitted, provided the original author(s) or licensor are credited and that the original publication in this journal is cited, in accordance with accepted academic practice. No use, distribution or reproduction is permitted which does not comply with these terms.
*Correspondence: Kehui Cui, Y3Vpa2VodWlAbWFpbC5oemF1LmVkdS5jbg==
Disclaimer: All claims expressed in this article are solely those of the authors and do not necessarily represent those of their affiliated organizations, or those of the publisher, the editors and the reviewers. Any product that may be evaluated in this article or claim that may be made by its manufacturer is not guaranteed or endorsed by the publisher.
Research integrity at Frontiers
Learn more about the work of our research integrity team to safeguard the quality of each article we publish.