- 1Chinese Cabbage Department, Institute of Vegetables and Flowers, Chinese Academy of Agricultural Sciences, Beijing, China
- 2Plant Breeding, Wageningen University and Research, Wageningen, Netherlands
The Brassica genus comprises many economically important worldwide cultivated crops. The well-established model of the Brassica genus, U’s triangle, consists of three basic diploid plant species (Brassica rapa, Brassica oleracea, and Brassica nigra) and three amphidiploid species (Brassica napus, Brassica juncea, and Brassica carinata) that arose through interspecific hybridizations. Despite being extensively studied because of its commercial relevance, several aspects of the origin of the Brassica species and the relationships within and among these six species still remain open questions. Here, we successfully de novo assembled 60 complete chloroplast genomes of Brassica genotypes of all six species. A complete map of the single nucleotide variants and insertions and deletions in the chloroplast genomes of different Brassica species was produced. The chloroplast genome consists of a Large and a Small Single Copy (LSC and SSC) region between two inverted repeats, and while these regions of chloroplast genomes have very different molecular evolutionary rates, phylogenetic analyses of different regions yielded no contradicting topologies and separated the Brassica genus into four clades. B. carinata and B. juncea share their chloroplast genome with one of their hybridization donors B. nigra and B. rapa, respectively, which fits the U model. B. rapa, surprisingly, shows evidence of two types of chloroplast genomes, with one type specific to some Italian broccoletto accessions. B. napus clearly has evidence for two independent hybridization events, as it contains either B. rapa chloroplast genomes. The divergence estimation suggests that B. nigra and B. carinata diverged from the main Brassica clade 13.7 million years ago (Mya), while B. rapa and B. oleracea diverged at 2.18 Mya. The use of the complete chloroplast DNA sequence not only provides insights into comparative genome analysis but also paves the way for a better understanding of the phylogenetic relationships within the Brassica genus.
Introduction
Chloroplast genome sequences have been used extensively for inferring plant phylogenies for several reasons. Chloroplast genomes of plants are known to be highly conserved and have a smaller effective population size and thus shorter coalescent time when compared with nuclear genomes (Birky et al., 1983; Jansen et al., 2007). Whole chloroplast genome analysis is known to provide high resolution plant phylogenies (Parks et al., 2009). The chloroplast genomes of angiosperms can be divided into large single copy (LSC) and small single copy (SSC) regions separated by inverted repeat (IR) regions (Palmer, 1985). Substitution rates of chloroplast genomes are much lower than those of nuclear genomes, and are further significantly reduced in the IR regions of chloroplast genomes compared to their LSC and SSC regions (Wolfe et al., 1987). Despite this low rate of change, progress in inferring phylogenetic relationships at lower taxonomic levels has been made using chloroplast DNA sequences. Examples are use of chloroplast genomes to find the origin of domesticated citrus species (Carbonell-Caballero et al., 2015) and apple varieties (Nikiforova et al., 2013), and to elucidate the difficult phylogeny at lower taxonomic levels in intractable plant groups, such as the Arundinarieae tribe (Ma et al., 2014). The chloroplast genome sequence of B. rapa is a circular unit of 153,482 bp length, composed of a pair of IR regions (26,212 bp each), a LSC region (83,282 bp), and a SSC region (17,776 bp) (Wu et al., 2012).
The Brassica genus comprises many economically important crops cultivated worldwide. The species demonstrate extreme morphological diversity and crop forms; for example, B. rapa includes Chinese cabbage (B. rapa L. ssp. pekinensis), turnip (ssp. rapa), Pak-choi (B. ssp. chinensis) and oil types (ssp. oleifera), and B. oleracea includes among others cauliflower (B. oleracea L. var. botrytis), broccoli (var. italica) and cabbage (var. capitata). The phylogeny of these species and their subspecies is not fully understood (Song et al., 1988).
The Brassica genus belongs to the Brassicaceae family which is composed of 338 genera and 3,709 species (Warwick et al., 2006). B. rapa (AA), B. nigra (BB), and B. oleracea (CC) are three basic diploid species that gave rise to three amphidiploid species, B. napus (AACC), B. juncea (AABB), and B. carinata (BBCC) through the interspecific hybridizations B. rapa × B. oleracea, B. rapa × B. nigra, and B. nigra × B. oleracea, respectively, as modeled in U’s triangle (Nagaharu, 1935). Although most studies on the species in U’s triangle have confirmed the diploid origins of the amphidiploid species, many questions remain concerning how and when the amphidiploid species evolved from their parental diploids (Prakash and Hinata, 1980). Also, very little is known about the origins of the diploid species themselves. Crosses between Brassica species to introgress specific traits or to broaden the gene pool, have likely also affected the maternal genome composition of the resulting progenies (Palmer et al., 1983; Qiu et al., 2006). Therefore, the Brassica genus provides an opportunity to study the origin and evolution of species over a wide range of timescales.
Cultivated Brassica species and closely related Arabidopsis diverged from a common ancestor, however, estimates of divergence time are controversial. Several studies estimate the divergence date around 20 million years ago (Mya); 24 Mya based on nuclear sequence variation (Koch et al., 2000), 14.5–20.4 Mya based on mitochondrial genes (Yang et al., 1999) and 23.35 Mya (Hohmann et al., 2015) based on the chloroplast genome calibrated using fossil evidence. Yet another study that also uses chloroplast genome data calibrated using evidence from four different fossils estimates this event at 43 Mya (Beilstein et al., 2010). Consequently, estimates of the divergence of B. rapa and B. oleracea from B. nigra also range from 20 Mya (Arias et al., 2014), till 7.9 Mya (Lysak et al., 2005). Divergence time of B. rapa and B. oleracea based on diverse nuclear sequences was estimated 3.7–4 Mya (Inaba and Nishio, 2002; Liu et al., 2014) or 1.37–0.12 Mya (Cheung et al., 2009). B. napus was formed 7500–12500 years ago by interspecific hybridization between B. rapa and B. oleracea (Chalhoub et al., 2014). From the above it is evident that divergence estimates within the Brassica genus are controversial (Lysak et al., 2005; Cheung et al., 2009; Beilstein et al., 2010; Arias et al., 2014; Hohmann et al., 2015).
Based on chloroplast DNA (Warwick and Black, 1991) and mitochondrial DNA (Palmer and Herbon, 1988), the Brassica species are divided into two evolutionary lineages: the nigra lineage and the rapa/oleracea lineage. Several studies concluded that formation of B. napus via hybridization occurred multiple times involving different maternal genotypes. RFLP marker analysis of both chloroplast and mitochondrial DNA of 46 accessions representing B. rapa, B. oleracea, B. montana, and B. napus indicated four major cytoplasm types, representing almost all B. rapa, broccoletto (a B. rapa subspecies), B. oleracea, and B. montana (Song and Osborn, 1992). These authors also provided evidence for multiple origins of B. napus and showed identical chloroplast RFLP patterns between B. montana and B. napus. In another study, chloroplast SSRs and nuclear RFLPs were used to identify the ancestors of B. napus (Allender and King, 2010). This study also concluded that multiple hybridization events with different maternal genotypes resulted in B. napus, and suggested as the maternal donors both weedy B. rapa accessions and broccoletto accessions with divergent chloroplast genomes, but found no indication for B. montana as maternal parent. These studies are based on genetic markers (RFLP, AFLP, SSRs) that only characterize a small part of the genomes.
Although previous studies on Brassica phylogenetic relationships were based on nuclear DNA markers (Song et al., 1990; Allender and King, 2010) or on partial cytoplasmic DNA sequences (Palmer et al., 1983; Warwick and Black, 1997; Allender and King, 2010), the analysis of the complete chloroplast genome should render phylogenetic relationships with stronger support. The recent availability of nuclear (Wang et al., 2011; Chalhoub et al., 2014; Liu et al., 2014; Parkin et al., 2014) and chloroplast (Wu et al., 2012; Hao et al., 2014; Prabhudas et al., 2015) reference genomes of Brassica species enables such more detailed studies of the origin and phylogenetic relationships within the Brassica’s. We assembled 60 de novo chloroplast genomes representative of the economically important Brassica species and constructed a maternal phylogeny. The study of the distribution of mutational events, such as single nucleotide variants (SNVs), deletions and insertions along the evolutionary history of the Brassica genus, allowed inference of the origin of the amphidiploid species. Additionally, this comparative analysis also produced new insights into divergence times between Brassica species.
Materials and Methods
Plant Material and DNA Extraction
Plant materials sequenced in this study were provided by the Chinese Cabbage Research Group of the Institute of Vegetables and Flowers, Chinese Academy of Agricultural Sciences (CAAS-IVF), from the Dutch Crop Genetic Resources Center (CGN) in Wageningen UR and from Bejo and Rijk Zwaan breeding companies, comprising a total of 60 Brassica accessions (Supplementary Table S1). For the accessions that were inbred or DH lines, DNA was isolated from 50 to 100 two-week-old seedlings (hypocotyls and cotyledons) or from leaves from a single plant. For GeneBank accessions, DNA was isolated from single plants, as accessions are generally heterogeneous. In the paper, we use the term accession for both the single plants representing GeneBank accessions and the DH or inbred lines from breeding companies. Total DNA was extracted from seedlings (hypocotyls and cotyledons) or fresh leaves of the 60 Brassica accessions by the conventional cetyltrimethylammonium bromide (CTAB) method (Chen and Ronald, 1999) and stored at -80°C. Chloroplast genome sequences of A. thaliana (NC_000932), A. lyrata (LN877383), B. rapa (DQ231548), B. juncea (KT581449), B. oleracea (KR233156), B. nigra (KT878383) and B. napus (KP161617, GQ861354, KM454973 and KJ872515) were obtained from the original publication.
Genome Sequencing
Whole genome sequences were generated using Illumina HiSeq2000 and HiSeq3000. In brief, short-insert (400-bp) libraries were constructed using the TruSeqTM DNA sample preparation kit (Illumina, USA) for Illumina HiSeq sequencing and following the manufacturer’s protocol (Illumina). DNA from the different samples was indexed by tags and pooled together in one lane of Illumina’s Genome Analyzer for sequencing. Raw reads were first filtered to obtain the high-quality clean data by removing adaptor sequences and low-quality reads with Q-value ≤20.
Chloroplast Genome Assembly, Annotation, Alignment, and Visualization
The chloroplast genomes were assembled using a highly automated five stage pipeline (manuscript in preparation). Briefly this pipeline consists of the following stages: (1) Extraction of a k-mer frequency table from raw data and detection of two linked peaks at frequency N and 2∗N, representing single copy and IR regions of the chloroplast genome, respectively, and extraction of underlying k-mers. (2) Extraction of reads containing these k-mers and initial assembly using a parameter spread. (3) Refinement of initial assembly by iterative selection of additional reads from raw data based on previous assembly and subsequent reassembly. (4) Local selection and reassembly of additional reads near scaffold ends to alleviate problems caused by variable representation of the chloroplast genome in the sequencing library. (5) Gap filling by local selection and de novo assembly of these gap-filling reads and insertion of gap-filling contigs into the scaffolds. This pipeline takes into account the circular and quadripartite [(LSC, IR (twice) and SSC] nature of the chloroplast genome, and may, if data supports this, produce finished circular chloroplast genome assemblies. The assembled chloroplast genomes were deposited to BRAD1.
The chloroplast genes were annotated using an online DOGMA tool (Wyman et al., 2004), using default parameters to predict protein-coding genes, transfer RNA (tRNA) genes, and ribosome RNA (rRNA) genes. Start and stop codons of protein-coding genes were searched and determined by BLASTX against the NCBI protein database, with B. rapa as a guide. Genome maps were drawn with OGDraw (Lohse et al., 2007). Multiple sequence alignments were performed in MAFFT version 5 (Katoh et al., 2005) under standard parameters, and adjusted manually where necessary. Full alignments with annotations were visualized using the VISTA viewer (Frazer et al., 2004). Pairwise assembly alignments were made between the reference genome and the de novo assembled genomes using MUMmer (Kurtz et al., 2004).
Variant Calling
Sample sequences were mapped against the B. rapa chloroplast genome (Chiifu). The mapping procedure applied in this study is summarized as follows: Burrows–Wheeler Aligner (BWA 0.6.2) software was used to map paired-end reads in this study (Li and Durbin, 2010), and reads with low-quality alignments were then filtered out from mapping using SAMtools (Li et al., 2009) with the default parameters. The final set of mapping files (BAM) was used to perform a multisample variant calling by using BCFtools. The calling parameters were adjusted to obtain both SNVs and InDels.
Phylogenetic Analysis
To evaluate consistency of phylogenetic trees produced from regions with different molecular evolutionary rates (Palmer, 1985), we extracted three subsets (LSC, SSC, and IRs) from the complete chloroplast data set, and combined these to produce three types of trees.
The program JModeltest 2 was used to find the optimal substitution model for each subset (Posada and Crandall, 1998; Darriba et al., 2012), using both the Bayesian information criterion (Felsenstein, 1988) and the Akaike information criterion (Akaike, 1974). Models for three datasets were explored: LSC+SSC dataset (GTR+G model), IR dataset (GTR+I model) and whole chloroplast genome (GTR+I+G model). Assembled chloroplast sequences were aligned with the help of MAFFT software (Katoh et al., 2005). The maximum likelihood (ML) tree was computed in PhyML (Guindon et al., 2010) using settings of the best-fitting model tested by JModeltest program (Darriba et al., 2012). The method used was a maximum-likelihood iterative model and a bootstrap of 1,000 repetitions was used to assess the reliability of the phylogeny reconstructed.
Estimation of Divergence Times within Brassica
Divergence times of Brassica species were estimated using the BEAST program with the Bayesian method (Drummond et al., 2012). Uncorrelated relaxed lognormal clock was used in our analysis that allows different rates to be optimized independently on each branch of the tree. The Yule process described the likelihood of speciation for this analysis (Yule, 1925), and branching rates were determined under an optimal GTR+I+G nucleotide model. Two independent Markov chain Monte Carlo runs in BEAST were conducted for 500,000,000 generations sampling every 50,000 generations for the chloroplast genome analysis. To calibrate the phylogenetic tree of the chloroplast genomes of the accessions belonging to the six Brassica species, estimations of dates of speciation events calculated by valid fossil evidence were used (Hohmann et al., 2015). In our experiment, Arabidopsis was the outgroup, and the root age was constrained by a normal distribution with a mean of 23.35 Mya. In addition, the divergence age of A. thaliana and A. lyrata was set at 5.97 Mya. We then used Tracer v. 1.6 to explore the output of BEAST (Rambaut et al., 2014). TreeAnnotator v. 1.8 (part of the BEAST package) was used to produce maximum clade credibility trees from the postburn-in trees and to determine the 95% posterior density of ages for all nodes in the tree.
Results
Characteristics of Data Sets and Chloroplast Assembly
Sixty sequenced chloroplast genomes of Brassica genotypes together with seven published chloroplast genomes were used in the present study, including chloroplast genomes of 30 B. rapa accessions representing nine different morphotypes, of 17 B. oleracea accessions representing seven different morphotypes, of eight B. napus accessions representing oilseed and swede morphotypes, of eight B. juncea accessions representing five morphotypes, of one B. nigra and of one B. carinata; as outgroup one chloroplast genome each of A. thaliana and A. lyrata was included (Supplementary Table S1). The raw reads were mapped onto the B. rapa subsp. pekinensis (Chiifu, DQ231548.1) chloroplast genome as described in the Section “Materials and Methods.” Mapped reads of each genotype for the sequences of 60 whole chloroplast genomes are listed in Supplementary Table S1. The chloroplast genome coverage of each sample was very high (Supplementary Table S1) because of the numerous copies of chloroplasts in leaf cells (Zhang et al., 2011).
In this study, we used k-mer frequency histograms to identify and extract the chloroplast reads from total DNA sequencing data (Supplementary Figure S1) and assembled chloroplast genomes for these accessions using a highly integrated and automated pipeline. Dot plot analyses using MUMmer software was performed to compare de novo assembled genomes with the reference genome (Figure 1). Assembled genomes of all six Brassica species are collinear to previously published chloroplast genomes of Brassica (Wu et al., 2012; Hao et al., 2014), as no rearrangements were identified. Their genome sizes are very similar, ranging from 152860 to 153700 bp (Supplementary Table S1).
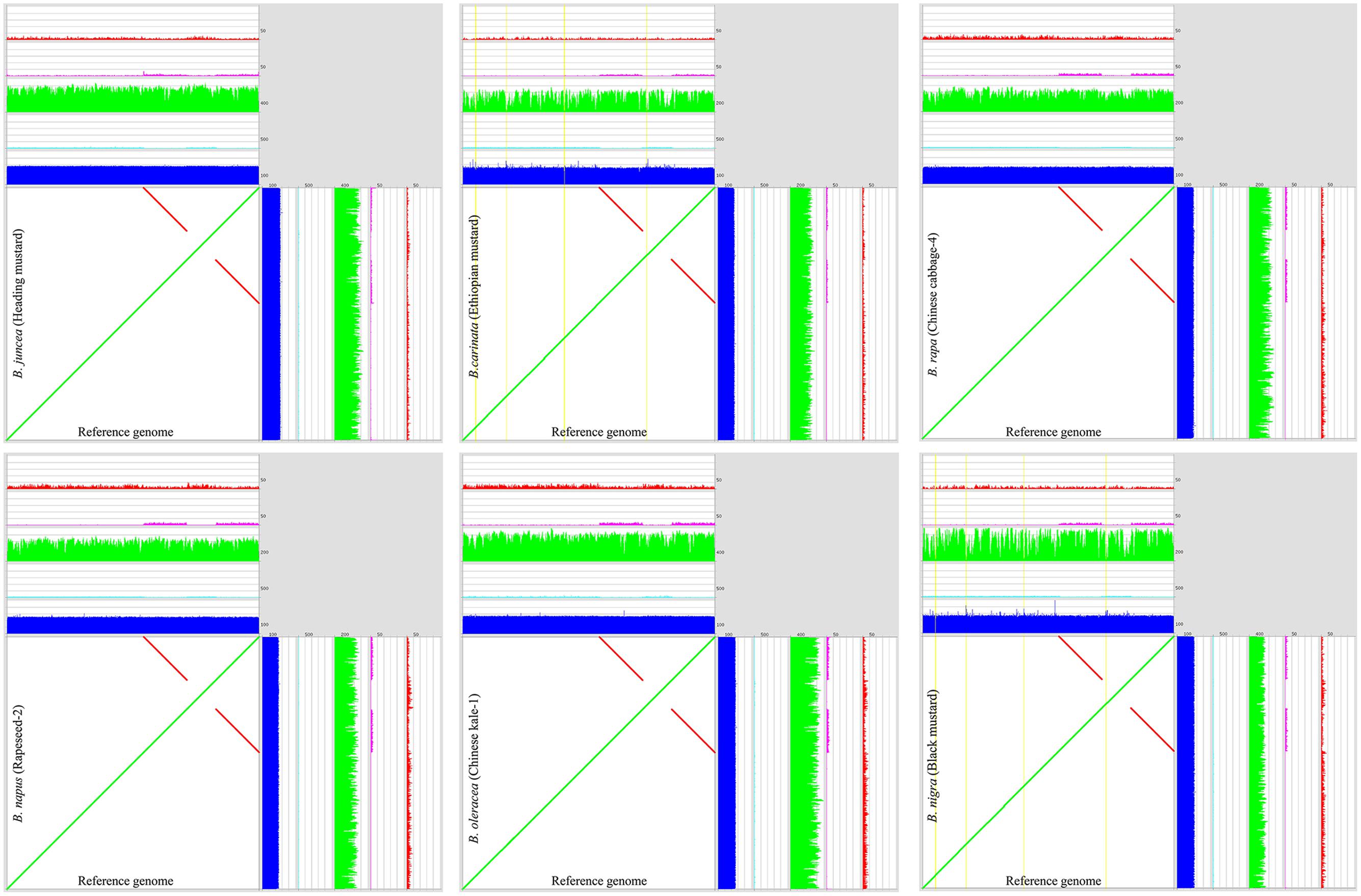
FIGURE 1. Comparison of the de novo assembled chloroplast genomes (along the Y-axis) with the reference chloroplast genome of B. rapa Chiifu (along the X-axis) visualized in a mummer-plot. In the MUMmer-plots, yellow lines denote areas with paired-end coverage of less than 10% of the average. The colored tracks shown in each figure are the average fragment length of the paired-end data (blue), relative number of non-mapped base pairs (cyan), paired-end sequenced DNA fragments coverage (green), nucleotide coverage by discordant read-pairs (pink) and nucleotide coverage by linking read-pairs (red), respectively.
Variant Analysis in Chloroplast Genomes of the Brassica Genus
A total of 2646 SNVs and 464 insertions and deletions (InDels) were detected by Burrows–Wheeler Aligner software (BWA) and SAMtools, summing up to a total of 3110 high-quality variant positions (Supplementary Tables S2 and S3). Manual curation and the high coverage used in this study allowed the detection of discrepancies with the reference genome that can most probably be attributed to Sanger sequencing errors in the original sequence. For example, position 116457 (A) of the B. rapa subsp. pekinensis (Chiifu) chloroplast genome that we re-sequenced was different from the reference (G, DQ231548.1). The number of changes observed for all the four nucleotides among the 2646 SNVs were 52.4% A or T, 47.6% G or C. In total, 56.3% SNVs were transversions and 43.7% SNVs were transitions. Of the transitions, 48 and 52% were A/G and T/C transitions, respectively. While A/C, A/T, C/G, and G/T transversions constituted 34.09, 16.31, 8.26, and, 41.34% of the cases, respectively. Also 25 triallelic positions were observed in these Brassica species.
The average SNV density in the whole chloroplast genome was 17.2 SNVs per kb, while the average SNV density for each region (LSC, SSC, and IRs) was 21.9, 33.0 and 4.5 SNVs per kb, respectively. The IR regions showed a much lower SNV density in comparison with the chloroplast average. The average SNV density for exons (total size 87356 bp), introns (total size 12574 bp) and intergenic regions (total size 53552 bp) was 12.5, 14.0 and 25.7 SNVs per kb, respectively. About 52.0% of variant positions (1376) were found in intergenic regions. Furthermore, some genes such as matK, rpoC2, rpoB, ycf2.1, ndhF, and ycf1.2, displayed a remarkably high number of SNVs in their exons (46, 71, 39, 40, 49, and 237, respectively; Supplementary Table S4). A 500 bp window size was used to calculate and draw the SNVs density distribution along the chloroplast genome, to identify regions with increased SNV densities that we consider hotspots of variation of chloroplast genomes (Figure 2). In addition, circular maps were drawn to show the hotspots of variation of chloroplast genomes in the three diploid Brassica species separately (Supplementary Figure S2). In B. rapa, based on 30 chloroplast genomes, the average SNV density in the chloroplast genome was 2.2 SNVs per kb, while the average SNV density for each region (LSC, SSC, and IRs) was 3.0, 3.9, and 0.5 SNVs per kb, respectively. In B. oleracea, based on 17 chloroplast genomes and the reference B. oleracea chloroplast genome, the average SNV density in the chloroplast genome was much lower with 0.1 SNVs per kb, while the average SNV density for each region (LSC, SSC, and IRs) was 0.2, 0.2, and 0 SNVs per kb, respectively. In B. nigra, based on two chloroplast genomes only the average SNV density was 0.5 SNVs per kb, while the average SNV density for each region (LSC, SSC, and IRs) was 0.6, 1.3, and 0.1 SNVs per kb, respectively. In total, 343 SNVs and 125 InDels were identified in the chloroplast genomes of the 30 B. rapa accessions. Interestingly, the chloroplast genomes of four B. rapa broccoletto accessions contributed many more SNVs and InDels than chloroplast genomes of other B. rapa accessions (Supplementary Table S1).
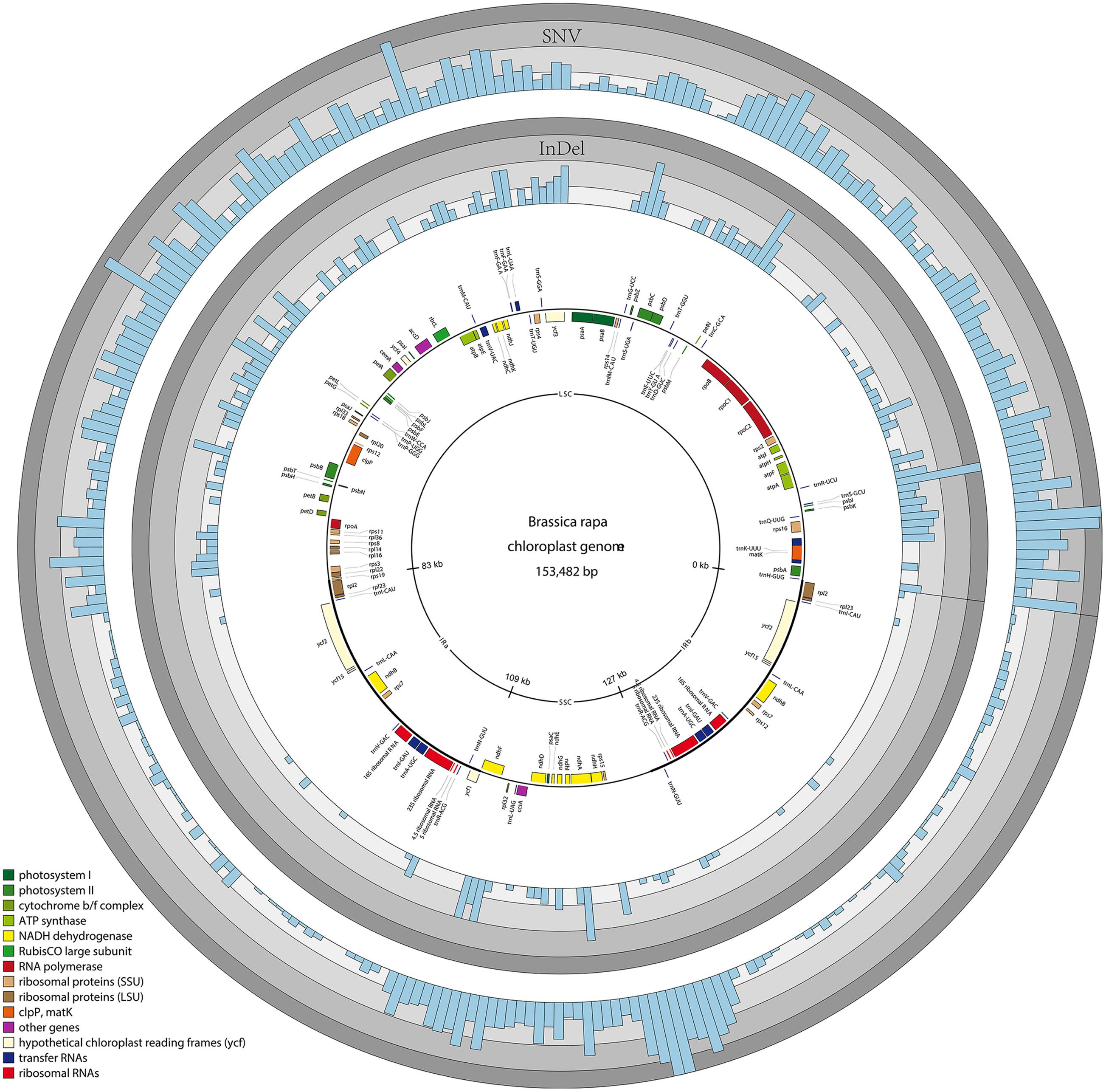
FIGURE 2. Variability of the six economically important Brassica species represented over the circular map of the B. rapa chloroplast genome. The two inverted repeat regions (IRa and IRb) separate the large (LSC) and small (SSC) single copy regions, respectively. The colored rings represent the density of single nucleotide variants (SNVs) (0–35) and InDels (0–16).
The average InDel density in the chloroplast genome, when chloroplast genomes of all six Brassica species were included was 3.0 InDels per kb, while the average InDel density for each region (LSC, SSC, and IRs) was 4.6, 3.3, and 0.4 SNVs per kb, respectively. The average InDel density for exons (total size 87356 bp), introns (total size 12574 bp) and intergenic regions (total size 53552 bp) was 0.1, 4.9, and 7.3 InDel per kb, respectively. In B. rapa, the average InDel density in the chloroplast genome was 0.8 InDel per kb, while the average InDel density for each region (LSC, SSC, and IRs) was 1.2, 1.1, and 0.1 InDel per kb, respectively. In B. oleracea, the average InDel density in the chloroplast genome was 0.04 InDel per kb, while the average InDel density for each region (LSC, SSC, and IRs) was 0.06, 0.06, and 0 InDel per kb, respectively. In B. nigra, the average InDel density in the chloroplast genome was 0.16 InDel per kb, while the average InDel density for each region (LSC, SSC, and IRs) was 0.28, 0.06, and 0.02 InDel per kb, respectively. Analysis of the distribution of the 464 InDels revealed that 392 (84.5%) were situated in intergenic regions whereas only 72 (15.5%) were located in genes (Supplementary Table S4; Supplementary Figure S3). Sixty one out of the 72 InDels were in introns whereas the remaining 11 InDels affected the coding regions of trnK-UUU, trnQ-UUG, rpoC2, trnS-UGA, and accD genes. A total of 464 manually curated high-quality InDels, ranging from 1 to 38 bp (including 41 triallelic positions), were detected. Only 6 out of 464 InDels were longer than 10 bp. Furthermore, 237 InDels were single-base InDels.
Tree Structure Using Different Data Subsets
As the single copy regions (LSC and SSC) and IRs regions have very different molecular evolutionary rates (Palmer, 1985), three subsets of the data were produced, containing either the combination of LSC and SSC, only the IR or the whole chloroplast genome sequence.
The phylogenetic relationships derived from the LSC+SSC dataset under GTR+G model, IR dataset under GTR+I model and whole chloroplast genome under GTR+I+G model are shown in Figure 3. The topologies estimated from ML analyses of different data subsets were similar to each other, with four clades clearly distinguished. The only differences were the poorly supported branches within lowly divergent clades (Figure 3). Overall, phylogenetic analyses of the three different datasets did not generate strongly supported topological conflict. The phylogenetic resolution of the LSC+SSC subset (Figure 3a) and the whole chloroplast genome sequences subset (Figure 3c) was higher than that of the IR subset (Figure 3b). Clade I contained the chloroplast genomes of mustards (B. juncea), of most B. rapa accessions and of two B. napus accessions. Clade I based on the LSC+SSC subset was divided into five subclades (subclade a–e), while divided in three subclades (subclade a–c) when based on the whole chloroplast genome. These subclade groupings do not fully agree with each other. The subclade ‘a’ in the LSC+SSC analysis, containing chloroplast genomes of all mustard accessions, and of several B. rapa accessions (Japanese leafy types and turnips, one Italian broccoletto, and a European turnip), generally corresponds to the subclade ‘a’ of the whole chloroplast analysis, except for the chloroplast genome of one European turnip (5). The subclade b of the whole chloroplast analysis, containing chloroplast genomes of several B. rapa morphotypes, corresponds to subclade b, c, and d of the LSC+SSC based analysis. Subclade ‘e’ with chloroplast genomes of sarsons (B. rapa) and B. napus is identical for the two analyses. The fact that the results of different datasets were almost identical, in addition to their corresponding internal bootstrap support, shows the reliability of the phylogenies. Moreover, ML analyses of the LSC+SSC chloroplast sequences demonstrated increased resolution in the tree topology with higher support values (Figure 3a) compared to the other two datasets. Thus, we mainly focus on results from the LSC+SSC region of chloroplast genome analyses.
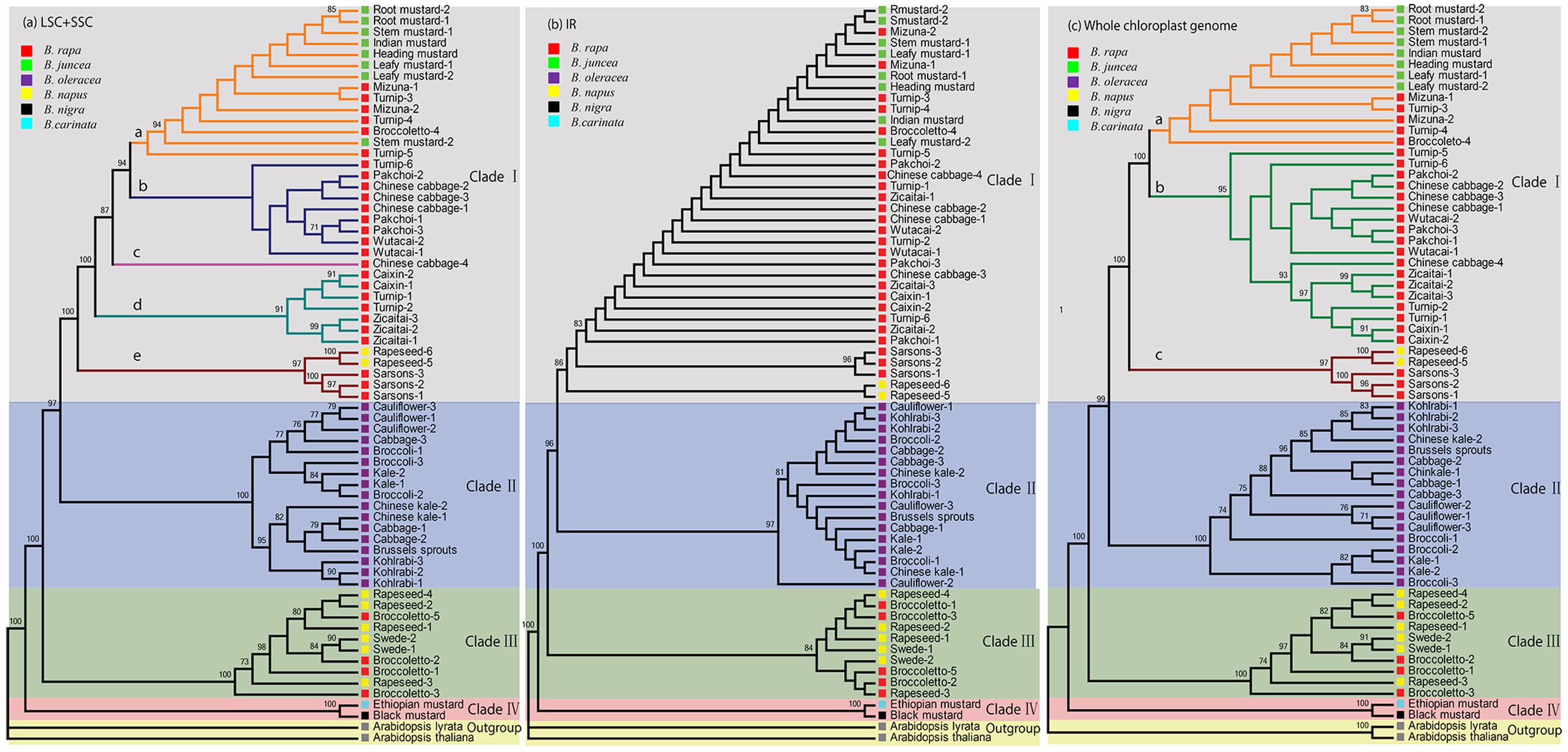
FIGURE 3. Tree structure of Brassica species inferred from maximum likelihood (ML) analyses of different chloroplast genome sequences. ML topology shown with bootstrap support values listed at each node, derived from the LSC+SSC dataset under GTR+G model (a), IR dataset under GTR+I model (b) and whole chloroplast genome under GTR+I+G model (c). Colored background of this figure indicates the four Brassica clades. Colored branches show subclades of clade I.
Phylogenetic Analysis of the Brassica Genus
We generated a ML phylogeny from the 67 single copy LSC+SSC regions of chloroplast genomes including 60 de novo assembled sequences and seven previously published chloroplast genomes. The phylogenetic tree was rooted with A. thaliana and A. lyrata as outgroup and showed a clustering topology in general well supported with high bootstrap values (Figure 4). Our phylogenetic analyses of chloroplasts sequences indicated a clear division of the genus Brassica into two separate evolutionary linages, including four different clades (Figure 4), which was quite similar to the phylogeny using whole chloroplast sequences (Supplementary Figure S4).
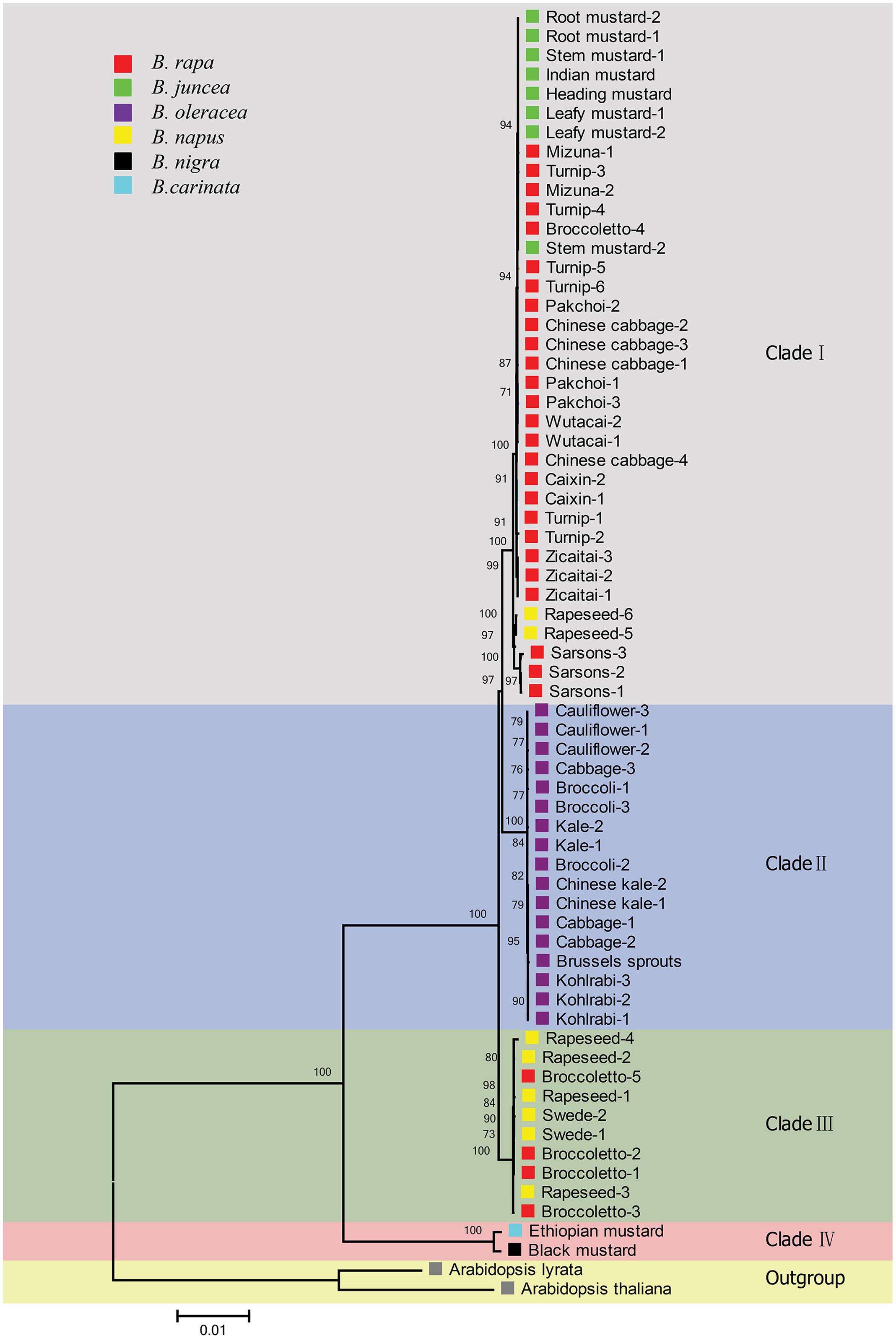
FIGURE 4. Phylogenetic tree constructed from ML analysis using the settings of the optimal substitution model (GTR+G nucleotide model) for the alignment of single copy regions of chloroplast genomes. Colors in the square box and the right side represent the different Brassica species and the separated chloroplast clades, respectively. Bootstrap support values for the clades are displayed in the corresponding branching points of the tree.
One of these two separate linages contained three clades formed by the chloroplast genomes of B. rapa, B. oleracea, B. napus and B. juncea accessions (Figure 4). The clade I (strongly supported with 100% BP), contained the chloroplast genomes of 26 B. rapa accessions representing all different B. rapa morphotypes, together with chloroplast genomes of two B. napus accessions and of all the eight B. juncea accessions. Furthermore, the chloroplast genomes of these two B. napus accessions were grouped with those of three B. rapa (sarsons) in this clade. The chloroplast genomes of all the B. juncea accessions were grouped with those of the B. rapa accessions in clade I, supporting that B. rapa is the maternal ancestor of B. juncea. In clade II, the chloroplast genomes of all the 17 B. oleracea accessions we examined were placed together. Interestingly, clade III in the phylogenetic tree is composed of the chloroplast genomes of six B. napus and four B. rapa broccoletto accessions, significantly supported by bootstrap values. The chloroplast genomes of the six B. napus accessions that include both rapeseed and swede accessions, were closely related to those of the four broccoletto accessions and less related to those of the other two rapeseed B. napus accessions that were placed in clade I, close to the chloroplast genomes of the B. rapa sarsons. Clade IV represents the other lineage, and corresponds to B. nigra (black mustard) and B. carinata (Ethiopian mustard) chloroplast genomes, supporting that B. nigra is the maternal ancestor of B. carinata. The branch length of the tree indicated that B. nigra and B. carinata chloroplast genomes are more distant from B. oleracea, B. napus, B. juncea and B. rapa chloroplast genomes than the chloroplast genomes of these four species are from each other, suggesting much earlier divergence of B. nigra from the other diploid Brassica’s.
Estimation of Divergence Times in the Brassica Genus
To estimate when separation of these chloroplast lineages in Brassica occurred, we used two calibration points of speciation events calibrated by valid fossil evidence (Hohmann et al., 2015). The results of our dating experiments are presented in Figure 5.
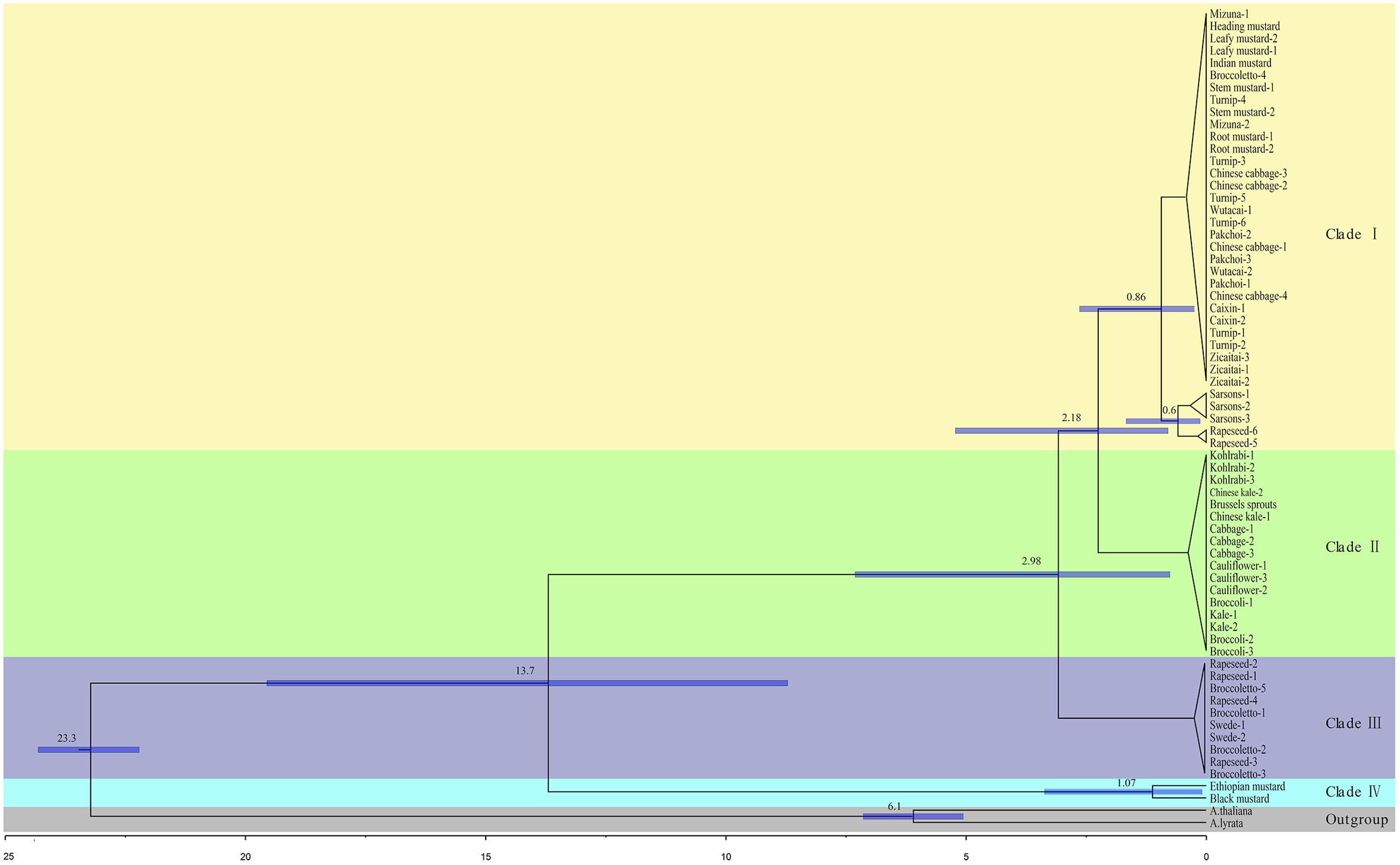
FIGURE 5. Brassica chronogram inferred using BEAST program from sequence alignment of chloroplast genomes using Arabidopsis as outgroup. The root age was constrained by a normal distribution with a mean of 23.35 Mya. In addition, the divergence age of A. thaliana and A. lyrata was set at 5.97 Mya. Dating for the clusters, which branching pattern could not be resolved in the ML analysis (Figure 4), was considered to be unreliable and is not shown here. Divergence times are indicated with 95% confidence intervals.
Our results indicate that, the ancestors of the current major Brassica groups were generated in several speciation events that started with a first radiation approximately 13.7 Mya separating clade IV (B. nigra and B. carinata) from the rest of Brassica species. B. nigra and B. carinata species diverged from each other 1.07 Mya. In a second period of speciation, the clade III (broccoletto and B. napus) separated from clade I (B. rapa, B. juncea and B. napus) and clade II (B. oleracea) at 2.98 Mya. In a third period, approximately 2.18 Mya, the chloroplast line shared by clade I (B. rapa, B. juncea and B. napus) diverged from clade II (B. oleracea). Later on, the Sarson chloroplast lines diverged from the B. rapa cluster approximately 0.86 Mya.
Discussion
As plant cells contain multiple copies of chloroplast genomes (Pyke, 1999), low coverage total genome sequencing data are useful for both nuclear marker acquisition as well as for the assembly of chloroplast genomes (Tangphatsornruang et al., 2010; Nock et al., 2011). Here we demonstrate that low coverage resequencing data (at 1x nuclear genome coverage) can be used for a complete de novo assembly of the chloroplast genomes of Brassica species. Despite the fact that complete chloroplast genome sequences can provide a wealth of information to study the origin of photosynthetic eukaryotes (Chu et al., 2004), few Brassica chloroplast genomes have been published during the past decade (Wu et al., 2012; Hao et al., 2014; Prabhudas et al., 2015). In this study, the assembly of 60 complete chloroplast genomes representing the three diploid and three amphidiploid Brassica species (Supplementary Table S1) has certainly added new and valuable information to solve important controversies on the evolution of the genus (Warwick and Black, 1991). The assembled genomes allowed us to derive a highly reliable phylogenetic tree, rooted with Arabidopsis as outgroup (Figure 4).
Due to the uniparental inheritance and absence of recombination, the chloroplast genome is phylogenetically linear over generations, changing only by occasional mutations. In this research, a total of 2646 SNVs and 464 InDels were identified, which rendered patterns of distribution across species compatible with the topology of the phylogenetic tree (Figure 3), providing an independent support to our proposal. In the chloroplast genomes of all the B. rapa accessions we detected, 343 SNVs and 125 InDels, whereas only 16 SNVs and 6 InDels were detected within the chloroplast genomes of 17 B. oleracea accessions. This observation agrees with the results from previous nuclear RFLP and chloroplast SSR analysis that indicated more genetic diversity in chloroplast genomes of B. rapa’s than of B. oleracea’s (Song et al., 1990; Allender and King, 2010). Several coding regions accumulated a higher number of variants compared with the average regions of the genome. Furthermore, several specific genes accumulated simultaneously a high number of SNVs and at least one InDel in their coding region (Supplementary Table S4), suggesting that these genes may function as general hotspots of natural genetic variation in Brassica chloroplast genomes. Meanwhile, the specific variations can be used as markers for haplotype identification to distinguish different cytoplasms. Our preliminary data indicate that the variations in IRs are much lower than in LSC and SSC regions. This difference in evolutionary rate has previously been attributed to conservation of the ribosomal RNA genes, which occupy about one-third of the IR region of the chloroplast genomes (Palmer, 1985).
As one of our aims is to see whether the amphidiploid species are the result of a single hybridization event or from multiple events, we first tried to distinguish the chloroplast genomes both within and between diploid Brassica species. We investigated the chloroplast genomes of 30 B. rapa accessions and 17 B. oleracea accessions, and found that the chloroplast genomes of B. rapa are separated into two clades as four broccoletto’s have clearly different chloroplast genomes compared to those of all the other B. rapa accessions. This means that we revealed two different chloroplast genome types in B. rapa, while we only identified one chloroplast genome type in B. oleracea. Interestingly, these four broccoletto accessions group with the other B. rapa accessions in nuclear phylogenies (including the broccoletto with the Clade I chloroplast genome) (Zhao et al., 2005) and so it is puzzling how broccoletto obtained another chloroplast genome. The broccoletto morphotype originates from Italy, where also many wild Brassica species can be found (Hammer et al., 1992), including many wild C9 species, but also B. repanda with n = 10. It is likely that broccoletto has been cultivated alongside wild Brassica species indigenous to Italy, providing the necessary opportunities for inter-specific crosses to occur (Allender and King, 2010). After interspecific crosses with wild Brassica’s as female parent, natural backcrosses may have reverted the nuclear genome to B. rapa broccoletto. The extensive genome synteny between Brassica species (Chalhoub et al., 2014; Liu et al., 2014), may have facilitated the generation of percentages of viable offspring from interspecific crosses, through chromosome pairing of syntenic regions in meiosis. This is in agreement with the hypotheses raised by Allender and King (2010). Another explanation is that, after interspecific crosses between wild Brassica’s and B. rapa, amphidiploids were generated due to spontaneous chromosome doubling of the hybrids (Zhou et al., 2016). Also these amphidiploids may have reverted to the diploid B. rapa genome trough many generations of backcrosses and resulting loss of the cytoplasm donor genome chromosomes (Gupta et al., 2015).
As chloroplasts in Brassica are exclusively maternally inherited (Scott and Wilkinson, 1999), chloroplast phylogenies represent the maternal lineage of the amphidiploid hybrids only, and each amphidiploid species will cluster with its maternal ancestor. The chloroplast genomes of all eight B. juncea accessions were clustering with the chloroplast genomes of B. rapa accessions in subclade ‘I-a’ (Japanese leafy types and turnips, plus one broccoletto), in agreement with the finding that B. rapa is the ancestral maternal parent of amphidiploid B. juncea (Palmer et al., 1983). Chloroplast genomes of B. carinata and B. nigra were grouped in clade IV, indicating that B. carinata has the cytoplasm origin of B. nigra. The fact that B. napus has two chloroplast types (I-e and III), suggests that the studied accessions may have been derived from independent hybridization events involving different female parental accessions, one with a broccoletto and the other event with a sarson as parent. It is not unexpected that B. napus is derived from a cross with a sarson like morphotype, as both are selected for their seeds and oil content. It is likely that both the sarsons and broccoletto would have been cultivated alongside B. oleracea crops such as kales, cabbages, and broccolis (Schery, 1972), providing the necessary opportunities for inter-specific crosses to occur. It is also possible that some B. oleracea hybridized with wild B. rapa as female, which was not sampled in our study, and then transferred cytoplasm to broccoletto through introgression. However, we did not find any amphidiploid hybrids grouped with B. oleracea, demonstrating that B. oleracea is not the donor to any amphidiploid species. This fits with the observation that in interspecific crosses, B. oleracea can only be used as male parent.
Estimations of the age of the Brassica genus in literature are scarce and controversial (Lysak et al., 2005; Cheung et al., 2009; Beilstein et al., 2010; Hohmann et al., 2015). Caution must be taken to choose correct anchoring points in order to produce correct time lines for the species we studied. In this study, we used the time-calibrated divergence time of 23.35 Mya for the radiation of Arabidopsis and Brassica, estimated from chloroplast sequence data involving four fossil constraints (Hohmann et al., 2015) and not of 43 Mya, as was the estimation based on fossil evidence of a Thlaspi primaevum (Brassicaceae, dated at 20.8–29.2 Mya) specimen. The main argumentation to reject the estimation based on the Thlaspi fossil (Wing, 1987; Franzke et al., 2010) is that identification of this genus has been difficult when relying only on morphological characteristics (Mummenhoff et al., 1997; Hohmann et al., 2015).
The calibrated tree presented in Figure 5 clearly depicts an evolutionary scenario with three main periods of speciation, which can be considered a first attempt to date the evolutionary history of the members of the Brassica genus using chloroplast genomes. Previous studies of diversification dates based on nuclear and chloroplast markers estimate that B. rapa and B. oleracea diverged from B. nigra 7.9 Mya (Lysak et al., 2005) or 20 Mya (Arias et al., 2014), respectively, while our data shows the divergence at 13.7 Mya. Furthermore, the speciation of clade III from clade I and clade II 2.98 Mya had never been estimated based on nuclear genomes. At approximately 2.18 Mya, the chloroplast line shared by clade I (B. rapa, B. juncea and B. napus) diverged from clade II (B. oleracea), which is intermediate compared to previous studies estimating that the genomes of B. rapa and B. oleracea diverged from each other 3.7 Mya (Inaba and Nishio, 2002) or 1.37–0.12 Mya (Cheung et al., 2009).
In summary, we conclude that the chloroplast genomes of the six economically important Brassica species are organized in at least four different clades. B. rapa chloroplast genomes display most variation and are represented by both clade I with five subclades and clade III. B. juncea has the chloroplast origin of clade I-a B. rapa’s, while B. carinata has the chloroplast origin of clade IV B. nigra. B. napus has two chloroplast origins, one corresponding to clade I-e (the B. rapa sarsons) and the other one to clade III (a B. rapa broccoletto accession). In addition, we present estimates of divergence time for the different chloroplast genomes. Knowledge of the parental genotypes of the amphidiploid Brassica species is very relevant to design further crop improvement strategies of especially B. napus via interspecific crosses.
Author Contributions
PL extracted DNA, analyzed the data, and drafted the manuscript. FL prepared samples. SjZ, SfZ, HZ, and XW provided advices on our analysis. RS, TB, and GB designed and supervised the work, and contributed to writing the manuscript.
Conflict of Interest Statement
The authors declare that the research was conducted in the absence of any commercial or financial relationships that could be construed as a potential conflict of interest.
Acknowledgments
This work was supported by the National Key Research and Development Program of China (grant no. 2016YFD0100204 to RS). This study was also funded by the Science and Technology Innovation Program of the Chinese Academy of Agricultural Sciences (CAAS-ASTIP-IVFCAAS) and the Chinese Scholarship Council (CSC). Research was carried out in the Key Laboratory of Biology and Genetic Improvement of Horticultural Crops, Ministry of Agriculture, P.R. China and data analyses and manuscript writing were performed at Wageningen UR Plant Breeding.
Supplementary Material
The Supplementary Material for this article can be found online at: http://journal.frontiersin.org/article/10.3389/fpls.2017.00111/full#supplementary-material
FIGURE S1 | K-mer frequency histograms of Brassica species. Chloroplast genomes of six accessions were shown as examples. The two expected peaks for k-mers derived from the chloroplast genome sequences are clearly visible as sharp peaks and noted in this figure.
FIGURE S2 | Variability of chloroplast genomes of three diploid Brassica species represented over the circular map. The two inverted repeat regions (IRa and IRb) separate the large (LSC) and small (SSC) single copy regions, respectively. The colored rings represent the density of SNVs (0–16) and InDels (0–8).
FIGURE S3 | Sequence comparison of the eight chloroplast genomes generated with mVISTA. Gray arrows indicate the position and direction of each gene. Areas colored in blue and red indicate genic and non-coding regions, respectively. Black lines designate regions of sequence identity with B. rapa, using a 50% identity cutoff. Dashed rectangles indicate highly divergent regions of these species compared to B. rapa.
FIGURE S4 | Phylogenetic tree constructed from ML analysis using the whole chloroplast genomes.
TABLE S1 | Data summary of the chloroplast genomes of several Brassica species.
TABLE S2 | SNVs identified in chloroplast genomes of sequenced accessions.
TABLE S3 | InDels identified in chloroplast genomes of sequenced accessions.
TABLE S4 | Genes in the Brassica chloroplast genome containing SNVs and InDels.
Footnotes
References
Akaike, H. (1974). A new look at the statistical model identification. IEEE Trans. Autom. Control 19, 716–723. doi: 10.1109/TAC.1974.1100705
Allender, C. J., and King, G. J. (2010). Origins of the amphiploid species Brassica napus L. investigated by chloroplast and nuclear molecular markers. BMC Plant Biol. 10:54. doi: 10.1186/1471-2229-10-54
Arias, T., Beilstein, M. A., Tang, M., Mckain, M. R., and Pires, J. C. (2014). Diversification times among Brassica (Brassicaceae) crops suggest hybrid formation after 20 million years of divergence. Am. J. Bot. 101, 86–91. doi: 10.3732/ajb.1300312
Beilstein, M. A., Nagalingum, N. S., Clements, M. D., Manchester, S. R., and Mathews, S. (2010). Dated molecular phylogenies indicate a Miocene origin for Arabidopsis thaliana. Proc. Natl. Acad. Sci. U.S.A. 107, 18724–18728. doi: 10.1073/pnas.0909766107
Birky, C. W., Maruyama, T., and Fuerst, P. (1983). An approach to population and evolutionary genetic theory for genes in mitochondria and chloroplasts, and some results. Genetics 103, 513–527.
Carbonell-Caballero, J., Alonso, R., Ibañez, V., Terol, J., Talon, M., and Dopazo, J. (2015). A phylogenetic analysis of 34 chloroplast genomes elucidates the relationships between wild and domestic species within the genus Citrus. Mol. Biol. Evol. 32, 2015–2035. doi: 10.1093/molbev/msv082
Chalhoub, B., Denoeud, F., Liu, S., Parkin, I. A., Tang, H., Wang, X., et al. (2014). Early allopolyploid evolution in the post-Neolithic Brassica napus oilseed genome. Science 345, 950–953. doi: 10.1126/science.1253435
Chen, D.-H., and Ronald, P. (1999). A rapid DNA minipreparation method suitable for AFLP and other PCR applications. Plant Mol. Biol. Rep. 17, 53–57. doi: 10.1023/A:1007585532036
Cheung, F., Trick, M., Drou, N., Lim, Y. P., Park, J.-Y., Kwon, S.-J., et al. (2009). Comparative analysis between homoeologous genome segments of Brassica napus and its progenitor species reveals extensive sequence-level divergence. Plant Cell 21, 1912–1928. doi: 10.1105/tpc.108.060376
Chu, K. H., Qi, J., Yu, Z.-G., and Anh, V. (2004). Origin and phylogeny of chloroplasts revealed by a simple correlation analysis of complete genomes. Mol. Biol. Evol. 21, 200–206. doi: 10.1093/molbev/msh002
Darriba, D., Taboada, G. L., Doallo, R., and Posada, D. (2012). jModelTest 2: more models, new heuristics and parallel computing. Nat. Methods 9, 772–772. doi: 10.1038/nmeth.2109
Drummond, A. J., Suchard, M. A., Xie, D., and Rambaut, A. (2012). Bayesian phylogenetics with BEAUti and the BEAST 1.7. Mol. Biol. Evol. 29, 1969–1973. doi: 10.1093/molbev/mss075
Felsenstein, J. (1988). Phylogenies from molecular sequences: inference and reliability. Annu. Rev. Genet. 22, 521–565. doi: 10.1146/annurev.genet.22.1.521
Franzke, A., Koch, M. A., Couvreur, T. L. P., Lysak, M. A., and Mummenhoff, K. (2010). On the age of the mustard family (Brassicaceae). Nature 467:755.
Frazer, K. A., Pachter, L., Poliakov, A., Rubin, E. M., and Dubchak, I. (2004). VISTA: computational tools for comparative genomics. Nucleic Acids Res. 32, W273–W279. doi: 10.1093/nar/gkh458
Guindon, S., Dufayard, J.-F., Lefort, V., Anisimova, M., Hordijk, W., and Gascuel, O. (2010). New algorithms and methods to estimate maximum-likelihood phylogenies: assessing the performance of PhyML 3.0. Syst. Biol. 59, 307–321. doi: 10.1093/sysbio/syq010
Gupta, M., Gupta, S., Kumar, H., Kumar, N., and Banga, S. (2015). Population structure and breeding value of a new type of Brassica juncea created by combining A and B genomes from related allotetraploids. Theor. Appl. Genet. 128, 221–234. doi: 10.1007/s00122-014-2423-7
Hammer, K., Knüpffer, H., Laghetti, G., and Perrino, P. (1992). Seeds from the Past; a Catalogue of Crop Germplasm in South Italy and Sicily. Bari: Germplasm Institute. Consiglio Nazionale delle Ricerche, 29.
Hao, W., Fan, S., Hua, W., and Wang, H. (2014). Effective extraction and assembly methods for simultaneously obtaining plastid and mitochondrial genomes. PLoS ONE 9:e108291. doi: 10.1371/journal.pone.0108291
Hohmann, N., Wolf, E. M., Lysak, M. A., and Koch, M. A. (2015). A time-calibrated road map of Brassicaceae species radiation and evolutionary history. Plant Cell 27, 2770–2784. doi: 10.1105/tpc.15.00482
Inaba, R., and Nishio, T. (2002). Phylogenetic analysis of Brassiceae based on the nucleotide sequences of the S-locus related gene, SLR1. Theor. Appl. Genet. 105, 1159–1165. doi: 10.1007/s00122-002-0968-3
Jansen, R. K., Cai, Z., Raubeson, L. A., Daniell, H., Leebens-Mack, J., Müller, K. F., et al. (2007). Analysis of 81 genes from 64 plastid genomes resolves relationships in angiosperms and identifies genome-scale evolutionary patterns. Proc. Natl. Acad. Sci. U.S.A. 104, 19369–19374. doi: 10.1073/pnas.0709121104
Katoh, K., Kuma, K.-I., Toh, H., and Miyata, T. (2005). MAFFT version 5: improvement in accuracy of multiple sequence alignment. Nucleic Acids Res. 33, 511–518. doi: 10.1093/nar/gki198
Koch, M. A., Haubold, B., and Mitchell-Olds, T. (2000). Comparative evolutionary analysis of chalcone synthase and alcohol dehydrogenase loci in Arabidopsis, Arabis, and related genera (Brassicaceae). Mol. Biol. Evol. 17, 1483–1498. doi: 10.1093/oxfordjournals.molbev.a026248
Kurtz, S., Phillippy, A., Delcher, A. L., Smoot, M., Shumway, M., Antonescu, C., et al. (2004). Versatile and open software for comparing large genomes. Genome Biol. 5:R12. doi: 10.1186/gb-2004-5-2-r12
Li, H., and Durbin, R. (2010). Fast and accurate long-read alignment with Burrows–Wheeler transform. Bioinformatics 26, 589–595. doi: 10.1093/bioinformatics/btp698
Li, H., Handsaker, B., Wysoker, A., Fennell, T., Ruan, J., Homer, N., et al. (2009). The sequence alignment/map format and SAMtools. Bioinformatics 25, 2078–2079. doi: 10.1093/bioinformatics/btp352
Liu, S., Liu, Y., Yang, X., Tong, C., Edwards, D., Parkin, I. A., et al. (2014). The Brassica oleracea genome reveals the asymmetrical evolution of polyploid genomes. Nat. Commun. 5:3930. doi: 10.1038/ncomms4930
Lohse, M., Drechsel, O., and Bock, R. (2007). OrganellarGenomeDRAW (OGDRAW): a tool for the easy generation of high-quality custom graphical maps of plastid and mitochondrial genomes. Curr. Genet. 52, 267–274. doi: 10.1007/s00294-007-0161-y
Lysak, M. A., Koch, M. A., Pecinka, A., and Schubert, I. (2005). Chromosome triplication found across the tribe Brassiceae. Genome Res. 15, 516–525. doi: 10.1101/gr.3531105
Ma, P.-F., Zhang, Y.-X., Zeng, C.-X., Guo, Z.-H., and Li, D.-Z. (2014). Chloroplast phylogenomic analyses resolve deep-level relationships of an intractable bamboo tribe Arundinarieae (Poaceae). Syst. Biol. 63, 933–950. doi: 10.1093/sysbio/syu054
Mummenhoff, K., Franzke, A., and Koch, M. (1997). Molecular data reveal convergence in fruit characters used in the classification of Thlaspi s.l. (Brassicaceae). Bot. J. Linn. Soc. 125, 183–199. doi: 10.1111/j.1095-8339.1997.tb02253.x
Nagaharu, U. (1935). Genome analysis in Brassica with special reference to the experimental formation of B. napus and peculiar mode of fertilization. Japan J. Bot. 7, 389–452.
Nikiforova, S. V., Cavalieri, D., Velasco, R., and Goremykin, V. (2013). Phylogenetic analysis of 47 chloroplast genomes clarifies the contribution of wild species to the domesticated apple maternal line. Mol. Biol. Evol. 30, 1751–1760. doi: 10.1093/molbev/mst092
Nock, C. J., Waters, D. L., Edwards, M. A., Bowen, S. G., Rice, N., Cordeiro, G. M., et al. (2011). Chloroplast genome sequences from total DNA for plant identification. Plant Biotechnol. J. 9, 328–333. doi: 10.1111/j.1467-7652.2010.00558.x
Palmer, J. D. (1985). Comparative organization of chloroplast genomes. Annu. Rev. Genet. 19, 325–354. doi: 10.1146/annurev.genet.19.1.325
Palmer, J. D., and Herbon, L. A. (1988). Plant mitochondrial DNA evolved rapidly in structure, but slowly in sequence. J. Mol. Evol. 28, 87–97. doi: 10.1007/BF02143500
Palmer, J. D., Shields, C., Cohen, D., and Orton, T. (1983). Chloroplast DNA evolution and the origin of amphidiploid Brassica species. Theor. Appl. Genet. 65, 181–189. doi: 10.1007/BF00308062
Parkin, I. A., Koh, C., Tang, H., Robinson, S. J., Kagale, S., Clarke, W. E., et al. (2014). Transcriptome and methylome profiling reveals relics of genome dominance in the mesopolyploid Brassica oleracea. Genome Biol. 15, 1–18. doi: 10.1186/gb-2014-15-6-r77
Parks, M., Cronn, R., and Liston, A. (2009). Increasing phylogenetic resolution at low taxonomic levels using massively parallel sequencing of chloroplast genomes. BMC Biol. 7:84. doi: 10.1186/1741-7007-7-84
Posada, D., and Crandall, K. A. (1998). Modeltest: testing the model of DNA substitution. Bioinformatics 14, 817–818. doi: 10.1093/bioinformatics/14.9.817
Prabhudas, S. K., Raju, B., Kannan Thodi, S., Parani, M., and Natarajan, P. (2015). The complete chloroplast genome sequence of Indian mustard (Brassica juncea L.). Mitochondrial DNA A DNA Mapp. Seq. Anal. 27, 4622–4623. doi: 10.3109/19401736.2015.1101586
Prakash, S., and Hinata, K. (1980). Taxonomy, cytogenetics and origin of crop Brassicas: a review. Opera Bot. 55, 1–57.
Pyke, K. A. (1999). Plastid division and development. Plant Cell 11, 549–556. doi: 10.1105/tpc.11.4.549
Qiu, D., Morgan, C., Shi, J., Long, Y., Liu, J., Li, R., et al. (2006). A comparative linkage map of oilseed rape and its use for QTL analysis of seed oil and erucic acid content. Theor. Appl. Genet. 114, 67–80. doi: 10.1007/s00122-006-0411-2
Rambaut, A., Suchard, M., Xie, D., and Drummond, A. (2014). Tracer v1. 6. Available at: http://beast.bio.ed.ac.uk/Tracer
Scott, S. E., and Wilkinson, M. J. (1999). Low probability of chloroplast movement from oilseed rape (Brassica napus) into wild Brassica rapa. Nat. Biotechnol. 17, 390–392. doi: 10.1038/7952
Song, K., Osborn, T., and Williams, P. (1988). Brassica taxonomy based on nuclear restriction fragment length polymorphisms (RFLPs): 2. Preliminary analysis of subspecies within B. rapa (syn. campestris) and B. oleracea. Theor. Appl. Genet. 76, 593–600. doi: 10.1007/BF00260914
Song, K., and Osborn, T. C. (1992). Polyphyletic origins of Brassica napus: new evidence based on organelle and nuclear RFLP analyses. Genome 35, 992–1001. doi: 10.1139/g92-152
Song, K., Osborn, T. C., and Williams, P. H. (1990). Brassica taxonomy based on nuclear restriction fragment length polymorphisms (RFLPs). Theor. Appl. Genet. 79, 497–506. doi: 10.1007/BF00226159
Tangphatsornruang, S., Sangsrakru, D., Chanprasert, J., Uthaipaisanwong, P., Yoocha, T., Jomchai, N., et al. (2010). The chloroplast genome sequence of mungbean (Vigna radiata) determined by high-throughput pyrosequencing: structural organization and phylogenetic relationships. DNA Res. 17, 11–22. doi: 10.1093/dnares/dsp025
Wang, X., Wang, H., Wang, J., Sun, R., Wu, J., Liu, S., et al. (2011). The genome of the mesopolyploid crop species Brassica rapa. Nat. Genet. 43, 1035–1039. doi: 10.1038/ng.919
Warwick, S., and Black, L. (1997). Phylogenetic implications of chloroplast DNA restriction site variation in subtribes Raphaninae and Cakilinae (Brassicaceae, tribe Brassiceae). Can. J. Bot. 75, 960–973. doi: 10.1139/b97-107
Warwick, S., Francis, A., and Al-Shehbaz, I. (2006). Brassicaceae: species checklist and database on CD-Rom. Plant Syst. Evol. 259, 249–258. doi: 10.1007/s00606-006-0422-0
Warwick, S. I., and Black, L. D. (1991). Molecular systematics of Brassica and allied genera (subtribe Brassicinae, Brassiceae)—chloroplast genome and cytodeme congruence. Theor. Appl. Genet. 82, 81–92. doi: 10.1007/BF00231281
Wing, S. L. (1987). Eocene and Oligocene floras and vegetation of the Rocky Mountains. Ann. Mo. Bot. Gard. 74, 748–784. doi: 10.2307/2399449
Wolfe, K. H., Li, W.-H., and Sharp, P. M. (1987). Rates of nucleotide substitution vary greatly among plant mitochondrial, chloroplast, and nuclear DNAs. Proc. Natl. Acad. Sci. U.S.A. 84, 9054–9058. doi: 10.1073/pnas.84.24.9054
Wu, J., Liu, B., Cheng, F., Ramchiary, N., Choi, S. R., Lim, Y. P., et al. (2012). Sequencing of chloroplast genome using whole cellular DNA and Solexa sequencing technology. Front. Plant Sci. 3:243. doi: 10.3389/fpls.2012.00243
Wyman, S. K., Jansen, R. K., and Boore, J. L. (2004). Automatic annotation of organellar genomes with DOGMA. Bioinformatics 20, 3252–3255. doi: 10.1093/bioinformatics/bth352
Yang, Y.-W., Lai, K.-N., Tai, P.-Y., and Li, W.-H. (1999). Rates of nucleotide substitution in angiosperm mitochondrial DNA sequences and dates of divergence between Brassica and other angiosperm lineages. J. Mol. Evol. 48, 597–604. doi: 10.1007/PL00006502
Yule, G. U. (1925). A mathematical theory of evolution, based on the conclusions of Dr. JC Willis, FRS. Philos. Trans. R. Soc. B 213, 21–87. doi: 10.1098/rstb.1925.0002
Zhang, T., Zhang, X., Hu, S., and Yu, J. (2011). An efficient procedure for plant organellar genome assembly, based on whole genome data from the 454 GS FLX sequencing platform. Plant Methods 7:38. doi: 10.1186/1746-4811-7-38
Zhao, J., Wang, X., Deng, B., Lou, P., Wu, J., Sun, R., et al. (2005). Genetic relationships within Brassica rapa as inferred from AFLP fingerprints. Theor. Appl. Genet. 110, 1301–1314. doi: 10.1007/s00122-005-1967-y
Keywords: chloroplast genome, assembly, Brassica, phylogeny, variation, divergence
Citation: Li P, Zhang S, Li F, Zhang S, Zhang H, Wang X, Sun R, Bonnema G and Borm TJA (2017) A Phylogenetic Analysis of Chloroplast Genomes Elucidates the Relationships of the Six Economically Important Brassica Species Comprising the Triangle of U. Front. Plant Sci. 8:111. doi: 10.3389/fpls.2017.00111
Received: 17 July 2016; Accepted: 19 January 2017;
Published: 02 February 2017.
Edited by:
Alessio Mengoni, University of Florence, ItalyReviewed by:
Pedro Edson Moreira Guimaraes, Laboratory of Evolution of Genes and Genomes, BrazilMarcial Escudero, University of Seville, Spain
Copyright © 2017 Li, Zhang, Li, Zhang, Zhang, Wang, Sun, Bonnema and Borm. This is an open-access article distributed under the terms of the Creative Commons Attribution License (CC BY). The use, distribution or reproduction in other forums is permitted, provided the original author(s) or licensor are credited and that the original publication in this journal is cited, in accordance with accepted academic practice. No use, distribution or reproduction is permitted which does not comply with these terms.
*Correspondence: Theo J. A. Borm, dGhlby5ib3JtQHd1ci5ubA== Guusje Bonnema, R3V1c2plLkJvbm5lbWFAd3VyLm5s Rifei Sun, c3VucmlmZWlAY2Fhcy5jbg==