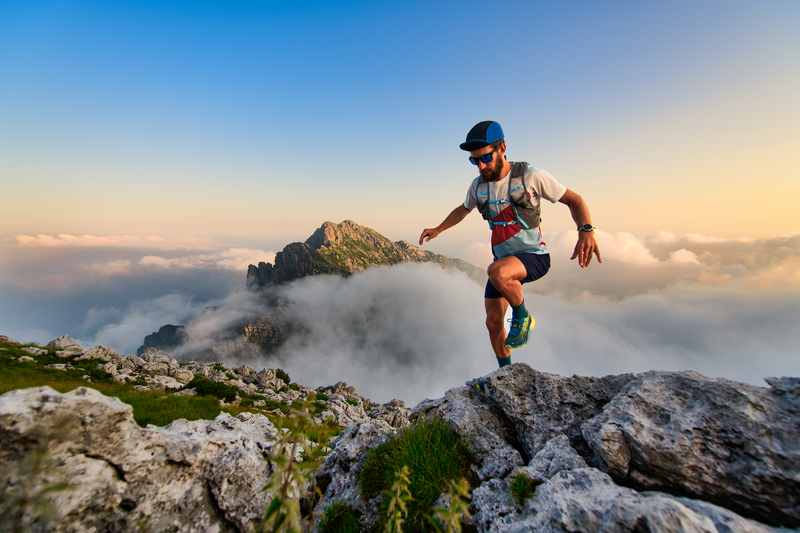
95% of researchers rate our articles as excellent or good
Learn more about the work of our research integrity team to safeguard the quality of each article we publish.
Find out more
ORIGINAL RESEARCH article
Front. Plant Sci. , 09 February 2017
Sec. Plant Pathogen Interactions
Volume 8 - 2017 | https://doi.org/10.3389/fpls.2017.00102
Trichoderma species are soil-borne filamentous fungi widely utilized for their many plant health benefits, such as conferring improved growth, disease resistance and abiotic stress tolerance to their hosts. Many Trichoderma species are able to produce the auxin phytohormone indole-3-acetic acid (IAA), and its production has been suggested to promote root growth. Here we show that the production of IAA is strain dependent and diverse external stimuli are associated with its production. In in vitro assays, Arabidopsis primary root length was negatively affected by the interaction with some Trichoderma strains. In soil experiments, a continuum effect on plant growth was shown and this was also strain dependent. In plate assays, some strains of Trichoderma spp. inhibited the expression of the auxin reporter gene DR5 in Arabidopsis primary roots but not secondary roots. When Trichoderma spp. and A. thaliana were physically separated, enhancement of both shoot and root biomass, increased root production and chlorophyll content were observed, which strongly suggested that volatile production by the fungus influenced the parameters analyzed. Trichoderma strains T. virens Gv29.8, T. atroviride IMI206040, T. sp. “atroviride B” LU132, and T. asperellum LU1370 were demonstrated to promote plant growth through volatile production. However, contrasting differences were observed with LU1370 which had a negative effect on plant growth in soil but a positive effect in plate assays. Altogether our results suggest that the mechanisms and molecules involved in plant growth promotion by Trichoderma spp. are multivariable and are affected by the environmental conditions.
Plant-microbe interactions in the rhizosphere are key determinants of plant health, productivity and soil fertility (Souza et al., 2015). Plant roots synthesize metabolites that are recognized by microorganisms, which in response, produce signals that initiate microbial colonization (Berg, 2009). Plant roots also secrete sucrose as a food source to support colonization by the microorganisms (Druzhinina et al., 2011; Vargas et al., 2011).
The mechanism by which microorganisms promote plant growth has previously been studied for both bacteria (Crozier et al., 1988; Ahmad et al., 2005; Idris et al., 2007) and fungi (Contreras-Cornejo et al., 2009; Salazar-Badillo et al., 2015). It has been suggested that over 80% of rhizosphere bacteria synthesize indole-3-acetic acid (IAA) (Patten and Glick, 1996), the plant hormone auxin that controls many aspects of plant growth and development (Grossmann, 2010). The ability to synthesize indole-3-acetic acid (IAA) is an attribute that many microorganisms possess, including both plant growth-promoters and some plant pathogens (Duca et al., 2014). IAA synthesized by plant root-associated microorganisms can interfere with plant development by disturbing the auxin balance in plants. For example, IAA synthesis can modify root architecture, resulting in increased root mass, and consequently, an increased area suitable for microbial colonization and a larger root system for nutrient uptake by the plant (Spaepen et al., 2007; Berg, 2009; Contreras-Cornejo et al., 2009).
Trichoderma species are common soil-borne filamentous fungi, with some strains capable of establishing beneficial relationships with plants (Harman et al., 2004; Druzhinina et al., 2011; Contreras-Cornejo et al., 2014). Direct plant growth promotion (Rando and Verstrepen, 2007; Richards et al., 2010; Jablonka, 2013) is one of many mechanisms in which Trichoderma spp. enhance plant health, however, the molecular mechanism that underlies this is still unclear. Contreras-Cornejo et al. (2009) suggested that Trichoderma spp. induce growth promotion by a fungal auxin-dependent mechanism. Using in vitro bioassay, they showed that Trichoderma virens Gv29.8 and T. atroviride IMI206040 can synthesize IAA (and some of its derivatives) and have been demonstrated to increase the production of Arabidopsis lateral roots (Contreras-Cornejo et al., 2009). However, the correlation between IAA synthesis and plant growth promotion of plants in soil-based systems is less convincing. Hoyos-Carvajal et al. (2009a) showed that many Trichoderma strains are capable of synthesizing IAA but only a few can promote plant growth.
Other microbially produced molecules known to be important in promoting plant growth are the microbial Volatile Organic Compounds (mVOCs), which have been recognized as key players in plant growth promotion (Vespermann et al., 2007; Minerdi et al., 2011; Zhi-Lin et al., 2012; Paul and Park, 2013; D'Alessandro et al., 2014; Garnica-Vergara et al., 2015; Kanchiswamy et al., 2015; Lee et al., 2015, 2016; Salazar-Badillo et al., 2015). mVOCs are low-molecular weight lipophilic compounds that can easily evaporate at room temperature and pressure, and suggested to play a role in long distance communication between organisms. mVOCs belong to different chemical classes, including mono- and sesquiterpenes, alcohols, ketones, lactones, esters, thioalcohols, thioesters and cyclohexenes (Schenkel et al., 2015). Sesquiterpenes from ectomycorrhizal fungi have been shown to induce lateral root formation in diverse plants such as Populus and A. thaliana (Ditengou et al., 2015). 6-pentyl-2H-pyran-2-one (6-PP) is a compound detected in diverse Trichoderma species, including T. atroviride IMI206040 (Reithner et al., 2005; Stoppacher et al., 2010), T. asperellum (Wickel et al., 2013; Kottb et al., 2015), T. viride (Collins and Halim, 1972), T. harzianum (Claydon et al., 1987), T. koningii (Simon et al., 1988), T. citrinoviride, and T. hamatum (Jeleń et al., 2014), and its production by T. atroviride has been shown to induce lateral root formation in A. thaliana (Garnica-Vergara et al., 2015). Intriguingly not all Trichoderma species synthesize 6-PP (Atanasova et al., 2013) yet most are able to induce plant growth promotion (Kottb et al., 2015) suggesting the correlation is not strong as observed recently by Lee et al. (2016).
The aim of this study is to analyse the effect of diverse Trichoderma strains on plant growth promotion and identify the signals that may be involved. Three well-studied Trichoderma model strains and seven Trichoderma strains previously screened for their ability to promote root growth in willow (Salix x matsudana) (Braithwaite et al., 2009) and Impatiens walleriana (Clouston et al., 2010) were selected for this study using A. thaliana as a host.
The New Zealand Trichoderma strains used in this study were isolated from different locations and chosen for their ability to induce root development in cuttings of commercial ornamental plant species. These strains were sourced from the Microbial Biocontrol Culture Collection (MBCC, Bio-Protection Research Centre, Lincoln University) and represent a range of species as well as strains with previously known biocontrol and growth promotion activity (Supplementary Table 1S). Trichoderma reesei QM6a (Simmons, ATCC® 13631) was provided by The American Type Culture Collection (ATCC). Trichoderma atroviride IMI206040 and T. virens Gv29.8 were kindly provided by Alfredo Herrera-Estrella (Langebio, Mexico) and Charles Kenerley (Texas A&M, USA), respectively. All Trichoderma strains in the MBCC were stored in 25% glycerol at −80°C and were previously identified using morphology and five different genetic markers (tef1α, ACLA1, Calm1, LAS1, and RPB2) under separate research initiatives (Hoyos-Carvajal et al., 2009b; Braithwaite et al., 2016). Strains LU132, LU660 and LU668 were closely related to T. atroviride and were defined as Trichoderma sp. “atroviride B” (Braithwaite et al., 2016).
For all experiments, fungal inoculum consisted of conidia harvested from 7 d old PDA cultures grown at 25°C under a 12/12 light/dark photoperiod. The resulting conidial suspension was filtered through two layers of Miracloth (Merck Millipore) and adjusted to the concentration required for each experiment.
All mutants and transgenic lines are derived from parental Arabidopsis thaliana ecotype Col-0. DR5::GUS (Ulmasov et al., 1997). DR5rev::GFP (Friml et al., 2003), PIN1::PIN1-GFP (Benková et al., 2003), PIN2::PIN2-GFP (Xu and Scheres, 2005), PIN4::PIN4-GFP (Blilou et al., 2005), PIN7::PIN7-GFP (Blilou et al., 2005) and PIN3::PIN3-GFP (Zádníková et al., 2010) transgenic lines were previously described and are listed in Supplementary Table 2S.
Trichoderma strains with plant growth promotion potential were evaluated for their ability to promote growth of the model plant Arabidopsis in sterile soil. Arabidopsis thaliana (Col-0) seeds were surface sterilized in 95% (v/v) ethanol for 5 min and 20% (v/v) bleach for 7 min, washed five times with sterile water, and kept in 2 mL sterile water at 4°C for 2 d to break dormancy and synchronize germination. Seeds were then incubated for 5 d at 25°C under a 16 h light: 8 h dark (16L: 8D) day/night cycle on standard MS medium: 0.2 X MS medium (Murashige and Skoog basal salt mixture, catalog M5524, Sigma-Aldrich) containing 1.0% (w/v) agar (Sigma), then supplemented with 0.6% sucrose and adjusted to pH 7.0 (Lopez-Bucio, personal communication) before being poured into Petri dishes. The resulting seedlings were then transferred to gamma irradiated sterile soil (chemical composition is described in Mendoza-Mendoza et al., 2015) which was previously inoculated with 1 × 106 spores per g soil of Trichoderma spp. (T. trixiae LU297, T. sp. “atroviride B” LU660, T. sp. “atroviride B” LU668, Trichoderma sp. nov LU753, T. sp. “novaeharzianum” LU1328, T. asperellum LU1370, T. atroviride IMI206040). The plants were assessed 4 weeks after planting for survival and the total fresh weight was measured. The experimental design was a complete randomized block with 7 treatments and 1 negative control with 4 replicates, a total of 32 plots. Each plot consisted of 4 pots (50 mm diameter, 50 mm deep) containing a single Arabidopsis plant. The experiment was repeated twice with similar results.
Enhanced growth in the presence of Trichoderma is likely due to changes in the plant root architecture. To investigate how the Trichoderma strains influence root structure, strains were evaluated in an Arabidopsis agar plate bioassay (Contreras-Cornejo et al., 2009). Ten sterilized/stratified seeds were placed on MS agar (section Influence of Trichoderma on Arabidopsis Growth in Sterile Soil) in a row 1.5 cm from the edge of the Petri dish. The Petri dishes were sealed with plastic film to prevent cross-contamination and moisture loss and placed on their edge at an angle of approximately 65° to allow root growth down along the agar surface and upward shoot growth. The seedlings were pre-incubated for 5 d at 25°C under a 16L: 8D day/night cycle (Morange, 2002) and then 1 × 106 Trichoderma spores (strains mentioned in section Influence of Trichoderma on Arabidopsis Growth in Sterile Soil, and T. sp. “atroviride” B LU132; T. virens Gv29.8 and T. reesei QM6a) in a 5 μL aliquot of water were inoculated 5 cm below the growing root tips. Petri dishes were re-sealed with plastic film and incubated for a further 5 d under the same conditions mentioned above. Control plates were inoculated with water only. At the end of the experiment, the length of each primary root was measured and the number of lateral roots counted. The root density was then calculated as a function of lateral root number divided by the primary root length (Contreras-Cornejo et al., 2009). Each Trichoderma strain was assayed in four independent experiments using 10 replicate Petri dishes.
Regulation of plant auxins has been linked to root growth promotion by microorganisms. To investigate if the presence of Trichoderma influences auxin accumulation in the plant, confrontation assays were done using an A. thaliana transgenic line with the auxin responsive DR5 promoter fused to the β-glucuronidase gene (gus) as described by Ulmasov et al. (1997). Assays were conducted as described in section Influence of Trichoderma on Arabidopsis Root Architecture on Agar Plates except A. thaliana DR5::GUS was selected as the plant line. After 5 d interaction with Trichoderma spp. (T. atroviride IMI206040, T. reesei QM6a, T. sp. “atroviride B” LU668, T. virens Gv29.8, T. sp. “atroviride B” LU132, T. sp. “atroviride B” LU660, T. asperellum LU1370) DR5::GUS seedlings were removed and incubated at 37°C overnight in GUS staining solution (50 mM sodium phosphate buffer, pH 7.0, 0.1% Triton X-100, 1.5 mM potassium ferricyanide, 1.5 mM potassium ferrocyanide, and 1.5 mg/mL X-Gluc). After staining, the samples were cleared by incubation with 7% NaOH for 20 min and 60 min with 100% ethanol followed by 60 min hydration with serial ethanol dilutions 70, 60, 40, 20, and 10%. Then the seedlings were incubated for 30 min with 25% glycerol and overnight with 50% glycerol at room temperature. Seedlings were visualized using an Olympus BX51 compound microscope. Images were captured using an Olympus DP70 digital camera system and processed with the software CellF (Olympus). The experiment was repeated once with similar results using 10 replicate plants for each repeat.
For the localization of the different auxin transporters, confrontation assays were performed as described above using T. virens Gv29.8 for inoculum and multiple Arabidopsis transgenic lines. These lines correspond to the marker for auxins DR5::GFP, and different auxin transporters fused to the green fluorescent protein: PIN1::PIN1-GFP, PIN2::PIN2-GFP, PIN4::PIN4-GFP, PIN7::PIN7-GFP and PIN3::PIN3-GFP (Supplementary Table 2S). After 5 d interaction, seedlings were removed from the interaction plate and directly mounted on a slide for observation on an Olympus BX51 compound microscope. Images were captured using an Olympus DP70 digital camera system and processed with the software CellF (Olympus). The experiment was repeated once using 10 replicate plants each time.
Thin layer chromatography (TLC) was used to further investigate the role of auxins in Trichoderma growth promotion. IAA and its derivatives were isolated and identified from different Trichoderma strains (T. sp. “atroviride B” LU660, T. sp. “atroviride B” LU668, T. sp. “novaeharzianum” LU1328, T. asperellum LU1370, T. sp. “atroviride B” LU132, T. atroviride IMI206040, T. virens Gv29.8, and T. reesei QM6a). Trichoderma spp. spores were inoculated at 1 × 106 spores/mL in 100 mL of 0.2 X MS (pH 7.0) in 500 mL flasks and incubated shaking (120 rpm) for 48 h at 25°C. Mycelia were collected by filtration on sterile filter paper (Whatman 3MM) and equally divided before inoculated in either 0.2 × MS (pH 7.0) with or without 10 mM L-Tryptophan (Trp). Flasks were incubated shaking (120 rpm) for an additional 24 and 48 h at 25°C. The supernatant from each culture was then filtered to remove the fungal material. The supernatants were adjusted to pH 3.0 with HCl and indole derivatives extracted from 30 mL of supernatant mixed with 15 mL ethyl acetate, shaking at 200 rpm for 3 h in darkness. The organic phase was recovered and evaporated in a CentriVap Centrifugal Vacuum Concentrator (Labconco). The dry pellet was dissolved in 50 μL of ethyl acetate and 5 μL of each sample was loaded onto a TLC silica gel 60. The TLC silica gel was sprayed evenly with van Urk and Salkowski reagents (1:3) (Ehmann, 1977) and developed by incubation at 90°C for 7 min. The indole derivatives were separated for 45 min using n-hexane:ethyl acetate:isopropanol:glacial acetic acid (40:20:5:1, v/v) as mobile phase (Chung et al., 2003). Indole derivative compounds were identified against commercial standard compounds (L-Tryptophan, Tryptamine hydrochloride, 3-indole acetonitrile, DL-indole-3-lactic acid, indole-3-acetaldehyde, and indole-3-acetic acid) from Sigma-Aldrich; 25 μg of each indole standard was loaded per lane. To compare IAA production under different nutrient conditions, a second experiment was done using PDB instead of MS medium and strains T. sp. “atroviride B” LU132, T. reesei QM6a, T. atroviride IMI206040, and T. virens GV29.8 were assayed. Both assays were repeated once.
The activity of Trichoderma indole-derivatives on Arabidopsis auxin accumulation was assessed using an A. thaliana transgenic line with the auxin responsive DR5 promoter fused to β-glucuronidase gene (gus) as described by Ulmasov et al. (1997). DR5::GUS A. thaliana seeds, were surface sterilized and stratified as described in section Influence of Trichoderma on Arabidopsis Growth in Sterile Soil and individually germinated for 6 d in wells of a 96-microtiter plate containing 100 μL of standard MS broth at 25°C under 16 h light/8 h dark settings. MS broth was then replaced with 100 μL fresh standard MS broth containing 10 μL of ethyl acetate containing the dissolved pellet from supernatant extracts from T. sp. “atroviride B” LU132, T. reesei QM6a, T. atroviride IMI206040, or T. virens GV29.8 grown with or without tryptophan for 48 h (section Effect of Trichoderma on Arabidopsis Auxin Accumulation and Transport Using Transgenic Arabidopsis Lines) and incubated for an additional 24 h. Each treatment was allocated 16 wells. The control for the solvent was ethyl acetate, 10 μg/mL of commercial indole-3-acetic acid (sodium salt, Sigma Aldrich) was used as positive control, and distilled water as the negative control. The seedlings were incubated for 24 h at 25°C under 16 h light/8 h dark settings. Seedlings were removed and GUS staining was conducted as described above in section Effect of Trichoderma on Arabidopsis Auxin Accumulation and Transport Using Transgenic Arabidopsis Lines. Ten replicates per treatment were assessed and the entire experiment was repeated once.
The effect of Trichoderma VOCs on plant growth promotion and chlorophyll content was assessed using a split-plate system. Five seeds of A. thaliana (Col-0) were surface sterilized and stratified as described in section Influence of Trichoderma on Arabidopsis Growth in Sterile Soil and then placed onto one side of a 90 mm diameter double compartment Petri plate (Labserv) containing Standard MS medium. Plates were sealed with plastic film and placed on their edge at an angle of approximately 65° and incubated at 22°C under 16 h light/8 h dark settings. After 7 d, 5 μL of a Trichoderma spore suspension (containing 1 × 106 spores) was inoculated into the opposite compartment then the plates were re-sealed and incubated for additional 7 d. The Trichoderma strains used for this experiment were T. atroviride IMI206040, T. virens Gv29.8, T. sp. “atroviride B” LU132, T. reesei QM6a, and T. asperellum LU1370. Fresh weight of shoots, roots and total biomass (roots + shoots) per plant were measured. Shoots or roots of 10 plants were pooled and biomass was measured (n = 7, corresponding to 70 plants). The weight was divided by the number of plants to express the weight per plant. Relative chlorophyll content was quantified from 10 leaves per treatment. Chlorophyll content from the third leaf was determined using a SPAD 502 Plus Chlorophyll Meter. The SPAD values were converted to chlorophyll using the formula described by Ling et al. (2011) (nmol chlorophyll/cm2 = 0.0105 × 2 + 0.4119x + 0.3810). The experiment was carried out twice with 10 plants measured each time.
The VOC profiles of T. atroviride IMI206040, T. sp. “atroviride B” LU132, T. virens Gv29.8 and T. asperellum LU1370 were analyzed using a Shimadzu GCMS-QP2010 (Shimadzu Corporation, Japan) gas chromatograph - mass spectrometer fitted with a Restek Rxi-5 ms fused silica capillary column (30.0 m × 0.25 mm i.d. × 0.25 μm, Bellefonte, PA, USA) and an auto-sampler for solid phase micro-extraction (SPME). Fungal cultures were grown in Petri dishes on standard MS medium at 25°C and 12 h light/12 h darkness for 4 d. Four mycelial plugs (5 mm diameter) were punched out with a sterile cork borer from the outer growing zone of each Trichoderma colony and placed in an amber glass headspace vial (20 mL) sealed with a screw cap containing a blue PTFE/silicone septum (Sigma-Aldrich, Australia). The vials were incubated at 25°C for 24 h before sampling of the headspace VOCs was conducted according to Stoppacher et al. (2010). An SPME fiber coated with 65 μm polydimethylsiloxane/divinylbenzene was used to extract the fungal VOCs from the headspace vial for 30 min without agitation. After injection, the compounds were desorbed for 2 min in a split/splitless injector at 250°C. The oven temperature was held at 40°C for 2 min, then raised to 200°C at 10°C min−1 and 260°C at 25 min−1 and then held at this temperature for 5 min. Helium was used as carrier gas at a constant flux of 1 mL min−1. Compounds were identified by matching their mass spectra and linear retention indices using GCMS solution v. 2.72 (Shimadzu Corporation, Japan) software with NIST 11 and Wiley 10 mass spectrum libraries and by using the software MassFinder4 with a specialized terpenoids library (Hochmuth Scientific Software, Hamburg, Germany). The background of PDA plates without the fungus was extracted analysing the volatiles of four agar medium plugs. Three biological replicates were analyzed for each strain.
Data analyses from fresh weight of Arabidopsis plants grown in sterile soil (Figure 1), effect of Trichoderma on A. thaliana root architecture (Figure 2), and the effect of Trichoderma volatile organic compounds on Arabidopsis plant biomass using a split-plate assay (Figure 7) were analyzed by Analysis of Variance (ANOVA) using the statistical software GenStat Version 18. Differences between treatment means were compared by the unrestricted least significant difference (LSD) analysis at P < 0.05.
Figure 1. Influence of Trichoderma inoculation on the fresh weight of Arabidopsis thaliana plants grown in sterile soil. (A) Fresh weight of A. thaliana plants after 4 weeks interaction with Trichoderma. (B) Morphology of plant seedlings in co-culture with T. atroviride IMI206040 or T. asperellum LU1370.
Figure 2. Effect of Trichoderma on Arabidopsis thaliana root architecture. The results from two independent experiments are presented. (A) A. thaliana seedlings after 5 d growth in the presence of Trichoderma. (B) Experiment 1—primary root length. (C) Experiment 1—number of emerged lateral roots per plant. (D) Experiment 1—root density. (E) Experiment 2—primary root length. (F) Experiment 2—number of emerged lateral roots per plant. (G) Experiment 2—root density.
Seven Trichoderma strains, chosen for their ability to induce growth promotion in commercial plants, and the reference strain T. atroviride IMI206040, were tested for their ability to promote growth of A. thaliana in sterile soil. After 4 weeks of interaction, three outcomes were evident: an increase in plant biomass, a decrease in biomass, and no effect at all when compared to plants grown in un-inoculated soil. Trichoderma atroviride IMI206040 and T. sp. “atroviride B” LU660 increased Arabidopsis fresh biomass by 72% and 73% respectively, whereas T. asperellum LU1370 significantly inhibited Arabidopsis growth as measured by a reduction of up to 74% (P < 0.05) in biomass with respect to the plants grew in non-inoculated soil. Trichoderma sp. “atroviride B” LU668, T. “novaeharzianum” LU1328, T. trixiae LU297, and Trichoderma spp. LU753 had no significant effect on plant growth (Figure 1).
The influence of Trichoderma inoculation on root architecture was investigated in more detail on MS agar. In the absence of Trichoderma spp., A. thaliana produced long primary roots with few secondary roots and consequently exhibited low root density (Figure 2). When the plates were inoculated with Trichoderma, the primary root length decreased by 13 to 25% and the number of secondary roots increased by 64 to 90%, which in turn resulted in a massive increase in root density (Figures 2B–D). To corroborate if the reduction of primary root length was a common phenomenon during the Trichoderma interaction, additional strains belonging to three different species were added to our analyses: T. virens Gv29.8, which has previously analyzed for their ability to induce A. thaliana secondary roots on MS medium (Contreras-Cornejo et al., 2009), T. sp. “atroviride B” LU132, a commercial biocontrol strain with plant growth promotion capability (Maag et al., 2014), and T. reesei QM6a, a commercial strain used for its capacity to secrete large amounts of cellulases and hemicellulases (Peterson and Nevalainen, 2012; Mukherjee et al., 2013; Strakowska et al., 2014) (Figures 2E–G). Two of these strains, T. sp. “atroviride B” LU132 and T. virens Gv29.8, induced a reduction in primary root length and an increase in the number of secondary roots, while T. reesei QM6a did not induce significant changes with respect to the control (Figures 2E–G). Interestingly, Trichoderma spp. LU753 did not significantly alter root architecture on MS plates even though it was capable of increasing root biomass in willow cuttings (Braithwaite et al., 2009). In trials on ornamental cuttings conducted on commercial nurseries, LU753 did not promote root biomass when applied alone but was effective when applied as part of an effective mixture which included two other Trichoderma strains (M. Braithwaite, pers. comm.) and T. sp. “atroviride B” LU660 promoted lateral root growth on A. thaliana in the plate bioassays (Figures 2B–D) but had no effect on root promotion in the willow screening trial.
A reddening of the leaves was observed for the majority of the Trichoderma treatments indicating strong anthocyanin production. Two strains (T. sp. “novaeharzianum” LU1328 and T. reesei QM6a) did not induce anthocyanin production (Supplementary Figure 1S).
The influence of Trichoderma spp. in the induction of auxins was assessed using DR5::GUS plant seedlings and Trichoderma strains on MS plates. DR5: GUS activity was profoundly visible in the primary and secondary root tips in the control seedlings (Figure 3). Unlike the control, GUS was weakly expressed in the primary root tip when A. thaliana DR5::GUS line interacted with T. atroviride IMI206040 and T. reesei QM6a. No GUS activity was observed in the primary roots with the remaining strains (Figure 3, PR). When secondary roots from A. thaliana DR5::GUS were analyzed, greater activity was observed in seedlings interacting with T. virens Gv29.8, T. sp. “atroviride B” LU668 and LU660, in comparison to the control and other Trichoderma strains (Figure 3, SR). Unexpectedly, T. asperellum LU1370 completely induced an inhibition of DR5 expression in secondary roots (Figure 3) but induced lateral root promotion on plates (Figure 2).
Figure 3. Histochemical analysis of GUS activity driven by the DR5 promoter in the Arabidopsis DR5::GUS line after 5 d growth in the presence of Trichoderma. DR5::GUS expression levels in the Primary root (PR) and Secondary root (SR) tips. Scale bar, 100 μm.
Although auxin is synthesized in the plant apices of shoots and roots, it is transported throughout the plant via the phloem, forming concentration gradients and accumulating in different tissues (Friml et al., 2003). PIN proteins operate as efflux carriers for auxin localization (Petrásek et al., 2006). These proteins are polarly localized in plants, including A. thaliana (Chen et al., 1998; Luschnig et al., 1998; Müller et al., 1998; Shin et al., 2005). To assess if polarity localization of PIN proteins is altered in A. thaliana by the interaction with T. virens Gv29.8, reporter lines of A. thaliana containing different PIN proteins fused to GFP were used. Expression of GFP modulated by DR5 was inhibited by the presence of T. virens, in contrast to the control where the typical localized GFP signal was observed (Figure 4). When the localization of PIN transporters in the primary roots (PIN1::PIN1:GFP, PIN2::PIN2:GFP, PIN3::PIN3:GFP, and PIN7::PIN7:GFP) were analyzed on MS medium, specific localization occurred in the different lines; however in the presence of the fungus, expression of PIN2 and PIN3 was completely inhibited and a diffuse localization of PIN1 and PIN7 was observed (Figure 4).
Figure 4. GFP expression in Arabidopsis DR5REV::GFP and PIN transporter lines after 5 d growth in the presence of Trichoderma virens Gv29.8. DIC = differential interference contrast. Fluo = fluorescence microscopy.
Changes in growth promotion activity by Trichoderma species on Arabidopsis plantlets may depend on their capacity to produce auxins. Therefore as a first step to investigate the role of auxins indole derivatives from Trichoderma were extracted and separated on TLC plates. On standard MS medium two T. sp. “atroviride B” strains (LU668 and LU132), T. reesei QM6a and T. sp. “novaeharzianum” LU1328 synthesized a compound that migrated to the same Rf as the commercial 3-indole acetic acid (IAA) (Figure 5). Interestingly, four strains did not produce IAA on MS standard medium (T. atroviride IMI206040, T. virens Gv29.8, T. sp. “atroviride B” LU660 and T. asperellum LU1370). In the absence of Trp, no production of indole derivatives was observed, with the exception of T. virens which produced a compound that migrated to the same Rf as IAA but with a different color; this was present but less evident when Trp was added (Figure 5A). Additionally T. virens synthesized additional compounds in standard MS independently of the presence of Trp.
Figure 5. Indoles derivatives recovered from supernatants of Trichoderma strains incubated with (+) or without (−) 10 mM of L-Tryptophan. (A) Basal medium was MS. The standards used for identification of indole derivatives are indicated in the first 6 lanes. Samples incubated for 48 h with or without tryptophan are presented. (B) Basal medium was PDB. IAA was used as a standard in the first and last lane.
Four strains from the first experiment were used to assess the IAA production in PDB: T. atroviride IMI206040, T. virens Gv29.8, T. sp. “atroviride B” LU132, and T. reesei QM6a. All strains were able to synthesize a molecule that migrated to the same Rf as commercial IAA (Figures 5A,B).
Strains T. sp. “atroviride B” LU132, T. reesei QM6a, T. atroviride IMI206040 and T. virens GV29.8 were selected for further analysis on the basis of their variable pattern on the TLC plates. Culture supernatants were assessed for their bioactivity on auxin accumulation in the reporter line A. thaliana DR5::GUS. Arabidopsis seedlings exhibited significantly more GUS activity after a 12-h treatment with 5 μM of commercial 3-indol-acetic acid than the control seedlings (Figure 6A). For the supernatants produced in MS medium, only those generated from T. reesei QM6a in the presence of tryptophan resulted in a clear increase in auxin accumulation in both the leaves and roots (Figures 6B,C). All culture supernatants produced in PDB in the presence of tryptophan resulted in a clear increase in auxin accumulation in both leaves and roots (Figures 6B,C).
Figure 6. Effect of indole-derivatives on DR5::GUS expression in 5-day-old A. thaliana seedlings. (A) DR5::GUS expression in leaves and primary roots after treatment with ethyl acetate (control) or 1 μM indole-3-acetic acid (IAA) for 12 h. (B) DR5::GUS expression in leaves inoculated with 10 μL of supernatant from Trichoderma grown in MS supplemented or not with 10 mM Tryptophan. (C) DR5::GUS expression in leaves inoculated with 10 μL of supernatant from Trichoderma grown in PDB supplemented or not with 10 mM Tryptophan.
To assess the effect of mVOCs emitted by Trichoderma in growth promotion, co-cultivation experiments were done using a split-plate assay to keep both organisms physically separated. After 7 days of co-cultivation VOCs emitted by four Trichoderma strains (T. sp. “atroviride B” LU132 and IMI206040; T. virens Gv29.8 and T. asperellum LU1370) showed a significant increase in shoot, root and total biomass compared with the control seedlings (Figure 7). mVOCs emitted by T. reesei QM6a had no effect in increasing biomass in both shoots and roots with respect to the control.
Figure 7. Effect of Trichoderma volatile organic compounds on Arabidopsis plant biomass using a split-plate assay. (A) Morphology of 14-day old seedlings co-inoculated or not with Trichoderma spp. in double compartment plates. A close-up of roots morphology from each interaction is illustrated in right panel. Scale bar corresponds to 0.5 mm. (B) Shoot biomass. (C) Root biomass. (D) Total biomass. (E) Magnified view of leaves exposed to Trichoderma spp. volatiles. Scale bar corresponds 0.5 mm. (F) Chlorophyll content of leaves exposed to Trichoderma spp. volatiles.
Some VOCs emitted by microbes increase the efficiency of photosynthesis and chlorophyll content in A. thaliana by modulating the sugar content (Zhang et al., 2008). In this study, a clear difference in color was observed in leaves exposed to Trichoderma in comparison to the controls, with the exception of T. reesei QM6a which showed no difference (Figure 7E). Analysis of the chlorophyll content supported these observations; all strains except T. reesei QM6a induced a significant increase in chlorophyll content (Figure 7F).
The mVOC profiles of the four strains identified as promoting growth in the absence of physical contact (section Growth Response of A. thaliana to Trichoderma spp. Volatile Organic Compounds (VOCs).) were analyzed by GCMS. Trichoderma sp. “atroviride B” LU132 and T. atroviride IMI206040 differed in emission of mVOCs when grown on standard MS medium; T. sp. “atroviride B” LU132 produced 6-PP in large quantities whereas T. atroviride IMI206040 emitted only minor quantities of this compound but higher quantities of 1-Octen-3-ol and 3-Octanone (Tables 1, 2). For T. virens Gv29.8, 29 sesquiterpenes were identified and of these β-Elemene and ε-Amorphene were significantly overrepresented in the mixture (Table 3). Of the mVOCs emitted by T. asperellum LU1370, 1,3-Octadiene, Limonene and β-Eudesmol + Valerianol were the most abundant in the mixture (Table 4).
In nature, multiple symbiotic relationships form between plants and microorganisms and these relationships can be manipulated to improve plant productivity. One such trait is plant growth promotion. It has been reported that several species of Trichoderma have the ability to induce growth promotion on diverse crop plants regardless of the place from which they were isolated (Harman et al., 2004; Mastouri et al., 2010; Lee et al., 2016). The Trichoderma strains tested in this study were collected from both free-living and plant symbiotic sources and, as has been reported, there appeared to be no correlation between isolation source and the ability to promote plant growth. However, our work clearly demonstrates that growth promotion is not a universal trait of all Trichoderma. Similar to that observed by Lee et al. (2016), we observed promotion, no effect at all, and detrimental effects on plants. Further, the variability in plant growth promoting potential appears to be more strongly influenced by environmental parameters than the choice of plant host. This work highlights the need for greater research into the environmental control of successful plant health enhancement.
Detailed biochemical studies on growth promotion, by necessity, require the use of in vitro systems, however the choice of system can directly affect the outcome and will not necessarily reflect how the microorganism behaves in nature. Originally isolated from healthy Hydrangea roots, when tested in sterile soil in this study, T. asperellum LU1370 significantly reduced Arabidopsis fresh weight. Interestingly, this strain also significantly reduced the survival of Lophomyrtus cuttings by 40% (data not shown) and had no effect on the growth parameters of tomato seedlings (M Braithwaite, unpublished data.). However, when T. asperellum LU1370 was tested on agar plates, a positive effect on Arabidopsis growth was observed.
The inhibitory effects of Trichoderma on plants have previously been reported, particularly with respect to seed germination on diverse plants including lettuce, onion and chicory (Ousley et al., 1993; Celar and Valic, 2005). Trichoderma spp. synthesize a plethora of secondary metabolites with diverse biological function in plants (Reino et al., 2008). For example, trichosetin, a secondary metabolite isolated from dual cultures of T. harzianum-Catharanthus roseus callus (Marfori et al., 2002), inhibited root and shoot growth in five plant species (Oryza sativa, Vigna radiate, Medicago sativa, Capsicum frutescens, and Lycopersicon esculentum) by damaging the cell membrane (Marfori et al., 2003). Roots treated with trichosetin were mostly dead, indicating a clear phytotoxic effect of this compound (Marfori et al., 2003). Additional compounds with negative effects on plant growth include Trichocaranes (A, B, and C) (Macías et al., 2000), konionginins (B, C, E, and G) (Cutler et al., 1989; Parker et al., 1995), cyclonerodiol (Cutler et al., 1991), and a laevorotatory form of harzianopyridone (Cutler and Jacyno, 1991). The last compound caused necrosis in bean, tobacco and corn in a concentration dependent manner (Cutler and Jacyno, 1991). Trichoderma virens also synthesizes negative plant growth promoters such as viridiol, a potent herbicidal compound, which is effective for weed control (Héraux et al., 2005).
The biosynthesis of secondary metabolites is modulated by environmental conditions. The general effect of T. asperellum LU1370 on plant growth in soil was an inhibition in plant size without causing plant death, by contrast, on plate assays the general effect was stimulatory, suggesting that either different molecules are produced in both environments (soil and plates) or the proportion of specific metabolites are differently accumulated in these conditions. The dual effect of specific metabolites have been documented before, for example, 6-pentyl- 2H-pyran-2-one (6-PP) induces plant growth promotion, depending on the concentration (Garnica-Vergara et al., 2015). At high concentrations 6-PP has an inhibitory effect and at low concentrations it promoted growth in wheat seedlings (Vinale et al., 2008). However, Lee et al. (2016) recently reported no correlation between 6-PP and growth promotion. Trichoderma asperellum IsmT5 synthesizes 6-PP (Kottb et al., 2015) but we did not identify this molecule in T. asperellum LU1370, although it may be induced by the plant as was recently reported for T. atroviride (Garnica-Vergara et al., 2015). The role of 6-PP in the strains presented in this study is unknown.
It is known that some microorganisms have the ability to produce phytohormones like IAA (Robinson et al., 1998; Contreras-Cornejo et al., 2011; Hilbert et al., 2012; Fu et al., 2015).
In bacteria, indole acetic acid (IAA) biosynthesis varies significantly depending on the media used (Spaepen et al., 2007). In fungi, the most well-known mechanism of IAA biosynthesis is tryptophan-dependent, and consequently the addition of this amino acid to the media results in the production of higher levels of IAA (Robinson et al., 1998; Chung et al., 2003; Spaepen and Vanderleyden, 2011; Hilbert et al., 2012). An example of how media determine the production of IAA is shown in Figure 5 where T. virens and T. atroviride synthesize IAA in PDB supplemented with L-Tryptophan but not in MS supplemented with the same amino acid. The ambient pH was different between the PDB and MS media, pH 5.8 ± 0.2 and pH 7.0 respectively. MS media used in this study contained 0.6% sucrose while PDB formulation contained 2% glucose (dextrose), these differences may have an impact on the mechanisms of synthesis of IAA. In fungi, environmental factors, including pH and temperature have an impact on IAA biosynthesis (Yu et al., 1970; Strzelczyk et al., 1992; Bose et al., 2013; Sun et al., 2014). Carbon and nitrogen sources have been shown to be essential factors influencing fungal and bacterial IAA biosynthesis (Yurekli et al., 2003; Shokri and Emtiazi, 2010); whether they play an intrinsic role in fungal IAA production remains to be explored.
By comparing MS medium supplemented with or without L-tryptophan, Salas-Marina et al. (2011) reported a ~2-fold increase of IAA derivatives secreted by T. atroviride IMI206040 and T. virens Gv298. This does not contradict to findings because the authors reported a total amount of indole derivatives using the Salkowski's reagent, which is a colorimetric method to detect general indole substances (Perley and Stowe, 1966). In this study, T. atroviride IMI206040 secreted multiple indole derivatives, however, the Rf differed from the commercial IAA standard. Furthermore, the indole derivatives induced in MS from T. atroviride IMI206040 and T. virens Gv29.8 did not display auxin activity. Further experimental work is required to understand the mechanisms of IAA synthesis by Trichoderma and how this molecule impacts plant physiology.
Currently IAA is thought to be a diffusible signal involved in interspecies communication (Fu et al., 2015); however, the final outcome of this communication is dependent on the organisms interacting and is also influenced by the environment where the interaction occurs. For example, while plant growth promotion induced by T. virens Gv29.8 and T. atroviride IMI206040 has been related to their ability to secrete IAA (Contreras-Cornejo et al., 2009), some other studies have observed no relation between IAA production and growth induction, at least when plants are tested in soil. Hoyos-Carvajal et al. (2009a) identified that of 101 Trichoderma strains collected from Colombia, 60% produced IAA-derivatives in vitro but only 18% had the ability to induce plant growth promotion in beans. In the present study, some Trichoderma strains produced significant quantities of IAA in vitro and also increased Arabidopsis seedling shoot fresh weight in soil experiments, providing a possible link between the two systems. However, the link between IAA production and plant growth promotion was not consistent for all strains. For instance, in the in vitro agar plate tests, five of the Trichoderma strains were similar to T. atroviride IMI206040 and T. virens Gv29.8 with respect to increases in lateral root growth and root density, but not all isolates produce IAA in liquid MS supplemented with L-Trp. A similar lack of correlation between growth promotion and 6-PP production was recently observed in Trichodema (Lee et al., 2016).
Several authors have reported that bacteria, microalgae, fungi, and plants exchange IAA as a signaling molecule that affects their physiology (Fu et al., 2015). The rice blast fungus Magnaporthe oryzae synthesizes IAA specifically in the area of the infection hyphae (Tanaka et al., 2011), but it is not clear whether this occurs to manipulate the host plant or to benefit its own self. Furthermore the endophytic fungus Piriformospora indica synthesizes IAA not for growth promotion but for plant colonization (Hilbert et al., 2012), suggesting that additional mechanisms must exist for this process. Further molecular studies on Trichoderma are required to understand the mechanisms of synthesis in the fungus and how this molecule affect the interaction with plant roots.
Altogether, our findings suggest that IAA production seems not to be a key determinant for plant growth promotion. However, in this stage we cannot discard the possibility that plants are triggering IAA production in Trichoderma as occurs in other microorganisms including Xanthomonas axonopodis (Costacurta et al., 1998) and Magnaporthe oryzae (Fu et al., 2015).
Cell polarity has been crucial for evolution of multicellular organisms (Offringa and Kleine-Vehn, 2013) and is one of the fundamental aspects of development (Dhonukshe, 2009). In plants, PIN proteins are integral membrane proteins implicated in the directional efflux of IAA (Luschnig and Vert, 2014). These proteins can alter their localization from one cell to another by developmental and environmental conditions (Friml et al., 2002, 2003, 2004; Kleine-Vehn et al., 2008). Our results showed that Trichoderma spp. inhibit primary root elongation and increase lateral root numbers (This study; Salazar-Badillo et al., 2015). Our results showed that in the primary tip, Trichoderma promotes a reduction in the accumulation of DR5::GUS and DR5::GFP expression, indicating a reduction of DR5 activity (Figures 3, 4). When the T. virens Gv29.8 and Arabidopsis interaction was analyzed, we observed decreased expression or delocalization of auxin PIN receptors. This reduction in the accumulation of PIN receptors might be the cause of the reduction of A. thaliana root length in the interaction with Trichoderma. Recently it was reported that Trichokonin VI (TK VI), a peptaibol produced by T. longibrachiatum SMF2, inhibits primary root growth on Arabidopsis by suppressing cell division and cell elongation, and disrupting root stem cell niche maintenance (Shi et al., 2016). Contreras-Cornejo et al. (2015) recently reported that the mitogen-activated protein kinase 6 (MPK6) has an important role in the induction of root length during the interaction with T. atroviride. Mutants of the mpk6 have an enhanced root inhibition when interacting with Trichoderma. The fact that Trichoderma spp. strongly activates DR5 in the secondary roots also suggests that additional molecules that work at distance might be involved, such as volatile organic compounds emitted by the fungi as was recently reported in T. atroviride (Garnica-Vergara et al., 2015).
Microbial volatile organic compounds have been described as important inducers of growth promotion in bacteria and fungi, including mycorrhizae and Trichoderma (Garnica-Vergara et al., 2015). The mechanisms behind microbial-induced plant growth promotion are complex, and, in the case of the strains discussed in the present work, may involve IAA but not as a key determinant. Although their exact mechanism is not known, the results from this study clearly demonstrate that VOCs are able to actively promote plant growth in the absence of physical fungus-plant contact. Four out of five strains tested in this study were able to increase plant biomass and chlorophyll content when physically separated from the plant. However, the wide and varied array of VOCs produced by the various strains suggests that non-contact induction may be due to a number of diverse compounds and mechanisms. Garnica-Vergara et al. (2015) reported that 6-pentyl-2H-pyran-2-one (6-PP) from T. atroviride is a key element in growth promotion. Interestingly, Garnica-Vergara et al. (2015) observed that higher concentrations of the pyrone induce a reduction of the primary root length. However, the concentrations used can be far from those observed in natural conditions. Furthermore, Lee et al. (2016) reported no correlation between 6-PP production and growth promotion.
In our study, we observed an atonishing diversity of VOCs produced by different strains, suggesting the existence of diverse mechanisms of induction. However, we cannot exclude the possibility of and influence of CO2 in plant growth promotion as has been suggested before (Ramadan et al., 2015), although Garnica-Vergara et al. (2015), and Kottb et al. (2015) discarded this possibility by using pure compounds instead of the fungi. In addition, Lee et al. (2016) recently reported that the amount of CO2 generated by Trichoderma spp. is insufficient to induce Arabidopsis growth promotion and the effects observed are attributable to VOCs synthesized by Trichoderma spp.
In summary, this study provides evidence that the communication between plants and Trichoderma involves the recognition of fungal-derived molecules such us auxins and VOCs, however this communication is strongly dependent on the environment. Many studies have been conducted on the effect of Trichoderma metabolites. However, there is a lack of strong evidence of a correlation between growth promotion and the production of a particular compound, suggesting a complex interplay of molecules is required. Future molecular studies examining the roles of specific genes and pathways may shed further light on the role of fungal-derived molecules in promotion of plant growth.
MFNJ, FBSB, DVN, MR, MB, JMS, MO, and AMM performed experimental work; MFNJ, AMM, JFJB, MB, and MR designed the experiments; MFNJ, AMM, FBSB, JFJB, MB, and JMS discussed and interpreted the results; AMM and AS designed the research, contributed to chemicals, and scientific advice; MFNJ, JMS, and AMM wrote the paper. All authors reviewed the final version of the paper.
This work was supported by the Pre-Seed Accelerator Fund (PSAF), the Tertiary Education Commission (contracts 38631 and 38651) and the Ministry for Science and Innovation. Fatima Salazar was supported by Tertiary Education Commission and Conacyt-Mexico for her stay at Lincoln University. JT acknowledges the financial support of CNPq and CAPES.
The authors declare that the research was conducted in the absence of any commercial or financial relationships that could be construed as a potential conflict of interest.
We acknowledges Jiri Friml (IST, Austria) for kindly providing us with seeds of fluorescent transgenic lines of A. thaliana; Alfredo Herrera-Estrella (Langebio, Mexico) and Charles Kenerley (Texas A&M, USA) for having provided us with Trichoderma atroviride IMI206040 and T. virens Gv28.9, respectively.
The Supplementary Material for this article can be found online at: http://journal.frontiersin.org/article/10.3389/fpls.2017.00102/full#supplementary-material
Ahmad, F., Ahmad, I., and Khan, M. S. (2005). Indole acetic acid production by the indigenous isolates of Azotobacter and fluorescent Pseudomonas in the presence and absence of tryptophan. Turkish J. Biol. 29, 29–34.
Atanasova, L., Le Crom, S., Gruber, S., Coulpier, F., Seidl-Seiboth, V., Kubicek, C. P., et al. (2013). Comparative transcriptomics reveals different strategies of Trichoderma mycoparasitism. BMC Genomics 14:121. doi: 10.1186/1471-2164-14-121
Benková, E., Michniewicz, M., Sauer, M., Teichmann, T., Seifertová, D., Jürgens, G., et al. (2003). Local, efflux-dependent auxin gradients as a common module for plant organ formation. Cell 115, 591–602. doi: 10.1016/S0092-8674(03)00924-3
Berg, G. (2009). Plant-microbe interactions promoting plant growth and health: perspectives for controlled use of microorganisms in agriculture. Appl. Microbiol. Biotechnol. 84, 11–18. doi: 10.1007/s00253-009-2092-7
Blilou, I., Xu, J., Wildwater, M., Willemsen, V., Paponov, I., Friml, J., et al. (2005). The PIN auxin efflux facilitator network controls growth and patterning in Arabidopsis roots. Nature 433, 39–44. doi: 10.1038/nature03184
Bose, A., Shah, D., and Keharia, H. (2013). Production of indole-3-acetic-acid (IAA) by the white rot fungus Pleurotus ostreatus under submerged condition of Jatropha seedcake. Mycology 4, 103–111. doi: 10.1080/21501203.2013.823891
Braithwaite, M., Johnston, P. R., Ball, S. L., Nourozi, F., Hay, A. J., Shoukouhi, P., et al. (2016). Trichoderma down under: species diversity and occurrence of Trichoderma in New Zealand. Australas. Plant Pathol. doi: 10.1007/s13313-016-0457-9. [Epub ahead of print].
Braithwaite, M., Minchin, R., Hill, R.A., and Stewart, A. (2009). “A bioassay to screen Trichoderma isolates for their ability to promote root growth in willow,” in Australasia Plant Pathology: Plant Health Management: An Integrated Approach, Australasia Plant Pathology Society Conference of APPS (Newcastle, NSW), 103.
Celar, F., and Valic, N. (2005). Effects of Trichoderma spp. and Gliocladium roseum culture filtrates on seed germination of vegetables and maize. Zeitschrift Fur Pflanzenkrankheiten Und Pflanzenschutz-J. Plant Dis. Prot. 112, 343–350.
Chen, R., Hilson, P., Sedbrook, J., Rosen, E., Caspar, T., and Masson, P. H. (1998). The Arabidopsis thaliana AGRAVITROPIC 1 gene encodes a component of the polar-auxin-transport efflux carrier. Proc. Natl. Acad. Sci. U.S.A. 95, 15112–15117. doi: 10.1073/pnas.95.25.15112
Chung, K. R., Shilts, T., Ertürk, U., Timmer, L. W., and Ueng, P. P. (2003). Indole derivatives produced by the fungus Colletotrichum acutatum causing lime anthracnose and postbloom fruit drop of citrus. FEMS Microbiol. Lett. 226, 23–30. doi: 10.1016/S0378-1097(03)00605-0
Claydon, N., Allan, M., Hanson, J., and Avent, A. (1987). Antifungal alkyl pyrones of Trichoderma harzianum. Trans. Br. Mycol. Soc. 88, 503–513. doi: 10.1016/S0007-1536(87)80034-7
Clouston, A., Hill, R., Minchin, R., Braithwaite, M., and Stewart, A. (2010). A bioassay screening Trichoderma isolates for enhancement of root development in Impatiens walleriana cuttings. N. Z. Plant Prot. 63, 33–38.
Collins, R. P., and Halim, A. F. (1972). Characterization of the major aroma constituent of the fungus Trichoderma viride. J. Agric. Food Chem. 20, 437–438. doi: 10.1021/jf60180a010
Contreras-Cornejo, H. A., López-Bucio, J. S., Méndez-Bravo, A., Macías-Rodríguez, L., Ramos-Vega, M., Guevara-García, A. A., et al. (2015). Mitogen-activated protein kinase 6 and ethylene and auxin signaling pathways are involved in Arabidopsis root-system architecture alterations by Trichoderma atroviride. Mol. Plant-Microbe Interact. 28, 701–710. doi: 10.1094/MPMI-01-15-0005-R
Contreras-Cornejo, H. A., Macías-Rodríguez, L., Alfaro-Cuevas, R., and López-Bucio, J. (2014). Trichoderma spp. improve growth of Arabidopsis seedlings under salt stress through enhanced root development, osmolite production, and Na(+) elimination through root exudates. Mol. Plant-Microbe Interact. 27, 503–514. doi: 10.1094/MPMI-09-13-0265-R
Contreras-Cornejo, H. A., Macías-Rodríguez, L., Beltrán-Peña, E., Herrera-Estrella, A., and López-Bucio, J. (2011). Trichoderma-induced plant immunity likely involves both hormonal- and camalexin-dependent mechanisms in Arabidopsis thaliana and confers resistance against necrotrophicfungi Botrytis cinerea. Plant Signal. Behav. 6, 1554–1563. doi: 10.4161/psb.6.10.17443
Contreras-Cornejo, H. A., Macías-Rodríguez, L., Cortés-Penagos, C., and López-Bucio, J. (2009). Trichoderma virens, a plant beneficial fungus, enhances biomass production and promotes lateral root growth through an auxin-dependent mechanism in Arabidopsis. Plant Physiol. 149, 1579–1592. doi: 10.1104/pp.108.130369
Costacurta, A., Mazzafera, P., and Rosato, Y. (1998). Indole-3-acetic acid biosynthesis by Xanthomonas axonopodis pv. citri is increased in the presence of plant leaf extracts. FEMS Microbiol. Lett. 159, 215–220.
Crozier, A., Arruda, P., Jasmim, J. M., Monteiro, A. M., and Sandberg, G. (1988). Analysis of indole-3-acetic-acid and related indoles in culture-medium from Azospirillum-lipoferum and Azospirillum-brasilense. Appl. Environ. Microbiol. 54, 2833–2837.
Cutler, H. G., Himmelsbach, D. S., Arrendale, R. F., Cole, P. D., and Cox, R. H. (1989). Koninginin A: a novel plant growth regulator from Trichoderma koningii. Agric. Biol. Chem. Tokyo 53, 2605–2611. doi: 10.1271/bbb1961.53.2605
Cutler, H. G., and Jacyno, J. M. (1991). Biological activity of (-)-Harzianopyridone isolated from Trichoderma harzianum. Agric. Biol. Chem. Tokyo 55, 2629–2631. doi: 10.1271/bbb1961.55.2629
Cutler, H. G., Jacyno, J. M., Phillips, R. S., VonTersch, R. L., Cole, P. D., and Montemurro, N. (1991). Cyclonerodiol from a novel source, Trichoderma koningii: plant growth regulatory activity. Agric. Biol. Chem. Tokyo 55, 243–244.
D'Alessandro, M., Erb, M., Ton, J., Brandenburg, A., Karlen, D., Zopfi, J., et al. (2014). Volatiles produced by soil-borne endophytic bacteria increase plant pathogen resistance and affect tritrophic interactions. Plant Cell Environ. 37, 813–826. doi: 10.1111/pce.12220
Dhonukshe, P. (2009). Cell polarity in plants: linking PIN polarity generation mechanisms to morphogenic auxin gradients. Commun. Integr. Biol. 2, 184–190.
Ditengou, F. A., Müller, A., Rosenkranz, M., Felten, J., Lasok, H., van Doorn, M. M., et al. (2015). Volatile signalling by sesquiterpenes from ectomycorrhizal fungi reprogrammes root architecture. Nat. Commun. 6, 6279. doi: 10.1038/ncomms7279
Druzhinina, I. S., Seidl-Seiboth, V., Herrera-Estrella, A., Horwitz, B. A., Kenerley, C. M., Monte, E., et al. (2011). Trichoderma: the genomics of opportunistic success. Nat. Rev. Microbiol. 9, 896–896. doi: 10.1038/nrmicro2689
Duca, D., Lorv, J., Patten, C. L., Rose, D., and Glick, B. R. (2014). Indole-3-acetic acid in plant-microbe interactions. A. Van Leeuw. 106, 85–125. doi: 10.1007/s10482-013-0095-y
Ehmann, A. (1977). The van URK-Salkowski reagent—a sensitive and specific chromogenic reagent for silica gel thin-layer chromatographic detection and identification of indole derivatives. J. Chromatogr. 132, 267–276. doi: 10.1016/S0021-9673(00)89300-0
Friml, J., Vieten, A., Sauer, M., Weijers, D., Schwarz, H., Hamann, T., et al. (2003). Efflux-dependent auxin gradients establish the apical-basal axis of Arabidopsis. Nature 426, 147–153. doi: 10.1038/nature02085
Friml, J., Wisniewska, J., Benková, E., Mendgen, K., and Palme, K. (2002). Lateral relocation of auxin efflux regulator PIN3 mediates tropism in Arabidopsis. Nature 415, 806–809. doi: 10.1038/415806a
Friml, J., Yang, X., Michniewicz, M., Weijers, D., Quint, A., Tietz, O., et al. (2004). A PINOID-dependent binary switch in apical-basal PIN polar targeting directs auxin efflux. Science, 306, 862–865. doi: 10.1126/science.1100618
Fu, S. F., Wei, J. Y., Chen, H. W., Liu, Y. Y., Lu, H. Y., and Chou, J. Y. (2015). Indole-3-acetic acid: a widespread physiological code in interactions of fungi with other organisms. Plant Signal. Behav. 10:e1048052. doi: 10.1080/15592324.2015.1048052
Garnica-Vergara, A., Barrera-Ortiz, S., Muñoz-Parra, E., Raya-González, J., Méndez-Bravo, A., Macias-Rodriguez, L., et al. (2015). The volatile 6-pentyl-2H-pyran-2-one from Trichoderma atroviride regulates Arabidopsis thaliana root morphogenesis via auxin signaling and ETHYLENE INSENSITIVE 2 functioning. New Phytol. 209, 1496–1512. doi: 10.1111/nph.13725
Grossmann, K. (2010). Auxin herbicides: current status of mechanism and mode of action. Pest Manag. Sci. 66, 113–120. doi: 10.1002/ps.1860
Harman, G. E., Howell, C. R., Viterbo, A., Chet, I., and Lorito, M. (2004). Trichoderma species - Opportunistic, avirulent plant symbionts. Nat. Rev. Microbiol. 2, 43–56. doi: 10.1038/nrmicro797
Héraux, F. M. G., Hallett, S. G., Ragothama, K. G., and Weller, S. C. (2005). Composted Chicken Manure as a medium for the production and delivery of Trichoderma virens for weed control. HortScience 40, 1394–1397.
Hilbert, M., Voll, L. M., Ding, Y., Hofmann, J., Sharma, M., and Zuccaro, A. (2012). Indole derivative production by the root endophyte Piriformospora indica is not required for growth promotion but for biotrophic colonization of barley roots. New Phytol. 196, 520–534. doi: 10.1111/j.1469-8137.2012.04275.x
Hoyos-Carvajal, L., Orduz, S., and Bissett, J. (2009a). Growth stimulation in bean (Phaseolus vulgaris L.) by Trichoderma. Biol. Control 51, 409–416. doi: 10.1016/j.biocontrol.2009.07.018
Hoyos-Carvajal, L., Orduz, S., and Bissett, J. (2009b). Genetic and metabolic biodiversity of Trichoderma from Colombia and adjacent neotropic regions. Fungal Genet. Biol. 46, 615–631. doi: 10.1016/j.fgb.2009.04.006
Idris, E. E., Iglesias, D. J., Talon, M., and Borriss, R. (2007). Tryptophan-dependent production of indole-3-acetic acid (IAA) affects level of plant growth promotion by Bacillus amyloliquefaciens FZB42. Mol. Plant-Microbe Interact. 20, 619–626. doi: 10.1094/MPMI-20-6-0619
Jablonka, E. (2013). Epigenetic inheritance and plasticity: The responsive germline. Prog. Biophys. Mol. Biol. 111, 99–107. doi: 10.1016/j.pbiomolbio.2012.08.014
Jeleń, H., Błaszczyk, L., Chełkowski, J., Rogowicz, K., and Strakowska, J. (2014). Formation of 6-n-pentyl-2H-pyran-2-one (6-PAP) and other volatiles by different Trichoderma species. Mycol. Prog. 13, 589–600. doi: 10.1007/s11557-013-0942-2
Kanchiswamy, C. N., Malnoy, M., and Maffei, M. E. (2015). Chemical diversity of microbial volatiles and their potential for plant growth and productivity. Front. Plant Sci. 6:151. doi: 10.3389/fpls.2015.00151
Kleine-Vehn, J., Dhonukshe, P., Sauer, M., Brewer, P. B., Wisniewska, J., Paciorek, T., et al. (2008). ARF GEF-dependent transcytosis and polar delivery of PIN auxin carriers in Arabidopsis. Curr. Biol. 18, 526–531. doi: 10.1016/j.cub.2008.03.021
Kottb, M., Gigolashvili, T., Grosskinsky, D. K., and Piechulla, B. (2015). Trichoderma volatiles effecting Arabidopsis: from inhibition to protection against phytopathogenic fungi. Front. Microbiol. 6:995. doi: 10.3389/fmicb.2015.00995
Lee, S., Hung, R., Yap, M., and Bennett, J. W. (2015). Age matters: the effects of volatile organic compounds emitted by Trichoderma atroviride on plant growth. Arch. Microbiol. 197, 723–727. doi: 10.1007/s00203-015-1104-5
Lee, S., Yap, M., Behringer, G., Hung, R., and Bennett, J. W. (2016). Volatile organic compounds emitted by Trichoderma species mediate plant growth. Fungal Biol. Biotechnol. 3:7. doi: 10.1186/s40694-016-0025-7
Ling, Q., Huang, W., and Jarvis, P. (2011). Use of a SPAD-502 meter to measure leaf chlorophyll concentration in Arabidopsis thaliana. Photosyn. Res. 107, 209–214. doi: 10.1007/s11120-010-9606-0
Luschnig, C., Gaxiola, R. A., Grisafi, P., and Fink, G. R. (1998). EIR1, a root-specific protein involved in auxin transport, is required for gravitropism in Arabidopsis thaliana. Genes Dev. 12, 2175–2187. doi: 10.1101/gad.12.14.2175
Luschnig, C., and Vert, G. (2014). The dynamics of plant plasma membrane proteins: PINs and beyond. Development 141, 2924–2938. doi: 10.1242/dev.103424
Maag, D., Kandula, D. R., Müller, C., Mendoza-Mendoza, A., Wratten, S. D., Stewart, A., et al. (2014). Trichoderma atroviride LU132 promotes plant growth but not induced systemic resistance to Plutella xylostella in oilseed rape. BioControl 59, 241–252. doi: 10.1007/s10526-013-9554-7
Macías, F. A., Varela, R. M., Simonet, A. M., Cutler, H. G., Cutler, S. J., Eden, M. A., et al. (2000). Bioactive carotanes from Trichoderma virens. J. Nat. Prod. 63, 1197–1200. doi: 10.1021/np000121c
Marfori, E. C., Kajiyama, S., Fukusaki, E., and Kobayashi, A. (2002). Trichosetin, a novel tetramic acid antibiotic produced in dual culture of Trichoderma harzianum and Catharanthus roseus callus. Zeitschrift fur Naturforschung C J. Biosci. 57, 465–470. doi: 10.1515/znc-2002-5-611
Marfori, E. C., Kajiyama, S., Fukusaki, E., and Kobayashi, A. (2003). Phytotoxicity of the tetramic acid metabolite trichosetin. Phytochemistry 62, 715–772. doi: 10.1016/S0031-9422(02)00629-5
Mastouri, F., Björkman, T., and Harman, G. E. (2010). Seed treatment with Trichoderma harzianum alleviates biotic, abiotic, and physiological stresses in germinating seeds and seedlings. Phytopathology 100, 1213–1221. doi: 10.1094/PHYTO-03-10-0091
Mendoza-Mendoza, A., Steyaert, J. M., Nieto-Jacobo, M. F., Holyoake, A., Braithwaite, M., and Stewart, A. (2015). Identification of growth stage molecular markers in Trichoderma sp. ‘atroviride type B’ and their potential application in monitoring fungal growth and development in soil. Microbiology 161, 2110–2126. doi: 10.1099/mic.0.000167
Minerdi, D., Bossi, S., Maffei, M. E., Gullino, M. L., and Garibaldi, A. (2011). Fusarium oxysporum and its bacterial consortium promote lettuce growth and expansin A5 gene expression through microbial volatile organic compound (MVOC) emission. FEMS Microbiol. Ecol. 76, 342–351. doi: 10.1111/j.1574-6941.2011.01051.x
Morange, M. (2002). The relations between genetics and epigenetics: a historical point of view. Ann. N. Y. Acad. Sci. 981, 50–60. doi: 10.1111/j.1749-6632.2002.tb04911.x
Mukherjee, P. K., Horwitz, B. A., Herrera-Estrella, A., Schmoll, M., and Kenerley, C. M. (2013). Trichoderma research in the genome era. Annu. Rev. Phytopathol. 51, 105–129. doi: 10.1146/annurev-phyto-082712-102353
Müller, A., Guan, C., Gälweiler, L., Tänzler, P., Huijser, P., Marchant, A., et al. (1998). AtPIN2 defines a locus of Arabidopsis for root gravitropism control. EMBO J. 17, 6903–6911. doi: 10.1093/emboj/17.23.6903
Offringa, R., and Kleine-Vehn, J. (2013). Cell polarity and development. J. Integr. Plant Biol. 55, 786–788. doi: 10.1111/jipb.12099
Ousley, M. A., Lynch, J. M., and Whipps, J. M. (1993). Effect of Trichoderma on plant-growth - a balance between inhibition and growth promotion. Microb. Ecol. 26, 277–285. doi: 10.1007/BF00176959
Parker, S. R., Cutler, H. G., and Schreiner, P. R. (1995). Koninginin C: a biologically active natural product from Trichoderma koningii. Biosci. Biotechnol. Biochem. 59, 1126–1127. doi: 10.1271/bbb.59.1126
Patten, C. L., and Glick, B. R. (1996). Bacterial biosynthesis of indole-3-acetic acid. Can. J. Microbiol. 42, 207–220. doi: 10.1139/m96-032
Paul, D., and Park, K. S. (2013). Identification of volatiles produced by Cladosporium cladosporioides CL-1, a fungal biocontrol agent that promotes plant growth. Sensors (Basel). 13, 13969–13977. doi: 10.3390/s131013969
Perley, J. E., and Stowe, B. B. (1966). An improvement in the sensitivity of the salkowski reagent for tryptamine, tryptophan and indoleacetic acid. Physiol. Plant 19, 683–690. doi: 10.1111/j.1399-3054.1966.tb07053.x
Peterson, R., and Nevalainen, H. (2012). Trichoderma reesei RUT-C30–thirty years of strain improvement. Microbiology 158(Pt 1), 58–68. doi: 10.1099/mic.0.054031-0
Petrásek, J., Mravec, J., Bouchard, R., Blakeslee, J. J., Abas, M., Seifertová, D., et al. (2006). PIN proteins perform a rate-limiting function in cellular auxin efflux. Science 312, 914–918. doi: 10.1126/science.1123542
Ramadan, M., Gigolashvili, T., Großkinsky, D. K., and Piechulla, B. (2015). Trichoderma volatiles effecting Arabidopsis: from inhibition to protection against phytopathogenic fungi. Front. Microbiol. 6:995. doi: 10.3389/fmicb.2015.00995
Rando, O. J., and Verstrepen, K. J. (2007). Timescales of genetic and epigenetic inheritance. Cell 128, 655–668. doi: 10.1016/j.cell.2007.01.023
Reino, J. L., Guerrero, R. F., Hernández-Galán, R., and Collado, I. G. (2008). Secondary metabolites from species of the biocontrol agent Trichoderma. Phytochem. Rev. 7, 89–123. doi: 10.1007/s11101-006-9032-2
Reithner, B., Brunner, K., Schuhmacher, R., Peissl, I., Seidl, V., Krska, R., et al. (2005). The G protein α subunit Tga1 of Trichoderma atroviride is involved in chitinase formation and differential production of antifungal metabolites. Fungal Genet. Biol. 42, 749–760. doi: 10.1016/j.fgb.2005.04.009
Richards, C. L., Bossdorf, O., and Pigliucci, M. (2010). What role does heritable Epigenetic variation play in phenotypic evolution? Bioscience 60, 232–237. doi: 10.1525/bio.2010.60.3.9
Robinson, M., Riov, J., and Sharon, A. (1998). Indole-3-acetic acid biosynthesis in Colletotrichum gloeosporioides f. sp. aeschynomene. Appl. Environ. Microbiol. 64, 5030–5032.
Salas-Marina, M., Silva-Flores, M., Uresti-Rivera, E., Castro-Longoria, E., Herrera-Estrella, A., and Casas-Flores, S. (2011). Colonization of Arabidopsis roots by Trichoderma atroviride promotes growth and enhances systemic disease resistance through jasmonic acid/ethylene and salicylic acid pathways. Eur. J. Plant Pathol. 131, 15–26. doi: 10.1007/s10658-011-9782-6
Salazar-Badillo, F. B., Sánchez-Rangel, D., Becerra-Flora, A., López-Gómez, M., Nieto-Jacobo, F., Mendoza-Mendoza, A., et al. (2015). Arabidopsis thaliana polyamine content is modified by the interaction with different Trichoderma species. Plant Physiol. Biochem. 95, 49–56. doi: 10.1016/j.plaphy.2015.07.003
Schenkel, D., Lemfack, M. C., Piechulla, B., and Splivallo, R. (2015). A meta-analysis approach for assessing the diversity and specificity of belowground root and microbial volatiles. Front. Plant Sci. 6:707. doi: 10.3389/fpls.2015.00707
Shi, W.-L., Chen, X.-L., Wang, L.-X., Gong, Z.-T., Li, S., Li, C.-L., et al. (2016). Cellular and molecular insight into the inhibition of primary root growth of Arabidopsis induced by peptaibols, a class of linear peptide antibiotics mainly produced by Trichoderma spp. J. Exp. Bot. 67, 2191–2205. doi: 10.1093/jxb/erw023
Shin, H., Shin, H.-S., Guo, Z., Blancaflor, E. B., Masson, P. H., and Chen, R. (2005). Complex regulation of Arabidopsis AGR1/PIN2-mediated root gravitropic response and basipetal auxin transport by cantharidin-sensitive protein phosphatases. Plant J. 42, 188–200. doi: 10.1111/j.1365-313X.2005.02369.x
Shokri, D., and Emtiazi, G. (2010). Indole-3-Acetic Acid (IAA) production in symbiotic and non-symbiotic nitrogen-fixing bacteria and its optimization by Taguchi design. Curr. Microbiol. 61, 217–225. doi: 10.1007/s00284-010-9600-y
Simon, A., Dunlop, R., Ghisalberti, E., and Sivasithamparam, K. (1988). Trichoderma koningii produces a pyrone compound with antibiotic properties. Soil Biol. Biochem. 20, 263–264. doi: 10.1016/0038-0717(88)90050-8
Souza, R., Ambrosini, A., and Passaglia, L. M. (2015). Plant growth-promoting bacteria as inoculants in agricultural soils. Genet. Mol. Biol. 38, 401–419. doi: 10.1590/S1415-475738420150053
Spaepen, S., and Vanderleyden, J. (2011). Auxin and plant-microbe interactions. Cold Spring Harb Perspect. Biol. 3:a001438. doi: 10.1101/cshperspect.a001438
Spaepen, S., Vanderleyden, J., and Remans, R. (2007). Indole-3-acetic acid in microbial and microorganism-plant signaling. FEMS Microbiol. Rev. 31, 425–448. doi: 10.1111/j.1574-6976.2007.00072.x
Stoppacher, N., Kluger, B., Zeilinger, S., Krska, R., and Schuhmacher, R. (2010). Identification and profiling of volatile metabolites of the biocontrol fungus Trichoderma atroviride by HS-SPME-GC-MS. J. Microbiol. Methods 81, 187–193. doi: 10.1016/j.mimet.2010.03.011
Strakowska, J., Blaszczyk, L., and Chelkowski, J. (2014). The significance of cellulolytic enzymes produced by Trichoderma in opportunistic lifestyle of this fungus. J. Basic Microbiol. 54, S2–S13. doi: 10.1002/jobm.201300821
Strzelczyk, E., Kampert, M., and Pachlewski, R. A. (1992). The effect of pH on production of plant growth regulators by mycorrhizal fungi. Symbiosis 14, 201–215.
Sun, P. F., Fang, W. T., Shin, L. Y., Wei, J. Y., Fu, S. F., and Chou, J. Y. (2014). Indole-3-acetic acid-producing yeasts in the phyllosphere of the carnivorous plant Drosera indica L. PLoS ONE 9:e114196. doi: 10.1371/journal.pone.0114196
Tanaka, E., Koga, H., Mori, M., and Mori, M. (2011). Auxin production by the rice blast fungus and its localization in host tissue. J. Phytopathol. 159, 522–530. doi: 10.1111/j.1439-0434.2011.01799.x
Ulmasov, T., Murfett, J., Hagen, G., and Guilfoyle, T. J. (1997). Aux/IAA proteins repress expression of reporter genes containing natural and highly active synthetic auxin response elements. Plant Cell 9, 1963–1971. doi: 10.1105/tpc.9.11.1963
Vargas, W. A., Crutcher, F. K., and Kenerley, C. M. (2011). Functional characterisation of a plant-like sucrose transporter from the beneficial fungus Trichoderma virens. Regulation of the symbiotic association with plants by sucrose metabolism inside the fungal cells. New Phytol. 189, 777–789. doi: 10.1111/j.1469-8137.2010.03517.x
Vespermann, A., Kai, M., and Piechulla, B. (2007). Rhizobacterial volatiles affect the growth of fungi and Arabidopsis thaliana. Appl. Environ. Microbiol. 73, 5639–5641. doi: 10.1128/AEM.01078-07
Vinale, F., Sivasithamparam, K., Ghisalberti, E. L., Marra, R., Barbetti, M. J., Li, H., et al. (2008). A novel role for Trichoderma secondary metabolites in the interactions with plants. Physiol. Mol. Plant Pathol. 72, 80–86. doi: 10.1016/j.pmpp.2008.05.005
Wickel, S. M., Citron, C. A., and Dickschat, J. S. (2013). 2H-Pyran-2-ones from Trichoderma viride and Trichoderma asperellum. Eur. J. Org. Chem. 2013, 2906–2913. doi: 10.1002/ejoc.201300049
Xu, J., and Scheres, B. (2005). Dissection of Arabidopsis ADP-RIBOSYLATION FACTOR 1 function in epidermal cell polarity. Plant Cell 17, 525–536. doi: 10.1105/tpc.104.028449
Yu, P. H., Chen, C.-C., and Wu, L. (1970). Production of indoleacetic acid by Nectria pterospermi saw. Bot. Bull. Acad. Sin. 11, 98–104.
Yurekli, F., Geckil, H., and Topcuoglu, F. (2003). The synthesis of indole-3-acetic acid by the industrially important white-rot fungus Lentinus sajor-caju under different culture conditions. Mycol. Res. 107, 305–309. doi: 10.1017/S0953756203007391
Zádníková, P., Petrásek, J., Marhavy, P., Raz, V., Vandenbussche, F., Ding, Z., et al. (2010). Role of PIN-mediated auxin efflux in apical hook development of Arabidopsis thaliana. Development 137, 607–617. doi: 10.1242/dev.041277
Zhang, H., Xie, X., Kim, M.-S., Kornyeyev, D. A., Holaday, S., and Paré, P. W. (2008). Soil bacteria augment Arabidopsis photosynthesis by decreasing glucose sensing and abscisic acid levels in planta. Plant J. 56, 264–273. doi: 10.1111/j.1365-313X.2008.03593.x
Keywords: Trichoderma, auxins, 3-indole-acetic acid, plant growth promotion, volatile organic compounds, 6-PP
Citation: Nieto-Jacobo MF, Steyaert JM, Salazar-Badillo FB, Nguyen DV, Rostás M, Braithwaite M, De Souza JT, Jimenez-Bremont JF, Ohkura M, Stewart A and Mendoza-Mendoza A (2017) Environmental Growth Conditions of Trichoderma spp. Affects Indole Acetic Acid Derivatives, Volatile Organic Compounds, and Plant Growth Promotion. Front. Plant Sci. 8:102. doi: 10.3389/fpls.2017.00102
Received: 28 November 2016; Accepted: 18 January 2017;
Published: 09 February 2017.
Edited by:
Essaid Ait Barka, University of Reims Champagne-Ardenne, FranceReviewed by:
Masoomeh Shams-Ghahfarokhi, Tarbiat Modares University, IranCopyright © 2017 Nieto-Jacobo, Steyaert, Salazar-Badillo, Nguyen, Rostás, Braithwaite, De Souza, Jimenez-Bremont, Ohkura, Stewart and Mendoza-Mendoza. This is an open-access article distributed under the terms of the Creative Commons Attribution License (CC BY). The use, distribution or reproduction in other forums is permitted, provided the original author(s) or licensor are credited and that the original publication in this journal is cited, in accordance with accepted academic practice. No use, distribution or reproduction is permitted which does not comply with these terms.
*Correspondence: Artemio Mendoza-Mendoza, YXJ0ZW1pby5tZW5kb3phQGxpbmNvbG4uYWMubno=
†Present Address: Mark Braithwaite, Plant Diagnostics Limited, Templeton, Christchurch, New Zealand
In Memoriam: This paper is dedicated to the memory of the late Annabel Clouston.
Disclaimer: All claims expressed in this article are solely those of the authors and do not necessarily represent those of their affiliated organizations, or those of the publisher, the editors and the reviewers. Any product that may be evaluated in this article or claim that may be made by its manufacturer is not guaranteed or endorsed by the publisher.
Research integrity at Frontiers
Learn more about the work of our research integrity team to safeguard the quality of each article we publish.