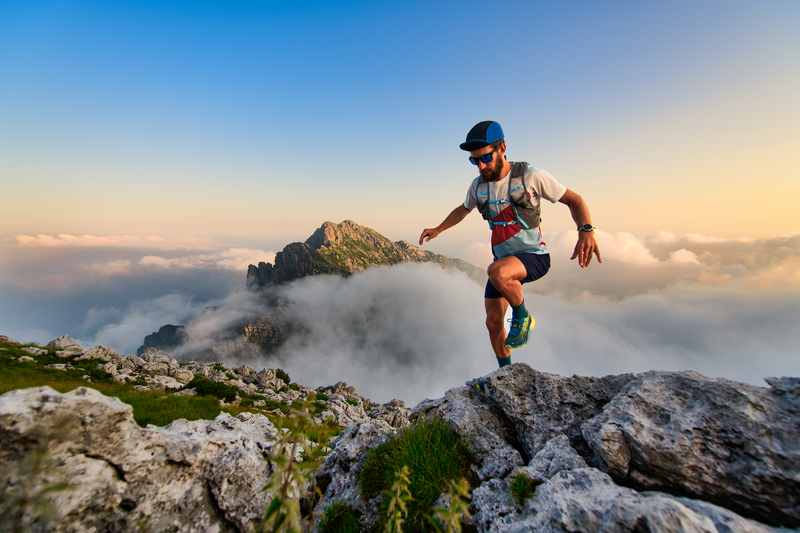
94% of researchers rate our articles as excellent or good
Learn more about the work of our research integrity team to safeguard the quality of each article we publish.
Find out more
HYPOTHESIS AND THEORY article
Front. Plant Sci. , 03 February 2017
Sec. Plant Breeding
Volume 8 - 2017 | https://doi.org/10.3389/fpls.2017.00074
The common assumption in potato virus epidemiology is that all daughter tubers produced by plants coming from infected mother tubers (secondary infection) will become infected via systemic translocation of the virus during growth. We hypothesize that depending on the prevalent environmental conditions, only a portion of the daughter tubers of a plant that is secondarily infected by viruses may become infected. To test this hypothesis experimental data from standardized field experiments were produced in three contrasting environments at 112, 3280, and 4000 m a.s.l. in Peru during two growing seasons. In these experiments, the percentage of infected daughter tubers produced by seed tubers that were infected with either potato potexvirus X (PVX), potato Andean mottle comovirus (APMoV), potato potyvirus Y (PVY) (jointly infected with PVX) or potato leafroll luteovirus (PLRV) was determined. Incomplete autoinfection was found in all cases, as the percentage of virus infected daughter tubers harvested from secondarily infected plants was invariably less than 100%, with the lowest percentage of infection being 30%. Changing the growing site to higher altitudes decreased autoinfection for all viruses. Therefore, the assumption of complete autoinfection of secondarily infected plants were rejected, while the hypothesis of environmentally dependent incomplete autoinfection was accepted. The findings help explain the occurrence of traditional seed management practices in the Andes and may help to develop locally adapted seed systems in environments of the world that have no steady access to healthy seed tubers coming from a formally certified seed system. The results obtained almost three decades ago are discussed in light of most recent knowledge on epigenetic regulation of host plant – virus interactions which allow for speculating about the underlying biological principles of the incomplete autoinfection. A research roadmap is proposed for achieving explicit experimental proof for the epigenetic regulation of incomplete autoinfection in the pathosystem under study.
Potato (Solanum tuberosum L.) seed tubers used for planting can harbor latent pathogens that subsequently reduce emergence, plant vigor, crop quality and/or yield. Since infected tuber seed is an important source from which these pathogens spread, the proportion of such tubers in a tuber seed lot can accumulate with each consecutive generation, resulting in degeneration of seed quality (Thomas-Sharma et al., 2016). Pathogens of many types are known to infect potato seed, including viroids, viruses, phytoplasmas, bacteria, fungi, and oomycetes (Stevenson, 2001). Historically, seed-borne pathogens have been dealt with in several ways, including selection of better looking mother plants and/or tubers for seed, or acquiring seed from areas known to produce a cleaner product (Young, 1990). For example, in the Andes there is a long-standing practice of producing seed in the cooler highlands and even of moving seed to the highlands to make it more vigorous (Thiele, 1999; De Haan and Thiele, 2003). Propagation systems for pathogen-free seed tubers have been developed that provide growers in industrialized countries with a readily available source of healthy planting stock (Frost et al., 2013). Progress toward developing similar pathogen-free seed propagation systems in the small-holder potato growing regions of the low-income countries has been slow and represents a major production limitation (Thiele, 1999; Thomas-Sharma et al., 2016). Typically, small-holder farmers in low-income countries still produce their own seed or acquire it locally from other farmers, and this seed represents many field generations that have never passed through a process of pathogen elimination.
The Andean region is the origin of the cultivated potato and represents an interesting case for studying seed degeneration. Studies done in traditional Andean potato seed systems over the past 30 years found considerably less than 100% of tubers infected with potato viruses. This was true for viruses that are transmitted by contact (potato potexvirus X (PVX), potato Andean mottle comovirus (APMoV)) or vectored by aphids [potato potyvirus Y (PVY) and potato leafroll luteovirus (PLRV)] (Bertschinger et al., 1990b; Fankhauser, 2000; Haan, 2009; Pérez Barrera et al., 2015). In these studies, PVX, which singly does not cause severe yield loss, was frequently found in relatively high incidence, while PVY and PLRV, which can cause severe yield loss, were generally rare (Bertschinger et al., 1996; Scheidegger et al., 1996; Pérez Barrera et al., 2015).
A potato virus disseminates by infecting during the current growing season a healthy plant subsequently producing infected daughter tubers which disseminate the virus further if they are used as mother tuber, i.e., seed tuber for the plantings of the next season. The terms of primary and secondary infection are commonly used in potato virus epidemiology for the two infection types since many years (see e.g., Beemster, 1967; Hooker, 1982). Primarily virus infected plants produce daughter tubers a proportion of which is usually virus infected (see e.g., Beemster, 1967). If a potato seed tuber (mother tuber) is virus infected, the plant grown from this tuber becomes systemically, so-called secondarily infected, i.e., the pathogen moves through the plant vascular system and eventually reaches, i.e., infects, the daughter tuber tissue, produced by this same plant. While several terms have been used for systemic infection of vegetatively propagated “seed,” such as “autoliberation” for potato viruses (Bertschinger et al., 1990a) or “reversion” (Pacumbaba, 1985), “recovery” (Fargette et al., 1994, 1996) or “self-elimination” (Jennings, 1960; Rossel et al., 1988) for cassava viruses, the common principle behind represents “autoinfection” as defined by Robinson (1976). This is an infection in which the donor (infector) host individual is the same as the recipient (infected) host individual. The term describes a general principle of plant pathogen propagation, which is also applicable to systemic virus infection of the potato daughter tubers produced by an infected mother tuber. The term autoinfection clearly represents in a single word without further descriptive expressions the biological situation of daughter tubers produced by secondarily infected plants, which become themselves systemically infected. This is why we subsequently continue to use this term.
Incomplete systemic infection of daughter tubers from mother tubers infected by mop-top furovirus (PMTV) or tobacco rattle tobravirus (TRV) was reported some 40 years ago (Cooper et al., 1976; Engsbro, 1976). This was recently confirmed with new data for mop-top furovirus (Carnegie et al., 2010; Davey et al., 2014). However, these reports are restricted to few potato-growing zones of the world. There have also been not widely diffused reports that autoinfection may be incomplete also with other more common potato viruses (e.g., Baldauf, 2008). But it is yet commonly held that all daughter tubers of secondarily infected plants will become infected, as explicitely stated in official technical guidance documents (Department of Agriculture and Food, 2016), or implicitly referred to it by not precisely distinguishing between primarily and secondarily infected plants and with what is meant with “infected” (Hooker, 1982; Jayasinghe, 1988).
Considering the limited access to clean-seed systems in the Andes, incidence of systemically infecting seed-borne pathogens such as viruses would be expected to become high over many successive generations with primary and secondary infections and on-site propagation. With 100% autoinfection a modest level of within-season primary disease spread and random seed selection, the overall infection incidence in a tuber progeny should rise successively with every generation to eventually reach high levels, potentially 100%, unless some factors are acting to limit the infection process, or reduce infection incidence once it has occurred. Thus, the low incidence of some major viruses found in Andean potato varieties would support the hypothesis that autoinfection from secondarily infected mother plants to daughter tubers must be incomplete under these circumstances. This incomplete autoinfection could be because not all sprouts or stems from an infected mother tuber will become infected, giving rise to healthy daughter tubers, or because not all daughter tubers on the same infected stem will become infected.
Here, we report on an experimental test of the hypothesis that environment-driven factors may limit autoinfection with four important potato viruses: PVX, APMoV, PVY, and PLRV and we confront the results of these experiments with most recent findings related with virus propagation. These experiments were done in 1987 and 1988 at different altitudes in Peru and were designed to quantify the percentage of daughter tubers becoming infected from mother tubers that were infected by each of the four mentioned viruses. The results clearly indicated that the tuber progeny from infected mother tubers is infected at an incidence much below 100%. The data were initially used to validate a model for temperature-modulated seed degeneration by potato viruses (Bertschinger et al., 1995a,b), which provided implicit evidence for the existence of such phenomenon. However, the findings were not published on their own, primarily because they appeared incompatible with the biological theory at that time. The authors believe that these results should now be made available to the scientific community for two important reasons. First, our knowledge of host-pathogen interactions has greatly advanced indicating how plant defense systems may limit the accumulation and spread of viruses within infected plants (Burgyán, 2006). The above-mentioned results must be re-discussed in view of the nowadays deepened knowledge of host-pathogen interactions with the opportunity to possibly come up with further research questions and sharpen our knowledge in potato virus epidemiology. The second reason is more related to the current on-farm situation. Potato yields are relatively poor in low and middle-low income countries (FAO, 2013) and a major cause of this low productivity is seed degeneration (Fuglie, 2007; Gildemacher et al., 2009; Cromme et al., 2010; Thomas-Sharma et al., 2016). The re-valued results of the above-mentioned studies could contribute to creating an integrated seed health strategy for potato making seed systems more sustainable. To address the constraint of seed degeneration, Thomas-Sharma et al. (2016) recently outlined such a strategy, within which host plant resistance and on-farm disease management play integral roles. They showed that such an approach, including positive selection and the regeneration of degenerated seed, can be used for designing adequate and sustainable seed systems to improve potato productivity in parts of the world that lack access to a continuous supply of healthy seed (Gildemacher et al., 2012; Schulte-Geldermann et al., 2012; Thomas-Sharma et al., 2016).
Thus, there is a need to recheck and confirm the Peruvian data on incomplete autoinfection; a greater understanding of the phenomenon promises to open up new perspectives that could benefit the locally adapted seed systems and eventually increase food security. We therefore first describe, how the Peruvian field data on autoinfection with potato viruses were obtained, document the evidence for the observed, environment-dependent phenomenon of incomplete autoinfection, and subsequently challenge these results with current knowledge in plant virology. Finally, we evaluate our findings and discuss their relevance. We conclude by hypothesizing that tuber infection with potato viruses is limited or even avoided by a mechanism preventing daughter tubers of an infected mother tuber to become infected and that this mechanism is temperature driven.
The experimental work was carried out with potato seed tubers infected with PVX, APMoV, PVY, and PLRV. All PVY infected seed tubers were co-infected with PVX. Damage from PVY is especially high if the plant is co-infected with PVX (Gibson, 1991). The probability for co-infections is high in Peru in view of the extremely high PVX incidence (Monasterios dela Torre, 1966; Bertschinger et al., 1990a,b; Martin-Hernandez and Baulcombe, 2008). For PVY and PVX, virus strain prevalence in the seed tubers utilized for this research was determined at the International Potato Center in Peru (International Potato Center (CIP), 1986, 1988, 1989) in view of the fact that contrasting strains had been identified and for therefore better characterizing the experimental situation. Approximately 90% of the PVY infected tubers were positive for a PVYN-like strain, while the remaining 10% were infected by a PVYO-like strain. PVX infections belonged to the common O serotype. These strains were the predominant types in the Andean Highlands at the time of the study (Fernandez-Northcote, 1990; Fernandez-Northcote and Lizárraga, 1991).
Virus-infected tubers were planted in 1987 in three locations representing different agroecological potato growing zones in Peru: Imperial (department: Lima; province: Cañete; district; Nuevo Imperial; latitude: 13°0′ S; longitude: 76°2′ W; elevation: 112 m a.s.l.), Santa Ana (Junin; Huancayo; El Tambo; 12°1′ S; 75°1′ W; 3280 m) and Chicche (Junin; Jauja; Apata; 11°5′ S; 75°2′ W; 4000 m) (Table 1). One plot per treatment was planted at each location in 1987, and the experiment was repeated in 1988 with a minor modification in the treatments described below.
The cultivar Yungay (Solanum tuberosum ssp. tuberosum ×S. tuberosum ssp. andigena) was chosen because it was widely grown in all zones and susceptible to each of the four viruses used in our study (International Potato Center (CIP), 1986). Infected seed tubers came from on-going experiments in the Mantaro Valley or were multiplied there for the sake of this experiment. Before planting, seed tubers were tested by the enzyme linked immunosorbent assay (ELISA) and selected for single infections with PVX, APMoV and PLRV, and for co-infections of PVX with PVY.
Seed tubers (mother tubers), confirmed to be infected according to the testing described below and consequently later giving rise to secondarily infected plants, were planted jointly with healthy seed tubers at the above mentioned research sites in plots with 10 rows, each of 10 m length with 1 m between-row spacing and 0.33 m between-plant spacing, giving a total of 300 plants per plot. Separate plots were planted for each virus species. The infected and the healthy seed tubers were uniformly distributed in each plot of 300 plants (Campbell and Madden, 1990), i.e., healthy and infected seed tubers were evenly distributed in the experimental field plots, while the position of each secondarily infected plant was marked and registered to be able to harvest their tubers for the purpose of this study. The plots were enlarged by extending each row by 2 m at both ends and by adding two border rows adjacent to rows 1 and 10, where on-farm sanitation (weeding and insecticide spraying if needed) was applied. This was to reduce co-infection of daughter tubers with other viruses due to primary infection of the mother plant that could possibly interfere with systemic tuber infection with the viruses under study. Plants received 90-180-180 kg ha-1 of N-P2O5-K2O at planting and a further 90 kg N ha-1 at hilling. Symptoms of secondarily infected plants were evaluated (presence or absence of symptoms) 6–10 weeks after planting, by checking whether secondarily infected plants were visible or not and whether the within field position of such plants was confirmed. Daily minimum and maximum temperatures were measured 1–2 m above ground and missing data were treated as described by Coakley (1989). The experiments were performed over two growing seasons (1987, 1988), by planting plots with the same experimental design but in a different field at the same site.
At harvest of the experimental plots, four randomly selected daughter tubers from each secondarily infected plant were collected. These tubers were stored before testing for virus infection (procedure see below) in paper bags (four tubers per bag, i.e., per plant) placed in wooden trays for 1–2 months after harvest at ambient temperature. Bags were sheltered from wind and rainfall, and protected from tuber moth (Phthorimaea operculella) and other storage insects with Sevin®(carbaryl dust) as appropriate. From the four tubers harvested per plant and stored as mentioned above, three tubers were tested with ELISA to determine virus presence as described below.
Infection of secondarily infected mother tubers was determined using ELISA on sprout sap after having been stored at 20°C in the dark with more than 60% relative humidity for a minimum of 5 weeks. This insured high virus concentration for subsequent ELISA tests. Sap from tuber sprouts was extracted by grinding jointly two sprouts per tuber in extraction buffer (phosphate buffered saline, 1%; PVP, 0.2%; egg albumen, 0.1%; Tween-20, 0.05%) for ELISA (1:5 w/v) (Gugerli, 1979, 1986). ELISA was performed as described below. Only tubers with proven infection as identified by this procedure were planted to give rise to secondarily infected plants for later measurement of virus infection of daughter tubers.
In preparing daughter tubers for ELISA-testing, tuber dormancy was broken with Rindite (250 ml/m3) or Bromoethane (200 ml/m3) treatment (Bryan, 1989) for 48 h at 20–25°C in a black box having an internal ventilation system and a capacity of eight trays holding approximately 50 kg of tubers. Trays were maintained afterward for 5 weeks in darkness at 20–25°C and 60–70% relative humidity. Daughter tubers were tested by analyzing tuber sap extracted by using a plant sap extractor (Tecan AG, Hombrechtikon, Switzerland) (Gugerli, 1979, 1986) (ELISA specifications see below). The accuracy of the ELISA assays was ensured by the following procedure, in order to prevent “false negatives” (i.e., tubers with an ELISA result indicating no infection while, in fact, the infection was not detected by ELISA due to limited sensitivity): 100 daughter tubers per Rindite or Bromoethane treatment lot were selected composed by tubers with potentially high likelihood of false negatives. Such a high likelihood was attributed to daughter tubers from secondarily infected plants that were classified by the daughter tuber test as ELISA positive on only one or two daughter tubers per plant but not on all three tested tubers. Confirmation tests were performed on tuber sprout sap or leaf extract from 4 to 5 weeks old plants grown from the selected 100 tubers in an aphid-proof screen house (grow-out tests). If more than 5% of tubers that did not seem to be infected based on the ELISA-test were in fact infected according to the results of the grow-out test, the entire lot was discarded and the data were not used in the subsequent data analyses (occurred in <5% of the tuber batches tested).
The ELISA test (Clark and Adams, 1977) was performed with polyclonal immunoglobulin (IgG) antisera by the International Potato Center (CIP) for PVX, PVY, and APMoV and with monoclonal antibodies for PLRV (Bioreba AG, Reinach, Switzerland). Polyclonal alkaline phosphatase (AP) conjugates were produced using a standard protocol giving a final concentration of 2580 U AP/0.646 mg IgG/ml. Optimal concentrations for plate coating with IgG and conjugate solution were determined for each antibody by ‘checkerboard’ testing (Converse and Martin, 1990). For coating, polyclonal IgG concentration was applied at 0.66–1.33 μg/ml, depending on the antibody lot. Enzyme conjugates were diluted 750- to 1500-fold. Monoclonal antisera were diluted 1000-fold. Coating IgG, plant extracts and enzyme conjugates were applied at 230, 180, and 200 μl/well, respectively, and incubated at 30°C for 5 h, at 4°C overnight, and at 30°C for 4 h, respectively. Nitrophenyl-phosphate was applied at 1 mg/ml substrate solution, 160 μl/well. Special additives included egg albumin (2% w/v) for extraction and conjugate buffer, and 1 mmol MgCl of conjugate buffer (Gugerli, 1986). Plates were evaluated visually on a backlit screen comparing yellowing intensities of particular wells to the reference wells with buffer, healthy and positive standard extracts (three wells per plate). Absorption values (405 nm) of some plates were measured (Titertek Uniskan II, Flow Laboratories, McLean, VA, USA) to ensure that the tuber treatment and incubation procedures were effective in giving high virus concentrations for easy visual identification of positive wells, and that antisera reactivity remained constant.
The number of secondarily infected plants considered per season and site varied according to the availability of secondarily infected plants grown from uncut seed tubers. Three sources of variance are to be distinguished for this study: virus, season (year) and site. The percentage of infected daughter tubers was calculated by summing up for each of the four virus classes studied the frequencies of tubers that were determined to be virus infected or uninfected in one particular season and site. Frequencies were then compared using Fisher’s exact test for 2×2 tables. This test enables comparison of low and high frequencies (Fisher, 1990). For each virus, all possible pair-wise comparisons between seasons and sites were made. Based on this analysis, the significance of differences between each frequency was determined and represented with equal or unequal letters in the respective figures and tables. The higher frequencies were compared using χ2- tests.
Incomplete autoinfection was found in all cases, as the incidence of virus infected tubers harvested from secondarily infected plants was invariably less than 100%. The phenomenon was highly variable, depending on virus and location, but with a tendency to have more autoinfection at the lowest altitude. The highest value of autoinfection was obtained with PLRV at 112 m (88%) in 1987 although this was not significantly different from the PLRV percentage observed in 1988 (83%) (Figure 1). Also PVX, APMoV, and PVY (together with PVX) were detected in over 80% of tuber infections at 112 m. The lowest percentage of infection (30%) was observed for APMoV at 4000 m in 1988 and 1989 (Figure 1).
FIGURE 1. Tuber infection (% infected tubers) of plants secondarily infected with PVX, APMoV, PVY and PLRV in three agro-ecological zones of Peru. The cultivar Yungay (Solanum tuberosum ssp. tuberosum x S. tuberosum ssp. andigena) was used. Numbers in columns represent the number of analyzed plants. Values within columns having the same letter and corresponding to the same virus are not significantly different in a comparison with Fisher’s exact test (P ≤ 0.05). Seed tubers infected with PVY were co-infected with PVX. No data are available for PVY for the seasons 1987 and 1987/88, respectively (N/A). Adapted from Bertschinger (1995).
The effect of the changing environment at the experimental sites was evident for all viruses. The extent of decreasing tuber infection while increasing elevation varied across sites and seasons depending on the virus. For PVX, there were significant differences between all growing sites, while the tuber infection level was in all seasons the same at one particular site. For APMoV, however, there were also significant differences in tuber infection between seasons at one particular site (at 112 and 3280 m) in addition to the differences across sites. Sharp decreases occurred for PVY and PLRV at elevations between 112 and 3280 m, with 27 and 50% average reduction in tuber infections, respectively. Infection did not decrease any further at higher elevations (4000 m). The pattern of symptom expression was correlated to the percentage of tuber infection for PVY, PLRV, and APMoV and was inversely related to the elevation of the potato production plots (Table 2). In contrast, PVX symptom expression increased at higher elevation. The highest percentage of secondarily infected plants with symptoms was observed for PLRV at 112 m (99%), while the lowest was observed for PVX also at 112 m (0%). This seems surprising at first instance, since a high systemic infection of tubers suggests high virus titres which – even if only assumingly – might relate to high symptom expression. One explanation for this unexpected result could be that genetic processes governing altered virus replication and translocation as components of ‘resistance’ sensu Cooper and Jones (1983) may not be the same as those governing symptom expression, being a component of plant ‘tolerance/sensitivity.’ These genetic processes may respond differently to contrasting environmental conditions in case of PVX. It can be concluded that symptom expression is in general not governed by the same mechanisms as a limitation of autoinfection of daughter tubers of secondarily infected mother plants with potato viruses. Furthermore, symptom evaluation is generally not an appropriate method for detecting virus-infected plants under Peruvian conditions in the case of PVX.
TABLE 2. Symptom expression of plants that were secondarily infected with PVX, APMoV, PVY, and PLRV in three agro-ecological zones of Peru (percent of plants with symptoms, cultivar Yungay a,b).
Grow-out tests (see ‘virus testing’ under materials and methods) did not provide evidence for infected tubers that were not detected by the ELISA-testing. There was 99% compliance between the proportion of daughter tubers that were infected with PLRV at 112 m in 1987 (88%) with the proportion of plants with PLRV symptoms in the respective grow-out test. The results of the grow-out tests and the other precautions taken to ensure accurate results (care in tuber storage and confirmation testing and grow-out plots; see ‘reference field data; virus testing’) assured reliability of ELISA-results, avoiding false negatives and assuring that daughter tuber considered to be healthy were indeed healthy and that the calculated autoinfection rates are trustworthy.
Several aspects of current knowledge were reviewed to assess their relevance to reduced autoinfection of daughter tubers from secondarily infected mother plants. These are:
– Host plant genetics;
– Mature plant resistance;
– Environmental factors;
– Anti-viral gene silencing and epigenetic effects.
Genetic factors of the host plant were shown to be involved in autoinfection with plant viruses for other pathosystems with autoinfection being an important component of host plant resistance (Fauquet et al., 1988; Fargette et al., 1996). Potato germplasm has not been characterized so far with regard to its genetic variability for autoinfection of secondarily infected plants, neither have the underlying genomics and their interaction with the environment been studied. However, genetic variability for systemic tuber infection in primarily infected plants has been reported, as shown for PLRV (Syller, 1991, 1994, 2003; Difonzo et al., 1994) or PVY (Gibson, 1991). Also, the results of various virus incidence surveys reported for a wide variety of potato genotypes in the Andes (Monasterios dela Torre, 1966; Fankhauser, 2000) point at genetic variability, even if survey data are not a compelling proof for genetic variability with regard to systemic tuber infection factors. Nonetheless, the surveys strongly support the hypothesis that reduced autoinfection may occur in many Andean potato genotypes and that autoinfection may constitute a general mechanism of virus-host pathosystems under Andean conditions. In view of the results of the studies cited, it can be concluded that host plant genetics are a major driver of a limited autoinfection in potato virus pathosystems.
This kind of resistance explains partial tuber infection in primarily infected plants. Beemster (1972) demonstrated for PVY that the older the plant when it becomes (primarily) infected, the more resistant it is in terms of the percentage of tubers that become infected (‘age’ or ‘mature plant’ resistance). Other studies present similar results for PLRV (Barker, 1987; Difonzo et al., 1994; Whitworth et al., 2000) and PVY (Gibson, 1991). This was explained by the fact that the later the plant becomes infected, the less time there is available and the less conducive the conditions are for virus replication, accumulation and systemic translocation inside the plant. Daughter tubers of primarily infected plants might consequently escape infection, be it because of less physiological time for virus multiplication and translocation for reaching tuber tissues or be it because of meristem exclusion mechanisms for virus invasion yet to be proven.
However, both mechanisms of limiting tuber infection can be excluded for secondarily infected plants: First, the period during which viruses can multiply, translocate and invade daughter tubers is not limited in secondarily infected plants, but rather lasts the entire seasons from planting to harvest. Thus, time is not a limiting factor for virus particles to invade tuber tissue. Second, if there were a meristem exclusion mechanism against virus invasion, more developmental time should result in less exclusion while the contrary was observed: at high altitudes, daughter tuber infection was lower than at low altitudes, while there was in fact 3–7 weeks more growth time available at high altitudes for the virus to multiply and translocate systemically (Table 1; Figure 1).
The diurnal temperature variations between the different experimental sites of this study were extremely different (Figure 2), providing a range of contrasting environments for studying plant–virus interactions. Temperature has a major and direct impact on virus behavior and the interactions of potato viruses with their host plant or insect vector. Primary infection with PVX or with PVYN or PVYO is altered by temperature (De Bokx and Piron, 1977; Tamada and Harrison, 1981). The same is true for systemic translocation of PVX, PVYN and PVYO (Wislocka, 1982; Adams et al., 1986), replication of PVX, PVY and PLRV (Adams et al., 1986; Gase et al., 1990), resistance of potato genotypes to potato virus M (Carlaviridae), PVX, PVY and PLRV infection (Gase et al., 1990), and replication of different strains of PVY (De Bokx and Piron, 1977). Additionally, temperature has been shown to alter cell-to-cell movement of viruses, i.e., the expression of viral encoded transport functions (e.g., for Potyviridae) (Zimmern and Hunter, 1983; Dougherty and Carrington, 1988). All these studies provide evidence for the fact that temperature affects plant–virus interaction for primary infections. The effect of temperature on secondary infections is less well-documented. PLRV accumulation in leaves of secondarily infected plants has been shown not to vary with temperature (Tamada and Harrison, 1981; Syller, 1994). However, even if experimental evidence is not yet available in all aspects mentioned above, many of the mechanisms documented by studies addressing primary infections are likely to impact also on systemic virus infection in secondarily infected plants. Interestingly, a temperature-driven model for autoinfection with potato viruses, relating accumulated developmental heat for virus proliferation of daughter tubers of secondarily infected potato plants (Bertschinger et al., 1995a) has been able to reproduce accurately the autoinfection documented in this paper, calibrated by the particular diurnal temperature variations observed in this study (Figure 2). Consequently, it can be hypothesized that temperature is a major factor in affecting viral replication and translocation and this may explain why temperature also affected autoinfection in our study.
FIGURE 2. Minimum and maximum temperatures at experimental sites representing three potato growing zones in Peru. Only monthly data were available for the Imperial site in 1987. Republished from Bertschinger (1995).
Low light intensity (irradiance) was shown to significantly increase the severity of mosaic disease caused by potato virus X and potato virus Y (Draper et al., 2002). This was explained by a tentative impact of irradiance on plant–virus interaction in epidermal cells by altering “local acquired resistance” (but not “systemic acquired resistance”) as evidenced with tobacco mosaic tobamovirus (TMV) (Chessin, 1982). However, if applying this knowledge to the symptom expression or autoinfection data from Peru at locations with different levels of irradiance (see Table 2), no conclusive pattern arises. Virus-induced leaf lesions, such as a necrosis, are associated with a high respiratory rate of plant cells. Substances involved in respiratory processes accumulate particularly in cells around the necrotic lesions (Yamaguchi, 1960; Parish et al., 1965). Only 60% of dioxygen available at 0 m a.s.l. is available at 4000 m a.s.l., but in our study symptom expression of PVX was observed to be higher at higher altitudes (Table 2). Therefore, the scientific data, available so far, on effects of irradiance or dioxygen concentration, are not consistent with the relationship found in this study between the experimental site (i.e., its altitude related with a specific irradiance and dioxygen level) and virus symptom expression or autoinfection.
At the time, when the materials presented in this paper were produced, biological theory excluded an active reaction of the plant against the invasion of a virus. However, nowadays, there is scientific evidence for RNA-silencing, a plant mechanism that limits the accumulation and spread of viruses after an infection, and for RNA silencing suppression by plant pathogens: defense, counter-defense, and counter-counter-defense (Pumplin and Voinnet, 2013; Sansregret et al., 2013). It has also been reported, that potato viruses are no exception to this (Voinnet et al., 1999). It is now known whether epigenetics may play a role in potato virus gene silencing (Dalmay et al., 2000). It has been found that temperature affects the regulation of RNA gene silencing and its pathogen regulated suppression. An increased temperature may, e.g., reduce suppression of antiviral gene silencing. But the effect of high temperature on antiviral RNA-silencing and pathogen-mediated suppressor of silencing pathways is not yet fully understood (Qu and Morris, 2005). Epigenetic effects are increasingly understood and potato virus X is one of the model organisms being studied. But many aspects require further elucidation before full comprehension is achieved (Rajeevkumar et al., 2015).
Experimental proof is still lacking that the incomplete autoinfection with potato viruses found in this study is due to temperature-driven epigenetic effects. However, the above-mentioned recent findings in understanding gene-silencing phenomena encourage studying whether these may contribute to observed partial autoinfection with potato viruses reported in our study. In this respect, we consider it promising that a model for temperature-modulated autoinfection (Bertschinger et al., 1995a) was accurately calibrated by the experimental autoinfection data obtained and the prevalent temperature fluctuations presented in this study (see Figures 1 and 2).
In traditional seed systems in the Andes, there is no steady supply of healthy seed delivered by a formal seed production system, as there is in industrialized countries. However, partly because of incomplete autoinfection, as shown below, there is in epidemiological terms under certain growth conditions no ultimate necessity of such a supply for preventing low yields because of potato virus epidemics. Assuming random selection of seed tubers from a harvested tuber lot, as well as ambient circumstances where autoinfection is lower than 100% and a low within-season infection rate occurs (i.e., few or no primary infections), e.g., due to low density or minimal prevalence of vectors, the incidence of virus-infected plants will approach zero in consecutive planting generations. This phenomenon may be offset by non-random selection of seed (particularly by a tendency to pick smaller seed which may disproportionately represent virus-infected plants) and/or by high primary infection rates. The interplay of degenerative and regenerative factors could explain why virus incidence in farmers’ seed used in the Andes is often found to be quite low despite the lack of a seed system providing virus-free seed (Bertschinger et al., 1990b; Haan, 2009; Pérez Barrera et al., 2015).
The effect of the three growing sites with contrasting altitudes on autoinfection efficiency in our study also implicitly explains the benefits gained by moving seed from sites at lower to higher elevations, a practice commonly applied by farmers in traditional Andean potato systems (Thiele, 1999). It appears that farmers cleverly made use of the reduced efficiency of autoinfection at higher altitudes to maintain crop productivity and prevent degeneration.
Crop management practices in farmers’ fields differ across agro-ecological zones of Peru represented in this study. For instance, farmers in these different zones may use different planting densities, plant nutrition and other parameters that influence crop performance, affecting tuber size, tuber numbers per plant and other variables. These management differences may also alter plant metabolism, which in turn may impact virus-plant interactions. To control for these potential crop management effects on autoinfection rates, crop management practices were standardized (e.g., uniform mineral fertilization) in all experimental fields of this study.
As noted above, farmers in the Andes have traditionally used altitude to manage and even reverse seed degeneration, but there are additional management options. For example, seed tuber management and crop management (mainly related to managing the maturity resistance), and early harvesting can reduce the level of autoinfection (Struik and Wiersema, 1999). Additionally, a technique to maintain or even improve seed quality has been widely advocated and disseminated by the International Potato Center for application in regions where farmer-saved seed is still dominant, such as Eastern Africa. This technique, referred to as positive selection, involves identifying plants that look healthy at an early stage of the season (e.g., at flowering), which will eventually be harvested for seed at the end of the growing season (Gildemacher et al., 2011, 2012; Schulte-Geldermann et al., 2012; Thomas-Sharma et al., 2016). The positive selection method is simple, cheap and robust, and can easily be adopted by small-holder seed growers or ware growers who plant their own seed. However, it may only work when high plant vigor correlates with low virus infection (i.e., low systemic virus-proliferation of the plant tissue), which might not always be the case, as shown in the study presented in this paper. Schulte-Geldermann et al. (2012) found that a very significant yield increase across agro-ecologies and varieties tested was associated with a reduction in virus incidence for PLPV, PVY, and PVX. However, the reduction in virus incidence could not completely account for the yield increase, suggesting that other factors were also important, such as the interactions among viruses in seed with multiple infections. Gildemacher et al. (2011) also found that yield increase due to positive selection was not always traceable to reduction in virus incidence or other factors.
Applying positive selection might regenerate a degenerated seed stock (i.e., it might reverse degeneration) if consistently and effectively applied across several generations. This is possible if clean seed or almost clean seed is still present in the seed lot that is planted in reasonably high proportions and if plants from those healthy seeds are effectively selected by the positive selection method. However, if this practice is combined with an environment-induced reduced efficiency of autoinfection as described in this paper, the sanitation effect of such practices should be significant and most of all agronomically most relevant. Although little literature data is available on long-term effects of positive selection, some research projects are currently ongoing in Uganda and elsewhere in Africa to assess to what extent regeneration is feasible and to what extent techniques such as positive selection can stop or delay autoinfection under severe or mild aphid pressure, starting with high-quality or low-quality seed lots (e.g., Priegnitz et al., 2014). In view of the data presented in this paper, the environment dependent contribution of incomplete autoinfection to seed regeneration must be taken into account for improving seed and seed systems. In light of the presented results, costly seed certification systems as found in the industrialized countries, which are based on epidemics with a rapidly increasing share of infected tubers due to the assumption of 100% autoinfection of secondarily infected plants, may be replaced by locally adapted seed multiplication systems for controlling virus incidence in seed.
The commonly held theory in potato virus epidemiology that all daughter tubers of a seed-tuber infected mother plant (secondary infection) are systemically infected, is rejected by this study. The hypothesis that potato tuber infection by viruses (in the present study PVX, APMoV, PVY, and PLRV) may be limited or even avoided by a mechanism preventing daughter tubers of an infected mother tuber to become infected, is clearly supported by the presented field data.
This seemingly simple theory has great practical consequences, since costly, formal seed potato systems in industrialized countries in temperate climates are based on this assumption, supported by evidence under these climates. However, these formal systems have also been applied (albeit unsuccessfully) in low-income countries with different climatic conditions and traditional, informal seed systems.
Based on an extensive literature review, it can be concluded that reduced autoinfection is seemingly environment-dependent and most likely temperature-driven. Recent findings regarding the epigenetic regulation of plant virus – host interactions suggest that temperature-driven anti-viral gene silencing may explain the described phenomenon observed in Peruvian potato fields. However, this remains yet to be experimentally proven for the specific potato virus pathosystems addressed in this paper.
While the traditional epidemiological theory of potato viruses must be reconsidered in the light of these findings, the latter open up new perspectives for improving food security by contributing to environmentally adapted effective and sustainable seed potato systems and also for breeding purposes. These results should be of particular benefit in potato growing zones where a continuous supply of healthy seed produced by a formal certification has, to date, been unsuccessful. Also, the combination of the findings with those developed in other studies for improving seed systems, e.g., implementing on-farm practices such as positive selection, will contribute to the design of a farmer-friendly package of management practices that further improve the productivity of the crop.
The discovered environmental dependency of incomplete autoinfection is also of great importance in view of the challenges experienced by climate change. A change in plant virus epidemics may be forecasted for global potato growing zones, which could inform policy designed to mitigate potentially negative effects of climate change.
Explicit experimental proof of the hypothesized temperature-driven RNA-silencing in the addressed potato virus pathosystems would facilitate exploitation of the principle of incomplete autoinfection. The genetic base and inheritance of incomplete autoinfection with potato viruses should be characterized, as well as its epigenetic dimension. Quantifying temperature-driven regulation of the observed phenomenon would facilitate efforts to improve seed quality and food security under varying environmental conditions.
Conceived and designed the experiments: LBe, CG, EK, and US. Performed the experiments and analyzed data: LBe. Discussed and validated the results in view of new research findings and scientific evidence and wrote the paper: LBe, LBü, BDuf, BDup, GF, US, and PS.
This work was supported by the Swiss Agency for Development and Cooperation (www.sdc.admin.ch). The funding agency had no role in study design, data collection and analysis, decision to publish, or preparation of the manuscript.
The authors declare that the research was conducted in the absence of any commercial or financial relationships that could be construed as a potential conflict of interest.
The authors are grateful to Victor Laura for allowing us to conduct the field experiments on his land in Chicche, Peru. We thank Cesar Cerrón, Thomás García Salazar, and Genaro Sanabria for collection of weather data, Ofelia Pinillos and Rosario Gonzales (INIAA) for performing ELISA tests. We are very grateful to Fernando Ezeta, regional director at CIP, Antenor Hidalgo, Peruvian potato research program director (PIPA/INIAA), and Cesar Vitorelli and Efrain Franco, leader of the Peruvian seed production project (INIAA/CIP/SDC), for providing the institutional backbone of the studies made. Thank you to Carole Balmelli (Agroscope) and John A. Pickett (Rothamsted Research) for offering review and helpful comments to the manuscript. We thank the Swiss Development Cooperation (SDC) for providing funding support for the field studies and analytical work.
Adams, S. E., Jones, R. A. C., and Coutts, R. H. A. (1986). Effect of temperature on potato virus X infection in potato cultivars carrying different combinations of hypersensitivity genes. Plant Pathol. 35, 517–526. doi: 10.1111/j.1365-3059.1986.tb02050.x
Baldauf, P. M. (2008). Studies on the Epidemiology of Potato Viruses in the Northeastern USA and the Biology of Potato Virus Y. Ph.D. thesis, Cornell University, New York, NY, 172.
Barker, H. (1987). Multiple components of the resistance of potatoes to potato leafroll virus. Ann. Appl. Biol. 111, 641–648. doi: 10.1111/j.1744-7348.1987.tb02021.x
Beemster, A. B. R. (1967). Partial infection with potato virus YN of tubers from primarily infected potato plants. Neth. J. Plant Pathol. 73, 161–164. doi: 10.1007/BF02000456
Beemster, A. B. R. (1972). “Virus translocation in potato plants and mature-plant resistance,” in Viruses of Potatoes and Seed-Potato Production, ed. J. A. de Bokx (Wageningen: Centre for Agricultural Publishing and Documentation), 144–151.
Bertschinger, L. (1995). Modelling of Potato Virus Pathosystems by Means of Quantitative Epidemiology: An Exemplary Case Based on Virus Degeneration Studies in Peru. Ph.D. thesis, Nr. 9756, Swiss Federal Institute of Technology in Zürich, Zürich, 111.
Bertschinger, L., Keller, E. R., and Gessler, C. (1995a). Characterization of the virus x temperature interaction in secondarily infected potato plants using EPIVIT. Phytopathology 85, 815–819. doi: 10.1094/Phyto-85-815
Bertschinger, L., Keller, E. R., and Gessler, C. (1995b). Development of EPIVIT, a simulation model for contact- and aphid-transmitted potato viruses. Phytopathology 85, 801–814. doi: 10.1094/Phyto-85-801
Bertschinger, L., Keller, E. R., Gessler, C., Ezeta, F., and Hidalgo, A. (1990a). “Epidemiology of potato viruses in Peru,” in Proceedings of the 11the Triennial Conference of the European Association of Potato Research, (Edinburgh: European Association of Potato Research), 263–264.
Bertschinger, L., Luther, L., Pinillos, O., and Hidalgo, A. (1990b). La incidencia de virus en cultivares nativos y mejorados en la sierra peruana. Rev. Latinoam. Papa 3, 62–79.
Bertschinger, L., Scheidegger, U. C., Muñoz, J., and Hidalgo, A. (1996). Efecto de diferentes virus sobre el rendimiento potencial de la papa y su interacción con el estado de brotamiento de tubérculos-semilla en la costa del Perú. Rev. Latinoam. Papa 7, 36–54.
Burgyán, J. (2006). Virus induced RNA silencing and suppression: defense and counter defense. J. Plant Pathol. 88, 233–244.
Campbell, C., and Madden, L. (1990). “Spatial aspects of plant disease epidemics. ii: analysis of spatial pattern,” in Introduction to Plant Disease Epidemiology, eds C. M. Campbell and L. V. Madden (New York, NY: John Wiley), 289–328.
Carnegie, S. F., Davey, T., and Saddler, G. S. (2010). Effect of temperature on the transmission of Potato mop-top virus from seed tuber and by its vector, Spongospora subterranea. Plant Pathol. 59, 22–30. doi: 10.1111/j.1365-3059.2009.02187.x
Chessin, M. (1982). Interference in plant virus infection: ultraviolet light and systemic acquired resistance. J. Phytopathol. 104, 279–283. doi: 10.1111/j.1439-0434.1982.tb00535.x
Clark, M. F., and Adams, A. N. (1977). Characteristics of the microplate method of enzyme-linked immunosorbent assay for the detection of plant viruses. J. Gen. Virol. 34, 475–483. doi: 10.1099/0022-1317-34-3-475
Coakley, S. M. (1989). “Historical weather data: its use in epidemiology,” in Plant Disease Epidemiology, Vol. II, eds K. J. Leonard and W. E. Fry (New York: Mc Graw-Hill Publishing Co.), 54–83.
Converse, R. H., and Martin, R. R. (1990). “Elisa methods for plant viruses,” in Serological Methods for Detection and Identification of Viral and Bacterial Plant Pathogens, eds R. Hampton, E. Ball, and S. De Boer (Saint Paul, MN: The American Phytopathological Society, APS Press), 179–196.
Cooper, J. I., and Jones, A. T. (1983). Responses of plants to viruses: proposals for the use of terms. Phytopathology 73, 127–128. doi: 10.1094/Phyto-73-127
Cooper, J. I., Jones, R. A. C., and Harrison, B. D. (1976). Field and glasshouse experiments on control of potato mop-top virus. Ann. Appl. Biol. 83, 215–230. doi: 10.1111/j.1744-7348.1976.tb00600.x
Cromme, N., Prakash, A. B., Lutaladio, N., and Ezeta, F. (2010). Strengthening Potato Value Chains. Technical and Policy Options for Developing Countries. Rome: Food and Agriculture Organization of the United Nations and the Common Fund for Commodities.
Dalmay, T., Hamilton, A., Mueller, E., and Baulcombe, D. C. (2000). Potato Virus X amplicons in Arabidopsis mediate genetic and epigenetic gene silencing. Plant Cell 12, 369–379. doi: 10.2307/3870942
Davey, T., Carnegie, S. F., Saddler, G. S., and Mitchell, W. J. (2014). The importance of the infected seed tuber and soil inoculum in transmitting Potato mop-top virus to potato plants. Plant Pathol. 63, 88–97. doi: 10.1111/ppa.12074
De Haan, S., and Thiele, G. (2003). “In situ conservation and potato seed systems in the Andes,” in Seed Systems and Crop Genetic Diversity on-Farm, eds I. Devra, J. R. Sevilla-Panizo, J. L. Chávez-Servia, and T. Hodgkin (Pucallpa: The International Plant Genetic Resources Institute (IPGRI)), 126.
De Bokx, J. A., and Piron, P. G. M. (1977). Effect of temperature on symptom expression and relative virus concentration in potato plants infected with potato virus YN and YO. Potato Res. 20, 207–213. doi: 10.1007/BF02418680
Department of Agriculture and Food (2016). Potato Leafroll Virus in Potato Crops. Government of Western Australia. Available at: https://www.agric.wa.gov.au/potato-leafroll-virus-potato-crops
Difonzo, C. D., Ragsdale, D. W., Radcliffe, E. B., and Banttari, E. E. (1994). Susceptibility to potato leafroll virus in potato: effects of cultivar, plant age at inoculation, and inoculation pressure on tuber infection. Plant Dis. 78, 1173–1177. doi: 10.1094/PD-78-1173
Dougherty, W. G., and Carrington, J. C. (1988). Expression and function of potyviral gene products. Annu. Rev. Phytopathol. 26, 123–143. doi: 10.1146/annurev.py.26.090188.001011
Draper, M. D., Pasche, J. S., and Gudmestad, N. C. (2002). Factors influencing PVY development and disease expression in three potato cultivars. Am. J. Pot. Res. 79, 155–165. doi: 10.1007/BF02871931
Engsbro, B. (1976). Investigations and experiments concerning soil-borne viruses. I. Rattle virus, continued investigations on potato 2. Tidsskr. Panteavl 80, 405–410.
Fankhauser, F. (2000). Seed-Transmitted Diseases as Constraints for Potato Production in the Tropical Highlands of Ecuador. Ph.D. thesis, Nr. 13770. Swiss Federal Institute of Technology Zurich, 141.
FAO (2013). Food and Agriculture Organization of the United Nations, FAOSTAT Database. Available at: http://faostat.fao.org/site/567/default.aspx#ancor
Fargette, D., Colon, L. T., Bouveau, R., and Fauquet, C. (1996). Components of resistance of cassava to African cassava mosaic virus. Eur. J. Plant Pathol. 102, 645–654. doi: 10.1007/BF01877245
Fargette, D., Thresh, J. M., and Otim-Nape, G. W. (1994). The epidemiology of African cassava mosaic geminivirus: reversion and the concept of equilibrium. Trop Sci. 34, 123–133.
Fauquet, C., Fargette, D., and Thouvenel, J. C. (1988). “Selection de maniocs sain obtenus par reversion dans les champs de manioc,” in La Mosaique Africaine du Manioc et Son Control, eds C. Fauquet and D. Fargette (Yamoussoukro: Editions de l’ORSTOM), 135–137.
Fernandez-Northcote, E. N. (1990). “Variability of PVX and PVY and its relationship to genetic resistance,” in Control of Virus and Virus-like Disease of Potato and Sweet Potato, ed. E. N. Fernandez-Northcote (Lima: International Potato Center), 131–139.
Fernandez-Northcote, E. N., and Lizárraga, C. H. (1991). Distribución geográfica de serotipos del virus x de la papa. Fitopatología 26, 13–18.
Fisher, R. A. (1990). “Statistical methods, experimental design, and scientific inference,” in A Re-issue of Statistical Methods for Research Workers: The Design of Experiments, and Statistical Methods and Scientific Inference, ed. J. H. Bennett (Oxford: Oxford University Press), 78–111.
Frost, K. E., Groves, R. L., and Charkowski, A. O. (2013). Integrated control of potato pathogens through seed potato certification and provision of clean seed potatoes. Plant Dis. 97, 1268–1280. doi: 10.1094/PDIS-05-13-0477-FE
Fuglie, K. O. (2007). Priorities for potato research in developing countries: results of a survey. Am. J. Pot. Res. 84, 353–365. doi: 10.1007/BF02987182
Gase, G., Arndt, S., Bittner, H., Jennerjahn, M., Kürzinger, B., Podelleck, R., et al. (1990). Untersuchungen zum Einfluss der Temperatur auf die Bestimmung der quantitativen Virusresistenz der Kartoffel an In-vitro-Material. Arch. Phytopathol. Plant Protect. 26, 483–486. doi: 10.1080/03235409009439012
Gibson, R. W. (1991). The development of mature plant-resistance in four potato cultivars against aphid-inoculated potato-virus YO and YN in four potato cultivars. Potato Res 34, 205–210. doi: 10.1007/BF02358042
Gildemacher, P. R., Demo, P., Barker, I., Kaguongo, W., Woldegiorgis, G., Wagoire, W., et al. (2009). A description of seed potato systems in Kenya, Uganda and Ethiopia. Am. J. Potato Res. 86, 373–382. doi: 10.1007/s12230-009-9092-0
Gildemacher, P. R., Leeuwis, C., Demo, P., Borus, D., Schulte-Geldermann, E., Kinyae, P., et al. (2012). Positive selection in seed potato production in Kenya as a case of successful research-led innovation. Int. J. Technol. Manage. Sust. Dev. 11, 67–92.
Gildemacher, P. R., Schulte-Geldermann, E., Borus, D., Demo, P., Kinyae, P., Mundia, P., et al. (2011). Seed potato quality improvement through positive selection by smallholder farmers in Kenya. Potato Res. 54, 253–266. doi: 10.1007/s11540-011-9190-5
Gugerli, P. (1979). Le test immuno-enzymatique (elisa) et son application pour le diagnostic rapide des viroses de la pomme de terre. Rev. Suisse Agric. 11, 253–260.
Gugerli, P. (1986). “Potato viruses,” in Methods of Enzymatic Analysis, ed. H. U. Bergmeyer (Weinheim: FRG: VCH Verlagsgesellschaft GmbH), 430–446.
Haan, S. (2009). Potato Diversity at Height: Multiple Dimensions of Farmer-Driven in-Situ Conservation in the Andes. Wageningen: Wageningen Agricultural University.
Hooker, W. J. (1982). Virus Diseases of Potato. Technical Information Bulletin 19. Lima: International Potato Center (CIP), 17.
International Potato Center (CIP) (1986). Pathogen-Tested List of Varieties and Advanced Cultivars. Lima: International Potato Center.
International Potato Center (CIP) (1988). Annual Report CIP 1988. Lima: International Potato Center, 210.
International Potato Center (CIP) (1989). Annual Report CIP 1989. Lima: International Potato Center, 194.
Jayasinghe, U. (1988). Potato Leafroll Virus PLRV. Technical Information Bulletin 22. Lima: International Potato Center (CIP).
Jennings, D. L. (1960). Observations on virus diseases of cassava in resistant and susceptible varieties. I. Mosaic disease. Emp. J. Exp. Agric. 28, 23–34.
Martin-Hernandez, A. M., and Baulcombe, D. C. (2008). Tobacco rattle virus 16-kilodalton protein encodes a suppressor of RNA silencing that allows transient viral entry in meristems. J. Virol. 82, 4064–4071. doi: 10.1128/JVI.02438-07
Monasterios de la Torre, T. (1966). Presence of viruses in Bolivian potatoes. Turrialba 16, 257–260.
Pacumbaba, R. P. (1985). Virus-free shoots from cassava stem cuttings infected with cassava latent virus. Plant Dis. 69, 231–232. doi: 10.1094/PD-69-231
Parish, C. L., Zaitlin, M., and Siegel, A. (1965). A study of necrotic lesion formation by tobacco mosaic virus. Virology 26, 413–418. doi: 10.1016/0042-6822(65)90005-X
Pérez Barrera, W., Valverde Miraval, M., Barreto Bravo, M., Andrade-Piedra, J., and Forbes, G. A. (2015). Pests and diseases affecting potato landraces and bred varieties grown in Peru under indigenous farming system. Rev. Latinoam. Papa 19, 29–41.
Priegnitz, U., Lommen, W., Van der Vlugt, R., Onakuse, S., and Struik, P. (2014). “Improving seed potato quality in southwestern Uganda for strengthening food and cash security,” in Poster Presentation at the Tropentag 2014, Bridging the Gap Between Increasing Knowledge and Decreasing Resources, Prague.
Pumplin, N., and Voinnet, O. (2013). RNA silencing suppression by plant pathogens: defence, counter-defence and counter-counter-defence. Nat. Rev. Microbiol. 11, 745–760. doi: 10.1038/nrmicro3120
Qu, F., and Morris, J. T. (2005). Suppressors of RNA silencing encoded by plant viruses and their role in viral infections. FEBS Lett. 579, 5958–5964. doi: 10.1016/j.febslet.2005.08.041
Rajeevkumar, S., Anunanthini, P., and Sathishkumar, R. (2015). Epigenetic silencing in transgenic plants. Front. Plant Sci. 6:693. doi: 10.3389/fpls.2015.00693
Rossel, H. W., Thottappilly, G., Van Lent, J. M. W., and Huttinga, H. (1988). “The etiology of cassava mosaic in Nigeria,” in Proceedings of the International Seminar of African Cassava Mosaic Disease and its Control, eds C. Fauquet and D. Fargette (Wageningen: CTA/FAO/ORSTOM/IITA/IAPC), 43–56.
Sansregret, R., Dufour, V., Langlois, M., Daayf, F., Dunoyer, P., Voinnet, O., et al. (2013). Extreme resistance as a host counter-counter defense against viral suppression of RNA silencing. PLoS Pathog. 9:e1003435. doi: 10.1371/journal.ppat.1003435
Scheidegger, U. C., Bertschinger, L., Luther, K., Pinillos, O., Muñoz, J., and Hidalgo, A. (1996). El efecto de diferentes virus sobre el rendimiento potencial de la papa en la Sierra Central del Perú. Rev. Latinoam. Papa 7, 25–35.
Schulte-Geldermann, E., Gildemacher, P. R., and Struik, P. C. (2012). Improving seed health and seed performance by positive selection in three Kenyan potato varieties. Am. J. Potato Res. 89, 429–437. doi: 10.1007/s12230-012-9264-1
Stevenson, W. (2001). Compendium of Potato Diseases. Saint Paul MN: American Phytopathological Society.
Struik, P. C., and Wiersema, S. G. (1999). Seed Potato Technology. Wageningen: Wageningen Academic Publishers, 383.
Syller, J. (1991). The effects of temperature on the susceptibility of potato plants to infection and accumulation of potato leafroll virus. J. Phytopathol. 133, 216–224. doi: 10.1111/j.1439-0434.1991.tb00156.x
Syller, J. (1994). The effects of temperature on the availability and acquisition of potato leafroll luteovirus by Myzus persicae. Ann. Appl. Biol. 125, 141–145. doi: 10.1111/j.1744-7348.1994.tb04955.x
Syller, J. (2003). Inhibited long-distance movement of potato leafroll virus to tubers in potato genotypes expressing combined resistance to infection, virus multiplication and accumulation. J. Phytopathol. 151, 492–499. doi: 10.1046/j.1439-0434.2003.00758.x
Tamada, T., and Harrison, B. D. (1981). Quantitative studies on the uptake and retention of potato leafroll virus by aphids in laboratory and field conditions. Ann. Appl. Biol. 98, 261–276. doi: 10.1111/j.1744-7348.1981.tb00759.x
Thiele, G. (1999). Informal potato seed systems in the Andes: why are they important and what should we do with them? World Develop 27, 83–99. doi: 10.1016/S0305-750X(98)00128-4
Thomas-Sharma, S., Abdurahman, A., Ali, S., Andrade-Piedra, J. L., Bao, S., Charkowski, A. O., et al. (2016). Seed tuber degeneration in potato: the need for a new research and development paradigm to mitigate the problem in developing countries. Plant Pathol. 65, 3–16. doi: 10.1111/ppa.12439
Voinnet, O., Pinto, Y. M., and Baulcombe, D. C. (1999). Suppression of gene silencing: a general strategy used by diverse DNA and RNA viruses of plants. PNAS 96, 14147–14152. doi: 10.1073/pnas.96.24.14147
Whitworth, J. L., Mosley, A. R., and Reed, G. L. (2000). Monitoring current season potato leafroll virus movement with an immunosorbent direct tissue blotting assay. Am. J. Potato Res. 77, 1–9. doi: 10.1007/BF02853656
Wislocka, M. (1982). Einfluss der Trockenheit vor und zu verschiedenen Zeitpunkten nach der Inokulation auf den Knollenbefall der Kartoffelsorte ’Uran’ mit Kartoffelvirus Y. Potato Res. 25, 293–298. doi: 10.1007/BF02357287
Yamaguchi, A. (1960). Increased respiration of leaves bearing necrotic local virus lesions. Virology 10, 287–293. doi: 10.1016/0042-6822(60)90020-9
Young, N. (1990). Seed Potato Systems in Developed Countries: Canada, the Netherlands and Great Britain. Lima: Peru International Potato Center (CIP).
Keywords: autoinfection, plant viruses, seed potato systems, seed degeneration, food security, climate change, epigenetics, gene-silencing
Citation: Bertschinger L, Bühler L, Dupuis B, Duffy B, Gessler C, Forbes GA, Keller ER, Scheidegger UC and Struik PC (2017) Incomplete Infection of Secondarily Infected Potato Plants – an Environment Dependent Underestimated Mechanism in Plant Virology. Front. Plant Sci. 8:74. doi: 10.3389/fpls.2017.00074
Received: 13 July 2016; Accepted: 12 January 2017;
Published: 03 February 2017.
Edited by:
Laurent Gentzbittel, Institut National Polytechnique de Toulouse – Ecole Nationale Supérieure Agronomique de Toulouse, FranceReviewed by:
Sébastien Guyader, Institut National de la Recherche Agronomique (INRA), GuadeloupeCopyright © 2017 Bertschinger, Bühler, Dupuis, Duffy, Gessler, Forbes, Keller, Scheidegger and Struik. This is an open-access article distributed under the terms of the Creative Commons Attribution License (CC BY). The use, distribution or reproduction in other forums is permitted, provided the original author(s) or licensor are credited and that the original publication in this journal is cited, in accordance with accepted academic practice. No use, distribution or reproduction is permitted which does not comply with these terms.
*Correspondence: Lukas Bertschinger, bHVrYXMuYmVydHNjaGluZ2VyQGFncm9zY29wZS5hZG1pbi5jaA==
†Tribute: In honor and memory of the Late Professor Ernst R. Keller (1921–1999).
Disclaimer: All claims expressed in this article are solely those of the authors and do not necessarily represent those of their affiliated organizations, or those of the publisher, the editors and the reviewers. Any product that may be evaluated in this article or claim that may be made by its manufacturer is not guaranteed or endorsed by the publisher.
Research integrity at Frontiers
Learn more about the work of our research integrity team to safeguard the quality of each article we publish.