- Key Laboratory of Crop Physiology and Ecology, Ministry of Agriculture, Nanjing Agricultural University and Jiangsu Collaborative Innovation Center for Modern Crop Production, Nanjing, China
Cottonseed is widely used as a source of ruminant feed and for industrial purposes. Therefore, there is a tremendous need to improve the nutritional value of cotton embryos. In this study, a conventional management (CM) and two integrated cotton management strategies (IMS1, IMS2) were performed at two soil fertility levels to study the relationships among soil N, N assimilation, embryonic protein accumulation and protein quality. The levels of proteins, essential amino acids, and semi-essential amino acids, especially those of glutamate, lysine, and methionine, were higher in IMS1 and IMS2 embryos than in CM embryos. These changes were significantly positively correlated with the soil-available N content, glutamine synthetase activity and peak value of protein accumulation rate and were negatively correlated with the free amino acid level. These results illustrated that integrated management strategies, especially the rates and timing of N application, raise the level of soil available N, which is beneficial for N assimilation in developing cotton embryos. The protein content was limited by the rate of protein accumulation rather than by the free amino acid content. The combination of target yield fertilization, a growth-driven N application schedule, a high plant density and the seedling raising with bio-organic fertilizer can substantially improve protein quality in cotton embryos, especially at a soil with low soil organic matter and total nitrogen.
Introduction
Cotton embryos accumulate 40%–55% of their dry weight (DW) as storage protein, which has a substantial effect on their nutritional value (Alford et al., 1996; Mujahid et al., 2000). Therefore, evaluating the mechanisms controlling storage protein accumulation and protein quality in these embryos is crucial step for improving seed quality. Recently, considerable progress has been made in elucidating the relationships among genotypes, environmental conditions and management practices, suggesting that protein content is a variety-specific quantitative characteristic (Yu et al., 2012) and that it is substantially influenced by environmental conditions (Li et al., 2009; Rotundo and Westgate, 2009) and crop management strategies (Sawan et al., 1993, 2001, 2006, 2009). Numerous studies show the many steps involved in protein accumulation in cottonseed embryos, involving seed biochemistry (Hodges, 2002; Hernandez-Sebastia et al., 2005; Zhang et al., 2014), N transport with the plant and from the soil (De Ruiter et al., 1986; Kullmann and Geisler, 1986; Crawford and Glass, 1998), soil N levels and the environment (Macduff and Hopper, 1986). Integration of the results of these studies into a complete management system targeting the final result of protein quality in cotton embryos is still needed.
Integrated management strategies are combinations of agronomic practices applied to achieve high yields, optimal quality, and long-term sustainability based on analyses of factors limiting the productivity and quality of crops under a given set of environmental conditions. The planting of high-yielding cultivars with optimal nutritional management (Sapkota et al., 2014) and water management strategies (Falkenberg et al., 2007), adequate plant populations (Dong et al., 2012), and strong seedlings (Mcgraw and Shaver, 2006) are major requirements for high yields and optimal quality. Integrated nutrient management improves the soil productivity, crop yield and quality of groundnuts (Prasad et al., 2002) and tomatoes (Javaria and Khan, 2011). Whether IMS can also improve protein quality in cotton embryos requires further elucidation.
A considerable amount of data is available regarding the “source-sink” relationships in the study of embryonic protein synthesis (Martre et al., 2003; Seong et al., 2004; Zhang et al., 2012), whereas the relationships between soil N and embryonic proteins are unclear. AV-N (i.e., the sum of -N and
-N), as an indicator of soil N and fertility, can be directly utilized by crop roots (Crawford and Glass, 1998) and ultimately incorporated into storage protein (Kullmann and Geisler, 1986). Soil N increased with increasing fertilization rate (Sainju et al., 2006). Integrated nutritional management (Javaria and Khan, 2011; Singh et al., 2014) and soil temperature (Macduff and Hopper, 1986) can influence seasonal and regional variations in the soil available N. Moreover, soil N also regulates N uptake and plant growth (Gastal and Lemaire, 2002). Therefore, it is necessary to explore the relationship between soil N and protein content.
Some of the crucial steps in initial N assimilation are the incorporation of N into carbon skeletons of storage proteins and its use for the biosynthesis of glutamine and then of Glu (Hodges, 2002). Glutamine synthetase (GS) and glutamate synthase (GOGAT) play a key role in N assimilation for its higher affinity to -N than glutamate dehydrogenase (GDH) (Stewart and Rhodes, 1978; Hodges, 2002; Hodges et al., 2003; Muro-Pastor and Florencio, 2003; Muro-Pastor et al., 2005; Potel et al., 2009). N assimilation uses ferredoxin (Fd-GOGAT, EC 1.4.7.1) as an electron carrier in photorespiratory tissues (i.e., leaf samples) and NADH (NADH-GOGAT; EC 1.4.1.14) as an electron carrier in non-photorespiratory tissues (i.e., roots and seeds) (Canovas et al., 1998; Tamura et al., 2010; Konishi et al., 2014). The
-N and
-N concentrations in the soil tightly regulate the GS and GOGAT activities (Zhao and Shi, 2006). Although the functional roles of GS (EC 6.3.1.2) and GOGAT (EC 1.4.1.14) in plant roots and leaves have been well described (Ishiyama et al., 1998; Muro-Pastor and Florencio, 2003; Potel et al., 2009), the role of nitrogen assimilation in non-photosynthetic organs is unclear, especially in protein-enriched cotton embryos.
Cotton embryos are characterized by high protein content and are rich in Lys and Glu, which serve as nutritional limiting factors for ruminants (Bertrand et al., 1998; Zhe et al., 2014). The globulin null soybean mutant accumulates high levels of free amino acids (Takahashi et al., 2003), suggesting a role of free amino acids in storage protein accumulation. Varietal and locational factors may influence the contents of free amino acids and storage protein in cottonseeds (Ikurior and Fetuga, 1987). A fundamental understanding of the conversion of free amino acids into storage proteins and the characterization of protein accumulation would clarify the factors limiting the protein accumulation rate.
The objective of the present study was to improve protein quality in cotton embryos by adopting IMS and to analyze how these strategies improve protein quality in terms of soil N, N assimilation, the protein accumulation rate, proteinogenic amino acids, and the protein content. The initial hypothesis was that the combination of optimal N management practices, an adequate plant density and strong seedlings could be beneficial to protein quality in cotton embryos.
Materials and Methods
Growth Conditions and Integrated Management Strategies
Field experiments were carried out at the Dafeng Experimental Station, Jiangsu, China (120°45′ E, 33°19′ N), using a widely grown cotton cultivar, Siza-3 (Gossypium hirsutum L.), in 2012 and 2013. The soil at the experiment site was a typical sandy loam, and there were significant differences in the contents of organic matter and total nitrogen (Table 1), with low-fertility soil containing an average of 14.31 ± 0.62 g kg-1 soil organic matter and 0.79 ± 0.04 g kg-1 total nitrogen and high-fertility soil containing an average of 17.76 ± 0.69 g kg-1 soil organic matter and 0.86 ± 0.03 g kg-1 total nitrogen at a 0–20 cm depth of the soil profile.
Cotton plants were planted using a CM system and two integrated management strategies (IMS1 and IMS2). A randomized complete block design with three replicates was used in a plot measuring 22 m long and 10 m wide. CM was a widely used practice in the Yangtze River cotton-producing region (i.e., 300 kg N ha-1 + 18,000 plants ha-1 + seedling transplantation, with 40% of the 300 kg N ha-1 applied as a basal fertilizer and 60% applied at the initial flowering stage). The two integrated management strategies (IMS1 and IMS2) included different combinations of N rates, N application schedules, plant densities, and seedling-raising methods. IMS1 included an economic N rate, a high plant density and substrate seedling-raising method (i.e., 375 kg N ha-1 + 30,000 plants ha-1 + substrate seedling raising). Compared to the CM, in IMS2, the N rate was increased to 525 kg N ha-1, the plant density was increased to 30,000 plants ha-1, and seedling transplantation was adopted (i.e., target yield fertilization + higher plant density + seedling transplantation).
Target yield fertilization and the economic N rate were calculated according to a previously described algorithm (Katayama et al., 1999; Baker and Young, 2004). The N application schedules of IMS1 and IMS2 were timed to coincide with growth-driven N demand of cotton plants, i.e., 20% applied as a basal fertilizer, 25% at the initial flowering stage, 40% at the full-bloom stage and 15% at the end of the flowering stage. N, P, and K were applied as urea (46% N), ordinary superphosphate (12% P2O5 and 12% sulfur) and potassium sulfate (50% K2O and 18% sulfur) at a ratio of 1.0:0.6:1.1. For substrate seedling raising, seeds were planted with bio-organic fertilizer (20 million g-1 efficacious living-cell and 43.6% organic matter, purchased from Jiangsu Tianniang Ltd., China) at the rate of 10 g per seedling, and seedlings were transplanted in the field with a bio-organic fertilizer on May 15th (3–4 true leaves). For seedling transplantation, cottonseeds were planted in a nursery bed, and then seedlings with 3–4 true leaves were transplanted in the field without bio-organic fertilizer on May 15th. Integrated pest management and furrow irrigation were applied to avoid any biotic and abiotic stresses during the cotton growth period.
Sampling
White flowers from first fruiting nodes at the 7th–8th sympodial branches were tagged with the flowering date to ensure that boll samples in each treatment were collected at equivalent metabolic and developmental boll ages. Tagged boll samples were collected once every 7 days from 17 days after anthesis (DAA) until boll opening from 9:00 to 10:00 a.m. local time. Collected bolls were quickly separated into fibers, carpels, seed coats and embryos at 4°C. About half of the embryo samples were placed in liquid nitrogen and stored at -80°C for assays of GS and NADH-dependent GOGAT. The other half of embryo samples were dried to a constant weight, and ground to pass through a 1 mm sieve, and then used to determine the free amino acid and protein contents, as well as the compositions of proteinogenic amino acids.
Soil Available Nitrogen
Fresh and uniformly mixed soil samples collected from 0–20 cm to 20–40 cm depths in each plot were assayed immediately. Soil samples (5 g) were extracted with 50 ml of 0.01 M CaCl2 for 1 h using a shaking table. A continuous flow analyser (Bran and Luebbe TRAACS Model 2000 Analyzer) was used for determinations of the soil nitrate-nitrogen ( -N) and ammonium nitrogen (
-N) levels (Dahnke and Johnson, 1990). The soil-available nitrogen level was calculated as the sum of the nitrate-nitrogen (
-N) and ammonium nitrogen (
-N) levels.
GS and NADH-Dependent GOGAT Assays
The extraction and measurement of GS (EC 6.3.1.2) and NADH-GOGAT (EC 1.4.1.14) were performed according to the method of Lea et al. (1990). Frozen embryo tissues (0.3 g) were homogenized in 5 ml buffer containing 50 mM KH2PO4 (pH 7.2), 2 mM EDTA, 2 mM DTT, 5% (v/v) glycerol, and 1% (w/v) insoluble PVPP. After centrifugation at 12,000 × g for 20 min at 4°C, the supernatant homogenates were stored at 4°C for assays of GS, NADH-dependent GOGAT and soluble protein, which were conducted immediately.
GS Assay
The standard assay mixture (1.6 ml) contained 0.15 mM imidazole buffer (pH 7.0), 0.010 mM MgSO4, 0.12 mM Glu-Na, and 0.012 mM ATP-Na. After adding 1.2 ml crude enzyme and heating for 5 min at 25°C, the reaction was initiated by 0.2 ml of 1 M NH2OH for 15 min at 25°C. The amount of γ-glutamyl hydroxamate (GH) generated was determined by adding 0.8 ml ferric chloride reagent (0.37 mM FeCl2, 0.67 mM HCl and 0.2 mM trichloroacetic acid) and spectrophotometrically measuring absorbance at 540 nm. The activity of GS is expressed as micromoles of γ-glutamyl hydroxamate formed per milligram of soluble protein per hour.
NADH-GOGAT Assay
NADH-dependent GOGAT was assayed at 340 nm by coupling the reaction to NADH oxidation mediated by reductive amination of α-ketoglutarate at saturating substrate concentrations (Muhitch, 2006). The reaction mixture contained 0.1 M Tris-HCl (pH 8.5), 0.2 M α-ketoglutarate, 1.0 mM CaCl2, and 0.2 mM NADPH. After heating to 25°C, the reaction was initiated by adding monosodium Glu to a final volume 2.1 ml. After incubating the mixture in a water bath at 30°C for 30 min, the rate of decline in the NADH concentration was determined using a spectrophotometer at 340 nm. The activity of GOGAT is expressed as micromoles of NADH per milligram of soluble protein per hour.
Soluble Protein Assay
Soluble protein was quantified using the Bradford protocol (Bradford, 1976). The standard mixture contained 0.01% (w/v) Coomassie brilliant blue (G-250), 4.7% (w/v) ethanol and 8.5% (w/v) phosphoric acid. After adding 50 μL of extract to the standard mixture in a total volume of 5 ml, the contents were mixed thoroughly, and absorbance was spectrophotometrically measured at 595 nm against a blank reagent at the indicated time points, ranging from 2 to 30 min. Soluble protein was quantified using a standard soluble protein (Bovine Serum Albumin).
Free Amino Acid Content
Free amino acids were extracted from dried and powdered cotton embryo tissues (0.1 g) with 5 ml of 80% ethanol in a water bath at 80°C for 30 min (Hendrix, 1993). After three rounds of extraction, the samples were diluted to a final volume of 25 ml. Assays were performed in a 96-well polystyrene plate with a Benchmark microplate reader (Bio-Rad). The free amino acid content is expressed as mg g-1 DW.
Protein Content and Proteinogenic Amino Acid Profiles
Protein content was calculated as the product of the 100-embryo weight (g), N concentration (%) and 6.25. Proteinogenic amino acids were isolated from dried and powdered cotton embryos (30 mg) via acidolysis with 10 ml of 6 M HCl for 24 h at 110°C. The solution was diluted to a final volume of 50 ml with 0.02 M HCl. Profiles of proteinogenic amino acids were determined using an automatic amino acid analyzer (L8900, Hitachi, Tokyo, Japan) (Hao et al., 2014).
A sigmoid growth curve was used to assess the accumulation of storage proteins in developing cotton embryos, where embryo protein is the protein content (g 100 embryos-1) at developmental time (d), Pmax is the protein content at maturity, a and b are constants, DAA1 is the start time of rapid embryo protein accumulation, and DAA2 is the termination time of rapid embryo protein accumulation. The duration is the difference (in days) between the two dates.
Statistical Analysis
Three-way analysis of variance was performed on at least three replicates to examine the effects of years, fertility levels, IMS and their interactions on the composition of proteinogenic amino acids. The measurements are expressed as the mean ± SE. Fisher’s least significant difference (LSD) test was used for statistical analyses. Statistical significance is indicated by P < 0.05 or by P < 0.01. SAS software was used for principal component analysis (PCA), and graphs were plotted using Origin software, version 9.0.
Results
Protein Quality in Cotton Embryos
When seeds were mature, the cotton embryos accumulated 42.7–46.9% of their DW as storage protein. The protein contents ranged (g 100 embryos-1) from 2.18–2.68 g in 2012 to 2.63–3.30 g in 2013 (Table 2). The protein contents in the IMS1 and IMS2 embryos were 14.5 and 24.3% higher at low soil fertility levels and 3.3 and 12.7% higher at high soil fertility levels, respectively, compared to those in the CM embryos (P < 0.05).
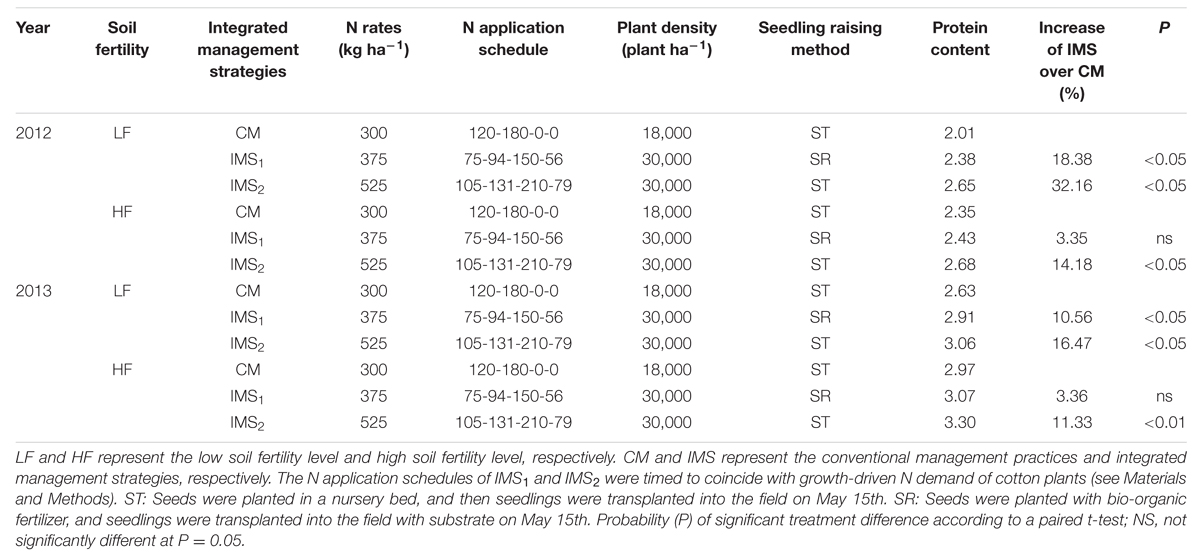
TABLE 2. Mean protein content (g 100 embryos-1) of recommended integrated crop management (ICM) as compared with conventional management practice (CM) for cottonseed embryos in 2012 and 2013.
The protein content increased significantly with increases in the N rate and plant density (Table 2). The application of 20% N as basal fertilizer, 25% N at the initial flowering stage, 40% N at the full-bloom stage and 15% N at the end of the flowering stage showed advantages over the N application schedule of CM. The seedling-raising method also had beneficial effects on the protein content.
The acidolysis of proteinogenic amino acids from storage proteins indicated that the contents of total amino acids and each proteinogenic amino acid in the IMS1 and IMS2 embryos were increased compared to those in CM (Table 3). The contents of total proteinogenic, essential, semi-essential and non-essential amino acids in IMS2 increased by averages of 7.61, 8.04, 4.64, and 8.32%, respectively, whereas they did not significantly differ between IMS1 and CM. Although, the soil fertility level did not affect the composition of proteinogenic amino acids, the interaction effect between fertility level and IMS was significant (p < 0.05, Table 4), demonstrating IMS did not only affect proteinogenic amino acids profiles, but they also have a beneficial effect on soil fertility level.
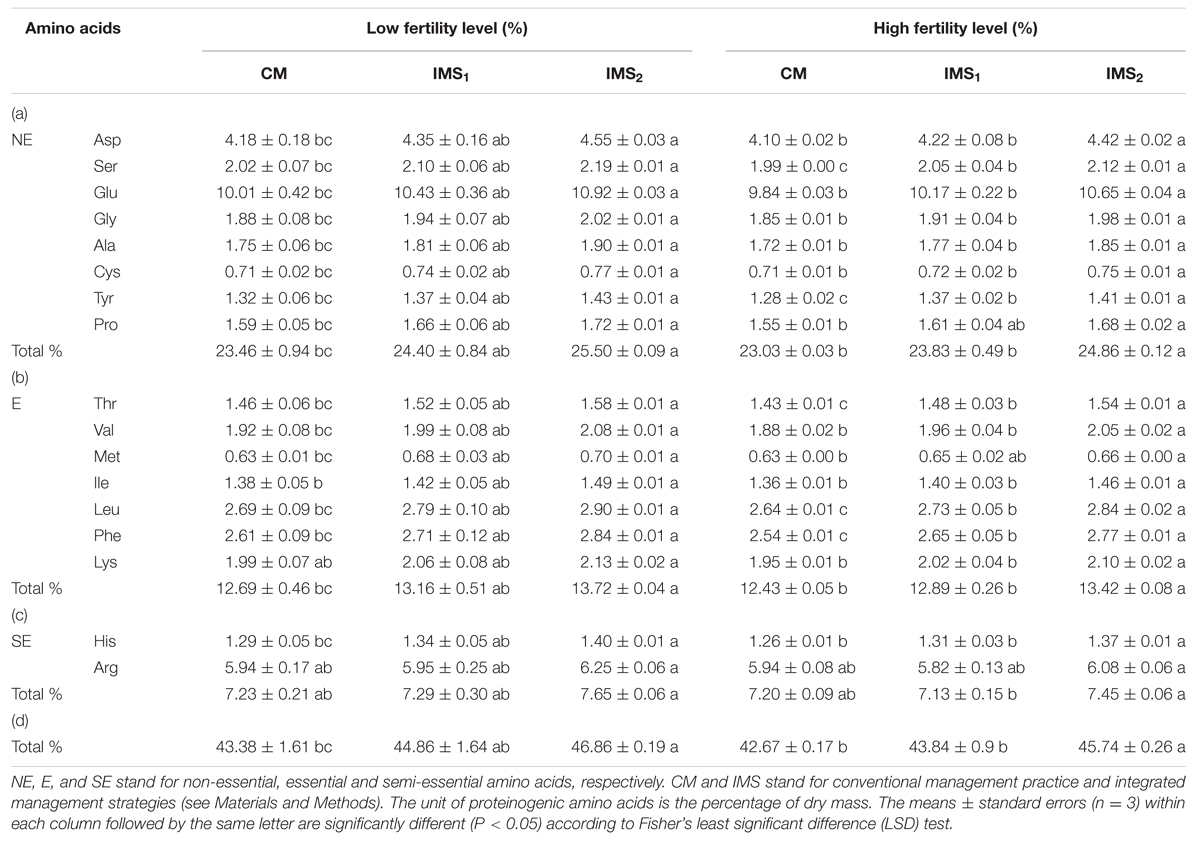
TABLE 3. Proteinogenic amino acid contents of cotton embryos under conventional management practices and two integrated management strategies at two fertility levels.
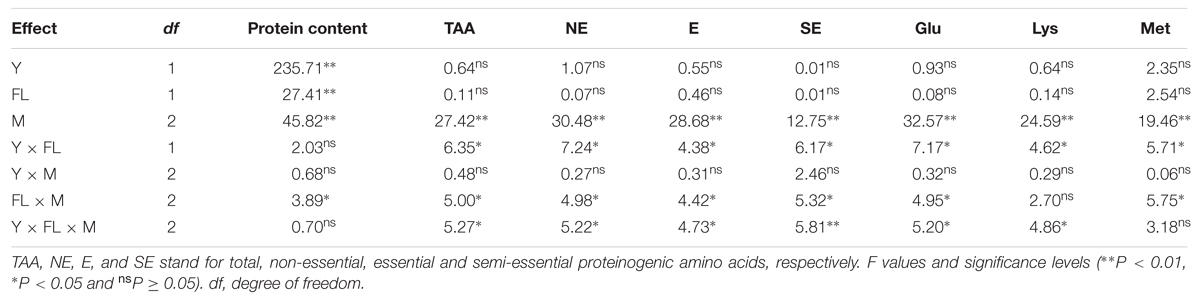
TABLE 4. Results of ANOVA on the effects of year (Y), fertility levels (FL), integrated management strategies (M) and their interactions on protein content and proteinogenic amino acids in cotton embryos in 2012 and 2013.
Glu, Lys, and Met are nutritional limiting factors for ruminants fed cottonseed kernel protein flour. The contents of Glu, Lys, and Met in IMS2 were increased by 4.7, 2.9, and 3.4% at a low fertility level and by 4.7, 1.5, and 4.0% at a high fertility level, respectively, compared to those embryos in CM (Figure 1). The ratio of each amino acid to the total proteinogenic amino acid content was relatively stable. These results indicate that the IMS increased the levels of proteinogenic amino acids but did not lead to an alteration in the level of any proteinogenic amino acid.
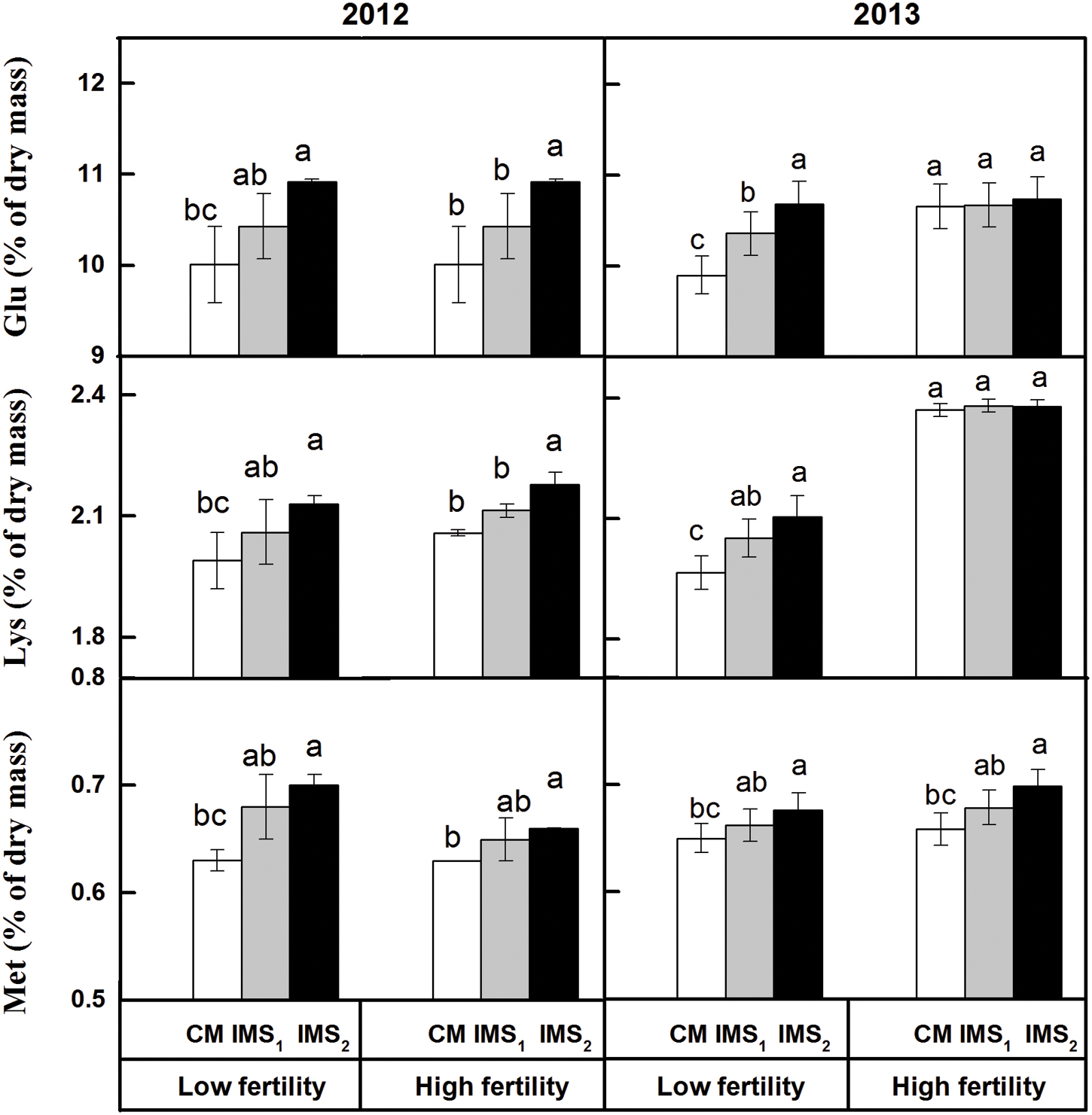
FIGURE 1. Contents of Glu, Lys and Met in cotton embryos under conventional management practices and two integrated management strategies at two fertility levels in 2012 and 2013. The data points represent the means, and the bars are the SEs. CM and IMS stand for conventional management practice and integrated management strategies (see Materials and Methods). Different letters indicate significant difference at the level of p < 0.05.
Soil-Available Nitrogen Content
Regardless of the soil fertility levels or soil depth, the contents of -N and
-N were consistently significantly higher in IMS1 and IMS2 than in CM at soil depths of 0–20 cm and 20–40 cm (Figures 2A,B). Furthermore, the
-N and
-N contents at the initial flowering stage were higher than those at the end of flowering and full-bloom stages. IMS2 exhibited the highest level of AV-N (sum of
-N and
-N). Compared with CM, the contents of AV-N in IMS1 and IMS2 increased by averages of 6.37 and 16.86%, respectively, at soil depths of 0–20 cm and by 9.72 and 21.52%, respectively, at soil depths of 20–40 cm. These results indicated that soil N availability was significantly increased in both IMS1 and IMS2 compared with CM.
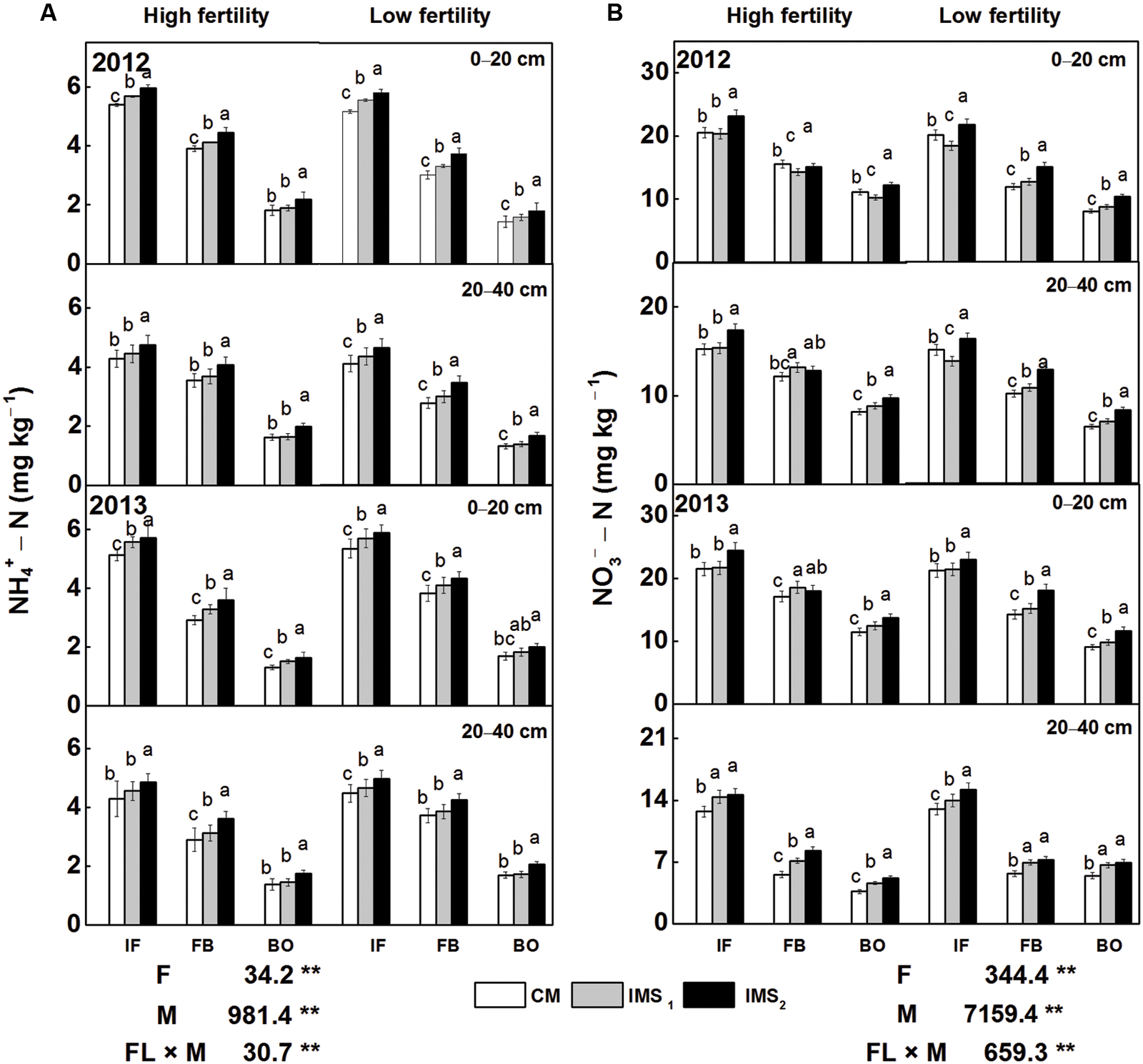
FIGURE 2. Soil -N (A) and
-N (B) levels under conventional management practices and two integrated management strategies at two fertility levels at soil depths of 0–20 cm and 20–40 cm in 2012 and 2013. CM and IMS stand for conventional management practices and integrated management strategies (see Materials and Methods). IF, BF, and BO stand for the initial flowering stage, full-bloom stage, and boll-opening stage, respectively. Different letters indicate significant difference at the level of P < 0.05.
GS and NADH-Dependent GOGAT in Developing Cotton Embryos
The enzymatic activities of GS and NADH-dependent GOGAT showed similar patterns of alterations, with their activities declining according to the duration of embryo growth in 2012 and 2013 (Figure 3). Compared with CM, GS activity increased during IMS1 and IMS2 embryo growth, with average increases of 4.5 and 13.6% at high fertility levels and of 11.2 and 16.5% at low fertility levels, respectively. Further, the NADH-dependent GOGAT activities in IMS1 and IMS2 increased by 13.9 and 24.1% at high fertility levels and by 9.1 and 20.6% at low fertility levels, respectively. These results indicated that the IMS enhanced the initial N assimilation in the developing cotton embryos.
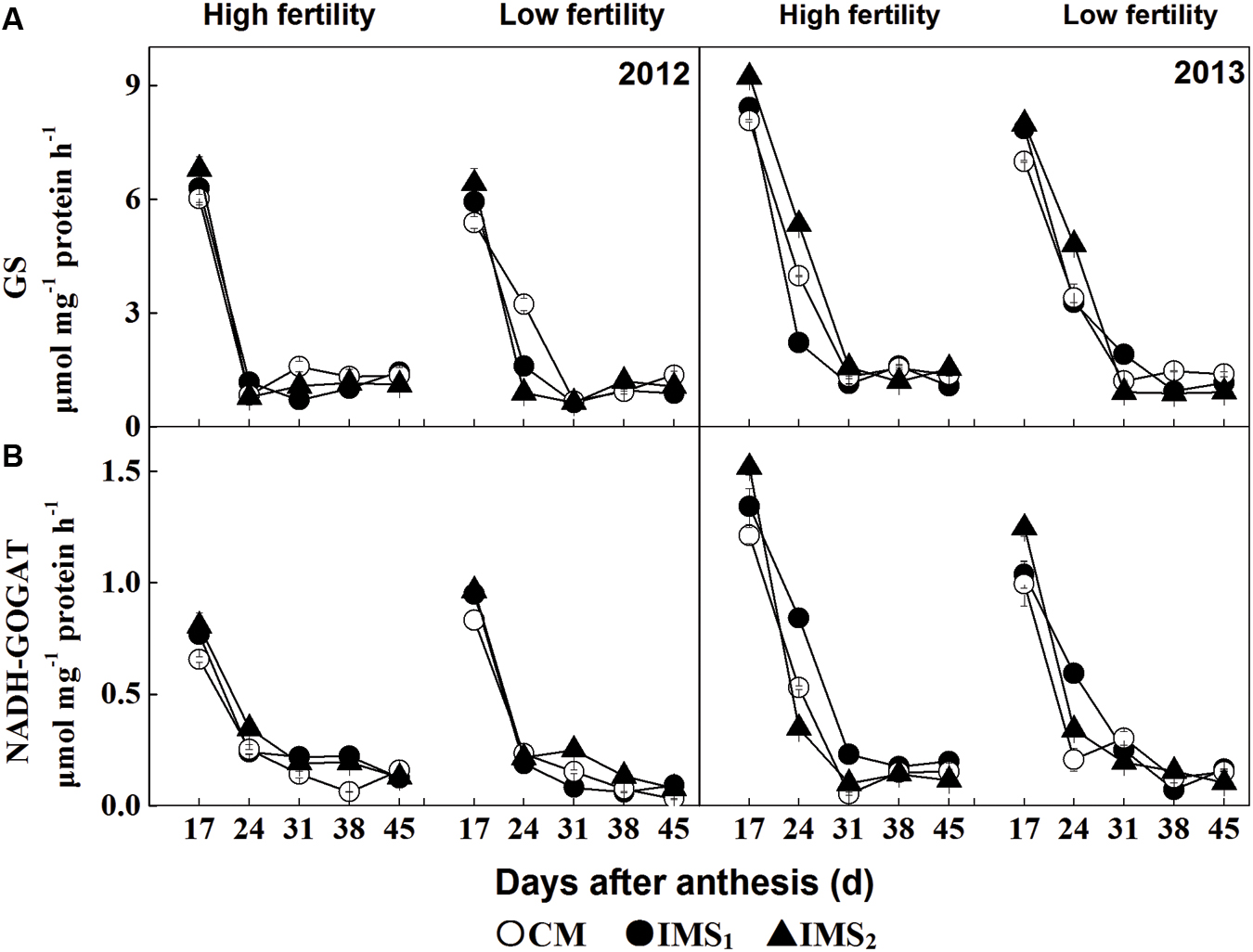
FIGURE 3. Developmental profiles of glutamine synthetase (A) (GS, μmol γ-glutamyl-hydroxamate h-1 g-1 soluble protein) and (B) NADH-dependent glutamate synthase (GOGAT, μmol NADH h-1 g-1 soluble protein) for conventional management practices and two integrated management strategies at two fertility levels in 2012 and 2013. For each sample point, we tested at least three independent samples. The data points represent the means, and the bars are the SEs. CM and IMS stand for conventional management practices and integrated management strategies (see Materials and Methods).
Free Amino Acid Supply and Protein Accumulation in Developing Cotton Embryos
Free amino acids are precursors to storage proteins and are incorporated into proteins during the growth of cotton embryos. The free amino acid content increased sharply after 17 DAA, rising from a very low level to a maximum level at 24 DAA and then declining (Figure 4A). This content decreased dramatically, accompanied by rapid protein accumulation in the cotton embryos after 24 DAA. Moreover, at 24 DAA, the free amino acid content was significantly higher in CM than in IMS1 or IMS2.
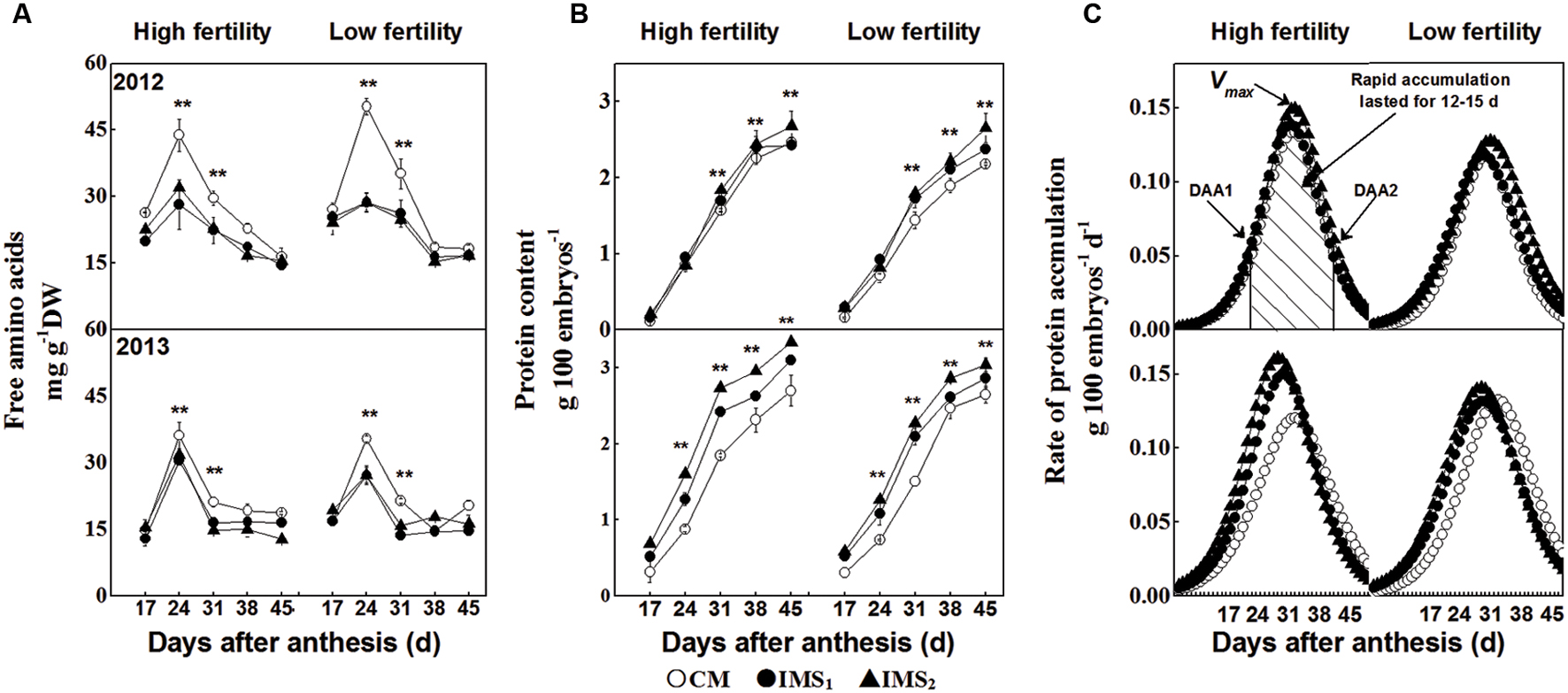
FIGURE 4. The free amino acid contents (A) and protein accumulation (B,C) in cotton embryos under conventional management practices and two integrated management strategies at two fertility levels in 2012 and 2013. The data points represent the means, and the bars are the SEs. Characterization of proteins accumulated in the cotton embryos was calculated using sigmoid growth curves (see Materials and Methods). CM and IMS stand for conventional management practice and integrated management strategies (see Materials and Methods). ∗ and ∗∗ denotes significant at the 0.05 level and 0.01 level, respectively.
Sigmoid growth curves, used to quantify the rate of protein accumulation (g 100 embryos d-1), may reveal the reasons and key stages associated with the differences in protein content among integrated management treatments (Figures 4B,C). Rapid embryo protein accumulation began at 17–22 DAA and terminated at 32–37 DAA, lasting from 11 to 15 days in 2012 and 2013 (Table 5). The peak rate of protein accumulation was positively correlated with the protein content at maturity. Change tendency of the protein content was opposite to that of the free amino acids. Accumulation of a large amount of protein coincided with a rapid decrease in the free amino acid content, and evaluation of the different management treatments revealed that the protein content was limited by the protein accumulation rate rather than by the free amino acid content.
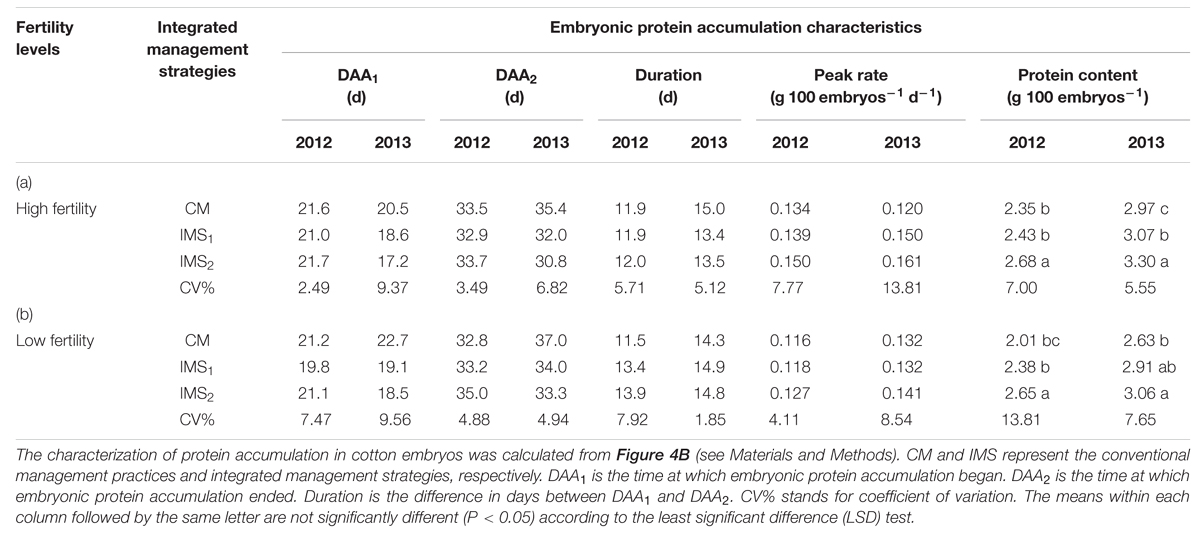
TABLE 5. Protein accumulation characteristics of cotton embryos under conventional management practices and two integrated management strategies at two fertility levels in 2012 and 2013.
Protein Quality and Relationships
The protein content was positively and significantly correlated with the content of soil available N, GS activity and peak rate of rapid protein accumulation, whereas it was negatively correlated with the free amino acid content (Table 6). Similar relationships were also observed for the levels of total proteinogenic amino acids, essential amino acids, semi-essential amino acids, Glu, Lys and Met.
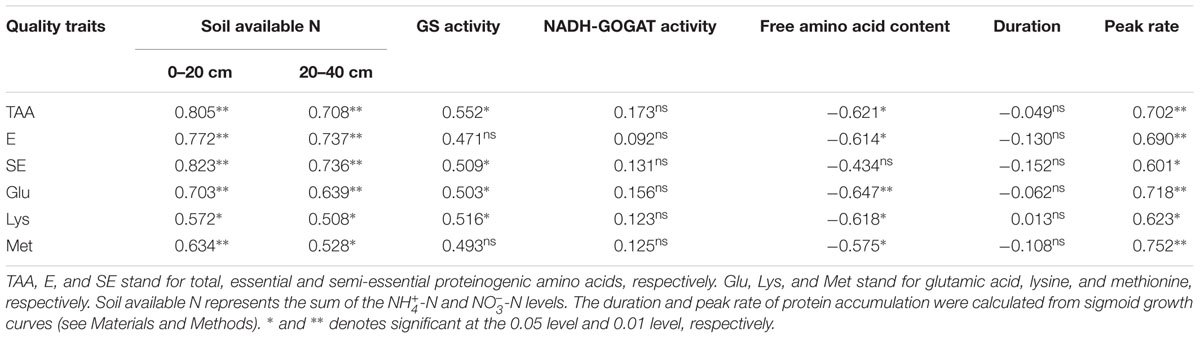
TABLE 6. Correlations among protein traits, soil available N, N assimilation and characterization of protein accumulation.
In PCA, the first two principal components explained 61.4% and 18.5% of the total variance in the sample set, respectively, whereas the third principal component only accounted for 7.5% of the variance (Figure 5). Essential amino acids, semi-essential amino acids, Glu, Lys and Met were clustered as a group in the score plot. The content of soil available N and the peak rate of protein accumulation were most closely related to the proteinogenic amino acid profiles in the score plot. These results indicated that the improvements in the nutritional value of the cotton embryos observed with use of the IMS could be attributed to increases in soil N availability, the initial step of N assimilation and the conversion of free amino acids into storage proteins (Figure 6).
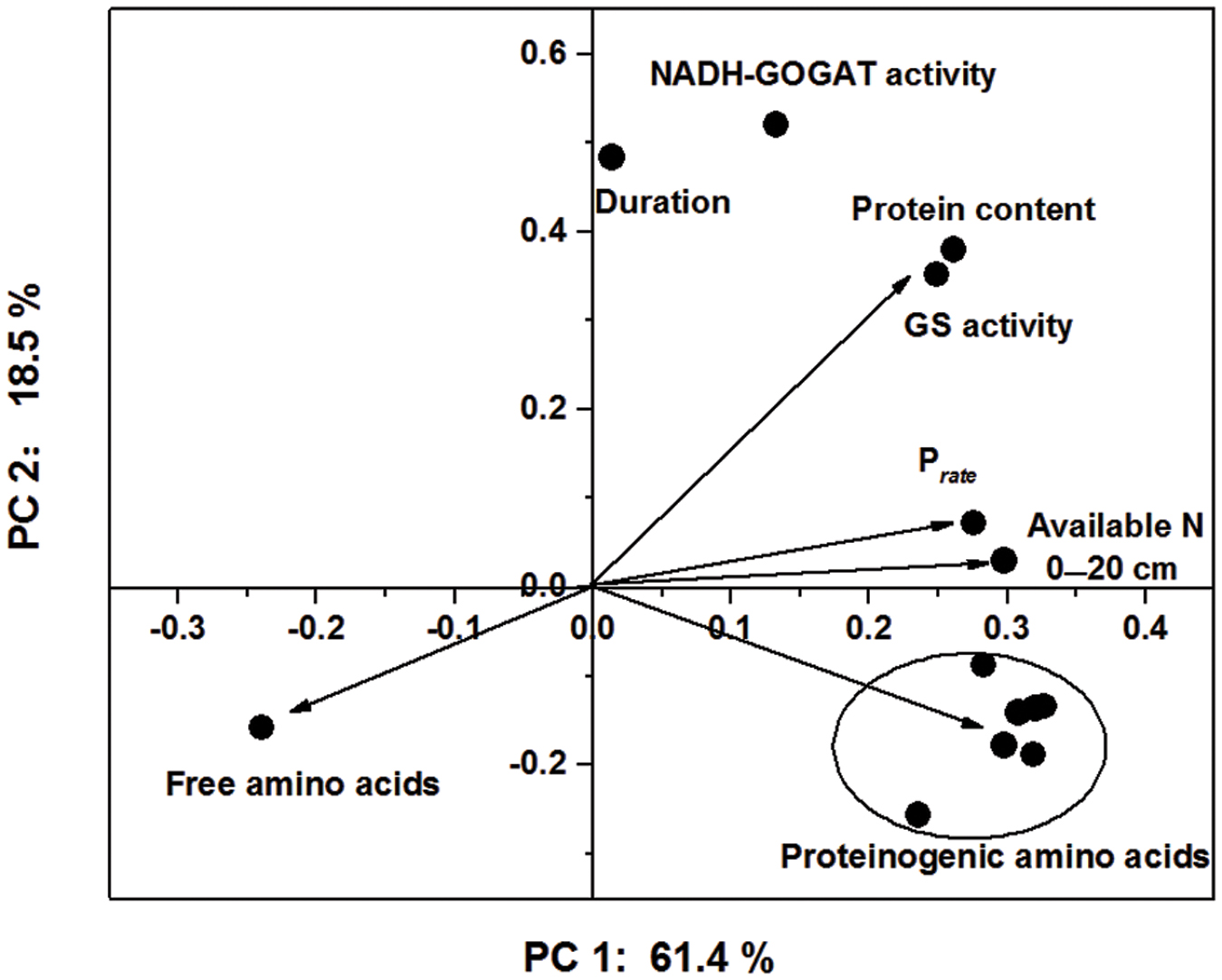
FIGURE 5. Principal component analysis of the soil-available N content, N assimilation, rate of protein accumulation and proteinogenic amino acid profile based on the first two principal components (PC 1: principal component 1; and PC 2: principal component 2). The arrows represent the principal component loadings, and the ellipses represent the proteinogenic amino acid profiles.
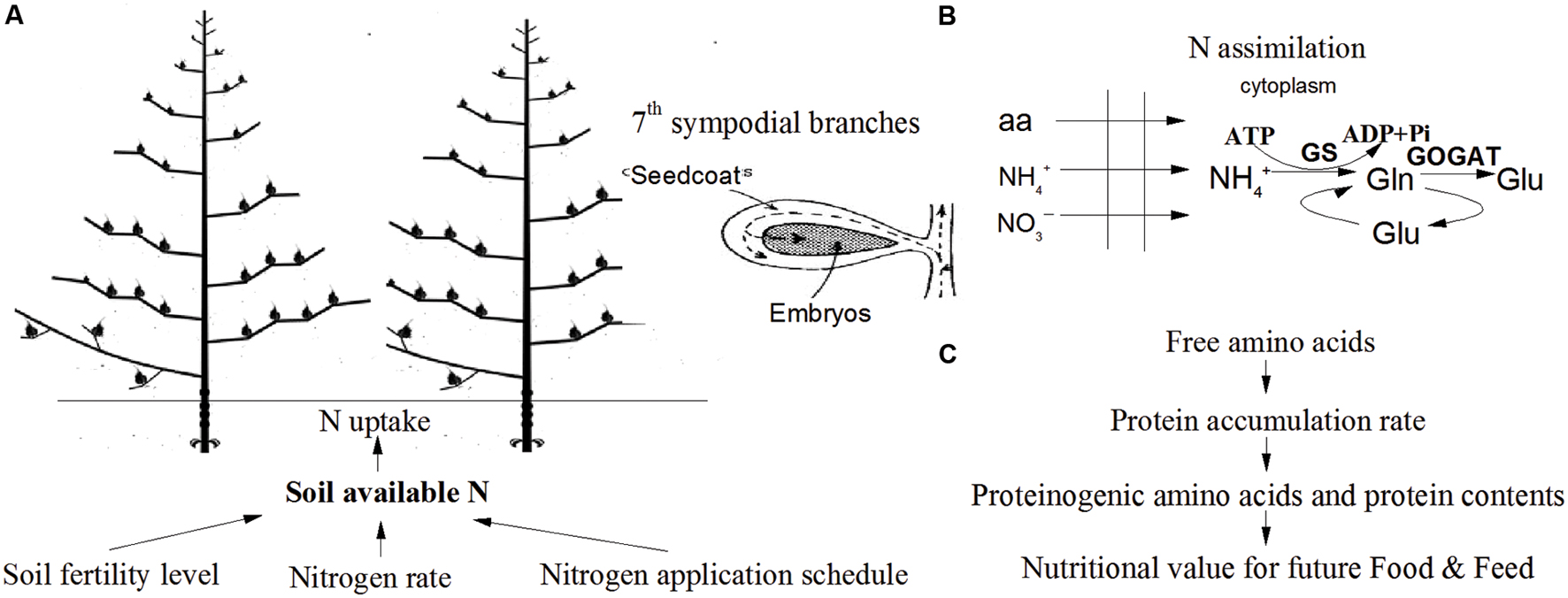
FIGURE 6. Schematic overview of the integrated management strategies on protein quality of cotton embryos. (A) Integrated management strategies increases the soil available N content. (B) Nitrogen uptake, translocation and assimilation were enhanced by soil available N content. (C) Cotton embryonic protein content was limited by the conversion of free amino acids into storage protein rather than by the free amino acid content.
Discussion
The initial question that motivated us to perform this study was whether matching a high-yielding variety with IMS would prove useful in improving the nutritional value of cotton embryos. We were also particularly interested in the following questions: What improves their nutritional value? What is the physiological basis of high quality? What are the guidelines for increasing crop quality in other cotton-producing regions?
Most of the previous studies are focusing on the developing high fiber yield and optimal fiber propriety through crop breeding (Cai et al., 2014; Guan et al., 2014; Ning et al., 2014), stress-resistance cultivation (Kawakami et al., 2012; Liu et al., 2015; Kuai et al., 2016), and crop management (Kawakami et al., 2012). However, high-quality and nutritional value-added cottonseed by improving management practices can increase crop yields and improve farmers’ livelihoods dramatically. In this study, the levels of proteins, essential amino acids, and semi-essential amino acids, especially those of Glu, Lys, and Met, were higher in IMS1 and IMS2 embryos than in CM embryos (Tables 2 and 3; Figure 1). In addition, fiber yield, seed yield, seed weight were also increased dramatically in IMS1 and IMS2 treatments (data not given). The impact of IMS on cotton embryo protein quality was more obvious at a low soil fertility level than at a high soil fertility level (Tables 2 and 3). Previously it has been reported that ruminants fed with cottonseed, like cows produce more milk and its protein content were also increased (Bertrand et al., 1998; Meyer et al., 2001; Taghizadeh et al., 2005). Therefore, an increase in the proteinogenic amino acid content achieved by adopting IMS resulted in enhanced nutritional value of the cotton embryos.
Nitrogen fertilization rate, plant density, and plant growth regulators have been shown to have a positive effect on cottonseed oil and protein contents (Sawan et al., 1993, 2006). In this study (using IMS), the N rate and fertility level contributed to the increase of protein content (Table 2), are in agreement with the results of the previous single-factor experiments (Chen et al., 2015), suggesting that protein content were increased with increasing N fertilization rate (Sawan et al., 1988; Egelkraut et al., 2004). As the plant density increased, the protein content were also increased (Rafiq et al., 2010), and it would not need an additional labor input because of mechanized transplanting. The N rate in IMS1 was an economic fertilization rate as reported previously (Chen et al., 2016) and it was higher compared with those reported at the other experimental stations (Rochester et al., 2001) might be attributed to the saline-alkali soil (pH 8.26–8.47). Salt stress limits plant growth (Zhang et al., 2015) and therefore the high economic fertilization N rate (375 kg ha-1). Further, increase in the application of N at flowering and boll-forming stage not only increased cotton yield and biomass (Yang et al., 2011), but also proved to be useful for increasing the protein content in cotton embryos (Table 2). Sufficient N supply from flowering to boll forming stage was the main reason for the increment of protein content. Additionally, this study also found that the substrate seedling-raising method contributed to increase in protein content. The probable reason behind this was that bio-organic fertilizer bring the efficacious living-cell into a soil and then affect the N availability in the soil (Inselsbacher et al., 2010). Due to unusual weather changes in recent years, temperature and photosynthetically active radiation in 2013 was higher than 2012, like cumulative photo-thermal product increased by 30.8% during the seed growth. Seed weight was increased dramatically; therefore, the protein content (g 100 embryos-1) was higher than the year in 2012. Therefore, a set of the best management practices for cotton production was integrated that could increase protein quality in cotton embryos for feed purposes.
A study using a method based on isotope (15 and 15
) labeling has demonstrated that soil N is ultimately translocated into seeds (Kullmann and Geisler, 1986), suggesting a potential contribution of soil N to the protein content of seeds. Embryos take up N, carbohydrates and free amino acids from seed coats (De Ruiter et al., 1986) and use them for embryo growth and the synthesis of storage proteins. Based on these studies, it appears that the soil N and seed protein contents are closely interrelated. In this study, as shown in Figure 2 and Table 2, the soil-available N and protein contents were higher in both IMS1 and IMS2 compared with that in CM. Similar results have been reported in tomato (Javaria and Khan, 2011) and potato (Zotarelli et al., 2015), suggesting that rate and timing of nitrogen fertilizer application increases the soil-available N content. Furthermore, principal component and Pearson’s correlation analyses revealed that the soil-available nitrogen content was positively correlated with the nutritional value of cotton embryos and that the soil-available nitrogen and proteinogenic amino acid profiles were closely matched in a score plot (Table 6; Figure 5). Therefore, high soil-available nitrogen content contributes to the nutritional value of cotton embryos.
A study using the isotope (15N) labeling also has shown that GS and NADH-GOGAT are correlated with N assimilation and re-distribution (Zhang et al., 2014). In this study, comparisons of management treatments at two fertility levels demonstrated that the protein content (g 100 embryos-1) was positively correlated with the GS and NADH-dependent GOGAT activities (Table 6). Both soil N and GS activity increased protein content in cotton embryos (Figures 2 and 3; Table 2), that is consistent with previous research findings, suggesting that -N and
-N concentrations in the soil tightly regulate the GS and GOGAT activities (Zhao and Shi, 2006), and that GS activity and actual accumulation of reduced N are closely interrelated in cotton (Radin et al., 1975) and wheat (Brunetti and Hageman, 1976). Therefore, a high level of soil available N increases N assimilation in developing cotton embryos (Figures 6A,B). Furthermore, GS plays a key role during the early stage of embryo growth.
A previous study reported that the synthesis of embryonic proteins is regulated by the supply of free amino acids (Takahashi et al., 2003; Hernandez-Sebastia et al., 2005). In this study, the free amino acid content decreased simultaneously with accumulating storage protein after 24 DAA (Figures 4A,B). The protein accumulation rate was higher in IMS1 and IMS2 embryos over CM, meanwhile the amino acids content showed the opposite trends, indicating that the protein content was limited by the protein accumulation rate rather than by the free amino acid content. Furthermore, the peak protein accumulation rate was positively associated with the protein content at maturity, exhibiting a period of rapid protein accumulation during 24–38 DAA (Figure 4C, Table 6), whereas the free amino acid content peaked at 24 DAA (Figure 4A). It seemed that embryos show an early period for free amino acids accumulation, which was characterized by large amount of free amino acid accumulation during 17–24DAA (Figure 4A). The embryos accumulate almost 75% of the total protein within rapid protein accumulation stage for a short period of time (lasted for 12–15 days) that was attributed to an early period of free amino acids accumulation. Similar study also observed in other researches, for instance in soybean (Rotundo et al., 2011), suggesting protein content was determined by different combinations of rate and duration of content accumulation (Figure 6C).
The indeterminate growth habit of cotton results in the formation of bolls over a range of time as the plant grows and under different environmental and nutritional conditions (Bondada and Oosterhuis, 2001). In this study, the soil available N decreased with plant growth, so, late season boll are susceptible to low soil N. This could explain why protein content in upper fruiting branches was higher than middle and lower fruiting branches (Chen et al., 2015). Therefore, adding extra N and allocating N to flowering and boll forming stage are beneficial to soil available N for the formation of late season bolls and it might increase the uniformity of cottonseed. Previous results also suggest adding extra potash fertilizer or allocating it for the development of late season bolls might result in longer fibers in upper fruiting branches (Yang et al., 2016). To sum up, whether adding extra nitrogen and potash fertilizer, and allocating these fertilizer to the flowering and boll formatting stage could result in both longer fiber and high seed protein quality still further results.
Conclusion
A set of best management practices for cotton embryonic protein, collectively known as IMS, was assembled. The combined application of the economic N rate, growth-driven N application schedule, a high plant density, and seedling raising with bio-organic matter markedly improved protein quality, especially the levels of Glu, Lys, and Met. Increased protein quality was attributed to a high level of soil-available N content. Soil -N and
-N transported into seed and then utilized through GS and GOGAT pathway that are beneficial for N assimilation in developing cotton embryos. Reduced N and incorporated into carbon skeletons of storage protein was determined by the rate and duration of protein accumulation. The key factor limiting the protein content was the protein accumulation rate rather than the free amino acid content. Therefore, selection of a high-yielding cultivar (SIZA-3) adapted to the cotton production region, transplantation of seedling with bio-organic fertilizer, a high plant density (30000 plant ha-1), and an economic N fertilization rate (375 kg ha-1, 20% applied as a basal fertilizer, 25% at the initial flowering stage, 40% at the full-bloom stage and 15% at the end of the flowering stage) are highly recommended for cotton management to promote protein quality of cotton embryos for ruminants feed (Figure 6).
Author Contributions
ZZ, YM, and BC conceived the idea and led the study design. HY carried out the experiment, performed analysis and wrote the paper. XZ assisted in plant sampling and laboratory analysis. WZ and YW assisted manuscript writing and editing. All authors reviewed the manuscript.
Funding
This study was financially supported from the China Agriculture Research System (CARS-18-20) and the Special Fund of National Public Welfare Industry (Agriculture) R & D Program (201203096, 201303002).
Conflict of Interest Statement
The authors declare that the research was conducted in the absence of any commercial or financial relationships that could be construed as a potential conflict of interest.
Acknowledgments
We really appreciate Dr. Muhammad Abid and Dr. Rizwan Zahoor for their comments on this paper. We also thank all members in Dafeng Experimental Station, Nanjing Agricultural University for their helps on cotton management.
Abbreviations
AV-N, soil available nitrogen; CM, conventional management practices; Glu, glutamate; GS, glutamine synthetase; IMS, integrated management strategies; Lys, lysine; Met, methionine; NADH-GOGAT, NADH-dependent glutamate synthase.
References
Alford, B. B., Liepa, G. U., and Vanbeber, A. D. (1996). Cottonseed protein: what does the future hold? Plant Foods Hum. Nutr. 49, 1–11. doi: 10.1007/bf01092517
Baker, D. A., and Young, D. L. (2004). Economically optimal nitrogen fertilization for yield and protein in hard red spring wheat. Agron. J. 96, 656–656. doi: 10.2134/agronj2004.1160
Bertrand, J. A., Pardue, F. E., and Jenkins, T. C. (1998). Effect of ruminally protected amino acids on milk yield and composition of Jersey cows fed whole cottonseed. J. Dairy Sci. 81, 2215–2220. doi: 10.3168/jds.S0022-0302(98)75800-X
Bondada, B. R., and Oosterhuis, D. M. (2001). Canopy photosynthesis, specific leaf weight, and yield components of cotton under varying nitrogen supply. J. Plant Nutr. 24, 469–477. doi: 10.1081/pln-100104973
Bradford, M. M. (1976). A rapid and sensitive method for the quantitation of microgram quantities of protein utilizing the principle of protein-dye binding. Anal. Biochem. 72, 248–254. doi: 10.1006/abio.1976.9999
Brunetti, N., and Hageman, R. H. (1976). Comparison of in Vivo and in Vitro assays of nitrate reductase in wheat (Triticum aestivum L.) Seedlings. Plant Physiol. 58, 583–587. doi: 10.1104/pp.58.4.583
Cai, C., Ye, W., Zhang, T., and Guo, W. (2014). Association analysis of fiber quality traits and exploration of elite alleles in Upland cotton cultivars/accessions (Gossypium hirsutum L.). J. Integr. Plant Biol. 56, 51–62. doi: 10.1111/jipb.12124
Canovas, F. M., Canton, F. R., Garcia-Gutierrez, A., Gallardo, F., and Crespillo, R. (1998). Molecular physiology of glutamine and glutamate biosynthesis in developing seedlings of conifers. Physiol. Plant. 103, 287–294. doi: 10.1034/j.1399-3054.1998.1030217.x
Chen, B., Yang, H., Song, W., Liu, C., Xu, J., Zhao, W., et al. (2016). Effect of N fertilization rate on soil alkali-hydrolyzable N, subtending leaf N concentration, fiber yield, and quality of cotton. Crop J. (in press). doi: 10.1016/j.cj.2016.03.006
Chen, M. L., Zhao, W. Q., Meng, Y. L., Chen, B. L., Wang, Y. H., Zhou, Z. G., et al. (2015). A model for simulating the cotton (Gossypium hirsutum L.) embryo oil and protein accumulation under varying environmental conditions. Field Crops Res. 183, 79–91. doi: 10.1016/j.fcr.2015.07.011
Crawford, N. M., and Glass, A. D. M. (1998). Molecular and physiological aspects of nitrate uptake in plants. Trends Plant Sci. 3, 389–395. doi: 10.1016/S1360-1385(98)01311-9
Dahnke, W. C., and Johnson, G. V. (1990). “Testing soils for available nitrogen,” in Soil Testing and Plant Analysis, 3rd Edn. ed. R. L. Westerman (Madison, WI: SSSA), 127–139. doi: 10.2136/sssabookser3.3ed.c6
De Ruiter, H., Schuurmans, J., and Kollöffel, C. (1986). “Amino acid efflux from seed coats and from cotyledons of developing and germinating pea seeds,” in Fundamental, Ecological and Agricultural Aspects of Nitrogen Metabolism in Higher Plants, eds H. Lambers, J. J. Neeteson, and I. Stulen (Rotterdam: Springer), 123–125. doi: 10.1007/978-94-009-4356-8_17
Dong, H. Z., Li, W. J., Eneji, A. E., and Zhang, D. M. (2012). Nitrogen rate and plant density effects on yield and late-season leaf senescence of cotton raised on a saline field. Field Crops Res. 126, 137–144. doi: 10.1016/j.fcr.2011.10.005
Egelkraut, T. M., Kissel, D. E., Cabrera, M. L., Gascho, G. J., and Adkins, W. (2004). Nitrogen concentration in cottonseed as an indicator of N availability. Nutr Cycl. Agroecosys. 68, 235–242. doi: 10.1023/b:fres.0000019461.39144.29
Falkenberg, N. R., Piccinni, G., Cothren, J. T., Leskovar, D. I., and Rush, C. M. (2007). Remote sensing of biotic and abiotic stress for irrigation management of cotton. Agric. Water Manag. 87, 23–31. doi: 10.1016/j.agwat.2006.05.021
Gastal, F., and Lemaire, G. (2002). N uptake and distribution in crops: an agronomical and ecophysiological perspective. J. Exp. Bot. 53, 789–799. doi: 10.1093/jexbot/53.370.789
Guan, X., Song, Q., and Chen, Z. J. (2014). Polyploidy and small RNA regulation of cotton fiber development. Trends Plant Sci. 19, 516–528. doi: 10.1016/j.tplants.2014.04.007
Hao, X., Gao, J., Han, X., Ma, Z., Merchant, A., Ju, H., et al. (2014). Effects of open-air elevated atmospheric CO2 concentration on yield quality of soybean (Glycine max (L.) Merr). Agri. Ecosyst. Environ. 192, 80–84. doi: 10.1016/j.agee.2014.04.002
Hendrix, D. L. (1993). Rapid extraction and analysis of nonstructural carbohydrates in plant tissues. Crop Sci. 33, 1306–1311. doi: 10.2135/cropsci1993.0011183X003300060037x
Hernandez-Sebastia, C., Marsolais, F., Saravitz, C., Israel, D., Dewey, R. E., and Huber, S. C. (2005). Free amino acid profiles suggest a possible role for asparagine in the control of storage-product accumulation in developing seeds of low- and high-protein soybean lines. J. Exp. Bot. 56, 1951–1963. doi: 10.1093/jxb/eri191
Hodges, M. (2002). Enzyme redundancy and the importance of 2-oxoglutarate in plant ammonium assimilation. J. Exp. Bot. 53, 905–916. doi: 10.1093/jexbot/53.370.905
Hodges, M., Flesch, V., Galvez, S., and Bismuth, E. (2003). Higher plant NADP(+)-dependent isocitrate dehydrogenases, ammonium assimilation and NADPH production. Plant Physiol. Biochem. 41, 577–585. doi: 10.1016/s0981-9428(03)00062-7
Ikurior, S. A., and Fetuga, B. L. A. (1987). Composition of some recommended Nigerian commercial cottonseed varieties. Food Chem. 26, 307–314. doi: 10.1016/0308-8146(87)90072-0
Inselsbacher, E., Umana, H. N., Stange, F. C., Gorfer, M., Schüller, E., Ripka, K., et al. (2010). Short-term competition between crop plants and soil microbes for inorganic N fertilizer. Soil Biol. Biochem. 42, 360–372. doi: 10.1016/j.soilbio.2009.11.019
Ishiyama, K., Hayakawa, T., and Yamaya, T. (1998). Expression of NADH-dependent glutamate synthase protein in the epidermis and exodermis of rice roots in response to the supply of ammonium ions. Planta 204, 288–294. doi: 10.1007/s004250050258
Javaria, S., and Khan, M. Q. (2011). Impact of integrated nutrient management on tomato yield quality and soil environment. J. Plant Nutr. 34, 140–149. doi: 10.1080/01904167.2011.531605
Katayama, K., Ito, O., Adu-Gyamfi, J. J., Rao, T. P., Dacanay, E. V., and Yoneyama, T. (1999). Effects of NPK fertilizer combinations on yield and nitrogen balance in sorghum or pigeonpea on a vertisol in the semi. Soil Sci. Plant Nutr. 45, 143–150. doi: 10.1080/00380768.1999.10409330
Kawakami, E. M., Snider, J. L., Oosterhuis, D. M., and Mozaffari, M. (2012). Physiological and yield responses of field-grown cotton to application of urea with the urease inhibitor NBPT and the nitrification inhibitor DCD. Eur. J. Agron. 43, 147–154. doi: 10.1016/j.eja.2012.06.005
Konishi, N., Ishiyama, K., Matsuoka, K., Maru, I., Hayakawa, T., Yamaya, T., et al. (2014). NADH-dependent glutamate synthase plays a crucial role in assimilating ammonium in the Arabidopsis root. Physiol. Plant. 152, 138–151. doi: 10.1111/ppl.12177
Kuai, J., Chen, Y., Wang, Y., Meng, Y., Chen, B., Zhao, W., et al. (2016). Effect of water logging on carbohydrate metabolism and the quality of fiber in cotton (Gossypium hirsutum L.). Front. Plant Sci. 7:877. doi: 10.3389/fpls.2016.00877
Kullmann, A., and Geisler, G. (1986). “Translocation of labelled nitrogen in oilseed rape,” in Fundamental, Ecological and Agricultural Aspects of Nitrogen Metabolism in Higher Plants, eds H. Lambers, J. J. Neeteson, and I. Stulen (Rotterdam: Springer), 127–129. doi: 10.1007/978-94-009-4356-8_18
Lea, P. J., Kwell, R. D., Chen, F. L., and Hecht, U. (1990). Enzymes of ammonia assimilation. Methods Plant Biochem. 3, 257–276. doi: 10.1016/B978-0-12-461013-2.50022-8
Li, W., Meng, Y. L., Chen, B. L., and Wang, Y. H. (2009). Effects of climatic factors on fat and total protein contents in cottonseeds. Acta Ecol. Sin. 29, 1832–1839. doi: 10.3321/j.issn:1000-0933.2009.04.025
Liu, J. R., Meng, Y. L., Chen, B. L., Zhou, Z. G., Ma, Y. N., Lv, F. J., et al. (2015). Photosynthetic characteristics of the subtending leaf and the relationships with lint yield and fiber quality in the late-planted cotton. Acta Physiol. Plant. 37, 79. doi: 10.1007/s11738-015-1824-9
Macduff, J. H., and Hopper, M. J. (1986). Effects of root temperature on uptake of nitrate and ammonium ions by barley grown in flowing-solution culture. Plant Soil 91, 303–306. doi: 10.1007/bf02198112
Martre, P., Porter, J. R., Jamieson, P. D., and Triboï, E. (2003). Modeling grain nitrogen accumulation and protein composition to understand the sink/source regulations of nitrogen remobilization for wheat. Plant Physiol. 133, 1959–1967. doi: 10.1104/pp.103.030585
Mcgraw, J. B., and Shaver, G. R. (2006). Seedling density and seedling survival in Alaskan cotton grass tussock tundra. Ecography 5, 212–217. doi: 10.1111/j.1600-0587.1982.tb01039.x
Meyer, M., Shirley, J., Titgemeyer, E., Park, A., and VanBaale, M. (2001). Effect of mechanical processing and fat removal on the nutritive value of cottonseed for lactating dairy cows. J. Dairy Sci. 84, 2503–2514. doi: 10.3168/jds.s0022-0302(01)74701-7
Muhitch, M. J. (2006). Tissue distribution and developmental patterns of NADH- and ferredoxin-dependent glutamate synthase activities in maize (Zea mays) kernels. Physiol. Plant. 81, 481–488. doi: 10.1034/j.1399-3054.1991.810406.x
Mujahid, A., Abdullah, M., Barque, A. R., and Gilani, A. H. (2000). Nutritional value of cottonseeds and it’s derived products. II. Free gossypol, available lysine and in vitro protein digestibility. Asian-Australas. J. Anim. Sci. 13, 356–359. doi: 10.5713/ajas.2000.356
Muro-Pastor, M. I., and Florencio, F. J. (2003). Regulation of ammonium assimilation in cyanobacteria. Plant Physiol. Biochem. 41, 595–603. doi: 10.1016/s0981-9428(03)00066-4
Muro-Pastor, M. I., Reyes, J. C., and Florencio, F. J. (2005). Ammonium assimilation in cyanobacteria. Photosynthesis Res. 83, 135–150. doi: 10.1007/s11120-004-2082-7
Ning, Z., Chen, H., Mei, H., and Zhang, T. (2014). Molecular tagging of QTLs for fiber quality and yield in the upland cotton cultivar Acala-Prema. Euphytica 195, 143–156. doi: 10.1007/s10681-013-0990-3
Potel, F., Valadier, M. H., Ferrario-Méry, S., Grandjean, O., Morin, H., Gaufichon, L., et al. (2009). Assimilation of excess ammonium into amino acids and nitrogen translocation in Arabidopsis thaliana – roles of glutamate synthases and carbamoylphosphate synthetase in leaves. FEBS J. 276, 4061–4076. doi: 10.1111/j.1742-4658.2009.07114.x
Prasad, P. V. V., Satyanarayana, V., Murthy, V. R. K., and Boote, K. J. (2002). Maximizing yields in rice-groundnut cropping sequence through integrated nutrient management. Field Crops Res. 75, 9–21. doi: 10.1016/S0378-4290(01)00214-3
Radin, J. W., Sell, C. R., and Jordan, W. R. (1975). Physiological significance of the in vivo assay for nitrate reductase in cotton seedlings. Crop Sci. 15, 710–713. doi: 10.2135/cropsci1975.0011183x001500050029x
Rafiq, M. A., Ali, A., Malik, M. A., and Hussain, M. (2010). Effect of fertilizer levels and plant densities on yield and protein contents of autumn planted maize. Pak. J. Agric. Sci. 47, 201–208.
Rochester, I. J., Peoples, M. B., and Constable, G. A. (2001). Estimation of the N fertiliser requirement of cotton grown after legume crops. Field Crops Res. 70, 43–53. doi: 10.1016/s0378-4290(00)00150-7
Rotundo, J. L., Borrás, L., and Westgate, M. E. (2011). Linking assimilate supply and seed developmental processes that determine soybean seed composition. Eur. J Agron. 35, 184–191. doi: 10.1016/j.eja.2011.05.002
Rotundo, J. L., and Westgate, M. E. (2009). Meta-analysis of environmental effects on soybean seed composition. Field Crops Res. 110, 147–156. doi: 10.1016/j.fcr.2008.07.012
Sainju, U. M., Whitehead, W. F., Singh, B. P., and Wang, S. (2006). Tillage, cover crops, and nitrogen fertilization effects on soil nitrogen and cotton and sorghum yields. Eur. J. Agron. 25, 372–382. doi: 10.1016/j.eja.2006.07.005
Sapkota, T. B., Majumdar, K., Jat, M. L., Kumar, A., Bishnoi, D. K., Mcdonald, A. J., et al. (2014). Precision nutrient management in conservation agriculture based wheat production of Northwest India: profitability, nutrient use efficiency and environmental footprint. Field Crops Res. 155, 233–244. doi: 10.1016/j.fcr.2013.09.001
Sawan, Z. M., Basyony, A. E., McCuistion, W. L., and El Farra, A. H. A. (1993). Effect of plant population densities and application of growth retardants on cottonseed yield and quality. J. Am. Oil Chem. Soc. 70, 313–317. doi: 10.1007/BF02545314
Sawan, Z. M., Elfarra, A. A., and Ellatif, S. A. (1988). Cottonseed, protein and oil yields, and oil properties as affected by nitrogen and phosphorus fertilization and growth regulators. J. Agron. Crop Sci. 161, 50–56. doi: 10.1111/j.1439-037X.1988.tb00643.x
Sawan, Z. M., Fahmy, A. H., and Yousef, S. E. (2009). Direct and residual effects of nitrogen fertilization, foliar application of potassium and plant growth retardant on Egyptian cotton growth, seed yield, seed viability and seedling vigor. Acta Ecol. Sin. 29, 116–123. doi: 10.1016/j.chnaes.2009.05.008
Sawan, Z. M., Hafez, S. A., and Basyony, A. E. (2001). Effect of nitrogen fertilization and foliar application of plant growth retardants and zinc on cottonseed, protein and oil yields and oil properties of cotton. J. Agron. Crop Sci. 186, 183–191. doi: 10.1046/j.1439-037X.2001.00473.x
Sawan, Z. M., Hafez, S. A., Basyony, A. E., and Alkassas, A. (2006). Cottonseed, protein, oil yields and oil properties as affected by nitrogen fertilization and foliar application of potassium and a plant growth retardant. World J. Agric. Sci. 2, 56–65. doi: 10.3989/gya.2007.v58.i1.7
Seong, R. C., Ji, H. P., Park, S. J., and Cho, J. Y. (2004). Effects of source-sine alteration on dry matter accumulation and protein content in soybean. J. Bacteriol. 186, 3621–3630.
Singh, K., Chand, S., and Yaseen, M. (2014). Integrated nutrient management in Indian basil (Ocimum basilicum). Ind. Crop Prod. 55, 225–229. doi: 10.1016/j.indcrop.2014.02.009
Stewart, G., and Rhodes, D. (1978). Nitrogen metabolism of halophytes III. Enzymes of ammonia assimilation. New Phytol. 80, 307–316. doi: 10.1111/j.1469-8137.1978.tb01563.x
Taghizadeh, A., Mesgaran, M. D., Valizadeh, R., Shahroodi, F. E., and Stanford, K. (2005). Digestion of feed amino acids in the rumen and intestine of steers measured using a mobile nylon bag technique. J. Dairy Sci. 88, 1807–1814. doi: 10.3168/jds.s0022-0302(05)72855-1
Takahashi, M., Uematsu, Y., Kashiwaba, K., Yagasaki, K., Hajika, M., Matsunaga, R., et al. (2003). Accumulation of high levels of free amino acids in soybean seeds through integration of mutations conferring seed protein deficiency. Planta 217, 577–586. doi: 10.1007/s00425-003-1026-3
Tamura, W., Hidaka, Y., Tabuchi, M., Kojima, S., Hayakawa, T., Sato, T., et al. (2010). Reverse genetics approach to characterize a function of NADH-glutamate synthase1 in rice plants. Amino Acids 39, 1003–1012. doi: 10.1007/s00726-010-0531-5
Yang, G., Tang, H., Tong, J., Nie, Y., and Zhang, X. (2011). Effect of fertilization frequency on cotton yield and biomass accumulation. Fuel Energy Abstr. 125, 161–166. doi: 10.1016/j.fcr.2011.08.008
Yang, J., Hu, W., Zhao, W., Chen, B., Wang, Y., Zhou, Z., et al. (2016). Fruiting branch K+ level affects cotton fiber elongation through Osmoregulation. Front. Plant Sci. 7:13. doi: 10.3389/fpls.2016.00013
Yu, J. W., Yu, S. X., Fan, S. L., Song, M. Z., Zhai, H. H., Li, X. L., et al. (2012). Mapping quantitative trait loci for cottonseed oil, protein and gossypol content in a Gossypium hirsutum x Gossypium barbadense backcross inbred line population. Euphytica 187, 191–201. doi: 10.1007/s10681-012-0630-3
Zhang, S., Wang, T., Liu, Q., Gao, X., Zhu, X., Zhang, T., et al. (2015). Quantitative trait locus analysis of boll-related traits in an intraspecific population of Gossypium hirsutum. Euphytica 203, 121–144. doi: 10.1007/s10681-014-1281-3
Zhang, Y., Zhang, Y., Liu, N., Su, D., Xue, Q., Stewart, B. A., et al. (2012). Effect of source-sink manipulation on accumulation of micronutrients and protein in wheat grains. J. Plant Nutr. Soil Sci. 60, 1026–1036. doi: 10.1002/jpln.201100224
Zhang, Z. H., Wang, W. M., Guan, C. Y., Liu, Q., Rong, X. M., Han, Y. L., et al. (2014). Mechanisms of nitrogen re-disrtibution in response to enzyme activities and the effects on nitrogen use efficiency in brassica napus during later growing stage. Pak. J. Bot. 46, 1789–1795.
Zhao, X. Q., and Shi, W. M. (2006). Expression analysis of the glutamine synthetase and glutamate synthase gene families in young rice (Oryza sativa) seedlings. Plant Sci. 170, 748–754. doi: 10.1016/j.plantsci.2005.11.006
Zhe, S., Ying, L., Hong-bao, P., and Xue-jun, G. (2014). Application of protein feed processed by microbial fermentation to dairy cow. J. Northeast Agric. Univ. 21, 39–44. doi: 10.1016/S1006-8104(14)60020-9
Keywords: cotton embryonic protein, integrated management strategies, soil available N, N assimilation, proteinogenic amino acids
Citation: Yang H, Meng Y, Chen B, Zhang X, Wang Y, Zhao W and Zhou Z (2016) How Integrated Management Strategies Promote Protein Quality of Cotton Embryos: High Levels of Soil Available N, N Assimilation and Protein Accumulation Rate. Front. Plant Sci. 7:1118. doi: 10.3389/fpls.2016.01118
Received: 25 April 2016; Accepted: 13 July 2016;
Published: 02 August 2016.
Edited by:
Antonio M. De Ron, Misión Biológica de Galicia – CSIC, SpainReviewed by:
James Frelichowski, United States Department of Agriculture, USAMercedes Fernández-Pascual, Instituto de Ciencias Agrarias – CSIC, Spain
Copyright © 2016 Yang, Meng, Chen, Zhang, Wang, Zhao and Zhou. This is an open-access article distributed under the terms of the Creative Commons Attribution License (CC BY). The use, distribution or reproduction in other forums is permitted, provided the original author(s) or licensor are credited and that the original publication in this journal is cited, in accordance with accepted academic practice. No use, distribution or reproduction is permitted which does not comply with these terms.
*Correspondence: BingLin Chen, YmxjaGVuQG5qYXUuZWR1LmNu