- 1Department of Biology, Memorial University of Newfoundland, St. John’s, NL, Canada
- 2Department of Biochemistry and Cell Physiology, Voronezh State University, Voronezh, Russia
Organic acids are synthesized in plants as a result of the incomplete oxidation of photosynthetic products and represent the stored pools of fixed carbon accumulated due to different transient times of conversion of carbon compounds in metabolic pathways. When redox level in the cell increases, e.g., in conditions of active photosynthesis, the tricarboxylic acid (TCA) cycle in mitochondria is transformed to a partial cycle supplying citrate for the synthesis of 2-oxoglutarate and glutamate (citrate valve), while malate is accumulated and participates in the redox balance in different cell compartments (via malate valve). This results in malate and citrate frequently being the most accumulated acids in plants. However, the intensity of reactions linked to the conversion of these compounds can cause preferential accumulation of other organic acids, e.g., fumarate or isocitrate, in higher concentrations than malate and citrate. The secondary reactions, associated with the central metabolic pathways, in particularly with the TCA cycle, result in accumulation of other organic acids that are derived from the intermediates of the cycle. They form the additional pools of fixed carbon and stabilize the TCA cycle. Trans-aconitate is formed from citrate or cis-aconitate, accumulation of hydroxycitrate can be linked to metabolism of 2-oxoglutarate, while 4-hydroxy-2-oxoglutarate can be formed from pyruvate and glyoxylate. Glyoxylate, a product of either glycolate oxidase or isocitrate lyase, can be converted to oxalate. Malonate is accumulated at high concentrations in legume plants. Organic acids play a role in plants in providing redox equilibrium, supporting ionic gradients on membranes, and acidification of the extracellular medium.
Introduction
Organic acids are the product of incomplete oxidation of photosynthetic assimilates. They can either be converted back to carbohydrates or undergo terminal oxidation yielding CO2 and H2O. Their carbon skeletons can also be used for biosynthesis of amino acids. The “intermediate” nature of organic acids determines the flexibility of their role as important players in the maintenance of redox balance, production and consumption of ATP, support of protonic and ionic gradients on membranes, and acidification of extracellular spaces.
Organic acids are formed within metabolic cycles and pathways and represent the transitory or stored forms of fixed carbon. Considering a metabolic system at steady state, the reaction rate of every step can be determined as the concentration of molecules in this step, divided by the mean time that one molecule needs to move the next step. This mean time can be designated as transient time for this step, and according to Easterby (1996) the reaction rate can be written as
where vi is the reaction rate in the i-th step, Si is the concentration of the metabolites and ti is the transient time for this step. The concentrations of the metabolites at steady state, when all reactions proceed with the same rate (vi = J), can be written from the previous equation for every step as:
where J is the steady-state metabolic flux in the cycle (Fridlyand and Scheibe, 1999). Thus, organic acids accumulate if the transient time for their conversion is long. The increase of ti for an enzyme leads to the accumulation of metabolite at this step. This can be due to low activity of the enzyme or its low concentration. In the Calvin–Benson cycle, the concentrations of intermediates are established according to the reaction rates of corresponding enzymes and the transient times of metabolite conversions (Fridlyand and Scheibe, 1999). The stability of a cycle’s structure is connected with the existence of transient pools of intermediates that are accumulated during the operation of the cycle. Accumulation of organic acids in steady state conditions is always determined by the transient times of their conversion.
The structure of a metabolic cycle can also involve the secondary (auxiliary) pools of intermediate derivatives that are formed from the intermediates via their transformations that in many cases are reversible (Figure 1). These pools can stabilize operation of the cycle, ensure its maintenance when the main substrate is not efficiently supplied, and provide operation of the cycle as “incomplete” in order to modify its function in response to environmental changes and disturbances. The transient times of secondary intermediates are usually long, their conversion is slow (e.g., for oxalate or malonate), which determines high rates of their accumulation in particular conditions in certain species. The mentioned intermediate derivatives can acquire special functions in metabolism and increase its flexibility.
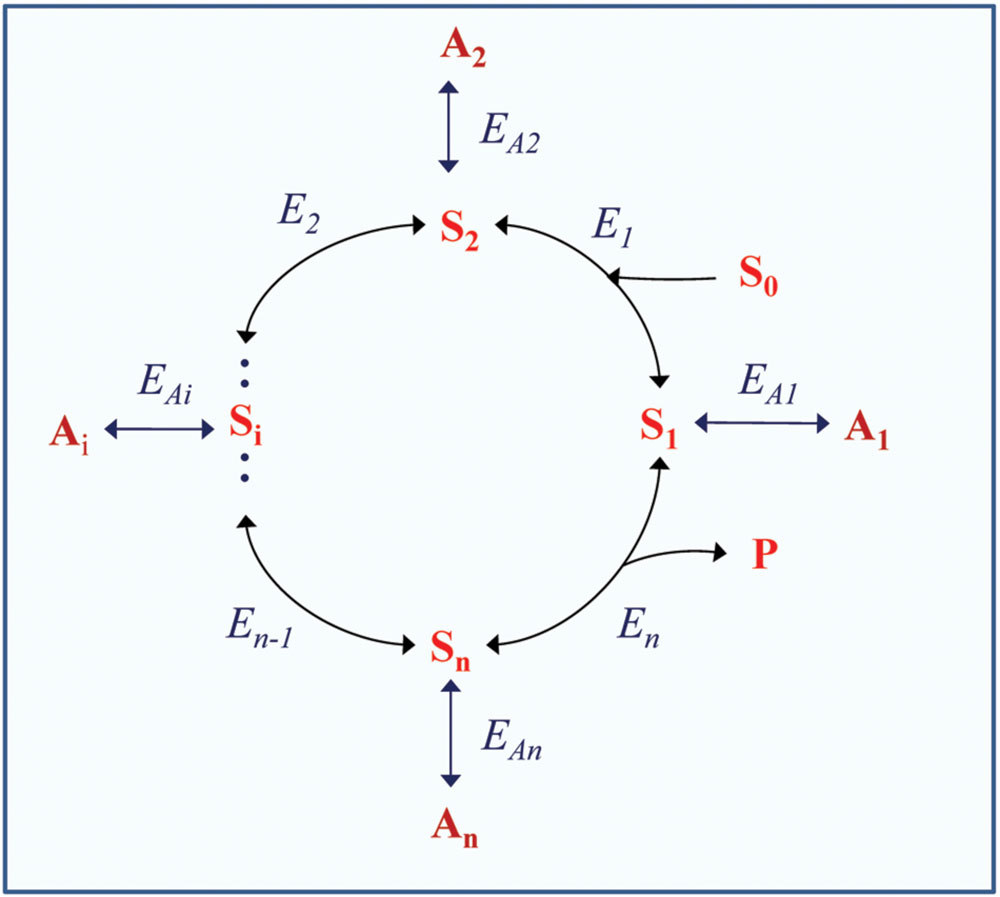
FIGURE 1. Scheme of a cyclic enzymatic pathway with secondary pools of intermediate derivatives. S0 is the initial substrate; S1, Si, and Sn are cycle intermediates; P is the product; E1, Ei, and En are the enzymes catalyzing corresponding steps within the cycle; A1, A2, Ai, An are intermediate derivatives; EA1, EA2, EAi, EAn are the enzymes catalyzing corresponding side reactions forming the secondary pools. The initial scheme of the cycle (without side reactions) is adapted with modifications from Fridlyand and Scheibe (1999).
Organic acids represent the category of compounds that contain carboxylic groups negatively charged at neutral pH and to a lesser extent at acidic pH. Their functions therefore can change depending on pH of the solution, and their excretion can result in the release of protons and, therefore, in acidification of soil, apoplast and vacuole. In this review, we discuss the accumulation and functional role of organic acids related mainly to the operation of the tricarboxylic acid (TCA) cycle and photorespiration. We do not analyze in detail the relation of organic acid and amino acid metabolism and their formation in glycolysis, methylglyoxal pathway and lysine catabolism.
Incomplete Tricarboxylic Acid Cycle and the Operation of Malate and Citrate Valves
The TCA cycle can regulate the redox and energy level in the cell and supply substrates for amino acid synthesis through the operation of malate and citrate valves, the intensities of which depend on the transformation of the TCA cycle into an open structure. Also the secondary derivatives of the TCA cycle intermediates form corresponding pools that can feed the cycle and stabilize its operation. Differences in the organization of metabolic pathways in different plants will lead to accumulation of different organic acids.
The TCA cycle can operate either in the complete (closed) or the incomplete (open) mode. The concept of the incomplete TCA cycle was initially suggested by Chen and Gadal (1990), Hanning and Heldt (1993) and Gardeström et al. (2002), proven by studying kinetics of NAD- and NADP-dependent isocitrate dehydrogenases by Igamberdiev and Gardeström (2003), confirmed by using stable isotopes of carbon by Tcherkez et al. (2009), and studied via flux modeling (Sweetlove et al., 2010). Transformation to the incomplete cycle is achieved due to the increase of redox level taking place, in particular, in the light, especially in photorespiratory conditions (Igamberdiev et al., 2001a; Igamberdiev and Gardeström, 2003; Gardeström and Igamberdiev, 2016). In the “non-cyclic” partial TCA cycle, one branch produces citrate which can be transformed to isocitrate, 2-oxoglutarate or their derivatives (including glutamate), while the other branch produces malate (or fumarate and even succinate) that can be exported from mitochondria and accumulated in vacuoles (Figure 2). The vacuole plays an important role as an intermediary store of malate, citrate and other organic acids; therefore, vacuolar transport functions as a very well-regulated process. This results in the cytosolic pool of malate (and likely of other organic acids) remaining quite stable, with the vacuole serving as a buffer (Meyer et al., 2010a, 2011).
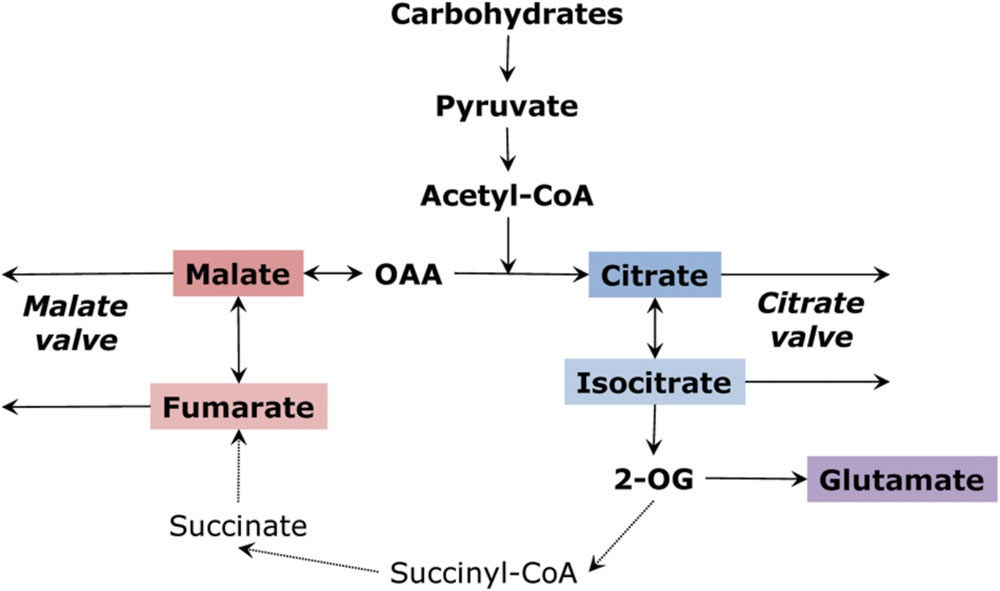
FIGURE 2. Operation of the tricarboxylic acid cycle in a “two branches” mode. Malate and citrate valves are the result of outflow of organic acids from the two branches.
Malate, in many plants, is the most accumulated acid and can participate in the transfer of redox equivalents between cell compartments (Geigenberger and Fernie, 2011; Maurino and Engqvist, 2015). Malate fulfills many functions in plant cells, one of which includes its role as an osmolyte and an anion, compensating the positive charge of potassium, particularly important in stomatal responses (Meyer et al., 2010b). The “malate valve” in photosynthetic cells is driven by NADPH formed by photosynthetic electron transport (Krömer and Scheibe, 1996). Malate accumulation is facilitated due to the equilibrium of malate dehydrogenase [OAA][NADH]/[Malate][NAD+] ∼ 2.8 × 10-5 (Guynn et al., 1973), established in the conditions of the physiological values of NADH/NAD+ ratio, which are equal to 10-3 in the cytosol and 10-2–10-1 in mitochondria in photosynthetic cells (Heineke et al., 1991; Igamberdiev et al., 2001a). In the light, the inhibition of succinate dehydrogenase and fumarase increases malate levels (Eprintsev et al., 2013, 2016; Daloso et al., 2015). The ratio of malate to oxaloacetate (OAA) is an indicator of the reduction level of NAD. The chloroplast NADP-malate dehydrogenase activity is generally lower than NAD-malate dehydrogenase (located in mitochondria, cytosol, peroxisomes, cell wall, some activity in chloroplasts) and does not contribute significantly to the accumulation of malate, except in special cases (photosynthetic induction etc., see Igamberdiev et al., 1998). The conversion of malate to pyruvate via NAD-malic enzyme in mitochondria (Tronconi et al., 2015) and NADP-malic enzyme in the cytosol (Gerrard Wheeler et al., 2009) is under the control of fumarate. When fumarate accumulates, the conversion of malate to pyruvate is facilitated. This takes place during light-enhanced dark respiration (LEDR) (Igamberdiev et al., 2001b).
Malate dehydrogenase, due to its high concentration in mitochondria and other cell compartments and high turnover number, has an important function of regulating the NADH/NAD+ ratio and keeping NADH at a low level. This prevents the inhibition of several enzymes resulting from the buildup of NADH and facilitates metabolic respiratory flux. The role of malate dehydrogenase as a “buffering” enzyme relieving NADH inhibition was demonstrated for the operation of glycine decarboxylase (Bykova et al., 2014) and pyruvate dehydrogenase (Igamberdiev et al., 2014a) complexes. This operation of malate dehydrogenase under conditions of saturation by malate represents an important function of the malate valve in the regulation of redox levels in different cell compartments.
Other organic acids associated with malate conversions are fumarate, succinate, and the derivatives of OAA (e.g., malonate). Although succinate dehydrogenase and fumarase genes are downregulated in the light via phytochrome A (Eprintsev et al., 2013, 2016; Igamberdiev et al., 2014b) and the enzyme proteins are inhibited by high redox levels (Daloso et al., 2015), even the decreased activity of fumarase in the light can result in the accumulation of fumarate at very high concentrations (more than 10 mM), e.g., in Arabidopsis (Chia et al., 2000). Operation of succinate dehydrogenase in the reverse direction is thermodynamically possible at high redox levels in conjunction with the operation of Complex I supplying electrons from NADH via ubiquinone (Grivennikova et al., 1993), however, this fumarate reductase activity has not been yet demonstrated in plants. Succinate accumulation under hypoxia (Menegus et al., 1989) is also due to higher reduction states in mitochondria and a possible fumarate reductase reaction; however, it may be connected with the inhibition of succinate dehydrogenase and operation of a γ-aminobutyric shunt (António et al., 2016), activated due to a decrease in the cytosolic pH (Crawford et al., 1994).
The “citrate valve” is driven by the increased reduction level in mitochondria (Igamberdiev and Gardeström, 2003), connected, in the light, with photorespiratory metabolism in C3 plants. This valve supplies the anabolic reduction power in the form of NADPH, formed by the activity of cytosolic isocitrate dehydrogenase, and the conversion of 2-oxoglutarate to glutamate, which, in some cases, becomes the main product of the partial TCA cycle (Tcherkez et al., 2009). It is likely that citrate is exported from mitochondria preferentially as compared to isocitrate and 2-oxoglutarate. The aconitase equilibrium is strongly displaced toward citrate, and the mitochondrial aconitase, as compared to the cytosolic form, has a lower affinity to citrate and a higher affinity to isocitrate that favors citrate accumulation (Eprintsev et al., 2015a). Citrate export is more than ten times as fast as the export of 2-oxoglutarate in isolated pea leaf mitochondria and twice as fast in spinach leaf mitochondria (Hanning and Heldt, 1993). The citrate carrier from pea is inactive with isocitrate, although the carrier from maize exhibited isocitrate transport capacity (McIntosh and Oliver, 1992). 13C nuclear magnetic resonance studies confirmed that in intact leaves in the light citrate is a major mitochondrial product (Gout et al., 1993). Isocitrate and 2-oxoglutarate are then formed from the exported citrate in the cytosol via the cytosolic aconitase and NADP-isocitrate dehydrogenase which activities are high and usually exceed the activities of the mitochondrial isoforms of these enzymes (Eprintsev et al., 2015a).
Cellular concentrations of citrate have a major impact on nuclear-encoded transcript abundance in Arabidopsis (Finkemeier et al., 2013). Isocitrate or other citrate analogs does not exhibit similar effects. The effects include regulation of the TCA cycle, nitrogen and sulfur metabolism, DNA synthesis and were found to be similar to observed after biotic stress treatments and the gibberellin biosynthesis inhibitor paclobutrazol. It is suggested that the changes in carboxylic acid abundances can be perceived in Arabidopsis by as yet unknown signaling pathways. Previously reported activation of transcription of the alternative cyanide-resistant oxidase in plants by citrate (Vanlerberghe and McIntosh, 1996) can be a part of the more general function of citrate at the transcriptional level. This regulation can be achieved via inhibition of aconitase by reactive oxygen and nitrogen species in stress conditions (Hausladen and Fridovich, 1994; Gupta et al., 2012), or by suppression of isocitrate oxidation at the increased redox level (Igamberdiev and Gardeström, 2003).
Operation of the citrate and malate valves takes place between mitochondria, cytosol and other organelles. This corresponds to the localization of several enzymes participating in the TCA cycle also in the cytosol and in other organelles. The universality of malate as a redox transport compound determines localization of different isoforms of NAD-malate dehydrogenase in mitochondria, cytosol, peroxisomes, plastids and apoplast (Gietl, 1992) as well as the presence of light-activated NADP-malate dehydrogenase in chloroplasts, NAD-malic enzyme in mitochondria and NADP-malic enzyme in plastids and cytosol (Drincovich et al., 2001). Aconitase is present in mitochondria, cytosol and likely absent from peroxisomes (Eprintsev et al., 2015a). While NAD-dependent isocitrate dehydrogenase is located in mitochondria, NADP-dependent isocitrate dehydrogenase is found in mitochondria, cytosol, peroxisomes and plastids (Randall, 1981; Corpas et al., 1999). Fumarase in several plants has not only mitochondrial but also cytosolic localization, and its cytosolic form can be linked to utilization of the glyoxylate cycle products in maize (Eprintsev et al., 2014), in allocation of photosynthates and growth on high nitrogen in Arabidopsis (Pracharoenwattana et al., 2010). Although the main route of succinate metabolism is the mitochondrial complex II, its oxidation can be linked to the reduction of nitrate on plasma membrane (Wienkoop et al., 1999) and to the malonate-insensitive activity of succinate oxidase in glyoxysomes of cereals (Igamberdiev et al., 1995). The coordinated operation of isoenzymes of the TCA cycle enzymes in mitochondria, cytosol and other organelles balances the intercompartmental redox regulation, supplies intermediates for biosynthetic pathways and provides the flexibility of the TCA cycle operation in open versus closed form. An important role in the coordination of regulation of two branches of the TCA cycle may belong to the non-glyoxysomal isocitrate lyase, which operates in the cytosol at low pH and activated by manganese (Eprintsev et al., 2015b). It may link the TCA cycle with glyoxylate/glycine metabolism, and, therefore, either supply glyoxylate for glycine and oxalate biosynthesis or utilize the photorespiratory glyoxylate in the reverse (synthase) reaction (Igamberdiev and Lea, 2002).
Di- and Tricarboxylic Acids in C4 and Cam Photosynthesis
C4 and CAM photosynthetic metabolism represent a special role for organic acids as intermediate pools of fixed carbon. While the product of CO2 fixation is PGA in the Benson–Calvin cycle, the fixation of CO2 by phosphoenolpyruvate carboxylase results in the formation of OAA which is then reduced to malate in C4 and CAM. Malate, thus, becomes the central metabolite in C4 and CAM photosynthesis, playing a double role as a product in the fixation of CO2 and a redox equivalent carrier. Its decarboxylation, via NAD- or NADP-malic enzyme, delivers carbon to the Benson-Calvin cycle. In some plants, OAA is preferentially transaminated to aspartate, and CO2 delivery occurs via PEP carboxykinase (Burnell and Hatch, 1988).
Although OAA and malate are the primary products of C4 and CAM photosynthesis, the plants of these photosynthetic types can intensively accumulate organic acids of the citrate branch. Citrate (or isocitrate) can be the most abundant stored form of carbon in several plants. These plants maintain their metabolic balance by transforming a part of stored malate to citrate via the TCA cycle (Lüttge, 1988). In Bryophyllum calycinum (Pucher et al., 1949) during morning hours, citrate and isocitrate were present in equal to the concentration of malate, while in evening hours their concentration exceeded the concentration of malate by fivefold, and isocitrate was the prevalent acid. Citrate and malate accumulated equally in the CAM cycling plant Euphorbia milii (Herrera, 2013). Independent fluctuations of malate and citrate, related to photosynthesis and respiration respectively, were found in the CAM tree Clusia hilariana (Miszalski et al., 2013).
Citrate/isocitrate synthesis and utilization can be analyzed in a model that considers the open structure of the TCA cycle in the light and closed structure in the dark (Cheung et al., 2014). Accumulation of citrate in the dark via degradation of starch through glycolysis, pyruvate dehydrogenase and citrate synthase, according to this model, provides a carbon-neutral route for transferring ATP and reducing power from the light phase to the dark phase, conserving the net carbon required to support dark metabolism. The role of citrate and isocitrate in C4 and CAM metabolism needs further investigation, and the link of the C4 cycle with the C6 organic acids as the intermediates of the stored fixed carbon is important for understanding the whole structure of metabolism and bioenergetics of C4 and CAM plants. Cheung et al. (2014) also consider the link between the two branches of the TCA cycle in the light via isocitrate lyase. The form of this enzyme that operates outside of the glyoxylate cycle (Igamberdiev et al., 1986; Eprintsev et al., 2015b) may be important for balancing of the fluxes of succinate and isocitrate and linking the TCA cycle via glyoxylate to glycine and serine metabolism. This form is activated by lower pH and manganese ions (Eprintsev et al., 2015b). The whole metabolic structure of C4 and CAM photosynthesis can be considered a consequence of transformation of malate and citrate valves into the open structure of the TCA cycle to accommodate carbon fixation via PEP carboxylase.
Trans-Aconitate – A Stored Tricarboxylic Acid Pool
The tricarboxylic branch of the TCA cycle involves citrate, which is formed as a product of citrate synthase reaction, and its isomer D-threo-isocitrate, which is further decarboxylated to 2-oxoglutarate by either an NAD- or NADP-dependent isocitrate dehydrogenase. The conversion of citrate to isocitrate by aconitase involves the formation of cis-aconitate, a TCA that, being converted to the trans-form, can exist as a stable pool. Another TCA derived from the TCA cycle intermediates is hydroxycitrate (Figure 3). The aconitase reaction establishes an equilibrium that includes three organic acids: citrate, cis-aconitate and isocitrate. The aconitase equilibrium is shifted toward citrate; however, the ratio between its intermediates depends on the concentration of magnesium, affinity of aconitase to its substrates and other factors (Blair, 1969; Eprintsev et al., 2015a). In the TCA cycle cis-aconitate appears as a low-concentration intermediate, however, it can be accumulated in plants as the more stable isomer, trans-aconitate (Stout et al., 1967). Its concentration is above 1% in cereals (MacLennan and Beevers, 1964). In some plants like Asarum europaeum (Krogh, 1971) or aconitum (Otten and Mehltretter, 1970) trans-aconitate is a prevalently accumulating organic acid. It is a strong inhibitor of aconitase (Eprintsev et al., 2015a); therefore, it needs to be compartmented from both mitochondrial and cytosolic forms of this enzyme and transferred to vacuole.
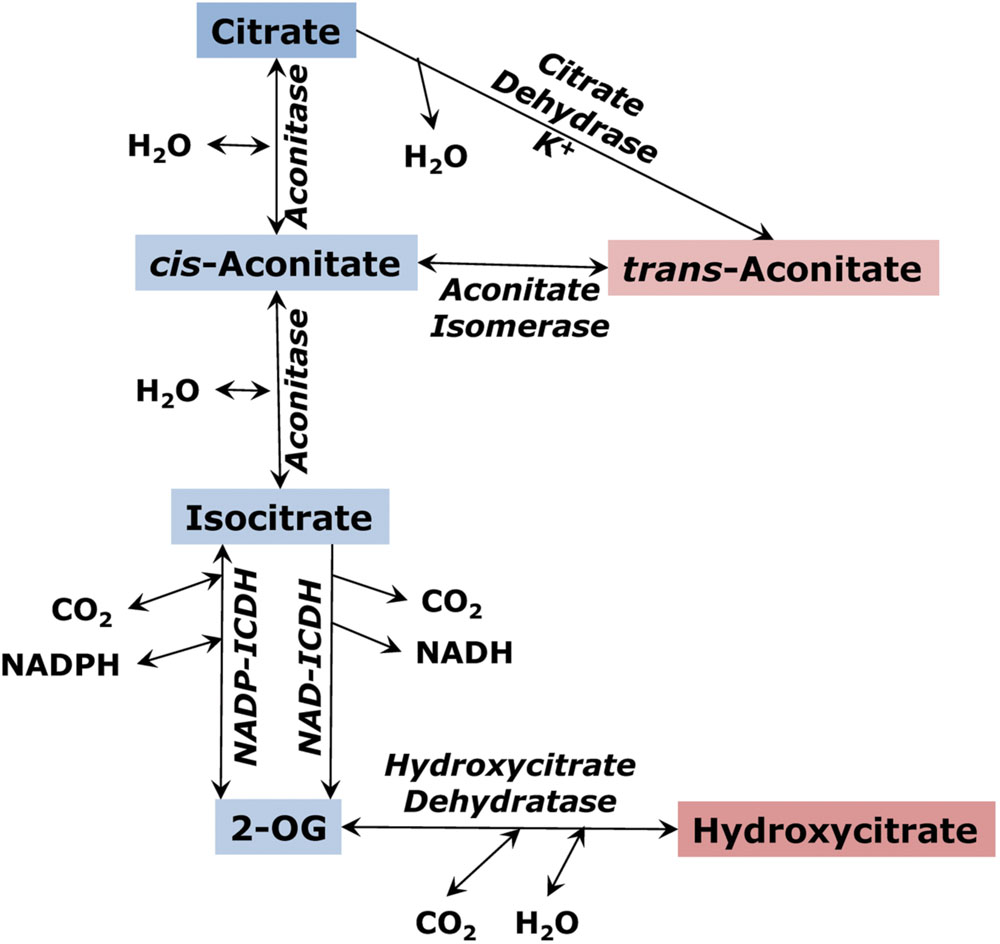
FIGURE 3. The citrate branch of the tricarboxylic acid cycle and the formation of trans-aconitate and hydroxycitrate as alternative pools of tricarboxylic acids.
Trans-aconitate is produced from cis-aconitate by aconitate isomerase (EC 5.3.3.7), an enzyme found in both microorganisms and plants (Thompson et al., 1997). Aconitate isomerase was detected first in bacteria (Rao and Altekar, 1961). MacLennan and Beevers (1964), by using a radioactive label, found that trans-aconitic acid is utilized by maize roots and therefore claimed that aconitate isomerase exists in higher plants. The location of aconitate isomerase in maize was more that 90% cytosolic, with the enzyme having a pH optimum of 8.0, a Km (trans-aconitate) of 3.3 mM, and a molecular weight of 80 kDa (Tkacheva and Zemlyanukhin, 1993). In barley leaves, aconitate isomerase was induced on the cytosolic ribosomes (cycloheximide-sensitive) by externally applied trans-aconitate occurring in the light but not in the dark (Figure 4), while in the roots its synthesis was induced by trans-aconitate in darkness (Zemlyanukhin and Eprintsev, 1979). This can indicate the role of trans-aconitate metabolism during photosynthesis in leaves.
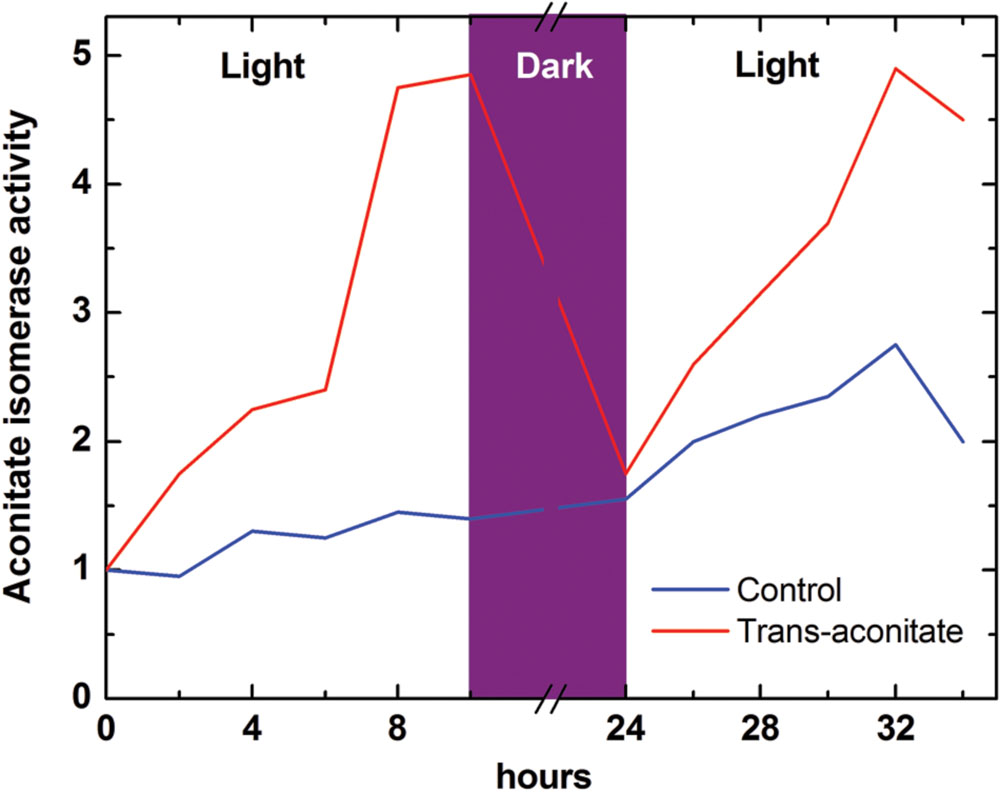
FIGURE 4. Induction of aconitate isomerase by trans-aconitate in the light in barley leaves (following Zemlyanukhin and Eprintsev, 1979). Aconitate isomerase activity was monitored as incorporation of the label from 6-14C-trans-aconitate (synthesized from 6-14C-citrate) into CO2 via aconitate isomerase, aconitase and isocitrate dehydrogenase reactions; the control value (at time 0 for leaves) is taken as 1. The induction of aconitate isomerase in roots by trans-aconitate was light-independent (not shown). Both in leaves and roots aconitate isomerase induction was suppressed by the inhibitor of translation on 80S ribosomes cycloheximide (not shown).
Another enzyme participating in trans-aconitic acid synthesis is citrate dehydrase (Brauer and Teel, 1981, 1982). This enzyme, in the presence of potassium, catalyzes the dehydration of citrate with the formation of trans-aconitate. The reaction is similar to that of aconitase, except with respect to the stereo-configuration of the product and its apparent inability to be converted to isocitrate. In this regard, the reaction produces a dead-end metabolic product, although its possible reversibility can return trans-aconitate back to metabolism. The kinetics of two molecular forms of K-dependent citrate dehydrase in leaves of maize (Zea mays L.) has been reported (Brauer and Teel, 1982). The isozymes were found to be compartmented in mitochondria (25%) and the cytosol (75%). The mitochondrial form exhibited hyperbolic kinetics with respect to both citrate and potassium with the Km values of 2.3 and 12 mM, respectively, and the pH optimum of 7.2. The cytosolic form exhibited the hyperbolic kinetics with respect to citrate (Km 0.6 mM) but the sigmoidal kinetics with respect to potassium. This may account for the positive correlation between leaf potassium and trans-aconitic acid in certain grasses (Clark, 1968). According to the reaction, citrate dehydrase represents an alternative pathway of citrate conversion in plants resulting in the formation of the compound that can be returned back to metabolism via the action of aconitate isomerase. The formation of trans-aconitate by citrate dehydrase in mitochondria takes place upon the increase of citrate concentration, while in the cytosol it is stimulated in a higher extent by the increase in potassium concentration. It is still not certain of what kind of enzyme it could be. There were no studies on identification of this protein or its genes, and no further investigation of its role in plant metabolism.
The action of the enzymes citrate dehydrase and aconitate isomerase results in plant cells in the alternative conversion of citrate, as compared to its primary metabolism toward isocitrate formation (aconitase) or acetyl-CoA and OAA production (ATP-citrate lyase). Similarly to fumarate, aconitic acid absorbs ultraviolet in the region at 240 nm. This light is completely absorbed by the ozone layer; therefore it is unlikely that this can have any physiological significance. Trans-aconitate may act as an antifeedant against brown plant hoppers (Katsuhara et al., 1993). It also induces tetany in ruminant animals by depleting free magnesium in blood (Bohman et al., 1969), which is caused at even higher intensity by the product of trans-aconitate conversion by bacteria, tricarballylate (Russell and Van Soest, 1984). Efficient binding of free magnesium displaces aconitase equilibrium, making it less directed toward citrate (Blair, 1969); it also affects operation of glycolysis and other processes (Igamberdiev and Kleczkowski, 2011). At zero magnesium, the citrate/isocitrate ratio established by aconitase is equal 9, while at 1 mM Mg2+ it is 21, and at 2 mM Mg2+ it is 36 (Blair, 1969); this means that in the conditions of effective binding of magnesium by trans-aconitate, organic acid metabolism is more directed toward isocitrate formation and, therefore, for amino acid biosynthesis. Trans-aconitate itself is an efficient inhibitor of the mitochondrial form of aconitase while its inhibition of the cytosolic form of aconitase is lower (Eprintsev et al., 2015a). Taken together, these data indicate the importance of trans-aconitate accumulation for regulation of the cytosolic and mitochondrial aconitases and possibly for directing metabolism toward isocitrate formation in the cytosol. Future studies should result in identification and characterizations of the genes of citrate dehydrase and aconitate isomerase in plants, their origin and distribution among plant species.
Hydroxycitrate and its Link to 2-Oxoglutarate
Hydroxycitrate was first discovered in sugar beet juice (Lippman, 1883) where it is present at low concentration. Later it was found at higher quantities in the species of Hibiscus (Griebel, 1942) and in fruits of Garcinia cambogia (up to 30% of dry weight) (Lewis and Neelakantan, 1965). Hydroxycitrate has two asymmetric centers and can exist as four stereo-isomers, however, only two of them were found in plants (Stallings et al., 1979). The first isomer, (+) allo-hydroxycitrate, was found in Hibiscus, while the second, (-) hydroxycitrate, was isolated from fruits of Garcinia. Shchiparev and Soldatenkov (1972) demonstrated that (+) allo-hydroxycitrate is readily metabolized not only in Hibiscus but also in maize, Phaseolus and other plants.
The enzyme interconverting hydroxycitrate and 2-oxoglutarate in Micrococcus bacteria has a molecular weight of 112 kDa, a Km (hydroxycitrate) of 0.35 mM, and a pH optimum of 7.7–8.3 (Zemlyanukhin and Zemlyanukhin, 1996). Such enzyme was also demonstrated by the same authors in the seedlings of Hibiscus cannabinus, but its purification was not achieved due to its instability. It may be similar to the enzyme dihydroxyacid dehydratase (EC 4.2.1.9) involved in metabolism of branched-chain amino acids (Kanamori and Wixom, 1963) and catalyzing dehydration of 2,3-dihydroxy-3-methylvalerate and 2,3-dihydroxyisovalerate to corresponding ketoacids, 2-keto-3-methylvalerate and 2-ketoisovalerate respectively (Oliver et al., 2012). This enzyme may be important for gametophyte development and for resistance to salinity stress in Arabidopsis (Zhang et al., 2015). Flint and Emptage (1988) and Pirrung et al. (1989) characterized this enzyme in spinach leaves and showed that it contains a [2Fe-2S] cluster, which resembles some similarity to aconitase. Isocitrate dehydrogenase (mostly NADP-dependent) exhibits some activity with the isomer of hydroxycitrate from Garcinia but not from Hibiscus (Plaut et al., 1975), forming 4-hydroxy-2-oxoglutarate. (-) Hydroxycitrate is a strong inhibitor of ATP-citrate lyase, which may be important for the suppression of lipid biosynthesis (Jena et al., 2002).
Accumulation of Malonate
Malonate is intensively accumulated in the plants belonging to legume family (Fabaceae). The pioneering work on malonate in plants (Bentley, 1952) demonstrated that it is usually accumulated at the levels of 0.5–2 mg per g of fresh weight in legumes. In Phaseolus vulgaris it is the main accumulating acid. Fougère et al. (1991) demonstrated that the highest concentration of malonate in alfalfa (Medicago sativa) plants is observed in bacteroids. Malonate is essential for symbiotic nitrogen metabolism (Kim, 2002). It exceeds 3.3% of dry weight in kidney vetch (Anthyllis vulneraria subsp. polyphylla), which corresponds to >10 mM, it accumulates at the level of 0.5% of dry weight in Astragalus dasyanthus, 0.2% in Trifolium pratense, i.e., in millimolar concentrations (Nikolaeva and Makeev, 1984). Malonate accumulates also at significant concentrations in the representatives of Umbelliferae (Apiaceae) family (e.g., in Anthriscus and Apium), where its concentration reaches 1 mg per g fresh weight (Bentley, 1952). While malonate is a potent inhibitor of succinate dehydrogenase and its exogenous application is toxic for plants (Chen et al., 2011), the efficient compartmentalization of this acid would prevent its strong inhibitory effect on metabolism.
de Vellis et al. (1963) and Shannon et al. (1963) studied malonate biosynthesis in bush bean roots and purified the enzyme catalyzing oxidative decarboxylation of OAA. The nature of this enzyme, however, was not further investigated. Ivanov and Zemlyanukhin (1991) traced the utilization of radiolabelled malate in different gaseous media and established the increase of malonate production from malate under hypoxia. This confirms that accumulation of malonate in these conditions can take place via the malate branch of the TCA cycle, presumably in the reaction of OAA decarboxylation.
Stumpf and Burris (1981) studied malonate biosynthesis in roots of soybean seedlings and showed that this compound is not a dead end product of metabolism. The pathway of malonate biosynthesis in young soybean root tissue takes place via acetyl-CoA carboxylase. It may involve further hydrolysis of malonyl-CoA, which is similar to the hydrolysis of acetyl-CoA leading to the formation of acetate in plants (Zemlyanukhina et al., 1986; Zeiher and Randall, 1990). In the pathway initiated by acetyl-CoA carboxylase, malonate serves as a direct precursor of neutral lipids. Groeneveld et al. (1992) demonstrated that malonate is the most effective precursor not only of neutral lipids but also of cardenolides (cardiac glycosides) via glucoevatromonoside in foxglove (Digitalis lanata). However, the pathway of lipid biosynthesis should start not necessarily from the carboxylation of acetyl CoA but can also involve directly the malonate pool. Chen et al. (2011) studied malonyl-CoA synthetase in Arabidopsis and showed that, although in plants as well as in animals, malonyl-CoA is commonly derived from acetyl-CoA by acetyl-CoA carboxylase (EC 6.4.1.2), it can be produced directly from malonic acid by malonyl-CoA synthetase (EC 6.2.1.14). Malonate can be oxidized with the formation of CO2, oxalate, glyoxylate and formate. This reaction is catalyzed by manganese peroxidase in the absence of hydrogen peroxide (Hofrichter et al., 1998). The pathways of malonate metabolism are schematically presented on Figure 5.
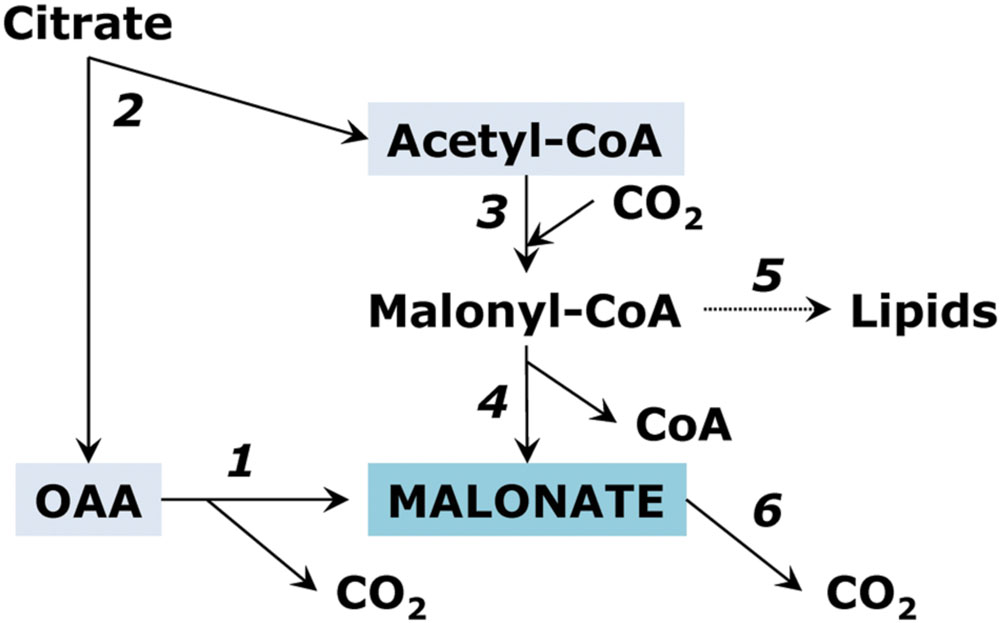
FIGURE 5. Reactions of malonate metabolism. (1) oxaloacetate decarboxylase reaction; (2) ATP-citrate lyase; (3) acetyl-CoA carboxylase; (4) malonyl-CoA hydrolase reaction; (5) biosynthesis of fatty acids; (6) oxidation of malonate to CO2.
Accumulation of Oxalate
Oxalate is one of the most actively accumulating organic acids in many plants such as spinach, beet, Oxalis. It can be present as a soluble form but also forms Ca-oxalate crystals in vacuoles, characterized by different shapes and different Ca2+ to oxalate ratios (Franceschi and Nakata, 2005). Oxalate crystal formation has a function of the storage of large amounts of Ca2+ in vacuoles, and prevention of the increase of Ca2+ level in other compartments, as well as the maintenance of the level of concentration gradient between the vacuole and the cytosol. Cereals, including wheat, are also efficient in oxalate accumulation, and its concentration in seeds may exceed 50 mg g-1, while in wheat bran it is 10 times higher (Siener et al., 2006).
The most evident way of oxalate accumulation is the oxidation of glyoxylate (Richardson and Tolbert, 1961; Igamberdiev et al., 1988; Yu et al., 2010). Glyoxylate can be formed during photorespiration in the reaction catalyzed by glycolate oxidase, or it can be produced by isocitrate lyase. Glycolate oxidase reaction was first demonstrated by Kolesnikov (1948) and Tolbert et al. (1949); later a possibility of glycolate dehydrogenase reaction was also suggested for higher plants (Bari et al., 2004). The enzymatic mechanism of glycolate and glyoxylate conversion is similar to that of lactate, the acid produced in high amounts during anaerobic fermentation.
Isocitrate lyase is active in the glyoxylate cycle during germination of oil-storing seeds but its different form is present in green leaves where it interconverts glyoxylate, succinate and isocitrate (Igamberdiev et al., 1986; Eprintsev et al., 2015b). This form is magnesium-independent, operates at low pH (6-6.5), localized in cytosol, and can be activated by manganese. The role of isocitrate lyase in the accumulation of oxalate was shown already in 1960s in Atriplex (Osmond and Avadhani, 1968). It is possible that respiratory intermediates can serve as precursors of oxalate in plant leaves. Millerd et al. (1962, 1963) detected isocitrate lyase in the absence of malate synthase in Oxalis seedlings and indicated the possibility of its participation in the biogenesis of oxalate. Recently the isocitrate pathway was demonstrated as dominant to oxalate biosynthesis in sorrel (Rumex obtusifolius) (Miyagi et al., 2013). Glycolate oxidase (or one of its forms) still can play a role in oxalate biosynthesis by utilizing, in its side reaction, the glyoxylate formed by isocitrate lyase. Splitting of OAA to oxalate and acetate is another possible pathway of oxalate synthesis (Chang and Beevers, 1968; Franceschi and Nakata, 2005). One more source of oxalate in plant tissues can be via degradation of ascorbate (Franceschi and Nakata, 2005; Green and Fry, 2005), however, recent studies show minor if any involvement of this pathway (Yu et al., 2010). Pathways of oxalate metabolism are schematically shown on Figure 6.
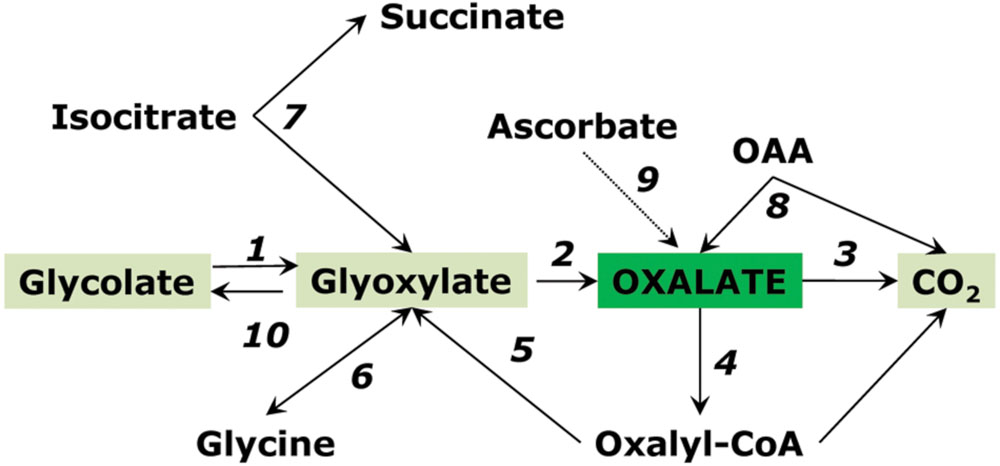
FIGURE 6. Pathways of oxalate conversion in plants. Enzymes: (1) glycolate oxidase; (2) glycolate oxidase (side reaction); (3) oxalate oxidase; (4) oxalyl-CoA synthetase; (5) oxalyl-CoA reductase; (6) aminotransferase; (7) isocitrate lyase; (8) oxaloacetate decarboxylase; (9) ascorbate pathway; (10) glyoxylate reductase. Reactions 1 and 2 can be catalyzed also by lactate dehydrogenase.
Accumulation of oxalate via glycolate oxidase can be explained by the kinetic properties of this enzyme, which exhibits the affinity not only to glycolate but also to its product glyoxylate, converting it to oxalate. Igamberdiev et al. (1988) studied the kinetics of glycolate oxidase isolated from wheat and sugar beet leaves and demonstrated that the affinity to glyoxylate of this enzyme is quite high (Km ∼1 mM) (Table 1). Figure 7 shows the rate of formation of glyoxylate and oxalate by the glycolate oxidase from wheat calculated from the obtained kinetic data and confirmed in the experiments on incorporation of the label from 1-14C-glycolate to glyoxylate and oxalate (Igamberdiev et al., 1988).
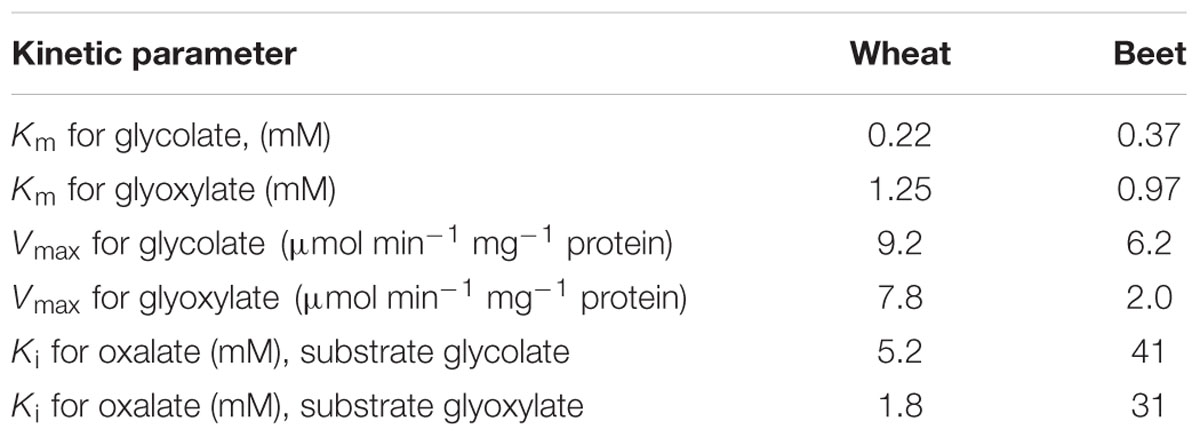
TABLE 1. Kinetic parameters of glycolate oxidase purified from wheat and sugar beet leaves (Igamberdiev et al., 1988).
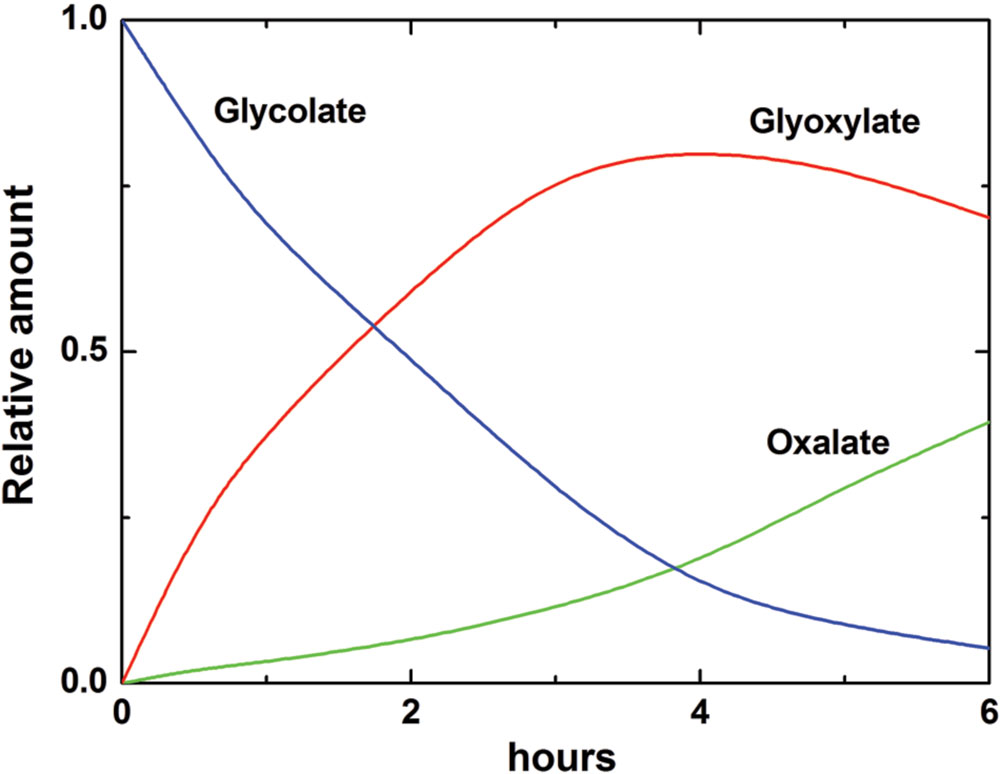
FIGURE 7. Oxidation of glycolate and formation of glyoxylate and oxalate by glycolate oxidase from wheat leaves. The changes of concentrations of glycolate, glyoxylate, and oxalate are calculated according to the kinetic equations based on the values of kinetic constants given in Table 1. Incorporation of the label from 1-14C-glycolate to glyoxylate and oxalate in the reaction catalyzed by the purified glycolate oxidase revealed a similar profile (Igamberdiev et al., 1988).
According to the Michaelis–Menten kinetics, the rate of oxidation of glycolate (and glyoxylate) is expressed by the following formula:
where S is the concentration of substrate (glycolate or glyoxylate), V is the maximal rate of oxidation of the corresponding substrate, Km is the Michaelis constant for the corresponding substrate, Ki is the inhibitory constant for the corresponding substrate by oxalate, I – concentration of oxalate. The inhibition by glyoxylate is negligible and, therefore, not included in the equation. The value of Ki for oxalate for the wheat enzyme was much lower in both reactions than for the sugar beet enzyme. This means that oxalate inhibits its own accumulation in wheat leaves but not in sugar beet leaves. The data indicate that glycolate oxidase may represent an efficient tool for oxalate accumulation via the reaction of glyoxylate oxidation.
The role of glycolate and glyoxylate metabolism in oxalate accumulation was supported by the data of Fujii et al. (1993) and in more detail confirmed in the study of Yu et al. (2010). The authors observed that when glycolate or glyoxylate were fed into detached leaves, both organic acids effectively stimulated oxalate accumulation, which was completely inhibited by the glyoxylate scavenger cysteine. Oxalate accumulation, however, was not correlated with photorespiration. It is possible that glyoxylate as a precursor of oxalate originates in several reactions, including the cytosolic isocitrate lyase. The same authors (Yu et al., 2010) showed that the downregulation of L-galactono-1,4-lactone dehydrogenase, a key enzyme for ascorbate biosynthesis, did not affect oxalate levels, which makes ascorbate the unlikely precursor of oxalate. On the other hand, the peroxisomal glycolate oxidase may not be a major route of oxalate formation (Xu et al., 2006; Tian et al., 2008). There are several enzymes that can oxidize glycolate and glyoxylate in higher plants (Havir, 1983), however, further studies are needed to identify all these proteins. Some of them have been recently characterized, e.g., glycolate oxidase 3 in Arabidopsis which is homologous to yeast L-lactate: cytochrome c oxidoreductase and supports L-lactate oxidation in roots (Engqvist et al., 2015). Glycolate metabolizing enzymes share specificity and sequence similarity with lactate metabolizing enzymes (Engqvist et al., 2009, 2015). Glycolate, similarly to lactate (both 2-hydroxyacids), is a major organic acid accumulating under hypoxic conditions in rice (Narsai et al., 2009), and its formation can take place via the reduction of glyoxylate at high reduction levels observed in anaerobic environments.
Oxalate can be efficiently incorporated in metabolism (Zemlyanukhin et al., 1972; Havir, 1984; Ivanov et al., 1989); therefore it is not an end product. Its incorporation may take place through the transformation to oxalyl-CoA by oxalyl-CoA synthetase (Giovanelli, 1966). Both oxalyl-CoA synthetase and oxalate oxidase are usually connected with oxalate degradation; however, oxalyl-CoA can also be incorporated in biosynthetic reactions via glyoxylate (Schneider et al., 2012). The formation of glyoxylate from the labeled oxalate was demonstrated by Havir (1984). Ivanov et al. (1989) showed that, in pea leaves, the label from oxalate incorporated into glycine and serine, and at low levels into glycolate, alanine, glutamate, citrate, and malate.
Recent identification of oxalyl-CoA synthetase in Arabidopsis provides a support for the pathway of oxalate degradation first proposed by Giovanelli and Tobin (1961), but it also indicates a possibility of oxalate incorporation in metabolism. Foster et al. (2012, 2016) have established the role of this enzyme in oxalate catabolism in Arabidopsis, in the regulation of calcium oxalate crystal accumulation, and in the defense against oxalate-secreting phytopathogens in Medicago truncatula. In some plant species, but not Arabidopsis, oxalate is degraded by oxalate oxidase, which activity is exhibited by some germin proteins (Lane et al., 1993; Druka et al., 2002). This activity produces hydrogen peroxide in vacuoles and cell walls playing a role in hypersensitive responses to pathogen attack. Oxalate strongly inhibits NADPH-dependent cytosolic glyoxylate/ hydroxypyruvate reductase (Kleczkowski et al., 1991; Igamberdiev and Kleczkowski, 2000) thus suppressing the oxidation of cytosolic NADPH and scavenging of glyoxylate and hydroxypuruvate leaked to the cytosol.
Another organic acid, linked to the glycolate pathway and glyoxylate conversion, is formate. Its metabolism was reviewed in detail in Igamberdiev et al. (1999). Formate can be produced in the reaction of glyoxylate with hydrogen peroxide (Grodzinski and Butt, 1976; Wingler et al., 1999). It can be oxidized to CO2; however, formate dehydrogenase is induced mostly in heterotrophic tissues under stress conditions (Hourton-Cabassa et al., 1998). It is regulated via phosphorylation in a similar way as pyruvate dehydrogenase (Bykova et al., 2003) pointing possible glycolytic origin of formate in stressed heterotrophic tissues. However, the enzyme that converts pyruvate to formate (pyruvate: formate lyase) has not been yet identified in plants, it was identified only in Chlamydomonas among eukaryotic photosynthetic organisms (Atteia et al., 2006). Formate potentially could be formed via the reduction of CO2, the reaction which was considered for CO2 fixation before the discovery of the Calvin-Benson cycle (Baeyer, 1870). A small amount of formate possibly could be produced in photosynthetic electron transport chain (Kent, 1972); however, this reaction can have only very minor, if any, contribution to CO2 fixation. Condensation of two formate molecules can potentially yield glyoxylate which is further converted to glycine and serine, and then to other metabolites. This C1 pathway of photosynthesis was claimed for greening potato tubers (Ramaswamy and Nair, 1984) but never confirmed (for details see Igamberdiev et al., 1999). The link of glyoxylate to formate and other organic acids is shown on Figure 8.
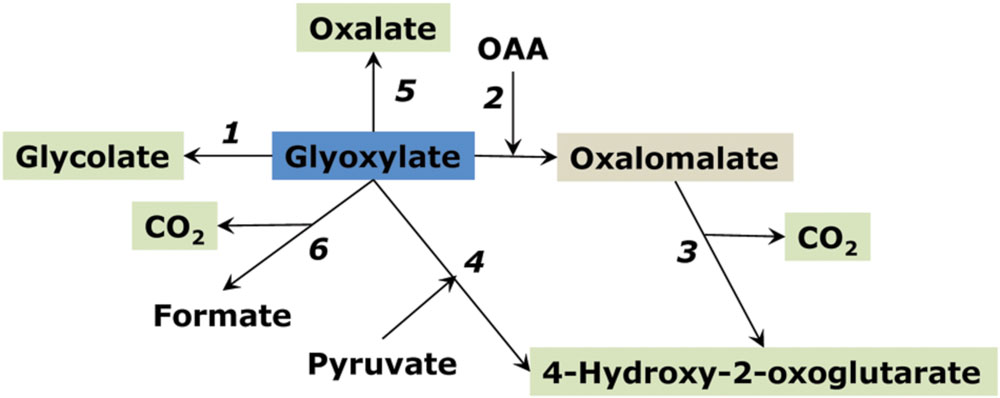
FIGURE 8. Pathways of glyoxylate conversion in plants. (1) reduction to glycolate; (2) non-enzymatic condensation with oxaloacetate (OAA) forming oxalomalate; (3) the latter is decarboxylated to 4-hydroxy-2-oxoglutarate; (4) enzymatic condensation with pyruvate forming 4-hydroxy-2-oxoglutarate; (5) oxidation to oxalate; (6) decarboxylation to formate.
Hydroxyketoglutarate and Oxalomalate
Hydroxyketoglutarate (4-hydroxy-2-oxoglutarate) can be formed from glyoxylate and pyruvate by the enzyme 4-hydroxy-2-oxoglutarate aldolase (EC 4.1.3.16) (Kobes and Dekker, 1966). Oxalomalate is formed from glyoxylate and OAA by the enzyme oxalomalate lyase (EC 4.1.3.13); it may also be a side activity of 4-hydroxy-2-oxoglutarate aldolase (Scholtz and Schuster, 1984) (Figure 8). Decarboxylation of oxalomalate results in hydroxyketoglutarate formation (Ruffo et al., 1967; Adinolfi et al., 1969). Glyoxylate for hydroxyketoglutarate formation can originate from isocitrate lyase reaction (Morton and Wells, 1964).
Both oxalomalate and hydroxyketoglutarate are strong inhibitors of aconitase and isocitrate dehydrogenase (oxalomalate is one order of magnitude stronger inhibitor). Although the data on enzymatic formation and conversion of these acids in plants is very limited, it is known that hydroxyketoglutarate accumulates in several plants at significant concentrations, especially in oxalate accumulating plants, which provides an indirect evidence of glyoxylate as a precursor of both oxalate and hydroxyketoglutarate (Morton and Wells, 1964). The efficiency of oxalomalate and hydroxyketoglutarate and of glyoxylate itself in the inhibition of key enzymes of the TCA cycle may be important in the suppression of mitochondrial respiration during photorespiration, resulting in the citrate efflux from mitochondria and activation of the alternative oxidase (Gupta et al., 2012). Hydroxyketoglutarate aldolase reaction can be detected in several plants but with low activity (Igamberdiev, unpublished) and may appear as a result of the side activity of some aldolase with a broad specificity. Hydroxyketoglutarate can undergo oxidative decarboxylation with the formation of malate and reduction of NAD+ (Payes and Laties, 1963). The side reaction of malate dehydrogenase can result in the formation of another accumulating organic acid, L-2-hydroxyglutarate, which is oxidized to 2-oxoglutarate by L-2-hydroxyglutarate dehydrogenase (Hüdig et al., 2015).
Excretion of Citrate, Malate and Oxalate and Weathering of Rocks
Organic acids in plants being the intermediates of photosynthetically driven carbon metabolism acquire several important physiological functions, one of which is related to their effects on the external environment. While vacuole represents the internalized external space being topologically equivalent to the cell exterior (Vitale and Denecke, 1999; Igamberdiev and Lea, 2002), the excretion of organic acids to the external medium is alternative to their vacuolar accumulation. In germinating cereal seeds, organic acids are excreted from scutellum and aleurone layer to endosperm to provide acidic conditions for breakdown of starch (Shchiparev et al., 1976; Ma et al., 2016). Roots can excrete diverse compounds of both primary and secondary metabolism, such as carbohydrates, carboxylates, phenolics and terpenoids, and the role of organic acids is especially important in building up of modern biosphere. Citrate and malate are the major excreted organic acids, and some plants excrete large quantities of oxalate (Meyer et al., 2010a).
Malate and citrate can be exported by three classes of transporters. Malate excretion at the plasma membrane occurs by aluminum-activated malate transporter (ALMT) channels, while it can also be excreted to the vacuole by tDT transporter. Citrate excretion is catalyzed by members of the MATE transporters. The detailed characterization of these transporters is given in the review of Meyer et al. (2010a). The excreted citrate, malate and oxalate can form complexes with micronutrients and toxic metals. At neutral pH, the stability constants between citrate and metals (Fe3+, Al3+, Zn2+ and Cd2+) are higher for citrate than for malate and oxalate, while at acidic pH, oxalate forms stronger complexes with metals than do malate and citrate due to its lower pK (Jones, 1998). The excretion of malate and citrate is very important for aluminum tolerance and for release of rock phosphate in cluster root forming plants, e.g., in white lupine (Massonneau et al., 2001). The aluminum tolerant cultivars of wheat have a greater ability to excrete malate from roots (Delhaize et al., 1993; de Andrade et al., 2011). In soybean cultivars, aluminum tolerance is associated with the excretion of citrate which sustained for longer period than the excretion of malate (Silva et al., 2001). Citrate plays also an important role for iron acquisition and transport within the plant (Durrett et al., 2007).
Excretion of citrate and malate is related to the intensity of enzymes of the two branches of the TCA cycle, while the mechanisms of oxalate excretion are less certain. The detailed studies have been performed to elucidate the mechanism of excretion of citrate. Overexpression of Arabidopsis citrate synthase in carrot cells improved the growth in aluminum phosphate medium as a result of citrate excretion, which was three to four times greater as compared to control (Koyama et al., 1999). When the gene for mitochondrial citrate synthase from carrot was introduced into Arabidopsis, a 2.5-fold increase in excretion of citrate was observed with a correlation between the levels of citrate synthase activity and the amounts of citrate excreted into the medium (Koyama et al., 2000). The overexpression of citrate synthase improved the growth in phosphorus limited soil as a result of enhanced citrate excretion from the roots. Aluminum tolerance also increased in transgenic canola overexpressing citrate synthase (Anoop et al., 2003). Overexpression of the mitochondrial malate dehydrogenase improved phosphorus acquisition by tobacco plants (Lü et al., 2012).
The role of excretion of organic acids is globally biospheric. It is considered as the main cause of soil formation via weathering of rocks starting from the Ordovician period, when bryophytes colonized land (Lenton et al., 2012), and then continuing with the rooted plants in Silurian and Devonian (Raven and Edwards, 2001; Igamberdiev and Lea, 2006). The decrease in the atmospheric CO2 during these times is also attributed to weathering of rocks when calcium silicates were replaced by carbonates (Berner, 1993, 1997). Although the regulation of atmospheric CO2 via the influence on weathering of rocks is attributed to the heterotrophic metabolism of roots, it uses photosynthetically fixed carbon and therefore complements the direct regulation of CO2/O2 ratio by photosynthesis and photorespiration (Tolbert et al., 1995).
Conclusion
Organic acids accumulate in plants mainly as a result of the incomplete oxidation of photosynthetic products and represent the stored pools of fixed carbon due to different transient times of conversion of carbon compounds in metabolic pathways. The increase in the redox level in the cell in the conditions of active photosynthesis results in the transformation of the TCA cycle to an open branched structure supplying citrate for the synthesis of 2-oxoglutarate and glutamate (citrate valve), while accumulation of malate regulates the redox balance in different cell compartments (via malate valve). Besides the organic acids – intermediates of the TCA cycle, several carboxylates are linked with photorespiration, catabolism of amino acids and other processes. The intermediates of the TCA cycle can be transformed to the secondary organic acids that form the additional pools of fixed carbon and stabilize the operation of TCA cycle and regulate redox balance. Metabolism of the secondary organic acid such as hydroxycitrate, trans-aconitate, hydroxyketoglutarate can be related to the side activities of known enzymes, however, in most cases it involves several plant enzymes that are not well characterized, such as citrate dehydrase, aconitate isomerase, putative hydroxycitrate dehydratase (decarboxylating), or hydroxyketoglutarate aldolase. Although the activities of these enzymes were detected and some of them are partially purified and characterized, they are not studied at the genetic level, and the knowledge about them is very incomplete. Further studies in this direction are important to clarify the specificity of plant carbon metabolism and to identify novel metabolic pathways operating in photosynthetic organisms.
Author Contributions
All authors listed, have made substantial, direct and intellectual contribution to the work, and approved it for publication.
Conflict of Interest Statement
The authors declare that the research was conducted in the absence of any commercial or financial relationships that could be construed as a potential conflict of interest.
Acknowledgments
This paper is dedicated to the memory of Professor Alexander Alexeyevich Zemlyanukhin (1918-1996) who made major contribution to the pioneering research in the fields of enzymology and metabolism of plant organic acids in Russia. This work was supported by the grant 14-14-00721 of the Russian Science Foundation (to AE and AI) and by the grant of the Natural Sciences and Engineering Research Council of Canada (to AI). The authors thank Professor Robert D. Hill (University of Manitoba, Canada) for his valuable comments and helpful suggestions.
References
Adinolfi, A., Moratti, R., Olezza, S., and Ruffo, A. (1969). Control of the citric acid cycle by glyoxylate. The mechanism of inhibition of oxoglutarate dehydrogenase, isocitrate dehydrogenase and aconitate hydratase. Biochem. J. 114, 513–518. doi: 10.1042/bj1140513
Anoop, V. M., Basu, U., McCammon, M. T., McAlister-Henn, L., and Taylor, G. J. (2003). Modulation of citrate metabolism alters aluminum tolerance in yeast and transgenic canola overexpressing a mitochondrial citrate synthase. Plant Physiol. 132, 2205–2217. doi: 10.1104/pp.103.023903
António, C., Päpke, C., Rocha, M., Diab, H., Limami, A. M., Obata, T., et al. (2016). Regulation of primary metabolism in response to low oxygen availability as revealed by carbon and nitrogen isotope redistribution. Plant Physiol. 170, 43–56. doi: 10.1104/pp.15.00266
Atteia, A., van Lis, R., Gelius-Dietrich, G., Adrait, A., Garin, J., Joyard, J., et al. (2006). Pyruvate formate lyase and a novel route of eukaryotic ATP synthesis in Chlamydomonas mitochondria. J. Biol. Chem. 281, 9909–9918. doi: 10.1074/jbc.M507862200
Baeyer, A. D. (1870). Ueber die Wasserentziehung und ihre Bedeutung für das Pflanzenleben und die Gährung. Ber. Dtsch. Chem. Ges. 3, 63–75. doi: 10.1002/cber.18700030123
Bari, R., Kebeish, R., Kalamajka, R., Rademacher, T., and Peterhänsel, C. (2004). A glycolate dehydrogenase in the mitochondria of Arabidopsis thaliana. J. Exp. Bot. 55, 623–630. doi: 10.1093/jxb/erh079
Bentley, L. E. (1952). Occurrence of malonic acid in plants. Nature 170, 847–848. doi: 10.1038/170847b0
Berner, R. A. (1993). Paleozoic atmospheric CO2: importance of solar radiation and plant evolution. Science 261, 68–70. doi: 10.1126/science.261.5117.68
Berner, R. A. (1997). The rise of plants and their effect on weathering and atmospheric CO2. Science 276, 544–546. doi: 10.1126/science.276.5312.544
Blair, J. M. (1969). Magnesium and the aconitase equilibrium: determination of apparent stability constants of magnesium substrate complexes from equilibrium data. Eur. J. Biochem. 8, 287–291. doi: 10.1111/j.1432-1033.1969.tb00526.x
Bohman, V. R., Lesperance, A. L., Harding, G. D., and Grunes, D. L. (1969). Induction of experimental tetany in cattle. J. Anim. Sci. 29, 99–102.
Brauer, D., and Teel, M. R. (1981). Metabolism of trans-aconitic acid in maize. 1. Purification of two molecular forms of citrate dehydrase. Plant Physiol. 68, 1406–1408. doi: 10.1104/pp.68.6.1406
Brauer, D., and Teel, M. R. (1982). Metabolism of trans-aconitic acid in maize. 2. Regulatory properties of two compartmented forms of citrate dehydrase. Plant Physiol. 70, 723–727. doi: 10.1104/pp.70.3.723
Burnell, J. N., and Hatch, M. D. (1988). Photosynthesis in phosphoenolpyruvate carboxykinase-type C4 plants: pathways of C4 acid decarboxylation in bundle sheath cells of Urochloa panicoides. Arch. Biochem. Biophys. 260, 187–199. doi: 10.1016/0003-9861(88)90440-7
Bykova, N. V., Møller, I. M., Gardeström, P., and Igamberdiev, A. U. (2014). The function of glycine decarboxylase complex is optimized to maintain high photorespiratory flux via buffering of its reaction products. Mitochondrion 19B, 357–364. doi: 10.1016/j.mito.2014.01.001
Bykova, N. V., Stensballe, A., Egsgaard, H., Jensen, O. N., and Møller, I. M. (2003). Phosphorylation of formate dehydrogenase in potato tuber mitochondria. J. Biol. Chem. 278, 26021–26030. doi: 10.1074/jbc.M300245200
Chang, C. C., and Beevers, H. (1968). Biogenesis of oxalate in plant tissues. Plant Physiol. 43, 1821–1828. doi: 10.1104/pp.43.11.1821
Chen, H., Kim, H. U., Weng, H., and Browse, J. (2011). Malonyl-CoA synthetase, encoded by ACYL ACTIVATING ENZYME13, is essential for growth and development of Arabidopsis. Plant Cell 23, 2247–2262. doi: 10.1105/tpc.111.086140
Chen, R. D., and Gadal, P. (1990). Do the mitochondria provide the 2-oxoglutarate needed for glutamate synthesis in higher plant chloroplasts? Plant Physiol. Biochem. 28, 141–145.
Cheung, C. Y., Poolman, M. G., Fell, D. A., Ratcliffe, R. G., and Sweetlove, L. J. (2014). A diel flux balance model captures interactions between light and dark metabolism during day-night cycles in C3 and Crassulacean acid metabolism leaves. Plant Physiol. 165, 917–929. doi: 10.1104/pp.113.234468
Chia, D. W., Yoder, T. J., Reiter, W. D., and Gibson, S. I. (2000). Fumaric acid: an overlooked form of fixed carbon in Arabidopsis and other plant species. Planta 211, 743–751. doi: 10.1007/s004250000345
Clark, R. B. (1968). Organic acids of maize (Zea mays L.) as influenced by mineral deficiencies. Crop Sci. 8, 165–167. doi: 10.2135/cropsci1968.0011183X000800020007x
Corpas, F. J., Barroso, J. B., Sandalio, L. M., Palma, J. M., Lupiáñez, J. A., and del Río, L. A. (1999). Peroxisomal NADP-dependent isocitrate dehydrogenase. Characterization and activity regulation during natural senescence. Plant Physiol. 121, 921–928. doi: 10.1104/pp.121.3.921
Crawford, L. A., Bown, A. W., Breitkreuz, K. E., and Guinel, F. C. (1994). The synthesis of γ-aminobutyric acid in response to treatments reducing cytosolic pH. Plant Physiol. 104, 865–871.
Daloso, D. M., Müller, K., Obata, T., Florian, A., Tohge, T., Bottcher, A., et al. (2015). Thioredoxin, a master regulator of the tricarboxylic acid cycle in plant mitochondria. Proc. Natl. Acad. Sci. U.S.A. 112, E1392–E1400. doi: 10.1073/pnas.1424840112
de Andrade, L. R., Ikeda, M., do Amaral, L. I., and Ishizuka, J. (2011). Organic acid metabolism and root excretion of malate in wheat cultivars under aluminium stress. Plant Physiol. Biochem. 49, 55–60. doi: 10.1016/j.plaphy.2010.09.023
de Vellis, J., Shannon, L. M., and Lew, J. Y. (1963). Malonic acid biosynthesis in bush bean (Phaseolus vulgaris var. Tendergreen) roots. I. Evidence for oxaloacetate as immediate precursor. Plant Physiol. 38, 686–690. doi: 10.1104/pp.38.6.686
Delhaize, E., Ryan, P. R., and Randall, P. J. (1993). Aluminum tolerance in wheat (Triticum aestivum L.). II. Aluminum-stimulated excretion of malic acid from root apices. Plant Physiol. 103, 695–702.
Drincovich, M. F., Casati, P., and Andreo, C. S. (2001). NADP-malic enzyme from plants: a ubiquitous enzyme involved in different metabolic pathways. FEBS Lett. 490, 1–6. doi: 10.1016/S0014-5793(00)02331-0
Druka, A., Kudrna, D., Kannangara, C. G., von Wettstein, D., and Kleinhofs, A. (2002). Physical and genetic mapping of barley (Hordeum vulgare) germin-like cDNAs. Proc. Natl. Acad. Sci. U.S.A. 99, 850–855. doi: 10.1073/pnas.022627999
Durrett, T. P., Gassmann, W., and Rogers, E. E. (2007). The FRD3-mediated efflux of citrate into the root vasculature is necessary for efficient iron translocation. Plant Physiol. 144, 197–205. doi: 10.1104/pp.107.097162
Easterby, J. (1996). The fusion of control analysis and temporal analysis of metabolic systems. J. Theor. Biol. 182, 327–331. doi: 10.1006/jtbi.1996.0171
Engqvist, M., Drincovich, M. F., Flügge, U. I., and Maurino, V. G. (2009). Two D-2-hydroxy-acid dehydrogenases in Arabidopsis thaliana with catalytic capacities to participate in the last reactions of the methylglyoxal and beta-oxidation pathways. J. Biol. Chem. 284, 25026–25037. doi: 10.1074/jbc.M109.021253
Engqvist, M. K., Schmitz, J., Gertzmann, A., Florian, A., Jaspert, N., Arif, M., et al. (2015). GLYCOLATE OXIDASE3, a glycolate oxidase homolog of yeast L-lactate cytochrome c oxidoreductase, supports L-lactate oxidation in roots of Arabidopsis. Plant Physiol. 169, 1042–1061. doi: 10.1104/pp.15.01003
Eprintsev, A. T., Fedorin, D. N., and Igamberdiev, A. U. (2013). Ca2+ is involved in phytochrome A-dependent regulation of the succinate dehydrogenase gene sdh1-2 in Arabidopsis. J. Plant Physiol. 170, 1349–1352. doi: 10.1016/j.jplph.2013.04.006
Eprintsev, A. T., Fedorin, D. N., Nikitina, M. V., and Igamberdiev, A. U. (2015a). Expression and properties of the mitochondrial and cytosolic forms of aconitase in maize scutellum. J. Plant Physiol. 181, 14–19. doi: 10.1016/j.plph.2015.03.012
Eprintsev, A. T., Fedorin, D. N., Salnikov, A. V., and Igamberdiev, A. U. (2015b). Expression and properties of the glyoxysomal and cytosolic forms of isocitrate lyase in Amaranthus caudatus L. J. Plant Physiol. 181, 1–8. doi: 10.1016/j.jplph.2015.02.014
Eprintsev, A. T., Fedorin, D. N., Sazonova, O. V., and Igamberdiev, A. U. (2016). Light inhibition of fumarase in Arabidopsis leaves is phytochrome A-dependent and mediated by calcium. Plant Physiol. Biochem. 102, 161–166. doi: 10.1016/j.plaphy.2016.02.028
Eprintsev, A. T., Fedorin, D. N., Starinina, E. V., and Igamberdiev, A. U. (2014). Expression and properties of the mitochondrial and cytosolic forms of fumarase in germinating maize seeds. Physiol. Plant. 152, 231–240. doi: 10.1111/ppl.12181
Finkemeier, I., König, A. C., Heard, W., Nunes-Nesi, A., Pham, P. A., Leister, D., et al. (2013). Transcriptomic analysis of the role of carboxylic acids in metabolite signaling in Arabidopsis leaves. Plant Physiol. 162, 239–253. doi: 10.1104/pp.113.214114
Flint, D. H., and Emptage, M. H. (1988). Dihydroxy acid dehydratase from spinach contains a [2Fe-2S] cluster. J. Biol. Chem. 263, 3558–3564.
Foster, J., Kim, H. U., Nakata, P. A., and Browse, J. (2012). A previously unknown oxalyl-CoA synthetase is important for oxalate catabolism in Arabidopsis. Plant Cell 24, 1217–1229. doi: 10.1105/tpc.112.096032
Foster, J., Luo, B., and Nakata, P. A. (2016). An oxalyl-CoA dependent pathway of oxalate catabolism plays a role in regulating calcium oxalate crystal accumulation and defending against oxalate-secreting phytopathogens in Medicago truncatula. PLoS ONE 11:e0149850. doi: 10.1371/journal.pone.0149850
Fougère, F., Le Rudulier, D., and Streeter, J. G. (1991). Effects of salt stress on amino acid, organic acid, and carbohydrate composition of roots, bacteroids, and cytosol of alfalfa (Medicago sativa L.). Plant Physiol. 96, 1228–1236. doi: 10.1104/pp.96.4.1228
Franceschi, V. R., and Nakata, P. A. (2005). Calcium oxalate in plants: formation and function. Ann. Rev. Plant Biol. 56, 41–71. doi: 10.1146/annurev.arplant.56.032604.144106
Fridlyand, L. E., and Scheibe, R. (1999). Regulation of the Calvin cycle for CO2 fixation as an example for general control mechanisms in metabolic cycles. BioSystems 51, 79–93. doi: 10.1016/S0303-2647(99)00017-9
Fujii, N., Watanabe, M., Watanabe, Y., and Shimada, N. (1993). Rate of oxalate biosynthesis from glycolate and ascorbic acid in spinach leaves. Soil Sci. Plant Nutr. 39, 627–634. doi: 10.1080/00380768.1993.10419179
Gardeström, P., and Igamberdiev, A. U. (2016). The origin of cytosolic ATP in photosynthetic cells. Physiol. Plant 157, 367–379. doi: 10.1111/ppl.12455
Gardeström, P., Igamberdiev, A. U., and Raghavendra, A. S. (2002). “Mitochondrial functions in the light and significance to carbon-nitrogen interactions,” in Photosynthetic Nitrogen Assimilation and Associated Carbon and Respiratory Metabolism (Advances in Photosynthesis and Respiration, vol. 12), Chap. 10, eds C. H. Foyer and G. Noctor (Dordrecht: Kluwer Academic Publishers), 151–172.
Geigenberger, P., and Fernie, A. R. (2011). Metabolic control of redox and redox control of metabolism in plants. Antiox. Redox Signal. 21, 1389–1421. doi: 10.1089/ars.2014.6018
Gerrard Wheeler, M. C., Arias, C. L., Maurino, V. G., Andreo, C. S., and Drincovich, M. F. (2009). Identification of domains involved in the allosteric regulation of cytosolic Arabidopsis thaliana NADP-malic enzymes. FEBS J. 276, 5665–5677. doi: 10.1111/j.1742-4658.2009.07258.x
Gietl, C. (1992). Malate dehydrogenase isoenzymes: cellular locations and role in the flow of metabolites between the cytoplasm and cell organelles. Biochim. Biophys. Acta 1100, 217–234. doi: 10.1016/0167-4838(92)90476-T
Giovanelli, J. (1966). Oxalyl-coenzyme A synthetase from pea seeds. Biochim. Biophys. Acta 118, 124–143. doi: 10.1016/S0926-6593(66)80151-0
Giovanelli, J., and Tobin, N. F. (1961). Adenosine triphosphate- and coenzyme A-dependent decarboxylation of oxalate by extracts of peas. Nature 190, 1006–1007. doi: 10.1038/1901006a0
Gout, E., Bligny, R., Pascal, N., and Douce, R. (1993). 13C nuclear magnetic resonance studies of malate and citrate synthesis and compartmentation in higher plant cells. J. Biol. Chem. 268, 3986–3992.
Green, M. A., and Fry, S. C. (2005). Vitamin C degradation in plant cells via enzymatic hydrolysis of 4-O-oxalyl-L-threonate. Nature 433, 83–87. doi: 10.1038/nature03172
Griebel, C. (1942). Über die Konstitution und den Nachweis von Hibiscussäure ((+)-allo-Oxycitronensäurelacton). Z. Untersuch. Lebensmittel 83, 481–486. doi: 10.1007/BF01796419
Grivennikova, V. G., Gavrikova, E. V., Timoshin, A. A., and Vinogradov, A. D. (1993). Fumarate reductase activity of bovine heart succinate-ubiquinone reductase. New assay system and overall properties of the reaction. Biochim. Biophys. Acta 1140, 282–292. doi: 10.1016/0005-2728(93)90067-P
Grodzinski, B., and Butt, V. S. (1976). Hydrogen peroxide production and the release of carbon dioxide during glycollate oxidation in leaf peroxisomes. Planta 128, 225–231. doi: 10.1007/BF00393233
Groeneveld, H. W., van Tegelen, L. J., and Versluis, K. (1992). Cardenolide and neutral lipid biosynthesis from malonate in Digitalis lanata. Planta Med. 58, 239–244. doi: 10.1055/s-2006-961444
Gupta, K. J., Shah, J. K., Brotmann, Y., Willmitzer, L., Kaiser, W. M., Bauwe, H., et al. (2012). Inhibition of aconitase by nitric oxide leads to induction of the alternative oxidase and to a shift of metabolism towards biosynthesis of amino acids. J. Exp. Bot. 63, 1773–1784. doi: 10.1093/jxb/ers053
Guynn, R. W., Gelberg, H. J., and Veech, R. L. (1973). Equilibrium constants of the malate dehydrogenase, citrate synthase, citrate lyase, and acetyl coenzyme A hydrolysis reactions under physiological conditions. J. Biol. Chem. 248, 6957–6965.
Hanning, I., and Heldt, H. W. (1993). On the function of mitochondrial metabolism during photosynthesis in spinach (Spinacia oleracea L.) leaves. Partitioning between respiration and export of redox equivalents and precursors for nitrate assimilation products. Plant Physiol. 103, 1147–1154.
Hausladen, A., and Fridovich, I. (1994). Superoxide and peroxynitrite inactivate aconitases, but nitric oxide does not. J. Biol. Chem. 269, 29405–29408.
Havir, E. A. (1983). Evidence for the presence in tobacco leaves of multiple enzymes for the oxidation of glycolate and glyoxylate. Plant Physiol. 71, 874–878. doi: 10.1104/pp.71.4.874
Havir, E. A. (1984). Oxalate metabolism by tobacco leaf discs. Plant Physiol. 75, 505–507. doi: 10.1104/pp.75.2.505
Heineke, D., Riens, B., Grosse, H., Hoferichter, P., Peter, U., Flügge, U. I., et al. (1991). Redox transfer across the inner chloroplast envelope membrane. Plant Physiol. 95, 1131–1137. doi: 10.1104/pp.95.4.1131
Herrera, A. (2013). Crassulacean acid metabolism-cycling in Euphorbia milii. AoB Plants 5:lt014. doi: 10.1093/aobpla/plt014
Hofrichter, M., Ziegenhagen, D., Vares, T., Friedrich, M., Jager, M. G., Fritsche, W., et al. (1998). Oxidative decomposition of malonic acid as basis for the action of manganese peroxidase in the absence of hydrogen peroxide. FEBS Lett. 434, 362–366. doi: 10.1016/S0014-5793(98)01023-0
Hourton-Cabassa, C., Ambard-Bretteville, F., Moreau, F., Davy de Virville, J., Rémy, R., and Francs-Small, C. C. (1998). Stress induction of mitochondrial formate dehydrogenase in potato leaves. Plant Physiol. 116, 627–635. doi: 10.1104/pp.116.2.627
Hüdig, M., Maier, A., Scherrers, I., Seidel, L., Jansen, E. E., Mettler-Altmann, T., et al. (2015). Plants possess a cyclic mitochondrial metabolic pathway similar to the mammalian metabolic repair mechanism involving malate dehydrogenase and L-2-hydroxyglutarate dehydrogenase. Plant Cell Physiol. 56, 1820–1830. doi: 10.1093/pcp/pcv108
Igamberdiev, A. U., Bykova, N. V., and Kleczkowski, L. A. (1999). Origins and metabolism of formate in higher plants. Plant Physiol. Biochem. 37, 503–513. doi: 10.1016/S0981-9428(00)80102-3
Igamberdiev, A. U., Bykova, N. V., Lea, P. J., and Gardeström, P. (2001a). The role of photorespiration in redox and energy balance of photosynthetic plant cells: a study with a barley mutant deficient in glycine decarboxylase. Physiol. Plant. 111, 427–438. doi: 10.1034/j.1399-3054.2001.1110402.x
Igamberdiev, A. U., Eprintsev, A. T., Fedorin, D. N., and Popov, V. N. (2014a). Phytochrome-mediated regulation of plant respiration and photorespiration. Plant Cell Environ. 37, 290–299. doi: 10.1111/pce.12155
Igamberdiev, A. U., and Gardeström, P. (2003). Regulation of NAD- and NADP-dependent isocitrate dehydrogenases by reduction levels of pyridine nucleotides in mitochondria and cytosol of pea leaves. Biochim. Biophys. Acta 1606, 117–125. doi: 10.1016/S0005-2728(03)00106-3
Igamberdiev, A. U., Hurry, V., Krömer, S., and Gardeström, P. (1998). The role of mitochondrial electron transport during photosynthetic induction. A study with barley (Hordeum vulgare) protoplasts incubated with rotenone and oligomycin. Physiol. Plant. 104, 431–439. doi: 10.1034/j.1399-3054.1998.1040319.x
Igamberdiev, A. U., and Kleczkowski, L. A. (2000). Capacity for NADPH/NADP turnover in the cytosol of barley seed endosperm: the role of NADPH-dependent hydroxypyruvate reductase. Plant Physiol. Biochem. 38, 747–753. doi: 10.1016/S0981-9428(00)01187-6
Igamberdiev, A. U., and Kleczkowski, L. A. (2011). Magnesium and cell energetics in plants under anoxia. Biochem. J. 437, 373–379. doi: 10.1042/BJ20110213
Igamberdiev, A. U., and Lea, P. J. (2002). The role of peroxisomes in the integration of metabolism and evolutionary diversity of photosynthetic organisms. Phytochemistry 60, 651–674. doi: 10.1016/S0031-9422(02)00179-6
Igamberdiev, A. U., and Lea, P. J. (2006). Land plants equilibrate O2 and CO2 concentrations in the atmosphere. Photosynth. Res. 87, 177–194. doi: 10.1007/s11120-005-8388-2
Igamberdiev, A. U., Lernmark, U., and Gardeström, P. (2014b). Activity of the mitochondrial pyruvate dehydrogenase complex in plants is stimulated in the presence of malate. Mitochondrion 19B, 184–190. doi: 10.1016/j.mito.2014.04.006
Igamberdiev, A. U., Popov, V. N., and Falaleeva, M. I. (1995). Alternative system of succinate oxidation in glyoxysomes of higher plants. FEBS Lett. 367, 287–290. doi: 10.1016/0014-5793(95)00563-O
Igamberdiev, A. U., Romanowska, E., and Gardeström, P. (2001b). Photorespiratory flux and mitochondrial contribution to energy and redox balance of barley leaf protoplasts in the light and during light-dark transitions. J. Plant Physiol. 158, 1325–1332. doi: 10.1078/0176-1617-00551
Igamberdiev, A. U., Zemlyanukhin, A. A., and Meshcheryakova, I. V. (1986). Extraglyoxysomal form of isocitrate lyase in higher plants. Sov. Plant Physiol. 33, 852–858.
Igamberdiev, A. U., Zemlyanukhin, A. A., and Rodionova, L. G. (1988). Glycolate oxidase from wheat and sugarbeet leaves – catalytic properties and role in oxalate biosynthesis. Biochemistry (Moscow) 53, 1499–1505.
Ivanov, B. F., Igamberdiev, A. U., and Zemlyanukhin, A. A. (1989). Non-photosynthetic utilization of exogenous 14C oxalate by pea and rice seedlings exposed in the helium and carbon-dioxide media. Fiziologiya i Biokhimiya Kulturnykh Rastenii 21, 329–333.
Ivanov, B. F., and Zemlyanukhin, A. A. (1991). Composition of the gaseous environment of plants and pathways of utilization of exogenous malate. Sov. Plant Physiol. 38, 553–561.
Jena, B. S., Jayaprakasha, G. K., Singh, R. P., and Sakariah, K. K. (2002). Chemistry and biochemistry of (-)-hydroxycitric acid from Garcinia. J. Agric. Food Chem. 50, 10–22. doi: 10.1021/jf010753k
Jones, D. L. (1998). Organic acids in the rhizosphere – a critical review. Plant Soil 205, 25–44. doi: 10.1023/A:1004356007312
Kanamori, M., and Wixom, R. L. (1963). Studies in valine biosynthesis. V. Characteristics of the purified dihydroxyacid dehydratase from spinach leaves. J. Biol. Chem. 238, 998–1005.
Katsuhara, M., Sakano, K., Sato, M., Kawakita, H., and Kawabe, S. (1993). Distribution and production of trans-aconitic acid in barnyard grass (Echinochloa crus-galli var. oryzicola) as putative antifeedant against brown planthoppers. Plant Cell Physiol. 34, 251–254.
Kent, S. S. (1972). Photosynthesis in the higher plant Vicia faba. II. The non-Calvin cycle origin of acetate and its metabolic relationship to the photosynthetic origin of formate. J. Biol. Chem. 247, 7293–7302.
Kim, Y. S. (2002). Malonate metabolism: biochemistry, molecular biology, physiology, and industrial application. J. Biochem. Mol. Biol. 35, 443–451. doi: 10.5483/BMBRep.2002.35.5.443
Kleczkowski, L. A., Randall, D. D., and Edwards, G. E. (1991). Oxalate as a potent and selective inhibitor of spinach (Spinacia oleracea) leaf NADPH-dependent hydroxypyruvate reductase. Biochem. J. 276, 125–127. doi: 10.1042/bj2760125
Kobes, R. D., and Dekker, E. E. (1966). Beef liver 2-keto-4-hydroxyglutarate aldolase: substrate stereospecificity and Schiff base formation with pyruvate and glyoxylate. Biochem. Biophys. Res. Commun. 25, 329–334. doi: 10.1016/0006-291X(66)90781-9
Kolesnikov, P. A. (1948). On the oxidation of glycolic acids in green cells. Doklady Akademii Nauk SSSR 60, 1205–1208.
Koyama, H., Kawamura, A., Kihara, T., Hara, T., Takita, E., and Shibata, D. (2000). Overexpression of mitochondrial citrate synthase in Arabidopsis thaliana improved growth on a phosphorus-limited soil. Plant Cell Physiol. 41, 1030–1037. doi: 10.1093/pcp/pcd029
Koyama, H., Takita, E., Kawamura, A., Hara, T., and Shibata, D. (1999). Overexpression of mitochondrial citrate synthase gene improves the growth of carrot cells on Al-phosphate medium. Plant Cell Physiol. 40, 482–488. doi: 10.1093/oxfordjournals.pcp.a029568
Krogh, A. (1971). The content of trans-aconitic acid in Asarum europaeum L. determined by means of a chromatogram spectrophotometer. Acta Chem. Scand. 25, 1495–1496. doi: 10.3891/acta.chem.scand.25-1495
Krömer, S., and Scheibe, R. (1996). Function of the chloroplastic malate valve for respiration during photosynthesis. Biochem. Soc. Trans. 24, 761–766. doi: 10.1042/bst0240761
Lane, B. G., Dunwell, J. M., Ray, J. A., Schmitt, M. R., and Cuming, A. C. (1993). Germin, a protein marker of early plant development, is an oxalate oxidase. J. Biol. Chem. 268, 12239–12242.
Lenton, T. M., Crouch, M., Johnson, M., Pires, N., and Dolan, L. (2012). First plants cooled the Ordovician. Nat. Geosci. 5, 86–89. doi: 10.1038/ngeo1390
Lewis, Y. S., and Neelakantan, S. (1965). (-)-Hydroxycitric acid-the principal acid in the fruits of Garcinia cambogia Desr. Phytochemistry 4, 619–625. doi: 10.1016/S0031-9422(00)86224-X
Lippman, E. O. (1883). Über eine neue im Rubensaff vorkommende Saure. Ber. Deut. Chem. 16:1078. doi: 10.1002/cber.188301601242
Lü, J., Gao, X., Dong, Z., Yi, J., and An, L. (2012). Improved phosphorus acquisition by tobacco through transgenic expression of mitochondrial malate dehydrogenase from Penicillium oxalicum. Plant Cell Rep. 31, 49–56. doi: 10.1007/s00299-011-1138-3
Lüttge, U. (1988). Day-night changes of citric acid levels in Crassulacean acid metabolism – phenomenon and ecophysiological significance. Plant Cell Environ. 11, 445–451. doi: 10.1111/j.1365-3040.1988.tb01782.x
Ma, Z., Marsolais, F., Bernards, M. A., Sumarah, M. W., Bykova, N. V., and Igamberdiev, A. U. (2016). Glyoxylate cycle and metabolism of organic acids in the scutellum of barley seeds during germination. Plant Sci. 248, 37–44. doi: 10.1016/j.plantsci.2016.04.007
MacLennan, D. H., and Beevers, H. (1964). Trans-aconitate in plant tissues. Phytochemistry 3, 109–113. doi: 10.1016/S0031-9422(00)84002-9
Massonneau, A., Langlade, N., Léon, S., Smutny, J., Vogt, E., Neumann, G., et al. (2001). Metabolic changes associated with cluster root development in white lupin (Lupinus albus L.): relationship between organic acid excretion, sucrose metabolism and energy status. Planta 213, 534–542. doi: 10.1007/s004250100529
Maurino, V. G., and Engqvist, M. K. (2015). 2-Hydroxy acids in plant metabolism. Arabidopsis Book 13:e0182. doi: 10.1199/tab.0182
McIntosh, C. A., and Oliver, D. J. (1992). Isolation and characterization of the tricarboxylate transporter from pea mitochondria. Plant Physiol. 100, 2030–2034. doi: 10.1104/pp.100.4.2030
Menegus, F., Cattaruzza, L., Chersi, A., and Fronza, G. (1989). Differences in the anaerobic lactate-succinate production and in the changes of cell sap pH for plants with high and low resistance to anoxia. Plant Physiol. 90, 29–32. doi: 10.1104/pp.90.1.29
Meyer, S., De Angeli, A., Fernie, A. R., and Martinoia, E. (2010a). Intra- and extra-cellular excretion of carboxylates. Trends Plant Sci. 15, 40–47. doi: 10.1016/j.tplants.2009.10.002
Meyer, S., Mumm, P., Imes, D., Endler, A., Weder, B., Al-Rasheid, K. A., et al. (2010b). AtALMT12 represents an R-type anion channel required for stomatal movement in Arabidopsis guard cells. Plant J. 63, 1054–1062. doi: 10.1111/j.1365-313X.2010.04302.x
Meyer, S., Scholz-Starke, J., de Angeli, A., Kovermann, P., Burla, B., Gambale, F., et al. (2011). Malate transport by the vacuolar AtALMT6 channel in guard cells is subject to multiple regulation. Plant J. 67, 247–257. doi: 10.1111/j.1365-313X.2011.04587.x
Millerd, A., Morton, R. K., and Wells, J. R. E. (1962). Role of isocitrate lyase in synthesis of oxalic acid in plants. Nature 196, 955–956. doi: 10.1038/196955a0
Millerd, A., Morton, R. K., and Wells, J. R. E. (1963). Enzymatic synthesis of oxalic acid in Oxalis pes-caprae. Biochem. J. 88, 281–287. doi: 10.1042/bj0880281
Miszalski, Z., Kornas, A., Rozpądek, P., Fischer-Schliebs, E., and Lüttge, U. (2013). Independent fluctuations of malate and citrate in the CAM species Clusia hilariana Schltdl. under low light and high light in relation to photoprotection. J. Plant Physiol. 170, 453–458. doi: 10.1016/j.jplph.2012.11.005
Miyagi, A., Uchimiya, M., Kawai-Yamada, M., and Uchimiya, H. (2013). An antagonist treatment in combination with tracer experiments revealed isocitrate pathway dominant to oxalate biosynthesis in Rumex obtusifolius L. Metabolomics 9, 590–598. doi: 10.1007/s11306-012-0486-5
Morton, R. K., and Wells, J. R. E. (1964). Isocitrate lyase and the formation of α-keto-γ-hydroxyglutaric acid in oxalis. Nature 201, 477–479. doi: 10.1038/201477a0
Narsai, R., Howell, K. A., Carroll, A., Ivanova, A., Millar, A. H., and Whelan, J. (2009). Defining core metabolic and transcriptomic responses to oxygen availability in rice embryos and young seedlings. Plant Physiol. 151, 306–322. doi: 10.1104/pp.109.142026
Nikolaeva, M. I., and Makeev, A. M. (1984). Effects of Uromyces LK fungi on changing biochemical composition of the diseased leguminous plants. Mikol. Fitopatol. 18, 420–423.
Oliver, J. D., Kaye, S. J., Tuckwell, D., Johns, A. E., Macdonald, D. A., Livermore, J., et al. (2012). The Aspergillus fumigatus dihydroxyacid dehydratase Ilv3A/IlvC is required for full virulence. PLoS ONE 7:e43559. doi: 10.1371/journal.pone.0043559
Osmond, C. B., and Avadhani, P. N. (1968). Acid metabolism in Atriplex. II. Oxalate synthesis during acid metabolism in the dark. Austr. J. Biol. Sci. 21, 917–927. doi: 10.1071/BI9680917
Otten, J. G., and Mehltretter, C. L. (1970). Separation of cis-aconitic acids and trans-aconitic acids by thin-layer chromatography. Anal. Biochem. 35, 302–303. doi: 10.1016/0003-2697(70)90041-2
Payes, B., and Laties, G. G. (1963). The inhibition of several tricarboxylic acid cycle enzymes by γ-hydroxy-α-ketoglutarate. Biochem. Biophys. Res. Commun. 10, 460–466. doi: 10.1016/0006-291X(63)90380-2
Pirrung, M. C., Ha, H.-J., and Holmes, C. P. (1989). Purification and inhibition of spinach α, β-dihydroxyacid dehydratase. J. Org. Chem. 54, 1543–1548. doi: 10.1021/jo00268a014
Plaut, G. W., Beach, R. L., and Aogaichi, T. (1975). Substrate activity of structural analogs of isocitrate for isocitrate dehydrogenases from bovine heart. Biochemistry 14, 2581–2588. doi: 10.1021/bi00683a004
Pracharoenwattana, I., Zhou, W., Keech, O., Francisco, P. B., Udomchalothorn, T., Tschoep, H., et al. (2010). Arabidopsis has a cytosolic fumarase required for the massive allocation of photosynthate into fumaric acid and for rapid plant growth on high nitrogen. Plant J. 62, 785–795. doi: 10.1111/j.1365-313X.2010.04189.x
Pucher, G. W., Vickery, H. B., Abrahams, M. D., and Leavenworth, C. S. (1949). Studies in the metabolism of crassulacean plants: diurnal variation of organic acids and starch in excised leaves of Bryophyllum calycinum. Plant Physiol. 24, 610–620. doi: 10.1104/pp.24.4.610
Ramaswamy, N. K., and Nair, P. M. (1984). Evidence for the operation of a C1 pathway for the fixation of CO2 in isolated intact chloroplasts from green potato tubers. Plant Sci. Lett. 34, 261–267. doi: 10.1016/S0304-4211(84)80005-X
Randall, D. D. (1981). Subcellular location of NADP-isocitrate dehydrogenase in Pisum sativum leaves. Plant Physiol. 68, 70–73. doi: 10.1104/pp.68.1.70
Rao, W. R. R., and Altekar, W. R. (1961). Aconitate isomerase. Biochem. Biophys. Res. Commun. 44, 101–105. doi: 10.1016/0006-291X(61)90355-2
Raven, J. A., and Edwards, D. (2001). Roots: evolutionary origins and biogeochemical significance. J. Exp. Bot. 52, 381–401. doi: 10.1093/jexbot/52.suppl_1.381
Richardson, K. E., and Tolbert, N. E. (1961). Oxidation of glyoxylic acid to oxalic acid by glycolic acid oxidase. J. Biol. Chem. 236, 1280–1284.
Ruffo, A., Testa, E., Adinolfi, A., Pelizza, G., and Moratti, R. (1967). Control of the citric acid cycle by glyoxylate. Mechanism of the inhibition by oxalomalate and γ-hydroxy-α-oxoglutarate. Biochem. J. 103, 19–23. doi: 10.1042/bj1030019
Russell, J. B., and Van Soest, P. J. (1984). In vitro ruminal fermentation of organic acids common in forage. Appl. Environ. Microbiol. 47, 155–159.
Schneider, K., Skovran, E., and Vorholt, J. A. (2012). Oxalyl-coenzyme A reduction to glyoxylate is the preferred route of oxalate assimilation in Methylobacterium extorquens AM1. J. Bacteriol. 194, 3144–3155. doi: 10.1128/JB.00288-12
Scholtz, J. M., and Schuster, S. M. (1984). Substrates of hydroxyketoglutarate aldolase. Bioorg. Chem. 12, 229–234. doi: 10.1016/0045-2068(84)90006-3
Shannon, L. M., de Vellis, J., and Lew, J. Y. (1963). Malonic acid biosynthesis in bush bean roots. II. Purification and properties of enzyme catalyzing oxidative decarboxylation of oxaloacetate. Plant Physiol. 38, 691–697. doi: 10.1104/pp.38.6.691
Shchiparev, S. M., Chuprova, G. V., and Polevoi, V. V. (1976). Secretion of acids by isolated scuttella of corn. Vestn. Leningr. Univ. Biol. 21, 130–133.
Shchiparev, S. M., and Soldatenkov, S. V. (1972). Study of the biosynthesis and metabolism of hydroxycitric acid in the leaves of ambari (Hibiscus cannabinus vulgaris). Sov. Plant Physiol. 19, 1261–1265.
Siener, R., Hönow, R., Voss, S., Seidler, A., and Hesse, A. (2006). Oxalate content of cereals and cereal products. J. Agric. Food. Chem. 54, 3008–3011. doi: 10.1021/jf052776v
Silva, I. R., Smyth, T. J., Raper, C. D., Carter, T. E., and Rufty, T. W. (2001). Differential aluminum tolerance in soybean: an evaluation of the role of organic acids. Physiol. Plant. 112, 200–210. doi: 10.1034/j.1399-3054.2001.1120208.x
Stallings, W. C., Blount, J. F., Srere, P. A., and Glusker, J. P. (1979). Structural studies of hydroxycitrates and their relevance to certain enzymatic mechanisms. Arch. Biochem. Biophys. 193, 431–448. doi: 10.1016/0003-9861(79)90050-X
Stout, P. R., Brownell, J., and Burau, R. G. (1967). Occurrences of trans-aconitate in range forage species. Agronomy J. 59, 21–24. doi: 10.2134/agronj1967.00021962005900010006x
Stumpf, D. K., and Burris, R. H. (1981). Biosynthesis of malonate in roots of soybean seedlings. Plant Physiol. 68, 992–995. doi: 10.1104/pp.68.5.992
Sweetlove, L. J., Beard, K. F., Nunes-Nesi, A., Fernie, A. R., and Ratcliffe, R. G. (2010). Not just a circle: flux modes in the plant TCA cycle. Trends Plant Sci. 15, 462–470. doi: 10.1016/j.tplants.2010.05.006
Tcherkez, G., Mahé, A., Gauthier, P., Mauve, C., Gout, E., Bligny, R., et al. (2009). In folio respiratory fluxomics revealed by 13C isotopic labeling and H/D isotope effects highlight the noncyclic nature of the tricarboxylic acid “cycle” in illuminated leaves. Plant Physiol. 151, 620–630. doi: 10.1104/pp.109.142976
Thompson, J. F., Schaefer, S. C., and Madison, J. T. (1997). Role of aconitate isomerase in trans-aconitate accumulation in plants. J. Agric. Food Chem. 45, 3684–3688. doi: 10.1021/jf970131s
Tian, H., Jiang, L., Liu, E., Zhang, J., Liu, F., and Peng, X. (2008). Dependence of nitrate-induced oxalate accumulation on nitrate reduction in rice leaves. Physiol. Plant. 133, 180–189. doi: 10.1111/j.1399-3054.2008.01052.x
Tkacheva, T. I., and Zemlyanukhin, L. A. (1993). Some properties of aconitate isomerase from corn, its intracellular location, and changes of activity during germination of seeds. Russ. J. Plant Physiol. 40, 73–76.
Tolbert, N. E., Benker, C., and Beck, E. (1995). The oxygen and carbon dioxide compensation points of C3 plants: possible role in regulating atmospheric oxygen. Proc. Natl. Acad. Sci. U.S.A. 92, 11230–11233. doi: 10.1073/pnas.92.24.11230
Tolbert, N. E., Clagett, C. O., and Burris, R. H. (1949). Products of the oxidation of glycolic acid and L-lactic acid by enzymes from tobacco leaves. J. Biol. Chem. 181, 905–914.
Tronconi, M. A., Wheeler, M. C., Martinatto, A., Zubimendi, J. P., Andreo, C. S., and Drincovich, M. F. (2015). Allosteric substrate inhibition of Arabidopsis NAD-dependent malic enzyme 1 is released by fumarate. Phytochemistry 111, 37–47. doi: 10.1016/j.phytochem.2014.11.009
Vanlerberghe, G. C., and McIntosh, L. (1996). Signals regulating the expression of the nuclear gene encoding alternative oxidase of plant mitochondria. Plant Physiol. 111, 589–595.
Vitale, A., and Denecke, J. (1999). The endoplasmic reticulum – gateway of the secretory pathway. Plant Cell 11, 615–628. doi: 10.2307/3870888
Wienkoop, S., Ullrich, W. R., and Stöhr, C. (1999). Kinetic characterization of succinate-dependent plasma membrane-bound nitrate reductase in tobacco roots. Physiol. Plant. 105, 609–614. doi: 10.1034/j.1399-3054.1999.105403.x
Wingler, A., Lea, P. J., and Leegood, R. C. (1999). Photorespiratory metabolism of glyoxylate and formate in glycine-accumulating mutants of barley and Amaranthus edulis. Planta 207, 518–526. doi: 10.1007/s004250050512
Xu, H. W., Ji, X. M., He, Z. H., Shi, W. P., Zhu, G. H., Niu, J. K., et al. (2006). Oxalate accumulation and regulation is independent of glycolate oxidase in rice leaves. J. Exp. Bot. 57, 1899–1908. doi: 10.1093/jxb/erj131
Yu, L., Jiang, J. Z., Zhang, C., Jiang, L. R., Ye, N. H., Lu, Y. S., et al. (2010). Glyoxylate rather than ascorbate is an efficient precursor for oxalate biosynthesis in rice. J. Exp. Bot. 61, 1625–1634. doi: 10.1093/jxb/erq028
Zeiher, C. A., and Randall, D. D. (1990). Identification and characterization of mitochondrial acetyl-coenzyme A hydrolase from Pisum sativum L. seedlings. Plant Physiol. 94, 20–27. doi: 10.1104/pp.94.1.20
Zemlyanukhin, A. A., Makeev, A. M., and Raikhinshtein, M. V. (1972). Metabolism of formic acid and related compounds in maize seedlings. Sov. Plant Physiol. 19, 473–480.
Zemlyanukhin, L. A., and Eprintsev, A. T. (1979). Induction of aconitate isomerase in higher plants. Doklady Akademii Nauk SSSR 246, 1020–1024.
Zemlyanukhin, L. A., and Zemlyanukhin, A. A. (1996). Metabolism of hydroxycitric acid in microorganisms and higher plants. Russ. J. Plant Physiol. 43, 108–110.
Zemlyanukhina, O. A., Filatov, G. P., Zemlyanukhin, L. A., and Tkacheva, T. I. (1986). Purification and properties of extramitochondrial acetyl-CoA hydrolase from barley seedlings. Doklady Akademii Nauk SSSR 291, 1250–1253.
Keywords: aconitate, citrate, fumarate, malate, organic acids, oxalate, redox level, tricarboxylic acid cycle
Citation: Igamberdiev AU and Eprintsev AT (2016) Organic Acids: The Pools of Fixed Carbon Involved in Redox Regulation and Energy Balance in Higher Plants. Front. Plant Sci. 7:1042. doi: 10.3389/fpls.2016.01042
Received: 20 April 2016; Accepted: 04 July 2016;
Published: 15 July 2016.
Edited by:
Veronica Graciela Maurino, Heinrich-Heine-Universitat Düsseldorf, GermanyReviewed by:
Enrico Martinoia, Universitat Zürich, SwitzerlandM. Teresa Sanchez-Ballesta, Instituto de Ciencia y Tecnología de Alimentos y Nutrición -Consejo Superior de Investigaciones Científicas, Spain
Copyright © 2016 Igamberdiev and Eprintsev. This is an open-access article distributed under the terms of the Creative Commons Attribution License (CC BY). The use, distribution or reproduction in other forums is permitted, provided the original author(s) or licensor are credited and that the original publication in this journal is cited, in accordance with accepted academic practice. No use, distribution or reproduction is permitted which does not comply with these terms.
*Correspondence: Abir U. Igamberdiev, aWdhbWJlcmRpZXZAbXVuLmNh