- 1Grupo Interações Planta-Ambiente and Biodiversidade (PlantStress&Biodiversity), Departamento Recursos Naturais, Ambiente e Território (DRAT), Linking Landscape, Environment, Agriculture and Food (LEAF), and Forest Research Center (CEF), Instituto Superior de Agronomia, Universidade de Lisboa, Oeiras, Portugal
- 2Departamento Ciências Agrárias e Biológicas, Centro Universitário Norte do Espírito Santo, Universidade Federal Espírito Santo, São Mateus, Brazil
- 3Setor Fisiologia Vegetal, Centro de Ciências e Tecnologias Agropecuárias, Universidade Estadual do Norte Fluminense, Rio de Janeiro, Brazil
- 4GeoBioTec, Faculdade Ciências Tecnologia, Universidade NOVA de Lisboa, Caparica, Portugal
- 5Unidade de Investigação em Biotecnologia e Recursos Genéticos, Instituto Nacional de Investigação Agrária e Veterinária, Oeiras, Portugal
- 6Departamento Produção Vegetal, Centro de Ciências Agrárias, Universidade Federal do Espírito Santo, Alegre, Brazil
- 7Departamento Biologia Vegetal, Universidade Federal de Viçosa, Viçosa, Brazil
Modeling studies have predicted that coffee crop will be endangered by future global warming, but recent reports highlighted that high [CO2] can mitigate heat impacts on coffee. This work aimed at identifying heat protective mechanisms promoted by CO2 in Coffea arabica (cv. Icatu and IPR108) and Coffea canephora cv. Conilon CL153. Plants were grown at 25/20°C (day/night), under 380 or 700 μL CO2 L−1, and then gradually submitted to 31/25, 37/30, and 42/34°C. Relevant heat tolerance up to 37/30°C for both [CO2] and all coffee genotypes was observed, likely supported by the maintenance or increase of the pools of several protective molecules (neoxanthin, lutein, carotenes, α-tocopherol, HSP70, raffinose), activities of antioxidant enzymes, such as superoxide dismutase (SOD), ascorbate peroxidase (APX), glutathione reductase (GR), catalase (CAT), and the upregulated expression of some genes (ELIP, Chaperonin 20). However, at 42/34°C a tolerance threshold was reached, mostly in the 380-plants and Icatu. Adjustments in raffinose, lutein, β-carotene, α-tocopherol and HSP70 pools, and the upregulated expression of genes related to protective (ELIPS, HSP70, Chape 20, and 60) and antioxidant (CAT, CuSOD2, APX Cyt, APX Chl) proteins were largely driven by temperature. However, enhanced [CO2] maintained higher activities of GR (Icatu) and CAT (Icatu and IPR108), kept (or even increased) the Cu,Zn-SOD, APX, and CAT activities, and promoted a greater upregulation of those enzyme genes, as well as those related to HSP70, ELIPs, Chaperonins in CL153, and Icatu. These changes likely favored the maintenance of reactive oxygen species (ROS) at controlled levels and contributed to mitigate of photosystem II photoinhibition at the highest temperature. Overall, our results highlighted the important role of enhanced [CO2] on the coffee crop acclimation and sustainability under predicted future global warming scenarios.
Introduction
Anthropogenic actions, mostly related to rising use of fossil fuels and land-use changes, have strongly contributed to the increase of atmospheric [CO2] from ca. 280 in the pre-industrial period to the actual 400 μL CO2 L−1. According to recent projections, depending on the greenhouse gas emission scenarios air [CO2] could rise to between 421 and 936 μL CO2 L−1, (or between 475 and 1313 μL CO2 L−1, considering CO2-equivalent concentrations that include the predicted concentrations of CH4 and N2O) by 2100. These will be accompanied by a global surface warming between 0.3 and 1.7°C (best scenario) and 2.6–4.8°C (worst scenario, without additional mitigation efforts), relative to 1986–2005 (IPCC, 2013, 2014).
This [CO2] boost can affect fundamental plant processes such as photosynthesis and respiration (Woodward, 2002; Ainsworth and Rogers, 2007; Kirschbaum, 2011), ultimately altering plant growth, yield, and crop quality (Luo et al., 1999; DaMatta et al., 2010). The actual atmospheric [CO2] is below the optimal for photosynthesis of C3 crops. Therefore, enhanced [CO2] is expected to increase net photosynthesis near or often above 50%, with trees showing the largest rise (Ainsworth and Rogers, 2007). However, projected global warming, concurrent to [CO2] increase may compromise the beneficial effects of C fertilization, and affect flower viability, fruit development, and yield (Prasad et al., 2003; Camargo, 2010), therefore constituting a major threat for many important agricultural crops. Indeed, heat modifies the use of solar energy, alters both the gas diffusion in the leaf mesophyll (Lambers et al., 2008) and photosynthetic pigment composition and content (Haldimann and Feller, 2004; Hasanuzzaman et al., 2013). Elevated temperatures also affect the water relations and evaporative demand, the fluidity and stability of membrane systems, as well as hormones and primary and secondary metabolites (Wahid et al., 2007). Collectively, these alterations could potentially disrupt cellular homeostasis and impair photosynthesis and other major physiological processes (Long et al., 2006; Suzuki and Mittler, 2006; Kirschbaum, 2011). In any case, elevated [CO2] can modify plant responses to environmental variables (Boisvenue and Running, 2006). Therefore, to properly predict plant responses to rising temperatures and atmospheric [CO2], studies must be undertaken considering both variables on a long-term basis, given that their interaction can either exacerbate or cancel their single independent effects on leaf physiology (Way et al., 2015).
The harmful effects of heat stress is frequently linked to the enhanced accumulation of reactive oxygen species (ROS) (Suzuki and Mittler, 2006; Hasanuzzaman et al., 2013). The upregulation of mechanisms controlling the production/scavenging of highly reactive molecules is determinant to cell homeostasis and to plant survival to environmental stresses. Chloroplast (and cell) oxidative stress usually arises under conditions that reduce energy use by photochemical processes without a significant reduction in energy capture. This imbalance promotes the over-production of molecules in the excited state, both of Chl and O2 [e.g., triplet state of chl (3Chl*), 1chl, singlet oxygen (1O2)]. Furthermore, O2 reduction in photosystems (particularly in PSI) may occur, producing and, thereafter, H2O2 and OH·, all of which can cause lipid peroxidation, bleaching of pigments (e.g., in P680), protein oxidation (e.g., D1), enzyme inactivation, and DNA degradation (Asada, 1994; Foyer, 2002; Logan, 2005). The control of such highly reactive molecules can be achieved indirectly, through the increase of energy dissipation mechanisms (e.g., photorespiration, pseudocyclic electron transport, and the synthesis of photoprotective pigments; Logan, 2005; Smirnoff, 2005; Batista-Santos et al., 2011), or directly, acting on their production/scavenging. The latter includes the complementary overexpression of enzymes (e.g., SOD, APX, GR), as well as the action of hydrophilic (ascorbate and glutathione) and lipophilic (e.g., zeaxanthin, β-carotene, and α-tocopherol) antioxidants (Foyer, 2002; Logan, 2005; Munné-Bosch, 2005; Smirnoff, 2005).
Coffee, an evergreen tropical tree species, is one of the most heavily globally traded commodities. The world coffee production is based on Coffea arabica L. and C. canephora Pierre ex A. Froehner, which produce ~2/3 and 1/3 of crop yield, respectively. The optimal annual average temperature is one of the well-known differences between C. arabica (18–23°C) and C. canephora (22–26°C) (DaMatta and Ramalho, 2006). Coffee crop chain of value generates an income of approximately US$ 170,000 million and involves ca. 100 million people worldwide, having a strong social and economic impact on many tropical countries (Bunn et al., 2015). Noticeably, the coffee production is mostly based on small holders that are currently facing growing challenges from climate changes; under these circumstances, both the coffee yields and beverage quality can be profoundly impacted, thus potentially affecting not only producers but also the coffee industry and consumers as a whole. In fact, over the last decade, several modeling studies anticipated remarkable climate impacts on the coffee crop (especially C. arabica), with large worldwide losses on suitable areas and productivity, although coffee production is believed to have already been affected by climate changes due to the occurrence of severe droughts and high temperatures (Bunn et al., 2015; Craparo et al., 2015; van der Vossen et al., 2015). However, neither the positive effects of elevated [CO2] on coffee photosynthesis (Ramalho et al., 2013b; DaMatta et al., 2016), leaf mineral balance (Martins et al., 2014), and bean yield (Ghini et al., 2015), nor the role of CO2 as a key player in coffee heat tolerance (Rodrigues et al., 2016), have been considered in those modeling studies, probably because this information has become available only very recently.
We previously demonstrated that both C. arabica and C. canephora species are remarkably heat tolerant up to 37/30°C, but at 42/34°C a threshold for irreversible non-stomatal deleterious effects on photosynthesis is reached, with greater impairments on C. arabica, particularly under normal air [CO2] (Rodrigues et al., 2016). Photosystems and thylakoid electron transport were shown to be quite heat tolerant, contrasting to the enzymes related to energy metabolism, including RuBisCO, which were the most sensitive components. We also demonstrated that elevated [CO2] does not provoke photosynthetic downregulation and remarkably mitigates the impact of temperature on both species, particularly at 42/34°C, modifying the response to supra-optimal temperatures. Here we complement our previous studies with the central objective of exploring potential protective mechanisms against heat stress, and how these mechanisms are affected by the elevated [CO2] in coffee. To reach these goals, we firstly assessed the occurrence of PSII photoinhibition (as a marker for photodamage), followed by an in-depth characterization of protective mechanisms, including dynamics of photosynthetic pigments, enzymatic and non-enzymatic antioxidant systems, quantification of raffinose family oligosaccharides (RFOs), and heat-shock protein pools, in addition to assessing the expression of selected genes with potential roles in heat acclimation.
Materials and Methods
Plant Material and Experimental Conditions
The plant materials were collected from the same experiments described in Rodrigues et al. (2016), therefore, using the same experimental conditions and treatments. In brief, three widely cropped coffee genotypes from the two main producing species, C. arabica L. (cvs. Icatu and IPR108) and C. canephora Pierre ex A. Froehner (cv. Conilon Clone 153—CL153), were evaluated. Plants, ca. 1.5 years of age, were transferred into two walk-in growth chambers (EHHF 10000, ARALAB, Portugal) differing in air [CO2] supply: 380 μL CO2 L−1 (380-plants) or 700 μL CO2 L−1 (700-plants). Both groups of plants were then grown for 10 months in 28 L pots in a substrate consisting of a mixture of soil, peat, and sand (3:1:3, v/v/v), as optimized for coffee plants (Ramalho et al., 2013a), and fertilized as previously described (Ramalho et al., 2013b). Environmental conditions of temperature (25/20°C, day/night), irradiance (ca. 700–800 μmol m−2 s−1), RH (75%), and photoperiod (12 h) were provided to the plants, and permanently monitored along the whole period. The temperature was then gradually increased from 25/20°C up to 42/34°C in both growth chambers at a rate of 0.5°C day−1, with 7 days of stabilization at 31/25, 37/30, and 42/34°C for evaluations.
All determinations were performed on newly matured leaves from the upper (illuminated) part of each plant. For biochemical evaluations, leaf material was collected after ca. 2 h of illumination from 6 to 8 plants of each genotype and used immediately or flash frozen in liquid N2 and stored at −80°C until analysis. Each biological replicate is a pool of leaves of each plant. Along the experiment the plants were maintained without restrictions in water, nutrients, and root development, the latter evaluated by visual examination at the end of the experiment after removing the plants from their pots (Rodrigues et al., 2016).
PSII Photoinhibition Status
The PSII photoinhibition indexes were calculated according to Werner et al. (2002), and included: (A) chronic photoinhibition (PIChr), representing the percent reduction in Fv/Fm at each temperature relative to the maximal Fv/Fm obtained during the entire experiment; (B) dynamic photoinhibition (PIDyn), representing the decline in Fv/Fm that is fully reversible overnight, being measured as the percent reduction in midday / relative to Fv/Fm at each temperature, relative to the maximal Fv/Fm from the entire experiment; (C) total photoinhibition (PITotal = PIChr + PIDyn). The Fv/Fm and / represented the maximal photochemical efficiency of PSII and the actual PSII efficiency of energy conversion under light exposure, respectively. Fv/Fm and / were obtained under dark adapted or photosynthetic steady-state conditions, respectively, exactly as detailed in Rodrigues et al. (2016).
Photosynthetic Pigment Characterization
Carotenoids (Car) were assessed from four frozen leaf discs (each 0.5 cm2). Sample processing and subsequent reverse-phase HPLC were carried out as optimized for coffee (Ramalho et al., 1997) with minor adjustments, using an end-capped (C18) 5-μm Spherisorb ODS-2 column (250 × 4.6 mm). Detection was performed at 440 nm in an HPLC system (Beckman, System Gold, Tulsa, USA) coupled to a diode-array (Model 168; Beckman) detector, and identification and quantification were performed using individual sugar standards. The de-epoxidation state, involving xanthophyll cycle components, was calculated as DEPS = (Zeaxanthin (Z) + 0.5 Antheraxanthin (A)) / (Violaxanthin(V) + A + Z).
Chlorophylls (Chls) from the same samples were extracted in 80% acetone, and quantified spectrophotometrically according to Lichtenthaler (1987).
Maximal Activities of Antioxidant Enzymes
Unless otherwise indicated, enzyme activities were determined in chloroplast extracts (obtained using 3–4 g FW of leaf tissue), as described previously (Ramalho et al., 1998). Superoxide dismutase (Cu,Zn-SOD, EC 1.15.1.1) was spectrophotometrically assessed at 550 nm. Ascorbate peroxidase (APX, EC 1.11.1.11) was assessed through ascorbate consumption (at 290 nm, 120 s, 25°C) using an extinction coefficient of 2.8 mM−1 cm−1 for calculations. Glutathione reductase (GR, EC 1.6.4.2) was assessed through the NADPH oxidation (at 340 nm, 120 s, 25°C). Catalase (CAT, EC 1.11.1.6) was assessed in whole-leaf extracts (prepared using 200 mg FW of leaf tissue), as described in Fortunato et al. (2010). Enzyme activity was evaluated through the rate of H2O2 consumption (240 nm, 120 s, 25°C), and a freshly made H2O2 standard curve (0–1 M) was used for quantification.
Quantification of Non-Enzymatic Antioxidants
Ascorbate (ASC) and α-tocopherol (TOC) determinations were performed on 100 and 200 mg FW of leaf tissue, respectively, based on HPLC methods, as described in detail in Fortunato et al. (2010).
HSP70 Quantification
For the heat shock protein 70 (HSP70) assays, 100 mg FW of frozen leaf tissues per plant were homogenized in 1 mL 200 mM Tris-HCl (pH 8.0), containing 20 mM β-mercaptoethanol, 2 mM dithiothreitol (DTT), 2% triton X-100, 4% (v/v) “Complete-protease inhibitor cocktail” with EDTA, 10% polyvinylpolypyrrolidone, and 10% glycerol. The homogenate was then centrifuged (10,000 g, 20 min, 4°C) and the supernatant was used for HSP70 quantification through Enzyme Linked Immunosorbent Assay (ELISA), using Flat-bottomed micro-ELISA plates (Costar, Corning, NY, USA) as described in Njemini et al. (2003) with minor modifications. Plates were covered with the primary antibody (100 μL; 5 μg mL−1) diluted in 100 mM carbonate buffer (pH 9.6). After incubating the plates at 37°C during 90 min, the coated plates were washed four times with phosphate-buffered saline (PBS) containing 0.1% Tween 20 (PBS/T) and non-specific binding sites blocked by incubation with 300 μL of PBS/T containing 0.1% BSA (PBS/T/BSA) for 60 min at 37°C. After washing, 50 μL of the standard and samples were added and the plates incubated for 90 min at 37°C. Plates were then washed four times and 100 μL of mouse monoclonal anti-HSP70 (1/400) diluted in PBS/T were added. After 60 min on a shaker at 37°C, plates were washed four times and incubated with 100 μL of the diluted (1/10,000 in PBS/T/BSA) secondary antibody (anti-mouse IgG, peroxidase, Sigma-Aldrich, USA) for 60 min at 37°C. Thereafter, plates were washed and 100 μL of ABTS (2,2′-Azino-bis-3-ethylbenzothiazoline-6-sulfonic acid) substrate were added. After 45 min at 37°C, the reaction was stopped with 50 μL of 1 N H2SO4 and the absorbance was determined at 405 nm using a microplate reader. HSP70 concentrations were detected by comparing sample absorbance with the absorbance of a reference purified HSP70 protein.
Total soluble protein contents of the enzyme extracts followed Bradford (1976), using bovine serum albumin (BSA) as a standard.
Raffinose Family Oligosaccharides Quantification
RFOs evaluation was performed in samples of 150 mg of powdered frozen material, following the HPLC method for soluble sugars described in Ramalho et al. (2013b), with some modifications. To overcome the presence of non-pure peaks the separation of sugars was performed using a Sugar-Pak 1 column (300 × 6.5 mm, Waters) at 90°C, with H2O as the eluent (containing 50 mg EDTA-Ca L−1 H2O) and a flow rate of 0.5 mL min−1. Another 20 μL aliquot of each sample was injected through a DionexCarboPac PA1 analytical column (4 × 250 mm, Thermo Scientific, USA) coupled to a DionexCarboPac PA1 Guard (4 × 50 mm) at 20°C. Ultrapure water and 300 mM NaOH were used as eluents (water from 0 to 50 min; NaOH from 50 to 65 min; and water from 65 to 80 min for re-equilibration) at a 1 mL min−1 flow rate. A refractive index detector (Model 2414, Waters, USA) was used for sugar detection. Sugars were quantified using specific standard curves.
Expression Studies of Selected Genes
Total RNA was isolated and quantified as described in Goulao et al. (2012). One microgram of DNA-free total RNA was used to synthesize first-strand cDNAs using oligo-(dT)18 primers and the SuperScript II first-strand synthesis system (Invitrogen, USA).
Genes related to the antioxidative system and other protection proteins were selected for the expression studies (Tables 1, 6), with all procedures performed as described in Goulao et al. (2012). Primers were designed using the C. canephora sequences (http://www.coffee-genome.org/coffeacanephora) with Primer3 software. Primer length was set to 19–20 bp, with a GC content between 45 and 60% and a melting temperature (Tm value) between 62 and 65°C. Amplicon length ranges were set to be between 80 and 150 bp. The probability of formation of hairpin structures and primer dimerization was subsequently checked using the Oligo Calculator algorithm. To determine the specificity of the primer pairs used in this study, melting/dissociation curve analysis was performed following the RT-qPCR experiment. A single peak in the obtained melting curve confirmed the specificity of the amplicon, and no signal was detected in the negative controls for all of the tested primers. All qRT-PCR reactions and relative gene expression were calculated after normalization with the reference genes ubiquitin-conjugating enzyme E2 (UbQ2) and eukaryotic initiation factor 4α(eLF-4).
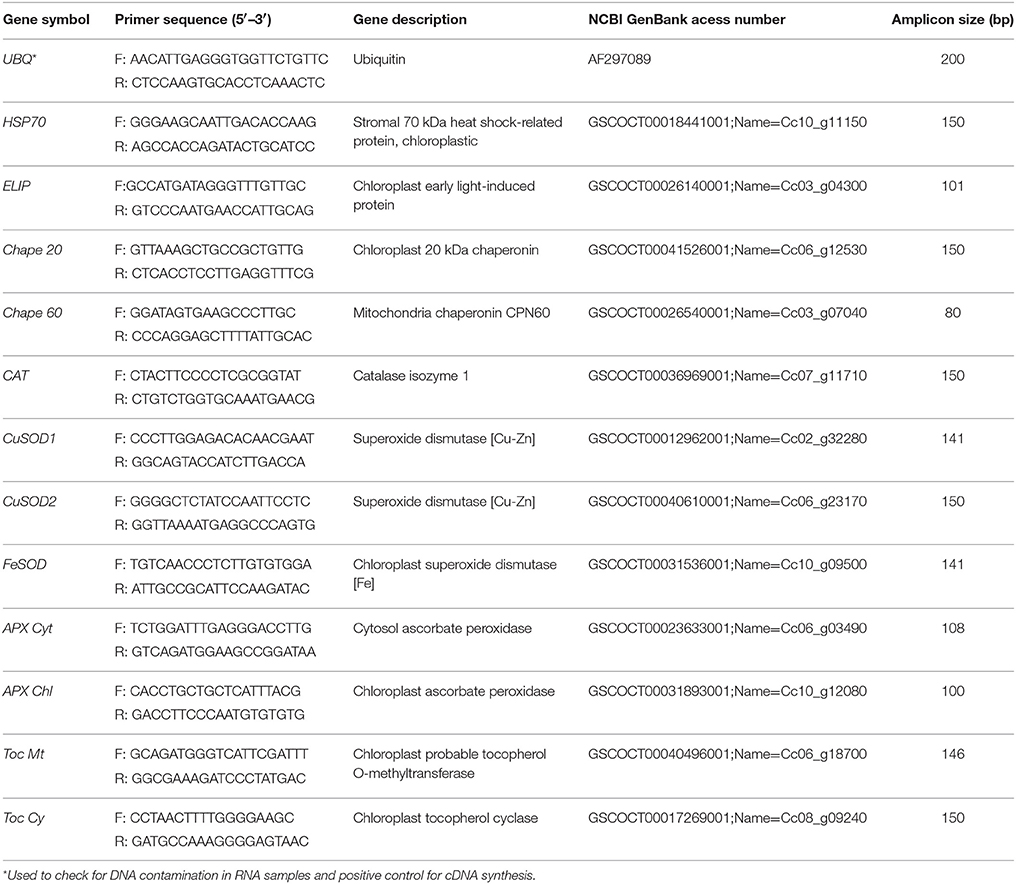
Table 1. Selected genes used for real-time qPCR studies, which are related to the oxidative stress control and/or repair mechanisms, homologies, primer sequences, access number on NCBI GenBank and amplicon size (bp).
Statistical Analysis
Data were analyzed using two-way ANOVAs (P < 0.05) to evaluate the differences between the two growth atmospheric [CO2] and among the several temperature treatments, as well as their interaction, followed by a Tukey's test for mean comparisons, except when otherwise stated. Each ANOVA was performed independently for each of the studied genotypes. Overall, the [CO2] × temperature interaction for most parameters was significant. To the sake of simplicity we do not considered also the comparison between genotypes within each [CO2] and temperature.
The relative expression ratio of each target gene was computed based on its real-time PCR efficiency and the crossing point (CP) difference of a target sample vs. a control (25/20°C, 380 μL CO2 L−1 air) within each genotype. Data analysis was performed using the Relative Expression Software Tool (REST 2009), available at http://www.genequantification.de/rest-2009.html. A 95% confidence level was adopted for all tests.
Results
PSII Photoinhibition
Overall, dynamic PSII photoinhibition (PIDyn) was mostly unresponsive to temperature and [CO2] (Figure 1A). Even so, CL153 380-plants maintained higher PIDyn values than those of under 700 μL CO2 L−1 throughout the experiment. In contrast, chronic PSII photoinhibition (PIChr) was manifested almost exclusively at 42/34°C (Figure 1B) and in the 380-plants, particularly in Icatu. Reflecting PIDyn and PIChr variations at 42/34°C, the total photoinhibition (PITotal) showed significantly higher values in the 380-plants of all genotypes (maximal in Icatu), as well as in the 700-plants of C. arabica genotypes (Figure 1C).
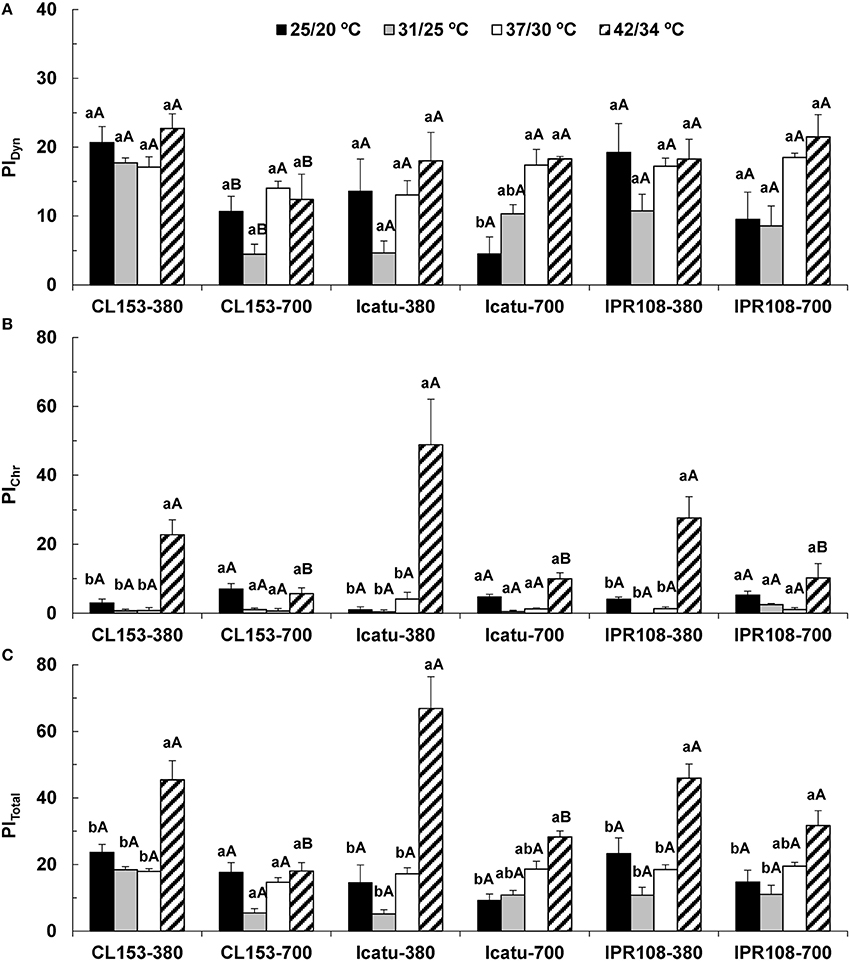
Figure 1. Leaf fluorescence parameters related to the photoinhibition status of PSII in C. canephora cv. Conilon (CL153) and C. arabica (Icatu and IPR108) plants, which were grown under 380 or 700 μL CO2 L−1 and submitted to control (25/20°C, day/night) and supra-optimal temperatures of 31/25°C, 37/30°C, or 42/34°C. The parameters include the calculation of (A) PIDyn, dynamic photoinhibition, (B) PIChr, chronic photoinhibition, and (C) PITotal, total photoinhibition. For each parameter, the mean values ± SE (n = 5–8) followed by different letters express significant differences between temperature treatments for the same CO2 level, separately for each genotype (a, b), or between CO2 levels for each temperature treatment, separately for each genotype (A, B).
Photosynthetic Pigment
At control temperature (25/20°C), enhanced [CO2] promoted a consistent tendency toward higher contents of several carotenoids (Car) in CL153 (Table 2), although significantly only for neoxanthin and carotenes. No changes were observed in total Chl contents (Table 3). The C. arabica plants showed a somewhat different pattern by the maintenance or even slight lower Car contents under high [CO2], namely in the pool of xanthophyll cycle pigments (VAZ), carotenes, and total Car.
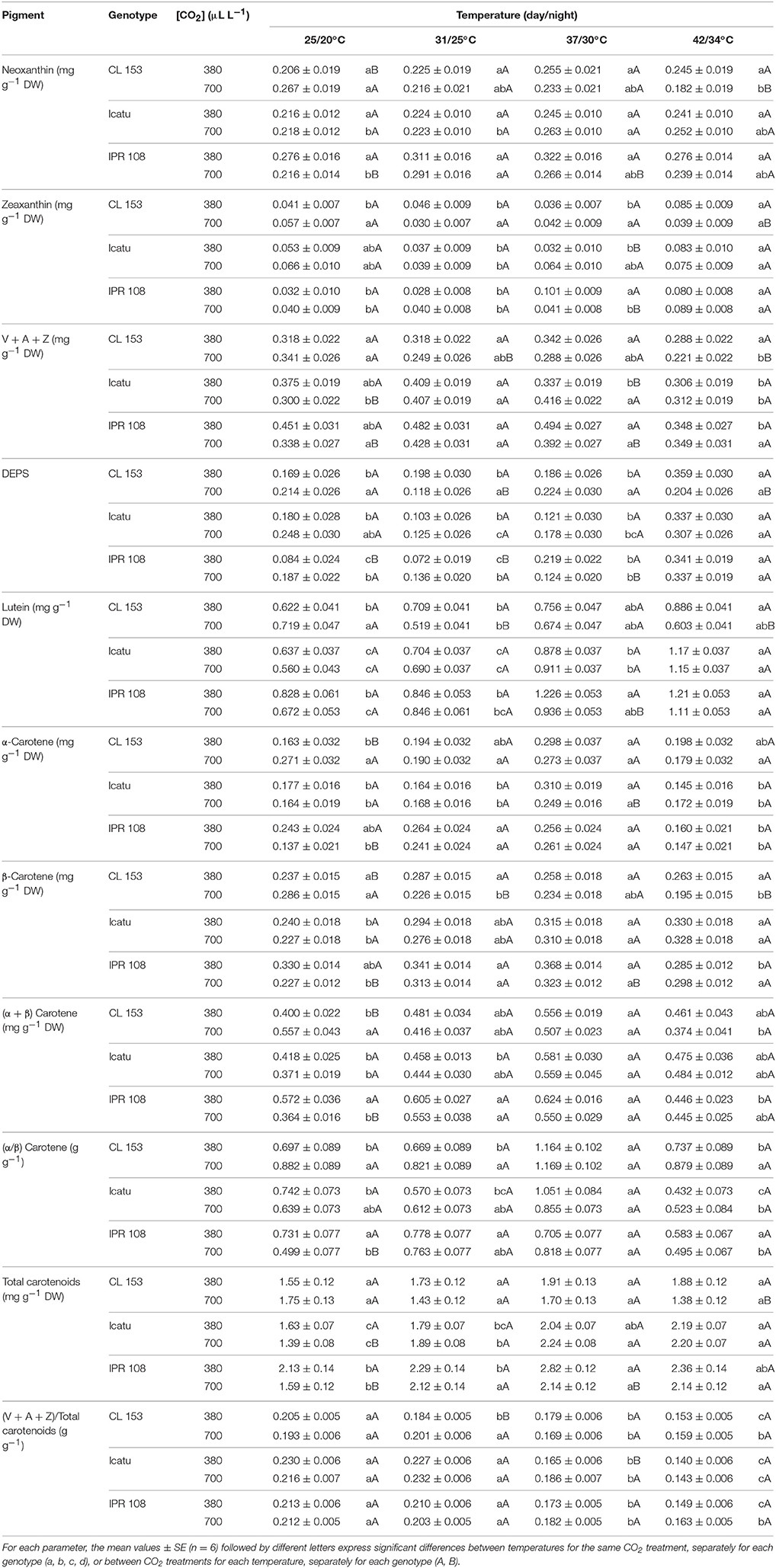
Table 2. Changes in leaf content (mg g−1 DW) of xanthophylls and carotenes, obtained through HPLC determinations in C. canephora cv. Conilon (CL153) and C. arabica (Icatu and IPR108) plants, grown under 380 or 700 μLCO2 L−1, at control (25/20°C, day/night) and supra-optimal temperatures of 31/25°C, 37/30°C, and 42/34°C.
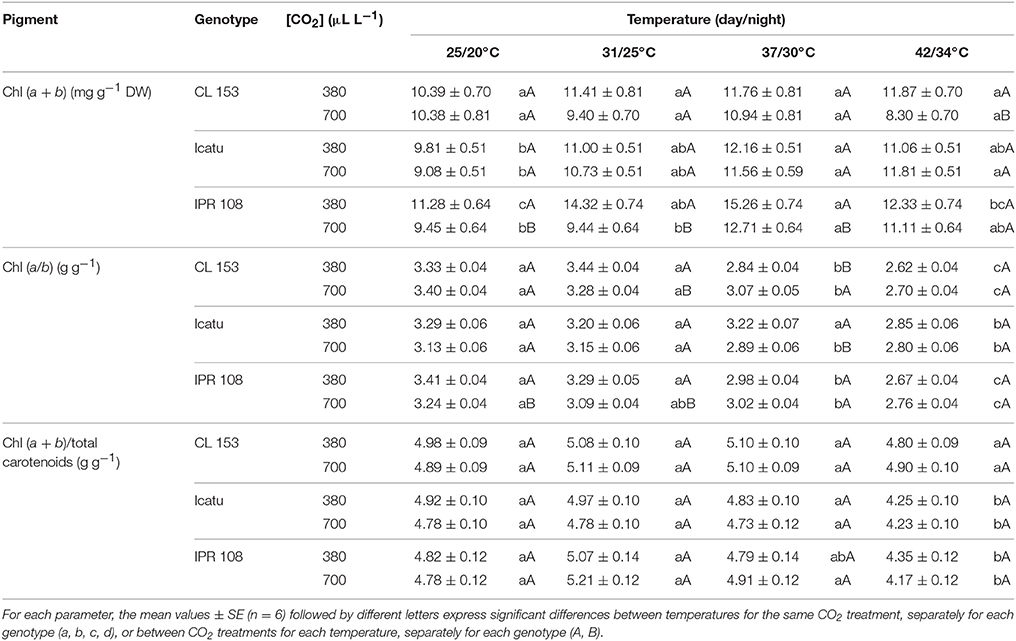
Table 3. Changes in leaf content of total chlorophyll, Chl (a + b) (mg g−1 DW), and in the ratios of a-to-b chlorophyll and of total chlorophyll-to-total carotenoids, obtained through spectrophotometric determinations, in C. canephora cv. Conilon (CL153) and C. arabica (Icatu and IPR108) plants, grown under 380 or 700 μLCO2 L−1, at control (25/20°C, day/night) and supra-optimal temperatures of 31/25°C, 37/30°C, and 42/34°C.
In C. canephora plants exposed to supra-optimal temperatures, decreases in most pigments (neoxanthin, VAZ pool, lutein, β-, and total carotenes) were observed in the 700-plants. Given that the 380-plants maintained or slightly increased (lutein, carotenes) their pigment contents (when compared to 25/20°C), differences between CO2 conditions emerged for most Car, significantly for neoxanthin, VAZ pool, lutein, and total Car under 42/34°C.
In C. arabica genotypes, [CO2] treatment caused marginal/occasional changes in pigment composition at high temperatures. Such changes were mostly restricted to 700-plants of IPR108, which at 37/30°C showed lower contents of neoxanthin, VAZ pool, DEPS, lutein, β- and total carotenes, total Car, and Chl (a + b) relative to their 380-counterparts. However, no significant differences associated with [CO2] treatments could be found at 42/34°C. In contrast to [CO2] treatment, increasing temperature remarkably impacted the C. arabica genotypes regardless of [CO2], as denoted by the increases in total Chls and total Car, reaching maximal values at 37/30°C or 42/34°C. The rise in total Car was related to increases in neoxanthin, Z and, especially, lutein and β-carotene (Table 2). In fact, at 42/34°C, rises in lutein pools reached 84 and 105% in Icatu, and 47 and 65% in IPR108, for the 380- and 700-plants, respectively, whereas the β-carotene pools increased ca. 40% in Icatu under both [CO2] conditions. In IPR108, maximal increases in β-carotene were found at 37/30°C, although with significance only for the 700-plants (42%).
Specifically regarding the photoprotective Z, moderately low values were maintained over the course of the experiment, but at 42/34°C significant Z increases (in parallel with moderate increases in DEPS) were observed in Icatu and IPR108 regardless of [CO2], as well as in CL153 380-plants. Furthermore, at 42/34°C most pigments (with the referred exceptions related to lutein and β-carotene) tended to lower concentrations than those observed at 37/30°C.
A moderate rise in total Chl was observed in C. arabica genotypes for both CO2 treatments, mostly until 37/30°C, as compared to 25/20°C values. In CL153 temperature rising did not provoke significantl changes in total Chl content within each [CO2]. However, at 42/34°C the CL153 700-plants displayed lower Chl content than 380-plants, resulting from slight divergent shifts promoted by temperature in these plant groups (Table 3). The values of Chl (a/b) ratio were similar between [CO2], but decreased at the two highest temperatures in all genotypes. Finally, the total Chl-to-total Car ratio, reflecting the light capture-to-dissipation pigment ratio, was quite stable until 37/30°C, but strongly decreased at 42/34°C in the C. arabica genotypes. No differences were found in this ratio in response to [CO2] over the entire experiment in all genotypes.
Antioxidant Enzyme Activities
The activities of the antioxidant enzymes (Table 4) showed some differences at control temperature among genotypes in response to [CO2], although the values were maintained within the range usually observed for genotypes of both C. arabica and C. canephora (Ramalho et al., 1998; Chaves et al., 2008; Fortunato et al., 2010; Pompelli et al., 2010). For all enzymes (Cu,Zn-SOD, APX, GR, CAT), IPR108 700-plants showed lower activities than 380-plants. In Icatu and CL153 that same trend was observed for GR and CAT, contrasting to higher activities observed in the 700-plants for APX and Cu,Zn-SOD (significantly only for the latter).
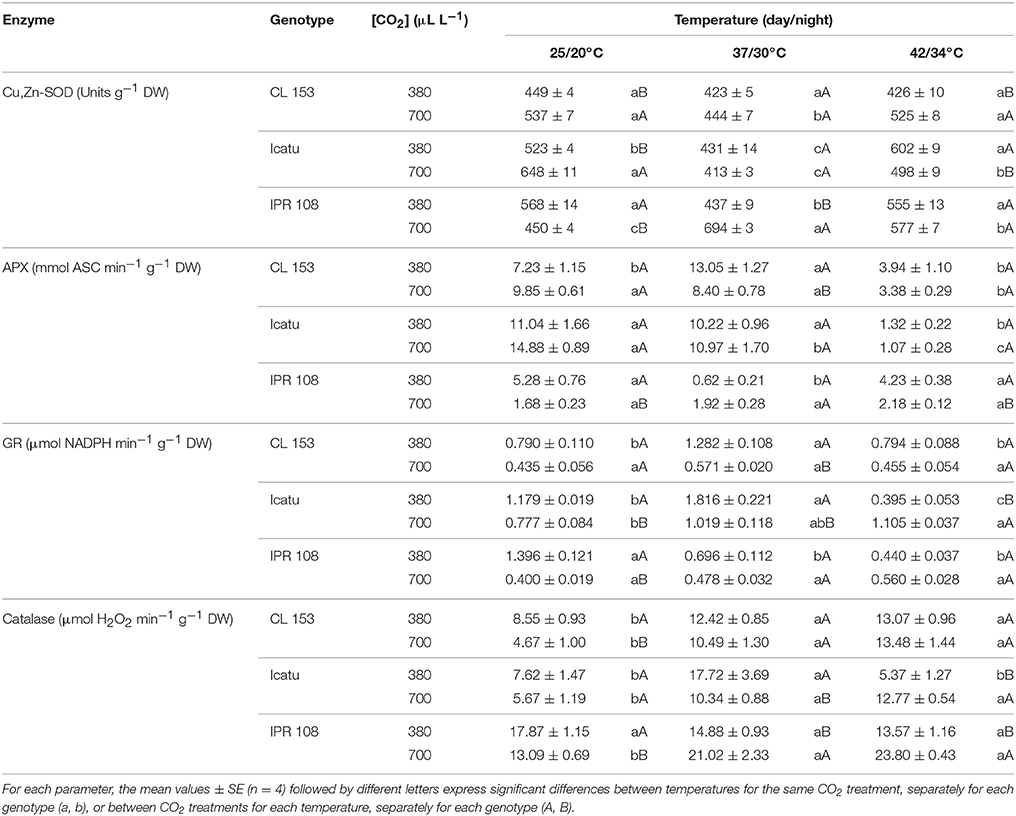
Table 4. Changes in chloroplastic maximal activities of the enzyme antioxidants Cu,Zn-superoxide dismutase (Cu,Zn-SOD), ascorbate peroxidase (APX), glutathione reductase (GR), as well as in cellular catalase in C. canephora cv. Conilon (CL153) and C. arabica (Icatu and IPR108) plants, grown under 380 or 700 μLCO2 L−1, at control (25/20°C, day/night) and maximal temperature exposure (42/34°C).
With temperature rise to 37/30°C, enzyme activities changed differently with respect to genotype and [CO2] conditions (Table 4). In CL153 380-plants, increases were found in APX, GR and CAT, whereas in the 700-plants only CAT activity was increased. In Icatu, increases in GR and CAT were observed under both CO2 conditions, whereas some decrease was found in Cu,Zn-SOD and APX (the latter only in the 700-plants). In IPR108, different patterns were observed related to [CO2], with activity increases in 700-plants (significantly for Cu,Zn-SOD and CAT), and decreases in 380-plants (non-significantly for CAT) in all enzymes.
Pronounced changes in enzyme activities were further noted when temperature increased from 37/30 to 42/34°C. In CL153 plants, regardless of [CO2], Cu,Zn-SOD and GR activities remained invariant in parallel with an enhanced CAT activity (53% for 380 plants and 189% for 700-plants), whereas APX activity was remarkably reduced (46 and 66% in 380- and 700-plants, respectively). Within C. arabica genotypes, Icatu displayed reductions in APX (near to 10% of the initial value, irrespective of [CO2]), GR and CAT (380-plants) and Cu,Zn-SOD (700-plants), in parallel with increases in both GR and CAT (700-plants) as well as in Cu,Zn-SOD (380-plants). In IPR108, the 700-plants showed increased activities of Cu,Zn-SOD (28%), APX (30%), GR (40%), and CAT, but only the activity of CAT varied significantly in response to [CO2] treatments.
In summary, APX was the most negatively affected enzyme at the highest temperature (in CL153 and Icatu), CAT activity increased or remained invariant, whereas Cu,Zn-SOD was the less responsive enzyme to heat stress.
Other Protective Molecules
Ascorbate (ASC) content was similar between CO2 conditions within each genotype under control temperature. However, C. arabica genotypes showed ca. three-fold higher ASC contents than those in CL153 (Table 5). Overall, ASC pools were clearly reduced with increasing temperatures, beginning at 31/25°C for CL153 and IPR108 700-plants, and for Icatu 380-plants. Remarkable decreases were observed at the two highest temperatures, ranging from 69 (IPR108-700) to 86% (CL153-380), as compared with the control temperature.
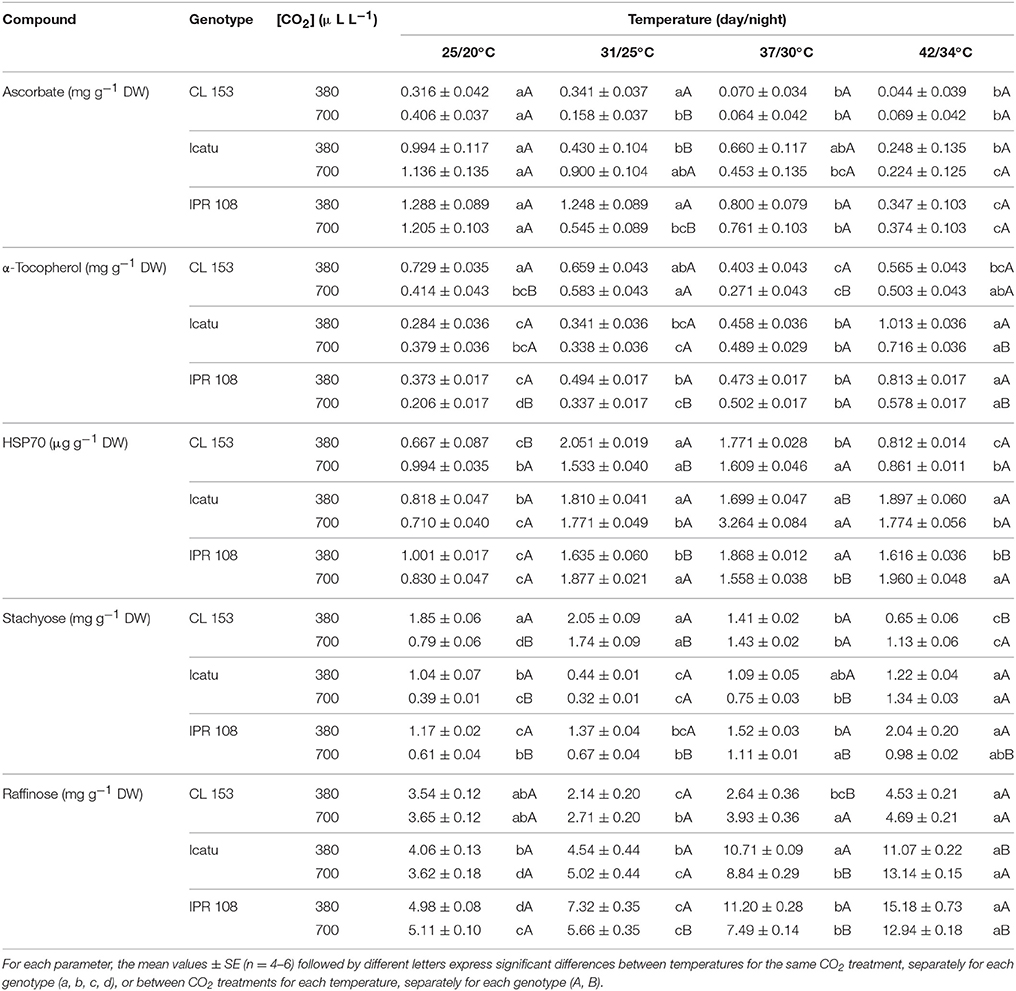
Table 5. Changes in the cellular content of the non-enzyme antioxidant molecules ascorbate and α-tocopherol, as well as HSP70 and the soluble sugars stachyose and, raffinose in C. canephora cv. Conilon (CL153) and C. arabica (Icatu and IPR108) plants, grown under 380 or 700 μLCO2 L−1, at control (25/20°C, day/night) and supra-optimal temperatures of 31/25°C, 37/30°C, and 42/34°C.
α-tocopherol (TOC) showed different trends between the two coffee species. Despite some fluctuations with rising temperatures, at 42/34°C TOC pools changed moderately in CL153 irrespective of [CO2], with a 22% decrease in 380-plants and a 21% rise in 700-plants. In contrast, at 42/34°C and under both CO2 conditions, TOC pools increased in Icatu and IPR108, ranging from 89 (Icatu-700) to 257% (Icatu-380), with 380-plants presenting the highest contents.
At 25/20°C the 700-plants showed similar (raffinose) or lower (stachyose) contents of RFOs than their 380-plant counterparts. Temperature rise prompted modifications in RFOs, with no clear relation with the [CO2] treatments. In CL153, significant increases were observed only for stachyose in the 700-plants from 31/25°C onwards. On the other hand, at 42/34°C, both stachyose and raffinose displayed greater contents in C. arabica plants, when compared to 25/20°C, particularly raffinose whose contents increased between 153% (IPR108-700) and 263% (Icatu-700). Also, in IPR108, both RFOs content were always higher in the 380-plants at supra-optimal temperatures.
The constitutive cell HSP70 pools (25/20°C) were similar between [CO2] in C. arabica genotypes, but presented a higher value in CL153 700-plants. Rising temperature clearly promoted HSP70 accumulation: significantly higher HSP70 contents were already observed at 31/25°C in all genotypes, which were mostly maintained until 42/34°C (C. arabica plants) or until 37/30°C in CL153 (decreasing afterwards). In all genotypes, maximal HSP70 contents, relative to control temperature, were observed in CL153-380 at 31/25°C (208%), and in the 700-plants of Icatu at 37/30°C (360%) and IPR108 at 42/34°C (136%).
Expression of Genes with a Potential Role in Heat Acclimation
Several changes in gene expression patterns were imposed by enhanced [CO2] and temperature (Table 6). At 25/20°C, the expression of genes coding for the protective proteins HSP70, ELIP, Chape 20 and Chape 60 was similar or lower in the 700-plants compared with their 380-plant counterparts in all genotypes. With rising temperature a global gene upregulation was observed (maximal at 42/34°C) in all genotypes and both [CO2], although stronger in the 700-plants of Icatu and CL153 and 380-plants of IPR. Notably, CL153 700-plants showed the highest increases: ca. 33- (Chape 60), 55- (ELIP), 59- (HSP70), and 145- (Chape 20) fold.
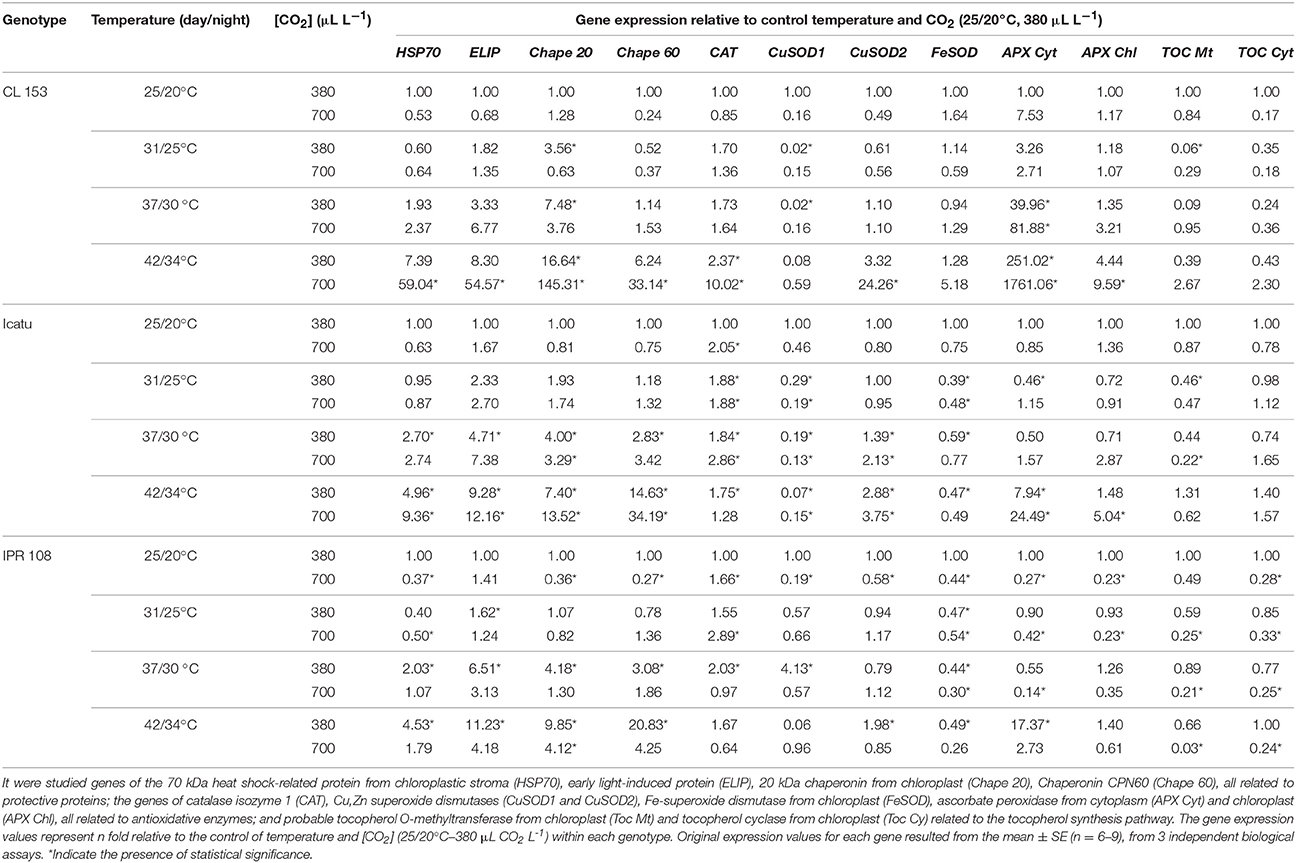
Table 6. Real-time PCR expression studies relative to the expression value observed under control conditions of temperature and CO2 (25/20°C, 380 μL CO2 L−1), within each genotype from leaves of C. canephora cv. Conilon (CL153) and C. arabica (Icatu and IPR108) plants, grown under 380 or 700 μLCO2 L−1, at control (25/20°C, day/night) and supra-optimal temperatures of 31/25°C, 37/30°C, and 42/34°C.
Overall, the expression patterns of genes related to antioxidative system components (CAT, SOD, APX, and TOC) tended to be lower in the 700-plants than in the 380-plants at the control temperature, mainly in the two C. arabica genotypes (except for CAT). Under rising temperatures CAT expression increased moderately (below three-fold) in most cases, and more markedly in CL153 700-plants (10-fold). The APX transcripts (particularly APX Cyt) increased with heat, with maximal abundance at 42/34°C, especially in Icatu and CL153 700-plants. In fact, the APX Cyt upregulation in CL153-700 (1760-fold) was, by far, the largest observed over the entire experiment for all studied genes. In contrast, CuSOD1 and FeSOD genes were mainly downregulated by temperature in all genotypes. Among the genes coding for SOD enzymes, only CuSOD2 was significantly upregulated at the two highest temperatures in both [CO2] in Icatu, and at 42/34°C in CL153-700 and IPR-380 plants. TOC genes were mainly downregulated (except CL153 700-plants). Notably, IPR108 had the strongest global downregulation for the genes related to the antioxidative system, with the exception of APX Cyt at 42/34°C in 380-plants.
Discussion
PSII Photoinhibition in Face of High CO2 and Temperature
The balance of energy use and/or dissipation capabilities, herein analyzed using the dynamic (PIDyn) and chronic (PIChr) PSII photoinhibition estimates (Figures 1A,B), remained nearly invariant until 37/30°C, a temperature that is considered well above the optimum for both C. arabica and C. canephora (DaMatta and Ramalho, 2006). These results evidence a considerable heat tolerance of the coffee's photosynthetic machinery until 37/30°C, which is accompanied by the maintenance of photochemical energy use (Rodrigues et al., 2016). In fact, photochemical components and RuBisCO activity were strengthened (especially under high [CO2]), in contrast to what is often observed in other species even at moderately high temperature (e.g., Haldimann and Feller, 2004). In sharp contrast, increases in both PIChr (and PITotal) were evident at 42/34°C (Figures 1B,C) in 380-plants, particularly in Icatu. This reflected a mitigatory impact of elevated [CO2] on the photochemical functioning, in line with the lower rate constant for PSII inactivation (Fs/) and non-photochemical quenching attributable to photo-inactivation and non-regulated energy dissipation in PSII (Y(NO)) observed in 700 plants at 42/34°C (Rodrigues et al., 2016). Taking all of this information together, it is clear that tolerance to heat stress was compromised at 42/34°C at normal [CO2], whereas at elevated [CO2] a mitigating effect against heat impacts was evident in this study. Similar findings have been reported in other species, where elevated [CO2] has been shown to preserve the photosynthetic functioning, as in the Mediterranean cork oak (Quercus suber) under the exposure to supra-optimal temperatures (Faria et al., 1996).
Triggering Heat-Protective Mechanisms under Enhanced [CO2]
Thermotolerance frequently involves the triggering of cellular mechanisms that prevent oxidative damage, interlinking temperature stress, and a balance between ROS signaling and control (Suzuki and Mittler, 2006; Hasanuzzaman et al., 2013). In addition, the strengthening of antioxidative mechanisms has proven to be decisive to stress acclimation in Coffea spp., namely, to cold, high irradiance, drought and nitrogen starvation (Ramalho et al., 1998, 2014; Fortunato et al., 2010; Batista-Santos et al., 2011; Cavatte et al., 2012). Therefore, under enhanced [CO2], robust thermal protective mechanisms are expected, supporting an unaltered photosynthetic functioning up to 37/30°C in addition to maintaining a better metabolic performance even at 42/34°C, when compared to normal [CO2], as reported previously (Rodrigues et al., 2016).
Pigment Dynamics
Carotenoids act as thermal quenchers and scavengers of highly reactive species of Chl and O2. Under control temperature, the high [CO2] prompted a tendency to higher carotenoid contents in CL153. This contrasted with a reduction trend in photoprotective carotenoids (VAZ pool, lutein, carotenes) in C. arabica genotypes, similarly to what has been found in other species, where a relaxation of the antioxidant system was associated with a higher C-assimilation that ultimately led to a reduced energy load on the photosynthetic apparatus (Erice et al., 2007).
Under supra-optimal temperatures, decreases of most carotenoids were found in the 700-plants of CL153 (and IPR108 until 37/30°C), when compared to their respective 380-plants. In contrast, C. arabica genotypes showed increased total Chl and total Car contents regardless of [CO2], suggesting a photosynthetic structures strengthening at 37/30°C (and even at 42/34°C in some cases) driven by temperature. Increases in total Car resulted mostly from the moderate Z increases and the strong rises in lutein and β-carotene that have complementary actions, decreasing the formation of highly reactive species of Chl and oxygen, preventing PSs and membrane photodamage, and protecting the Cyt b6/f complex from photobleaching promoted by 1O2 (Zhang et al., 1999). Z protects LHCs from both PSs by controlling 3Chl* and 1Chl* formation and by removing epoxy groups from the oxidized double bonds from the lipid phase of thylakoid fatty acids, whereas β-carotene and lutein-epoxide cycle (and neoxanthin) quenches 3Chl* and 1O2, which are lipoperoxidation initiators (Lichtenthaler and Babani, 2004; Logan, 2005; Kalituho et al., 2007; Matsubara et al., 2011). In addition, neoxanthin maintenance and lutein increase might improve antennae functionality given that these xanthophylls are integral components of the peripheral LHC and are important for its trimeric assembly, stability, and efficiency (Kalituho et al., 2007; Matsubara et al., 2011). These functions agree with the maintenance of PSI and PSII activities in C. arabica genotypes until 37/30°C (Rodrigues et al., 2016). Nevertheless, at 42/34°C PSII photoinhibition was evident in 380-plants (Figure 1), when pigments (except lutein) tended to lower values (Table 2) when compared with those observed at 37/30°C. Therefore, changes in photoprotective pigments were not strong enough to protect the PSs in the three genotypes, especially in Icatu, which was the most affected genotype by heat stress. However, the lower photoinhibition status in the 700-plants at 42/34°C (Figure 1) was not accompanied by clear increases in the pools of photoprotective pigments, suggesting that these molecules are not the only ones accounting for the better preservation of photosynthetic activity under heat stress at elevated [CO2].
Chl-to-Car ratio was quite stable along the experiment in CL153, and until 37/30°C in C. arabica plants (Table 3). This pattern was independent from [CO2], as also observed in other woody species (Bader et al., 2010). Chl (a/b) ratio showed similar values between [CO2], but decreased in all genotypes at the two highest temperatures, especially at 42/34°C. Since net degradation of Chls was apparently absent, this Chl (a/b) reduction reflected preferential Chl b synthesis, suggesting functional readjustments with a higher proportion of light harvesting chlorophyll-protein complex from PSII (LHCII), which contains the majority of Chl b (with a Chl a/b ratio around 1.1–1.3) (Lichtenthaler and Babani, 2004). Nonetheless, it should not be ruled out that decreases in Chl (a/b) might reflect some heat susceptibility given that this ratio has been shown to increase in heat-tolerant genotypes of other species (Bita and Gerats, 2013).
Antioxidative Enzymes
The ascorbate-glutathione cycle is a vital mechanism to control cell oxidative stress, and include both enzyme (e.g., Cu,Zn-SOD, APX, GR, among others) and non-enzyme (ASC, GSH) components, which altogether scavenge several ROS (, H2O2, OH·) (Asada, 1994; Foyer, 2002; Smirnoff, 2005). With the exception of Cu,Zn-SOD in CL153 and Icatu, 700-plants showed a somewhat downregulation of the antioxidative enzymatic system at control temperature. Such lower constitutive level of antioxidative capability under high [CO2] has been interpreted in other species as compromising the plant's ability to cope with sudden stress events (Pritchard et al., 2000). However, in coffee plants, this may be associated with higher photosynthetic rates (Rodrigues et al., 2016), which concomitantly with the inhibition of photorespiration under elevated CO2 (DaMatta et al., 2016), is believed to decrease the excitation pressure in the PSs and avoid ROS formation, thus ultimately precluding the need of developing a robust antioxidant system. In fact, although elevated CO2 may lead to decreases in SOD, APX, GR, and CAT activities (Pritchard et al., 2000; Erice et al., 2007; Vurro et al., 2009), even under supra-optimal temperatures (Erice et al., 2007; AbdElgawad et al., 2015), a concomitant reduction in the lipoperoxidation status has been often observed. Taken together, this information supports the notion that reduced ROS production under high CO2 conditions in C3 plants is indeed related to a higher photosynthetic functioning and inhibition of photorespiration (Erice et al., 2007; Vurro et al., 2009; AbdElgawad et al., 2015), as would also be the case in coffee plants at control temperature.
With rising temperatures thermotolerance can be improved by increasing transcript and protein levels of ROS-scavenging enzymes (Suzuki and Mittler, 2006; Hasanuzzaman et al., 2013), which agrees with the increases in APX, GR, and CAT in CL153-380, GR and CAT in Icatu (both CO2 conditions), and Cu,Zn-SOD and CAT in IPR108 700-plants. In addition, in line with the observed heat tolerance until 37/30°C the 380-plants from Icatu presented similar (Cu,Zn-SOD and APX) or higher (GR and CAT) activities than their 700-plants counterparts, compensating for the lower photosynthetic rates. Similarly, the IPR108 380-plants presented higher (Cu,Zn-SOD and CAT) or similar (GR and APX) enzyme activities than their respective 700-plants. Irrespective of genotypes and [CO2], FeSOD gene was not significantly upregulated under supra-optimal temperatures, and therefore removal through extra chloroplast SOD action is not expected to be reinforced.
The additional temperature increase to 42/34°C led to further changes in enzyme activities with varying genotypic patterns. In CL153 plants only APX activity was reduced (despite the APX Chl upregulation), but the H2O2 control might have been achieved in extra-chloroplast compartments in good agreement with both increases in CAT activity in both [CO2] and upregulation of CAT and APX Cyt genes (especially in the 700-plants) (Table 6). This responsiveness to H2O2 control, together with the stability in Cu,Zn-SOD and GR, could have alleviated heat stress in these plants by maintaining redox homeostasis (Li et al., 2014) and minimizing the heat-induced oxidative impairments to the photosynthetic apparatus (Figure 1; Rodrigues et al., 2016). These responses are in line with the well-known better heat tolerance of C. canephora (DaMatta and Ramalho, 2006). On the other hand, Icatu 380-plants showed reductions in the activity of all enzymes (except Cu,Zn-SOD), likely contributing to the strongest decrease in photosynthetic performance (Rodrigues et al., 2016) and highest photoinhibition status (Figure 1) relative to the other genotypes. Furthermore, in Icatu 380-plants the increase in Cu,Zn-SOD, but not in APX and CAT activities, might have limited an integrated H2O2 scavenging (Fortunato et al., 2010). Compared with Icatu 380-plants, their 700-counterparts (despite showing decreased APX activity) displayed a strong upregulation of GR and CAT activities, as well as APX Cyt (and APX Chl) gene expression at 42/34°C, which may have contributed to a lower impact on the photosynthetic performance in elevated [CO2]. At the two highest temperatures, the scavenging capability in IPR108 700-plants was not stronger than that of 380-plants (with the exception related to CAT activity), and a lower gene expression for the antioxidant enzymes and other protective proteins (HSP70, ELIP, Chape 20, and 60) was observed. Therefore, a better ROS control could be mostly prompted by the higher rates of electrons driven to C-assimilation, as also reported in grapevine (Vitis vinifera) under high [CO2] and increased temperature (Salazar-Parra et al., 2012).
Chloroplastic APX was the most affected enzyme at 42/34°C, contrasting to the large increase in APX Cyt expression, which was the most responsive gene to high temperature (in CL153). Since CAT was the only enzyme to present significant increased activities in the three genotypes in the 700-plants (and also in CL153 380-plants), this suggested a drift of H2O2 control from chloroplast APX to extra-chloroplast CAT (predominantly located in peroxisomes, glyoxissomes, and mitochondria) and possibly cytosolic APX. In fact, since H2O2 is capable of diffusing passively across membranes, extrachloroplastic scavenging systems are important H2O2 detoxification pathways (Feierabend, 2005; Logan, 2005).
Other Protective Molecules
ROS control can be complemented by ASC, TOC, HSPs, and other cellular protectants under stressful conditions (Suzuki and Mittler, 2006). The changes found in other protective molecules were mostly promoted by temperature rather than by enhanced [CO2] per se. This was the case of ASC, which decreased significantly with supra-optimal temperatures in all genotypes, which could have been promoted by TOC accumulation (Kanwischer et al., 2005) in C. arabica plants. At the highest temperature, ASC reductions were in line with losses in APX activity, as observed in CL153 and Icatu. Similar results have also been reported in other species (Erice et al., 2007). In fact, ASC reacts with H2O2 in a reaction catalyzed by APX, as well as non-enzymatically with 1O2, O2i−, OHi, and lipid hydroperoxides (Asada, 1994; Foyer, 2002; Logan, 2005). Given that ASC is also a substrate for V de-epoxidase to synthesize Z from V (Logan 2005; Smirnoff 2005), decreases in ASC pools might also have limited Z synthesis, thus ultimately resulting in a modest DEPS value (Table 2) under heat stress.
In plants, TOC pools are confined to chloroplasts (Munné-Bosch, 2005). Therefore, the remarkable increase of this lipophilic antioxidant under heat stress in C. arabica, especially in the 380-plants at 42/24°C, clearly reflected a positive stress response at chloroplast level, given that TOC deactivates 1O2, , OH•, limits lipid peroxidation by reducing lipid peroxyl radicals, and stabilizes membrane structures due to its interactions with polyunsaturated fatty acyl chains (Munné-Bosch, 2005; Smirnoff, 2005). This action was further reinforced by the presence of high β-carotene (Table 2) in C. arabica genotypes (mostly in Icatu) that co-operate in limiting 1O2 damages (Munné-Bosch, 2005) and regenerating carotenoid radicals produced with the reaction of carotenoid with lipid peroxyl radicals (Smirnoff, 2005). This TOC increase contrasted with an absence of upregulation of TOC My and TOC Cyt genes (genes coding for enzymes that catalyse the last two steps of TOC synthesis) in C. arabica plants regardless of [CO2]. However, this apparent discrepancy may be related to the observation that these final steps might be not limiting to TOC synthesis (Kanwischer et al., 2005).
The RFOs pathway is highly adjustable in plant stress response. The only RFOs detected in coffee leaves, stachyose, and raffinose, increased at the two highest temperatures. RFOs typically accumulate under environmental stresses (Sicher, 2013), having membrane protective roles (including the maintenance of thylakoid electron transport) against temperature, drought, and ROS impairments (Santarius, 1973; Santos et al., 2011; Sicher, 2013). Therefore, their increases were consistent with the preservation of thylakoid electron transport involving both photosystems capabilities in coffee (Rodrigues et al., 2016). Nevertheless, in IPR108, despite the buildup in stachyose and raffinose contents promoted by high temperature, it seems noteworthy that high [CO2] plants maintained significantly lower values than 380-plants. Assuming that these leaf metabolites accumulation was related to activation of stress related genes, a lower buildup under heat stress conditions could point that CO2 enrichment was able to mitigate this process, as suggested to occur in soybean leaflets, where high temperature promoted raffinose increase under ambient CO2, but not under high [CO2] (Sicher, 2013).
Plants evolved also molecular chaperones as stress defenses. These are a group of functional proteins with key roles in protein protection in both optimal and adverse conditions (Wang et al., 2004), including HSPs that are related to thermal acclimation. HSP70 prevents proteins of different metabolic pathways from denaturation and aggregation, helping in folding, refolding, and assembly, as well as in translocation processes and in facilitating the proteolytic degradation of unstable proteins (Wang et al., 2004; Fragkostefanakis et al., 2015). In coffee plants HSP70 content was not constitutively buildup by high [CO2], similar to the findings of Bokhari et al. (2007) in rice, accompanied by a downregulation tendency of HSP70 gene at 25/20°C in the 700-plants relative to the 380-ones. However, HSP70 synthesis was one of the earlier responses to rising temperature, from 31/25 upwards. Although some HSP70 downregulation occurred under high CO2 at control temperature, increased transcriptional levels were found at the two highest temperatures for all genotypes. Furthermore, although this response was not clearly related to high CO2,higher HSP70 gene upregulation were observed in 700-plants of CL153 and Icatu at 42/34°C. Similar strong and fast transcriptional activation has been considered essential for protein homeostasis and a requisite for development and survival under stress (Fragkostefanakis et al., 2015). HSP70 can also be involved in the protection and repair of PSII (Schroda et al., 1999) in addition to having a pivotal role in the upregulation of enzymatic antioxidant defenses, under single or combined drought and heat stresses, thereby indirectly helping ROS control (Hu et al., 2010). Therefore, HSP70 increases might have contributed to preserve PSII functioning at supra-optimal temperatures (Figure 1; Rodrigues et al., 2016) until 37/30°C for both [CO2], and to stimulate GR and CAT activities at 42/34°C (700-plants) (Table 4).
Concerning other protective molecules, the gene expression of chaperonins 20 and 60 (Chape 20 and 60) and early light-induced protein (ELIP) from the chloroplast was also examined (Table 6). As for HSP70, while significant changes in Chape 20 and 60 showed a clear upregulation under elevated CO2 at 42/34°C (in CL153 and Icatu), under control temperature the expression did not significantly change, or even tend to decrease, with CO2 enrichment, as also observed by Vicente et al. (2015) in durum wheat. In this study, a 4°C increase further downregulated Chape 60 transcription, especially under low N-availability. However, both chaperonin 20 and 60 have been associated with enhancement of stress tolerance as they play a crucial role by assisting a wide range of newly synthesized and newly translocated proteins to achieve their native forms, namely RuBisCO (see Wang et al., 2004). Furthermore, chaperonin 60 is also involved in the assembly of chloroplast ATP synthase (Mao et al., 2015). Therefore, the strong upregulation Chape 20 and Chape 60 at the two highest temperatures, especially in 700-plants of CL153 and Icatu, could helped to maintain ATP synthesis coupled to thylakoid electron transport, a lower PSII inactivation and higher photosynthetic potential, as observed at 42/24°C in these plants (Rodrigues et al., 2016).
The ELIP family members are nuclear-encoded photoprotective proteins that accumulate in thylakoid membranes in response to various abiotic stresses. They prevent free radical formation and participate in energy dissipation (Adamska, 2001). Indeed a strong relation between tolerance to photooxidation and ELIPs level has been reported (Hutin et al., 2003). Thus, the stronger ELIP upregulation on the 700-plants of CL153 and Icatu might have played a role in preserving thylakoid functions (Rodrigues et al., 2016), thus avoiding photoinhibition (Figure 1).
Conclusions
Relevant heat tolerance up to 37/30°C for both [CO2] and all coffee genotypes was observed, largely supported by the maintenance or increase of the pools of several protective molecules (neoxanthin, lutein, carotenes, TOC, HSP70, raffinose), by the activities of antioxidant enzymes (SOD, APX, QR, CAT), and by the upregulated expression of some genes (ELIP, Chape 20). Nevertheless, C. arabica plants seemed to be more responsive, although improved photosynthetic activity was promoted under high [CO2] in all genotypes (Rodrigues et al., 2016). However, at 42/34°C photosynthesis photoinhibition was manifested, especially in the 380-plants and in Icatu. At this temperature a global reinforcement of the antioxidative system was not observed, but gene upregulation of protective mechanisms (HSP70, chaperonins, ELIP, and APX Cyt), as well as raffinose content, constituted a common heat defense line in all genotypes. A consistently higher expression was observed in CL153 at the highest temperature, in agreement with its better tolerance to elevated temperatures. TOC and HSP70 seemed to be particularly relevant in C. arabica genotypes. The presence of RFOs, lutein, β-carotene, TOC, and HSP70, as well as the upregulated expression of genes related to protective proteins (ELIPS, HSP70, Chape 20, and 60) and antioxidant enzymes (CAT, CuSOD2, APX Cyt, APX Chl), which should act in concert to control ROS formation and scavenging, were mostly driven by temperature increase. Nevertheless, it was noteworthy that the plants grown under enhanced [CO2] maintained higher activities of GR (Icatu), CAT (Icatu and IPR), and kept (or even increased) the activities of Cu,Zn-SOD, APX, and CAT. These differences between [CO2] were particularly clear in Icatu, with the 380-plants suffering from the greatest photosynthetic impact amongst the genotypes. Furthermore, the strongest upregulation of genes related to protective proteins found in the 700-plants of CL153 and Icatu likely contributed to strengthen their ability to maintain higher functional levels. The simultaneous gene upregulation of antioxidative enzymes and molecular chaperones at the two highest temperatures fully agrees with the known pivotal protective roles of these proteins, as well as to the observed cross-talk between HSPs/chaperones and other stress response mechanisms under abiotic stress conditions (Wang et al., 2004).
Notably, our data also suggest a drift of H2O2 control from chloroplast (APX) to extra-chloroplast (CAT) taking into account the increases in CAT activities in all 700-plants (and CL153 380-plants) coupled with strong decreases in APX activity. The 700-plants showed higher photochemical energy use ability, which should constitute the major sink for electron transport, preventing electrons to react with O2 to produce ROS. Therefore, this should be perceived as a first line of defense against excessive energy load, constituting a primary mean of protection against oxidative conditions and photoinhibition, allowing acclimation to take place.
Overall, our results showed that high [CO2] mitigated the heat impact through higher photosynthetic functioning, upregulation of protective molecules, as well as through higher activity of some antioxidant enzymes. Such high [CO2]-dependent effects on antioxidant defenses likely favored the maintenance of ROS at controlled levels and would in turn justify the lower heat impact on the photosynthetic components under elevated [CO2] (Rodrigues et al., 2016). Therefore, our findings clearly extend our understanding why high [CO2] constitutes a key player to coffee heat resilience and acclimation, what is clearly relevant in the context of predicted future global warming scenarios for the coffee crop sustainability.
Author Contributions
According to their competences, all authors contributed transversally to the several stages of the work, including its design, data acquisition, analysis and interpretation, critically review of the manuscript, and approval of the submitted version. Furthermore, they agree to be accountable for all aspects of the work in ensuring that questions related to the accuracy or integrity of any part of the work were appropriately investigated and resolved.
Funding
This work was supported by national funds from Fundação para a Ciência e a Tecnologia through the projects PTDC/AGR-PRO/3386/2012, the research units UID/AGR/04129/2013 (LEAF) and UID/GEO/04035/2013 (GeoBioTec), as well through the grant SFRH/BPD/47563/2008 (AF) co-financed through the POPH program subsidized by the European Social Fund. Brazilian funding from CAPES (grants PDSE: 000427/2014-04, W.P. Rodrigues; 0343/2014-05, MM; 12226/12-2, LM), CNPq and Fapemig (fellowships to FDM, FP, and EC) are also greatly acknowledged.
Conflict of Interest Statement
The authors declare that the research was conducted in the absence of any commercial or financial relationships that could be construed as a potential conflict of interest.
Acknowledgments
The authors would like to thank DELTA Cafés (Portugal) for technical assistance, and Profs. T. Sera (IAPAR) for supplying IPR108 material.
Abbreviations
A, antheraxanthin; APX, ascorbate peroxidase; ASC, ascorbate; Chl, chlorophyll; 3Chl*, triplet state of Chl; Chl (a+b), Total Chl; Cu, Zn-SOD, Cu, Zn-superoxide dismutase; DHAR, dehydroascorbate reductase; GR, glutathione reductase; gs, stomatal conductance; H2O2, hydrogen peroxide; 1O2, singlet oxygen; , superoxide anion radical; •OH, hydroxyl radical; PIChr, PIDyn, and PITot, chronic photoinhibition, dynamic photoinhibition, and total photoinhibition; SD, stomatal density; SI, stomatal index; ROS, reactive oxygen species; TOC, α-tocopherol; V, violaxanthin; Z, zeaxanthin.
References
AbdElgawad, H., Farfan-Vignolo, A. R., Vos, D., and Asard, H. (2015). Elevated CO2 mitigates drought and temperature-induced oxidative stress differently in grasses and legumes. Plant Sci. 231, 1–10. doi: 10.1016/j.plantsci.2014.11.001
Adamska, I. (2001). “The Elip family of stress proteins in the thylakoid membranes of pro- and eukaryote,” in Advances in Photosynthesis and Respiration-Regulation of Photosynthesis, Vol. 11, ed E. M. Aro and B. Andersson (Dordrecht: Kluwer Academic Publishers), 487–505.
Ainsworth, E. A., and Rogers, A. (2007). The response of photosynthesis and stomatal conductance to rising [CO2]: mechanisms and environmental interactions. Plant Cell Environ. 30, 258–270. doi: 10.1111/j.1365-3040.2007.01641.x
Asada, K. (1994). “Mechanisms for scavenging reactive molecules generated in chloroplasts under light stress,” in Photoinhibition of Photosynthesis – From Molecular Mechanisms to the Field, eds N. R. Baker and J. R. Bowyer (Oxford: BIOS Scientific Publishers Ltd.), 129–142.
Bader, M. K.-F., Siegwolf, R., and Körner, C. (2010). Sustained enhancement of photosynthesis in mature deciduous forest trees after 8 years of free air CO2 enrichment. Planta 232, 1115–1125. doi: 10.1007/s00425-010-1240-8
Batista-Santos, P., Lidon, F. C., Fortunato, A., Leitão, A. E., Lopes, E., Partelli, F., et al. (2011). The impact of cold on photosynthesis in genotypes of Coffea spp. – Photosystem sensitivity, photoprotective mechanisms and gene expression. J. Plant Physiol. 168, 792–806. doi: 10.1016/j.jplph.2010.11.013
Bita, C. E., and Gerats, T. (2013). Plant tolerance to high temperature in a changing environment: scientific fundamentals and production of heat stress-tolerant crops. Front. Plant Sci. 4:273. doi: 10.3389/fpls.2013.00273
Boisvenue, C., and Running, S. W. (2006). Impacts of climate change on natural forest productivity – evidence since the middle of the 20th century. Global Change Biol. 12, 862–882. doi: 10.1111/j.1365-2486.2006.01134.x
Bokhari, S. A., Wan, X.-Y., Yang, Y. W., Zhou, L., Tang, W.-L., and Liu, J.-Y. (2007). Proteomic response of rice seedling leaves to elevated CO2 levels. J. Proteome Res. 6, 4624–4633. doi: 10.1021/pr070524z
Bradford, M. M. (1976). A rapid and sensitive method for the quantitation of microgram quantities of protein utilizing the principle of protein-dye binding. Anal. Biochem. 72, 248–254. doi: 10.1016/0003-2697(76)90527-3
Bunn, C., Läderach, P., Rivera, O. O., and Kirschke, D. (2015). A bitter cup: climate change profile of global production of Arabica and Robusta coffee. Clim. Change 129, 89–101. doi: 10.1007/s10584-014-1306-x
Camargo, M. B. P. (2010). The impact of climatic variability and climate change on arabic coffee crop in Brazil. Bragantia 69, 239–247. doi: 10.1590/S0006-87052010000100030
Cavatte, P. C., Oliveira, Á. A. G., Morais, L. E., Martins, S. C. V., Sanglard, L. M. V. P., and DaMatta, F. M. (2012). Could shading reduce the negative impacts of drought on coffee? A morphophysiological analysis. Physiol. Plant 114, 111–122. doi: 10.1111/j.1399-3054.2011.01525.x
Chaves, A. R. M., Ten-Caten, A., Pinheiro, H. A., Ribeiro, A., and DaMatta, F. M. (2008). Seasonal changes in photoprotective mechanisms of leaves from shaded and unshaded field-grown coffee (Coffea arabica L.). Trees 22, 351–361. doi: 10.1007/s00468-007-0190-7
Craparo, A. C. W., Van Asten, P. J. A., Läderach, P., Jassogne, L. T. P., and Grab, S. W. (2015). Coffea arabica yields decline in Tanzania due to climate change: global implications. Agric. For. Meteorol. 207, 1–10. doi: 10.1016/j.agrformet.2015.03.005
DaMatta, F. M., and Ramalho, J. C. (2006). Impacts of drought and temperature stress on coffee physiology and production: a review. Braz. J. Plant Physiol. 18, 55–81. doi: 10.1590/S1677-04202006000100006
DaMatta, F. M., Godoy, A. G., Menezes-Silva, P. E., Martins, S. C. V., Sanglard, L. M. V. P., Morais, L. E., et al. (2016). Sustained enhancement of photosynthesis in coffee trees grown under free-air CO2 enrichment conditions: disentangling the contributions of stomatal, mesophyll, and biochemical limitations J. Exp. Bot. 167, 341–352. doi: 10.1093/jxb/erv463
DaMatta, F. M., Grandis, A., Arenque, B. C., and Buckeridge, M. S. (2010). Impacts of climate changes on crop physiology and food quality. Food Res. Int. 43, 1814–1823. doi: 10.1016/j.foodres.2009.11.001
Erice, G., Aranjuelo, I., Irigoyen, J. J., and Sánchez-Díaz, M. (2007). Effect of elevated CO2, temperature and limited water supply on antioxidant status during regrowth of nodulated alfalfa. Physiol. Plant. 130, 33–45. doi: 10.1111/j.1399-3054.2007.00889.x
Faria, T., Wilkins, D., Besford, R. T., Vaz, M., Pereira, J. S., and Chaves, M. M. (1996). Growth at elevated CO2 leads to down-regulation of photosynthesis and altered response to high temperature in Quercus suber L. seedlings. J. Exp. Bot. 47, 1755–1761. doi: 10.1093/jxb/47.11.1755
Feierabend, J. (2005). “Catalases in plants: molecular and functional properties and role in stress defense,” in Antioxidants and Reactive Oxygen in Plants, ed. N. Smirnoff (Oxford: Blackwell Publishing), 99–140.
Fortunato, A., Lidon, F. C., Batista-Santos, P., Leitão, A. E., Pais, I. P., Ribeiro, A. I., et al. (2010). Biochemical and molecular characterization of the antioxidative system of Coffea sp. under cold conditions in genotypes with contrasting tolerance. J. Plant Physiol. 167, 333–342. doi: 10.1016/j.jplph.2009.10.013
Foyer, C. H. (2002). “The contribution of photosynthetic oxygen metabolism to oxidative stress in plants,” in Oxidative Stress in Plants, eds D. Inzé and M. Van Montagu (London: Taylor & Francis), 33–68.
Fragkostefanakis, S., Röth, S., Schleiff, E., and Scharf, K.-D. (2015). Prospects of engineering thermotolerance in crops through modulation of heat stress transcription factor and heat shock protein networks. Plant Cell Environ. 38, 1881–1895. doi: 10.1111/pce.12396
Ghini, R., Torre-Neto, A., Dentzien, A. F. M., Guerreiro-Filho, O., Lost, R., Patrício, F. R. A., et al. (2015). Coffee growth, pest and yield responses to free-air CO2 enrichment. Clim. Change 132, 307–320. doi: 10.1007/s10584-015-1422-2
Goulao, L., Fortunato, A., and Ramalho, J. C. (2012). Selection of reference genes for normalizing quantitative qReal-Time PCR gene expression data with multiple variables in Coffea spp. Plant Mol. Biol. Rep. 30, 741–759. doi: 10.1007/s11105-011-0382-6
Haldimann, P., and Feller, U. (2004). Inhibition of photosynthesis by high temperature in oak (Quercus pubescens L.) leaves grown under natural conditions closely correlates with a reversible heat-dependent reduction of the activation state of ribulose-1,5-bisphosphate carboxylase/oxygenase. Plant Cell Environ. 27, 1169–1183. doi: 10.1111/j.1365-3040.2004.01222.x
Hasanuzzaman, M., Nahar, K., and Fujita, M. (2013). “Extreme temperature responses, oxidative stress and antioxidant defense in plants,” in Abiotic Stress - Plant Responses and Applications in Agriculture, eds K. Vahdati and C. Leslie (InTech), 169–205. Available online at: http://cdn.intechopen.com/pdfs-wm/43317.pdf
Hu, X., Liu, R., Li, Y., Wang, W., Tai, F., Xue, R., et al. (2010). Heat shock protein 70 regulates the abscisic acid-induced antioxidant response of maize to combined drought and heat stress. Plant Growth Reg. 60, 225–235. doi: 10.1007/s10725-009-9436-2
Hutin, C., Nussaume, L., Moise, N., Moya, I., Kloppstech, K., and Havaux, M. (2003). Early light-induced proteins protect Arabidopsis from photooxidative stress. Proc. Natl. Acad. Sci. U.S.A. 100, 4921–4926. doi: 10.1073/pnas.0736939100
IPCC (2013). “Climate Change 2013: the physical science basis. summary for policymakers, technical summary and frequent asked questions,” in Part of the Working Group I Contribution to the Fifth Assessment Report of the Intergovernmental Panel on Climate Change, eds T. F. Stocker, D. Qin, G.-K. Plattner, M. M. B. Tignor, S. K. Allen, J. Boschung, A. Nauels, Y. Xia, V. Bex, and P. M. Midgley (Intergovernmental Panel on Climate Change), 203.
IPCC (2014). “Climate Change 2014: mitigation of climate change,” in Contribution of Working Group III to the Fifth Assessment Report of the Intergovernmental Panel on Climate Change, eds O. Edenhofer, R. Pichs-Madruga, Y. Sokona, J. C. Minx, E. Farahani, S. Kadner, K. Seyboth, A. Adler, I. Baum, S. Brunner, P. Eickemeier, B. Kriemann, J. Savolainen, S. Schlömer, C. von Stechow, and T. Zwickel (Cambridge; New York: Cambridge University Press), 1435.
Kalituho, L., Rech, J., and Jahns, P. (2007). The roles of specific xanthophylls in light utilization. Planta 225, 423–439. doi: 10.1007/s00425-006-0356-3
Kanwischer, M., Porfirova, S., Bergmüller, E., and Dörmann, P. (2005). Alterations in tocopherol cyclase activity in transgenic and mutant plants of Arabidopsis affect tocopherol content, tocopherol composition, and oxidative stress. Plant Physiol. 137, 713–723. doi: 10.1104/pp.104.054908
Kirschbaum, M. U. F. (2011). Does enhanced photosynthesis enhance growth? Lessons learned from CO2 enrichment studies. Plant Physiol. 155, 117–124. doi: 10.1104/pp.110.166819
Lambers, H., Chapin, F. S. III, and Pons, T. L. (2008). Plant Physiological Ecology, 2nd Edn. New York, NY: Springer-Verlag, 604.
Li, X., Ahammed, G., Zhang, Y., Zhang, G., Sun, Z., Zhou, J., et al. (2014). Carbon dioxide enrichment alleviates heat stress by improving cellular redox homeostasis through an ABA-independent process in tomato plants. Plant Biol. 17, 81–89. doi: 10.1111/plb.12211
Lichtenthaler, H. K. (1987). Chlorophylls and carotenoids: pigments of photosynthetic biomembranes. Methods Enzymol. 148, 350–382. doi: 10.1016/0076-6879(87)48036-1
Lichtenthaler, H. K., and Babani, F. (2004). “Light adaptation and senescence of the photosynthetic apparatus. Changes in pigment composition, chlorophyll fluorescence parameters and photosynthetic activity,” in Chlorophyll a Fluorescence: A Signature of Photosynthesis, eds G. C. Papageorgiou and Govindjee (Dordrecht: Springer), 713–736.
Logan, B. A. (2005). “Reactive oxygen species and photosynthesis,” in Antioxidants and Reactive Oxygen in Plants, ed N. Smirnoff (Oxford: Blackwell Publishing), 250–267.
Long, S. P., Ainsworth, E. A., Leakey, A. D. B., Nösberger, J., and Ort, D. R. (2006). Food for thought: lower-than-expected crop yield stimulation with rising CO2 concentrations. Science 312, 1918–1921. doi: 10.1126/science.1114722
Luo, Y., Reynolds, J., Wang, Y., and Wolfe, D. (1999). A search for predictive understanding of plant responses to elevated [CO2]. Global Change Biol. 5, 143–156. doi: 10.1046/j.1365-2486.1999.00215.x
Mao, J., Chi, W., Ouyang, M., He, B., Chen, F., and Zhang, L. (2015). PAB is an assembly chaperone that functions downstream of chaperonin 60 in the assembly of chloroplast ATP synthase coupling factor 1. Proc. Natl. Acad. Sci. U.S.A. 112, 4152–4157. doi: 10.1073/pnas.1413392111
Martins, L. D., Tomaz, M. A., Lidon, F. C., DaMatta, F. M., and Ramalho, J. C. (2014). Combined effects of elevated [CO2] and high temperature on leaf mineral balance in Coffea spp. plants. Clim. Change 126, 365–379. doi: 10.1007/s10584-014-1236-7
Matsubara, S., Chen, Y.-X., Caliandro, R., and Govindjee, Clegg, R. M. (2011). Photosystem II fluorescence lifetime imaging in avocado leaves: contributions of the lutein-epoxide and violaxanthin cycles to fluorescence quenching. J. Photochem. Photobiol. B 104, 271–284. doi: 10.1016/j.jphotobiol.2011.01.003
Munné-Bosch, S. (2005). The role of alpha-tocopherol in plant stress tolerance. J. Plant Physiol. 162, 743–748. doi: 10.1016/j.jplph.2005.04.022
Njemini, R., Demanet, C., and Mets, T. (2003). Determination of intracellular heat shock protein 70 using a newly developed cell lysate immunometric assay. J. Immunol. Methods 274, 271–279. doi: 10.1016/S0022-1759(03)00004-8
Pompelli, M. F., Martins, S. C. V., Antunesm, W. C., Chaves, A. R. M., and DaMatta, F. M. (2010). Photosynthesis and photoprotection in coffee leaves is affected by nitrogen and light availabilities in winter conditions. J. Plant Physiol. 167, 1052–1060. doi: 10.1016/j.jplph.2010.03.001
Prasad, P. V. V., Boote, K. J., Allen, L. H. Jr., and Thomas, J. M. G. (2003). Super-optimal temperatures are detrimental to peanut (Arachis hypogaea L.) reproductive processes and yield at both ambient and elevated carbon dioxide. Global Change Biol. 9, 1775–1787. doi: 10.1046/j.1365-2486.2003.00708.x
Pritchard, S. G., Ju, Z., Santen, E., Qiu, J., Weaver, D. B., Prior, S. A., et al. (2000). The influence of elevated CO2 on the activities of antioxidative enzymes in two soybean genotypes. Aust. J. Plant Physiol. 27, 1061–1068. doi: 10.1071/pp99206
Ramalho, J. C., Campos, P. S., Teixeira, M., and Nunes, M. A. (1998). Nitrogen dependent changes in antioxidant system and in fatty acid composition of chloroplast membranes from Coffea arabica L. plants submitted to high irradiance. Plant Sci. 135, 115–124. doi: 10.1016/S0168-9452(98)00073-9
Ramalho, J. C., DaMatta, F. M., Rodrigues, A. P., Scotti-Campos, P., Pais, I., Batista-Santos, P., et al. (2014). Cold impact and acclimation response of Coffea spp. plants. Theor. Exp. Plant Physiol. 26, 5–18. doi: 10.1007/s40626-014-0001-7
Ramalho, J. C., Fortunato, A. S., Goulao, L. F., and Lidon, F. C. (2013a). Cold-induced changes in mineral content in leaves of Coffea spp. Identification of descriptors for tolerance assessment. Biol. Plant. 57, 495–506. doi: 10.1007/s10535-013-0329-x
Ramalho, J. C., Pons, T., Groeneveld, H., and Nunes, M. A. (1997). Photosynthetic responses of Coffea arabica leaves to a short-term high light exposure in relation to N availability. Physiol. Plant. 101, 229–239. doi: 10.1111/j.1399-3054.1997.tb01841.x
Ramalho, J. C., Rodrigues, A. P., Semedo, J. N., Pais, I. P., Martins, L. D., Simões-Costa, M. C., et al. (2013b). Sustained photosynthetic performance of Coffea spp. under long-term enhanced [CO2]. PLoS ONE 8:e82712. doi: 10.1371/journal.pone.0082712
Rodrigues, W. P., Martins, M. Q., Fortunato, A. S., Rodrigues, A. P., Semedo, J. N., Simões-Costa, M. C., et al. (2016). Long-term elevated air [CO2] strengthens photosynthetic functioning and mitigates the impact of supra-optimal temperatures in tropical Coffea arabica and C. canephora species. Global Change Biol. 22, 415–431. doi: 10.1111/gcb.13088
Salazar-Parra, C., Aguirreolea, J., Sánchez-Díaz, M., Irigoyen, J. J., and Morales, F. (2012). Climate change (elevated CO2, elevated temperature and moderate drought) triggers the antioxidant enzymes response of grapevine cv. Tempranillo, avoiding oxidative damage. Physiol. Plant. 144, 99–110. doi: 10.1111/j.1399-3054.2011.01524.x
Santarius, K. A. (1973). The protective effect of sugars on chloroplast membranes during temperature and water stress and its relationship to frost, desiccation and heat resistance. Planta 113, 105–114. doi: 10.1007/BF00388196
Santos, T. B., Budzinski, I. G. F., Marur, C. J., Petkowicz, C. L. O., Pereira, L. F. P., and Vieira, L. G. E. (2011). Expression of three galactinol synthase isoforms in Coffea arabica L. and accumulation of raffinose and stachyose in response to abiotic stresses. Plant Physiol. Biochem. 49, 441–448. doi: 10.1016/j.plaphy.2011.01.023
Schroda, M., Vallon, O., Wollman, F. A., and Beck, C. F. (1999). A chloroplast targeted heat shock protein 70 (HSP70) contributes to the photoprotection and repair of photosystem II during and after photoinhibition. Plant Cell 11, 1165–1178. doi: 10.1105/tpc.11.6.1165
Sicher, R. (2013). Combined effects of CO2 enrichment and elevated growth temperatures on metabolites in soybean leaflets: evidence for dynamic changes of TCA cycle intermediates. Planta 238, 369–380. doi: 10.1007/s00425-013-1899-8
Smirnoff, N. (2005). “Ascorbate, tocopherol and carotenoids: metabolism, pathway engineering and functions,” in Antioxidants and Reactive Oxygen in Plants, ed N. Smirnoff (Oxford: Blackwell Publishing), 1–24.
Suzuki, N., and Mittler, R. (2006). Reactive oxygen species and temperature stresses: a delicate balance between signaling and destruction. Physiol. Plant. 126, 45–51. doi: 10.1111/j.0031-9317.2005.00582.x
van der Vossen, H., Bertrand, B., and Charrier, A. (2015). Next generation variety development for sustainable production of arabica coffee (Coffea arabica L.): a review. Euphytica 204, 243–256. doi: 10.1007/s10681-015-1398-z
Vicente, R., Pérez, P., Martínez-Carrasco, R., Usadel, B., Kostadinova, S., and Morcuende, R. (2015). Quantitative RT–PCR platform to measure transcript levels of C and N metabolism-related genes in durum wheat: transcript profiles in elevated [CO2] and high temperature at different levels of N supply. Plant Cell Physiol. 56, 1556–1573. doi: 10.1093/pcp/pcv079
Vurro, E., Bruni, R., Bianchi, A., and Toppi, L. S. (2009). Elevated atmospheric CO2 decreases oxidative stress and increases essential oil yield in leaves of Thymus vulgaris grown in a mini-FACE system. Environ. Exp. Bot. 65, 99–106. doi: 10.1016/j.envexpbot.2008.09.001
Wahid, A., Gelani, S., Ashraf, M., and Foolad, M. R. (2007). Heat tolerance in plants: an overview. Environ. Exp. Bot. 61, 199–223. doi: 10.1016/j.envexpbot.2007.05.011
Wang, W., Vinocur, B., Shoseyov, O., and Altman, A. (2004). Role of plant heat-shock proteins and molecular chaperones in the abiotic stress response. Trends Plant Sci. 9, 244–252. doi: 10.1016/j.tplants.2004.03.006
Way, D. A., Oren, R., and Kroner, Y. (2015). The space-time continuum: the effects of elevated CO2 and temperature on trees and the importance of scaling. Plant Cell Environ. 38, 991–1007. doi: 10.1111/pce.12527
Werner, C., Correia, O., and Beyschlag, W. (2002). Characteristic patterns of chronic and dynamic photoinhibition pf different functional groups in a Mediterranean ecosystem. Funct. Plant Biol. 29, 999–1011. doi: 10.1071/PP01143
Woodward, F. I. (2002). Potential impacts of global elevated CO2 concentrations on plants. Curr. Opin. Plant Biol. 5, 207–211. doi: 10.1016/S1369-5266(02)00253-4
Keywords: acclimation, antioxidants, coffee, chloroplast, climate change, enhanced [CO2], global warming, heat
Citation: Martins MQ, Rodrigues WP, Fortunato AS, Leitão AE, Rodrigues AP, Pais IP, Martins LD, Silva MJ, Reboredo FH, Partelli FL, Campostrini E, Tomaz MA, Scotti-Campos P, Ribeiro-Barros AI, Lidon FJC, DaMatta FM and Ramalho JC (2016) Protective Response Mechanisms to Heat Stress in Interaction with High [CO2] Conditions in Coffea spp. Front. Plant Sci. 7:947. doi: 10.3389/fpls.2016.00947
Received: 27 April 2016; Accepted: 14 June 2016;
Published: 29 June 2016.
Edited by:
Iker Aranjuelo, Instituto de Agrobiotecnología, CSIC, UPNA, SpainReviewed by:
Rubén Vicente, University of Barcelona, SpainGorka Erice, Estación Experimental del Zaidín CSIC, Spain
Copyright © 2016 Martins, Rodrigues, Fortunato, Leitão, Rodrigues, Pais, Martins, Silva, Reboredo, Partelli, Campostrini, Tomaz, Scotti-Campos, Ribeiro-Barros, Lidon, DaMatta and Ramalho. This is an open-access article distributed under the terms of the Creative Commons Attribution License (CC BY). The use, distribution or reproduction in other forums is permitted, provided the original author(s) or licensor are credited and that the original publication in this journal is cited, in accordance with accepted academic practice. No use, distribution or reproduction is permitted which does not comply with these terms.
*Correspondence: José C. Ramalho, Y29jaGljaG9yQG1haWwudGVsZXBhYy5wdA==; Y29jaGljaG9yQGlzYS51bGlzYm9hLnB0
†These authors have contributed equally to this work.