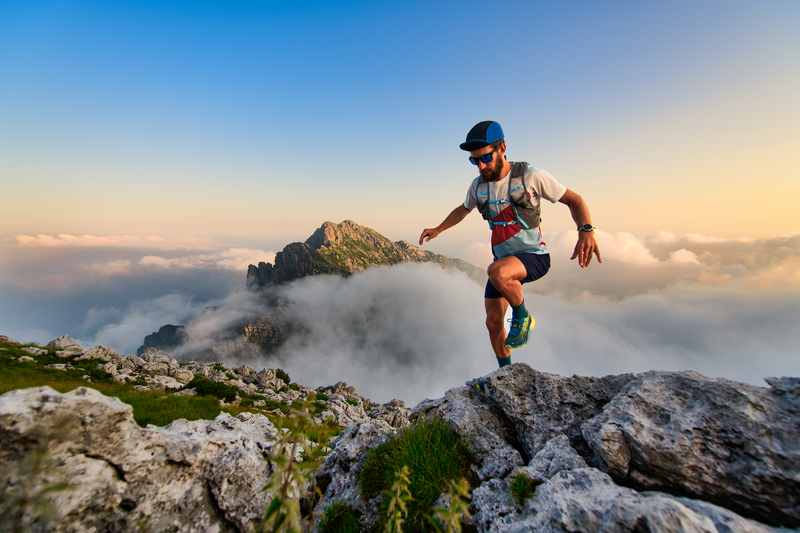
94% of researchers rate our articles as excellent or good
Learn more about the work of our research integrity team to safeguard the quality of each article we publish.
Find out more
REVIEW article
Front. Plant Sci. , 27 June 2016
Sec. Plant Breeding
Volume 7 - 2016 | https://doi.org/10.3389/fpls.2016.00913
This article mentions parts of:
Plant Breeding: A Success Story to be Continued Thanks to the Advances in Genomics
Flowering is a crucial determinant for plant reproductive success and seed-set. Increasing temperature and elevated carbon-dioxide (e[CO2]) are key climate change factors that could affect plant fitness and flowering related events. Addressing the effect of these environmental factors on flowering events such as time of day of anthesis (TOA) and flowering time (duration from germination till flowering) is critical to understand the adaptation of plants/crops to changing climate and is the major aim of this review. Increasing ambient temperature is the major climatic factor that advances flowering time in crops and other plants, with a modest effect of e[CO2].Integrated environmental stimuli such as photoperiod, temperature and e[CO2] regulating flowering time is discussed. The critical role of plant tissue temperature influencing TOA is highlighted and crop models need to substitute ambient air temperature with canopy or floral tissue temperature to improve predictions. A complex signaling network of flowering regulation with change in ambient temperature involving different transcription factors (PIF4, PIF5), flowering suppressors (HvODDSOC2, SVP, FLC) and autonomous pathway (FCA, FVE) genes, mainly from Arabidopsis, provides a promising avenue to improve our understanding of the dynamics of flowering time under changing climate. Elevated CO2 mediated changes in tissue sugar status and a direct [CO2]-driven regulatory pathway involving a key flowering gene, MOTHER OF FT AND TFL1 (MFT), are emerging evidence for the role of e[CO2] in flowering time regulation.
The Intergovernmental Panel on Climate Change Reports (e.g., IPCC, 2007, 2013) document evidence of increasing carbon-dioxide concentrations (e[CO2]) and other greenhouse gases leading to a higher frequency of extreme climate events such as heat waves and drought events. The impact of these climate events has already been documented on agricultural crop production, natural species diversity and distribution, and other ecosystem services such as flowering time, pollination etc. (Dale et al., 2001; Doney et al., 2012). Given that temperature is a major determinant of the timing and duration of key developmental phases, including flowering (Bahuguna and Jagadish, 2015), and [CO2] a major determinant of plant growth (Craufurd and Wheeler, 2009), climate change is likely to have significant impacts on key flowering processes.
Flowering time is defined here as the duration starting from seed germination till appearance of the first floral bud, open flower or anthesis (external appearance of anthers). Flowering time marks the visible transition from the vegetative to reproductive phase and is important because: (i) the duration from emergence to flowering determines the reproductive competency of a plant; (ii) the timing of flowering per se relative to the occurrence of abiotic and biotic constraints is critical for successful seed-set and propagation; (iii) the timing of flowering in ecosystems or plant communities may affect individual species “fitness” in relation to competition with other species; (iv) the synchrony of pollination with insects in ecosystems and hence the distribution of species may be affected. All the above functions can be affected by climate change; thus flowering time is one of the major factors determining the adaptation of plants to changing climate. Environmental factors such as photoperiod and temperature that control the timing of the first bud or flowering from a whole-plant physiological perspective has been described in detail by Craufurd and Wheeler (2009). In general, historical records of flowering time, herbaria and aerobiological documents on pollen data indicate advancement in flowering time in perennials (Crimmins et al., 2011; Hulme, 2011). However, shifts in flowering time among annuals, such as wheat (Triticum aestivum), which may be influenced by changes in crop management practices such as variable sowing dates, have to be interpreted with caution (Craufurd and Wheeler, 2009). The above is also true under conditions wherein crop breeding efforts are targeted toward earlier or later flowering to increase cultivar adaptability to environments faced with terminal or end of season drought stress.
A number of physiological processes that occur during anthesis, such as pollination, pollen germination and fertilization, are highly sensitive to extremes of temperature. The time of day of anthesis (TOA), which is defined as the appearance of the first open flower or anthesis on any particular flowering day, can also vary and provide an escape route to overcome the damage induced by high temperature. For example, in tropical and sub-tropical rice environments ambient air temperature gradually increases from early morning through solar noon and late afternoon, and decreases thereafter. Pollination occurs at or just prior to anthesis and fertilization is generally completed within a few minutes or hours after pollination (Cho, 1956; Kakani et al., 2005). High-temperature effects on processes from pollination through fertilization can be minimized by exploiting variation in the TOA, i.e., by selecting species or cultivars that flower earlier in the morning when temperatures are cooler. Some wild rice varieties have shown extremely large variation in the TOA, with Oryza alta flowering after 2100 h at night and O. officinalis as early as 0600 h in the morning (Sheehy et al., 2007). This variation, particularly from O. officinalis, has been exploited to develop rice varieties that flower a few hours earlier (toward dawn) in a day when temperatures are cooler, leading to enhanced seed-set even with exposure to early-afternoon hotter temperature (Ishimaru et al., 2010; Hirabayashi et al., 2015). Hence, some of the negative impacts of increasing temperature on the flowering events that affect seed-set (i.e., pollination, pollen germination and fertilization) can potentially be avoided by identifying and exploiting such unique flowering patterns, at least in annual grasses. This review aims to highlight ambient temperature and e[CO2] regulation on TOA and flowering time and implications for crop improvement under future climate.
Plants require a certain amount of heat units (thermal-time), synonymous with growing degree-days, to reach and progress to the next developmental stage. Provided the temperature is below a critical threshold, plants achieve these requirements earlier in warmer than cooler temperatures, thus increasing the rate of development (Craufurd and Wheeler, 2009). Many plant or crop models using cardinal temperatures (i.e., base, optimum, maximum temperature) and growing degree-days to drive development do not adequately account for the impact of high temperatures or short-term extremely high temperature stress events (but see Asseng et al., 2015). In addition, for species or cultivars sensitive to photoperiod, a greater understanding of the interactions between photoperiod and temperature above the optimum temperature is still required to improve existing crop/flowering models, especially for predicting responses to warmer and more extreme high-temperature events.
Research on high-temperature responses of mostly annual crop species such as rice (Oryza sativa L.) or sorghum (Sorghum bicolor), using controlled-environment conditions, has provided extensive knowledge on different high-temperature tolerance and escape mechanisms (Ishimaru et al., 2010; Jagadish et al., 2010; Jain et al., 2010; Talukder et al., 2013). Simulation models estimating temperature impacts on plants and crops have generally used standard meteorological air temperatures. However, canopy or floral tissue temperature can be considerably lower or higher than air temperature depending on soil water status and the microclimate surrounding sensitive plant organs (Yoshimoto et al., 2011; Guo et al., 2013; Julia and Dingkuhn, 2013). Significant variation in rice panicle temperature, from 9.5°C below to 2°C above ambient air temperature, was recorded with a common set of rice cultivars tested across contrasting environments in Senegal, France and the Philippines (Julia and Dingkuhn, 2013). Likewise, a 6°C lower rice panicle temperature in semi-arid climates of Australia (Matsui et al., 2007) and 4°C higher panicle temperature under humid conditions in China (Tian et al., 2010) and other rice-growing locations has been documented (Yoshimoto et al., 2011). The above provides strong evidence for large variation in air temperature and tissue (flower or spike or panicle) temperature, which can strongly influence flowering dynamics such as TOA (Julia and Dingkuhn, 2012). Interestingly, these differences in tissue temperature can be attributed largely to variation in the prevailing atmospheric relative humidity and hence demand for water mediated through vapor pressure deficit (VPD). This relationship strongly correlates with TOA and ultimately percent spikelet sterility (Shimono et al., 2005; Julia and Dingkuhn, 2013). Nonetheless, some effects attributed to changes in air temperature can be equally accounted for by water-deficit stress in crop models (such as maize in APSIM) that represent physiological processes (Lobell et al., 2013). This is because warmer temperatures increase VPD and demand for water that can lead to water-deficit stress.
There is also variation among species in their ability to regulate tissue temperature in floral organs. The presence of rigid epidermal pores on rice spikelets leads to lower flexibility in altering panicle temperature (Takahashi et al., 2008). The presence of active stomata on wheat glumes and awns (Steinmeyer et al., 2013) and buds of Brassica spp. (Guo et al., 2013) has been shown to actively moderate reproductive tissue temperatures when exposed to both high-temperature and water-deficit stress. Interestingly, water loss from detached leaves and buds of Brassica spp. indicated immediate closure of stomata on the leaf while the bud retained stomatal activity, extending evaporative cooling and leading to a delay in increasing bud temperature under drought stress (Guo et al., 2013). Canopy temperature depression (CTD) is being used as a measure of water-deficit stress avoidance facilitated by deeper roots in wheat (Lopes and Reynolds, 2010), differential leaf wax content for variation in reflecting radiation (Mahmud et al., 2016) or as a result of genetic variation in stomatal conductance in response to variation in VPD (Reynolds et al., 2007; Jagadish et al., 2011). The same concept has been recently extended to ear temperature depression (ETD) in wheat (Steinmeyer et al., 2013). Accounting for the variation in air and tissue (ear/floral) temperature can increase the precision of estimating TOA and seed-set in hotter environments. Hence, crop models parameterized with air temperature alone in environments varying in relative humidity or VPD do not predict responses to high temperatures well (for example, CSM-CROPSIM-CERES-Wheat, White et al., 2011; Asseng et al., 2015, for a comparison of wheat models). Thus, microclimate data that includes canopy and floral tissue temperature (including soil water potential while dealing with upland cereals) is proposed as the appropriate approach to fine-tune crop improvement traits such as TOA, in crop models as demonstrated recently for flooded rice (Julia and Dingkuhn, 2012, 2013; Yoshimoto et al., 2011).
Although the increase in global mean surface temperature is well documented, recent reports indicate a more rapid increase in minimum night temperature compared to maximum day temperature at the global (Vose et al., 2005), regional (Welch et al., 2010), and farm/field (Peng et al., 2004) level, reducing the day-night temperature amplitude (Christensen et al., 2007). This phenomena of warmer nights and warmer mean temperatures, leads to earlier TOA in cereals such as rice (Kobayashi et al., 2010; Julia and Dingkuhn, 2013). For example, variation in the mean minimum air temperature during the 7 days preceding anthesis was negatively correlated (R = −0.85) with the variation in time of day at peak anthesis (maximum number of open spikelets; Julia and Dingkuhn, 2012). In contrast, Shi et al. (2013) found no significant change in the flowering patterns in rice exposed to higher night temperatures between panicle initiation and flowering in a semi-controlled field experiment. Hence, more research on the effects of temperature amplitude on TOA, flowering pattern and other reproductive processes is needed.
Temperature affects flowering time by both affecting the rate of development directly and influencing vernalization (Craufurd and Wheeler, 2009). Vernalization is defined as the requirement for a period of chilling in winter to either break dormancy (in trees) or as an essential prerequisite to respond to other flowering-promoting stimuli in winter crops such as winter wheat or barley, and many bulb species (Amasino and Michaels, 2010). Predicted increase in mean temperatures of up to 3.7°C by the end of the century (IPCC, 2013) could have a significant impact on vernalization. For instance, a global analysis of the change in the “safe-winter-chill” period under different climate change scenarios suggests that there is likely to be a significant reduction in safe-winter-chill in warmer regions, which could have severe impacts on flowering time and production of temperate fruit and nut trees (Luedeling et al., 2011). With temperatures projected to increase at a higher rate during winters than during summers in cold regions, experimentally increased winter warming (0.4–2.4°C) resulted in a significant reduction in flower number and seed production. Interestingly, the impact of warmer winter temperatures was greatest on multi-inflorescence species rather than the single inflorescence species (Liu et al., 2012). Historical flowering date data of more than 400 plant species and deciduous trees at decadal time scale over a few centuries provides convincing evidence that flowering times have advanced by 4–6 days per single degree centigrade increase on average (Figure 1; Table 1; Table S1). For example, observations on flowering time in plant communities in Concord, Massachusetts, USA collected from 1852 through 2006 (Rushing and Primack, 2008), wherein temperature increased by 2.4°C, led to 7 days advancement in flowering date. Field-based experiments with annual crop plants using [CO2] and supplementary heating (temperature free-air-controlled enhancement (T-FACE); White et al., 2011), allowing wheat plants to be exposed to temperatures ranging from <0°C to >40°C, resulted in significantly early heading with increasing temperature (for details, see Figure 2 in White et al., 2011).
Figure 1. Change in flowering time (days) in plants under elevated CO2 (per 50 ppm) and high temperature (per 0.5°C). The difference in CO2 concentration (elevated vs. ambient) was divided by 50 ppm to get a numerical unit for the total change in CO2 levels. Change in flowering time (days) was then divided by the previously obtained numerical unit to get change per 50 ppm. Similarly, change in temperature (high vs. ambient) was divided by 0.5 to get a numerical unit and change in flowering time (days) was divided by respective numerical unit associated with studies referenced in Table 1 and Table S1. The bottom and the top of the box represent the 25th and 75th percentile while the band near the middle represents the 50th percentile. The whiskers represent the 5th and the 95th percentile and the dots the outliers. References provided in Table 1 and Table S1.
Table 1. Range in flowering time variation (days) under elevated CO2 and warming temperature at species to ecosystem level.
Flowering time in 40 published studies involving both crops and other plant species exposed to e[CO2] (from 350 to 1000 ppm) showed 28 cases (different species within the same study is considered a case) in which flowering time was earlier (average 8.6 days) and 12 cases in which flowering was delayed (average 5.2 days) (Figure 1; Table 1; see Table S1 for references and species). The effect of e[CO2] (680 ppm) on a grassland ecosystem using a FACE facility in California resulted in forbs flowering 2–4 days earlier, while in the dominant grass community flowering time was delayed by 2–6 days (Cleland et al., 2007). Reproductive traits in non-crop and wild species are known to respond less to e[CO2] than in crop species (Reekie et al., 1994; Jablonski et al., 2002; Kimball et al., 2002; Ainsworth and Long, 2004; see Table S1). Contrasting results have been documented showing no effect of e[CO2] on grass species in a mini-FACE experiment (Hovenden et al., 2008; Bloor et al., 2010) and similarly with maple trees (Norby et al., 2003). These differential responses could lead to changes in relative flowering times between species, thus affecting the ecosystem (Davis et al., 2010; Hulme, 2011). However, “Phenological complementarity” at an ecosystem level is known to promote coexistence of multiple species (McKane et al., 1990). Multiple environmental changes may alter the flowering time differentially in a natural landscape but the same would be more limited due to the domestication and breeding for uniformity in phenology among the crop plants.
In general, e[CO2] favors higher photosynthate (sugars and starch) accumulation in plants (Grodzinski et al., 1998; Springer and Ward, 2007). A sugar signaling metabolite trehalose-6-phosphate (T6P) showed a strong correlation (r2 = 0.94) with vegetative and shoot-apical meristem tissue sucrose levels in Arabidopsis (Wahl et al., 2013). T6P has been suggested to relay information about tissue carbohydrate availability and act as key signal for floral induction (Wahl et al., 2013). However, in Arabidopsis thaliana, the effect of e[CO2] on flowering time is the net result of the positive effect of e[CO2] on growth and a negative effect resulting from its tendency to increase leaf number, diluting the carbohydrate concentration in leaves at flowering (Johnston and Reekie, 2008). Interestingly, in A. thaliana, exposure to e[CO2] significantly advanced flowering time within a generation; however, across 15 generations, e[CO2] did not advance flowering time to the same level in each succeeding generation (Teng et al., 2009). Although the research was conducted in controlled environments, it provides clues that plants grown at e[CO2] for short-term may not evolve specific adaptations to e[CO2]. This was further confirmed by a reciprocal sowing experiment, which showed that e[CO2] did not produce detectable maternal effects on the offspring after 15 generations, indicating that e[CO2] may generate immediate change via phenotypic plasticity, but fail to produce genetic change (Teng et al., 2009).
Besides variable responses of flowering time under e[CO2] across non-crop species, studies with agricultural crops have shown an overall positive impact of e[CO2] on growth and yield. Most of this positive effect was attributed to a longer vegetative phase due to delays in flowering time under e[CO2], e.g., in pigeon pea (Sreeharsha et al., 2015), soybean (Bunce, 2015) and rice (Shimono et al., 2009). Flowering time is particularly sensitive to a variety of biotic and abiotic stresses that are expected to become more prevalent under future climates. Crops (or specific cultivars) with more flexibility to adjust flowering time to ensure vulnerable development phases escape stress under adverse environmental conditions could well determine their successful adaptation (Kazan and Lyons, 2016). Thus, molecular mechanisms regulating flowering time under a combination of e[CO2] with different biotic and abiotic stresses would help in tailoring climate resilient crops and utilize additional carbon for increasing yields under future climate.
Since e[CO2] would invariably drive temperatures higher, and with most climatic factors operating in tandem under field conditions, their combined effect on flowering time needs systematic evaluation. With a 4°C increase in temperature, flowering time was advanced by 50 and 31 days for Chenopodium album and Setaria viridis, respectively; but in combination with [CO2](1.8 times ambient [CO2]) it was 47 and 32 days, respectively (Lee, 2011). Moreover, with 22 different Asteraceae species, it was observed that e[CO2] advanced flowering by 4 days and in combination with increased temperature the flowering was advanced by an additional 3 days (Johnston and Reekie, 2008). Conversely, in several grass species it was demonstrated that e[CO2] delayed flowering by 2–7 days and high temperature (1.5°C above ambient) accelerated flowering by 2–5 days; in combination elevated [CO2] completely overcame the accelerated flowering time observed with increased temperature, i.e., a zero net change (Cleland et al., 2006). In another experiment the flowering and fruiting time of a grassland community with warming (increase in temperature +4.17°C), doubled precipitation and warming plus doubled precipitation (+4.83°C) was tested for one season (Sherry et al., 2007). Doubled precipitation had small and inconsistent effects whereas the warming plus doubled precipitation treatment was similar to warming only. This was to be expected as mean temperature varied by only 0.67°C with double precipitation and water-deficit stress has to be extreme to influence phenology (Craufurd et al., 1993). In summary, given that a doubling of [CO2] is predicted to occur by the end of the century, the observed changes in flowering time of 4–6 days or slightly more across a wide range of species are fairly modest, that is, <1 day per decade at most. It can be concluded that temperature is the major determining factor altering flowering time and ecosystem dynamics (see also Hovenden et al., 2008). There are contrasting responses between crop and non-crop or wild species, which may be associated with: (i) a combination of external factors such as elevated [CO2], nitrogen levels (Cleland et al., 2007); and (ii) due to the balance between “phenological complementarity” and resource acquisition, which further reduces the impact observed by e[CO2]. However, interactions between e[CO2] and temperature or photoperiod on flowering time can't be generalized and may be specific to a localized environment or plant species. Crops response to combined elevated CO2 and high temperature has been primarily quantified using controlled environment chambers (Madan et al., 2012) or poly tunnels (Dias de Oliveira et al., 2013, 2015a,b), wherein other interacting environmental factors such as wind speed, radiation could be considerably different compared to field conditions (Bahuguna et al., 2015). Developing economically feasible facilities to test this combined environmental change on field crops has been a major challenge and hence there is limited information on crops responses to this interaction under field conditions.
Flowering time is governed by a complex signaling network involving the regulation of environmental stimuli (i.e., photoperiod, temperature) as well as endogenous genetic traits. Until now, four major flowering pathways have been identified. The vernalization and photoperiod pathways mediate signals from the environment, the autonomous pathway monitors endogenous signals based on developmental state of the plants, and the gibberellic acid (GA) pathway forms the fourth distinctive promotive pathway (for details see Amasino, 2010; Amasino and Michaels, 2010). Although there are increasing number of studies elucidating the gene network regulating flowering, information on the impact of climate change factors (temperature, e[CO2]) on the regulation of flowering time pathways is limited.
Genetic screens have identified a group of late-flowering mutants of Arabidopsis representing the photoperiod, gibberellin and autonomous pathways. Flowering time in these mutants is affected by change in ambient temperature (16-23°C) via a thermo-sensory pathway that mediates flowering regulation through the autonomous pathway genes FCA and FVE via a FLOWERING LOCUS C (FLC)-independent pathway (Blázquez et al., 2003). Conversely, some microRNAs (miRNAs are non-coding RNAs that negatively regulate the expression of their target genes) are reported to regulate flowering time in plants under ambient temperature (Lee et al., 2010; Kim et al., 2011). Lee et al. (2010) showed that loss of SHORT VEGETATIVE PHASE (SVP) function alters the miR172 expression level, suggesting that SVP is an upstream mediator of miR172. Hence, it is hypothesized that SVP-miR172 signaling is ultimately integrated by FLOWERING LOCUS T (FT). This hypothesis is supported by early flowering of the miR172-overexpressing plants at high and optimum temperature (23 and 16°C, respectively) through up-regulation of FT expression independent of SVP. The SVP−miR172 regulatory circuit is proposed as a fine-tuning mechanism in response to changes in ambient temperature, which allows plants to modify their development toward changing temperature conditions (Lee et al., 2010). Likewise, the role of miR399 and its target gene PHOSPHATE 2 (PHO2) in flowering time regulation has been highlighted with miR399b over-expression and a loss of function allele of PHO2 resulting in early flowering under higher temperature (23°C) and long day conditions in Arabidopsis (Kim et al., 2011). The authors suggested that miR399-PHO2 module maintains phosphate homeostasis and regulates flowering time with increased expression of TWINSISTER OF FT (TSF). However, an indirect consequence of phosphate toxicity in miR399 overexpressing-pho2 mutant plants could also lead to early flowering and warrants further investigation (Kim et al., 2011; for review see Spanudakis and Jackson, 2014).
A basic helix-loop-helix transcription factor, PHYTOCHROME INTERACTING FACTOR 4 (PIF4) activates FT gene expression in Arabidopsis. Binding of PIF4 at FT promoter is temperature-dependent, with an approximately five-fold increase in binding at high temperature (27°C) compared to ambient temperature (12°C) (Kumar and Wigge, 2010). H2A.Z-nucleosome occupancy declines at higher temperatures suggesting that the presence of H2A.Z-nucleosomes is limiting PIF4 binding to the promoter of FT. A possible epigenetic modification allows removal of H2A.Z from nucleosome to allow PIF4 and FT transcription (Kumar et al., 2012). Interestingly, a recent report has documented that PIF5 along with PIF4 regulate FT expression under warm temperature (22°C) in Arabidopsis. Both PIF4 and PIF5 physically suppress phyB photoreceptor mediated suppression of CO resulting in early flowering under warm night conditions (Thines et al., 2014). Hence it can be concluded that warm night causes a phase shift in PIF4 and PIF5 accumulation in dark hours that favor early FT transcript increase during the daytime. Moreover, this pilot report indicates a possible mechanism of earlier TOA under warmer nights as documented in studies involving rice (Julia and Dingkuhn, 2013). Another temperature dependent mechanism of flowering regulation comprises two FLOWERING LOCUS M (FLM) protein splice variants (FLM-β and FLM-δ) which compete for interaction with the floral repressor SVP to regulate flowering (Pose et al., 2013). The predominant form of SVP-FLM-β complex at low temperatures prevents precocious flowering. The competing SVP- FLM-δ complex is impaired in DNA binding and acts as a dominant negative activator of flowering at higher temperatures. Apart from this, it can also be proposed that the opposing activities of two different splice variants of FLM and alternate splicing of transcription factors (which are temperature dependent), constitute a different regulatory pathway that acts in parallel to PIF4 to support the transition from vegetative to reproductive development with a change in ambient temperature (Figure 2).
Figure 2. Summary of flowering regulation by ambient temperature and e[CO2]. Solid black and red arrows represent definite positive and negative interaction, respectively whereas broken black and red arrows depict plausible positive and negative interactions, respectively. Ambient temperature regulates FT expression by different mechanisms including PIF4 dependent and independent pathways. Warmer night temperature could induce early morning flowering by accumulating PIF4, PIF5 which regulate CO mediated FT expression. Heat stress may affect flowering events by hampering carbon metabolism and sugar signaling or by inducing floral repressor HvODDSPC2. Conversely, e[CO2] may directly regulate FT expression through floral repressor FLC or alternatively, positive impact of e[CO2] on carbon metabolism and sugar signaling may induce flowering pathway genes by suppressing FLC expression. Major flowering pathways genes affected by temperature and e[CO2] are represented in the box in the center. Abbreviations: AP1, APETALA1; CO, CONSTANS; CAL, CAULIFLOWER; FLC, FLOWERING LOCUS C; FLM, FLOWERING LOCUS M; SVP, SHORT VEGETATIVE PHASE; FT, FLOWERING LOCUS T; FUL, FRUITFULL; HvODDSOC2, A MADS-box ßoral repressor; LFY, LEAFY; PIF4, PHYTOCHROME INTERACTING FACTOR 4; SOC1, SUPRESSION OF OVEREXPRESSION OF CONSTANS 1; T6P, trehalose-6-phosphate.
In Chinese narcissus, Narcissus tazetta var. chinensis, extended exposure to constant heat treatment (30°C) compared with low (15°C) and natural (ranging between 19 and 33°C) conditions promoted flower initiation and the Arabidopsis FT homolog FT-like (Narcissus FLOWERING LOCUS T1) NFT1 gene was up-regulated under higher temperature exposure (Li et al., 2013a). However, in chrysanthemums, reduced expression of floral inducer FLOWERING LOCUS T-like 3 (FTL3) coincided with delayed flowering as a consequence of high temperature (30°C) compared with control (20°C) treatment (Nakano et al., 2013). On the other hand, Hemming et al. (2012) reported progressive reproductive development in Triticum aestivum and Hordeum vulgare at higher temperature (25°C) under long days, whereas, under short days, the expression of FT-like genes was not responsive to 25°C heat treatment. A MADS-box floral repressor HvODDSOC2 that is highly expressed at high temperature in short days might contribute to the inhibition of early reproductive development, suggesting close interaction between high temperature and day-length conditions. These studies suggest that, under higher temperature, the expression of FT/FT-like genes might be species-/crop-specific and flowering induction might share common and distinct factors with those of the photoperiodic flowering pathway.
Effect of e[CO2] on flowering time has been reviewed by Springer and Ward (2007) who summarized 60 studies including 90 different crop and wild species grown under e[CO2] in controlled chambers and the field (using open top chambers and FACE) conditions. The physiological basis of e[CO2] effect on flowering time was to enhance relative growth rates, increase plant size at flowering and raise tissue sugar status (Springer and Ward, 2007). Contrasting responses of flowering time to e[CO2] among short and long day plants suggested a possible interaction of the photoperiod pathway with e[CO2] to regulate floral signaling (Reekie et al., 1994). In Arabidopsis, the sustained expression of the floral repressor gene FLC was reported to be associated with delayed flowering in the genotype that was selected for high seed yield under e[CO2] (Springer et al., 2008; Figure 2). Recently, MOTHER OF FT AND TFL1 (MFT), a homolog of FT and TERMINAL FLOWER 1 (TFL1), has been identified as a candidate gene influencing flowering time with e[CO2] (Ward et al., 2012). In comparison to the impact of temperature, the current literature on the involvement of e[CO2] influencing the expression of flowering genes is limited. Only recently, the involvement of sugars in the regulation of flowering has been reported in Arabidopsis, wherein adequate sugar levels in the vegetative tissue (leaf) and shoot apical meristem produce TREHALOSE-6-PHOSPHATE (T-6-P) as a proxy signal for floral transition and initiation under inductive environmental conditions (Wahl et al., 2013). Interestingly, Arabidopsis plants with mutation in the TREHALOSE-6-PHOSPHATE SYNTHASE gene (AT1G78580) failed to flower, showing the essential role of sugar signaling (T-6-P) in regulation of flowering time (vanDijken et al., 2004). Elevated [CO2] can induce floral transition with enhanced substrate supply through increased photosynthesis (Grodzinski et al., 1998; Springer and Ward, 2007; Figure 2). However, excess foliar sugar accumulation (beyond a threshold) under e[CO2] may delay flowering in several plant species (for review see Springer and Ward, 2007). For instance, e[CO2] delayed flowering in Arabidopsis plants with a 41 and 105% increase in foliar sucrose and starch content, respectively (Bae and Sicher, 2004), indicating differential response to foliar sugars levels below and above threshold limits. Thus, varying sensitivity to sugar concentration under e[CO2] within or across species warrants further investigation to find a links between e[CO2] and flowering competency. It is envisioned, in the near future, that the yet-to-be-identified novel regulators involved in the signaling network modulating floral initiation in response to elevated temperature and e[CO2] will facilitate understanding and identifying options to develop plants or breed crops to better adapt to changing climate.
It is essential to identify contributing candidates (either QTLs or candidate genes) influencing flowering time at the genetic level to accurately estimate phenological shifts or to select crops better adapted to changing climates. To map regions responsible for e[CO2] responsiveness, a random inbred line (RIL) population between the Arabidopsis cultivars Cvi-0 (no response) and SG (delayed flowering time), was studied and a major QTL contributing 30% of the variation in flowering time was mapped on Chromosome 1 (Ward et al., 2012). Subsequent mutant analysis resulted in the identification of MFT as a candidate gene for altered flowering time under elevated [CO2] of 700 ppm (Ward et al., 2012).
Genetic analysis of high temperature responsiveness in grapevines was studied using a bi-parental population following a growing degree day approach. This resulted in the identification of two independent QTLs responsible for variation in phenological duration to flowering. By utilizing the complete grapevine genome sequence, a number of candidate genes influencing flowering were identified including FRUITFUL, SEPALLATA, and FLC. However, VvFT on chromosome 7 and a CONSTANS-like VvCOL2 gene on chromosome 14 were the most reliable candidates identified from two of the underlying QTLs co-localizing for the flowering time (Duchêne et al., 2012). Over expression of VvFT in Arabidopsis is shown to hasten flowering (Carmona et al., 2007) while VvCOL2 is known to be associated with genetic variations of flowering time in Medicago truncatula (Pierre et al., 2010) and Medicago sativa (Herrmann et al., 2010). By employing the diverse global core collection of 473 Arabidopsis accessions and subjecting them to four simulated climatic conditions representing current and future climatic scenarios (2010, 2025, 2040, and 2055), a genome wide association analysis (GWAS) identified five independent main-effect QTLs for both days to flowering and thermal sensitivity (Li et al., 2013b). A total of just 12 and 7% of the phenotypic variation was accounted by all the five candidates SNP locations identified for flowering time and thermal sensitivity, respectively, with the polygenic background accounting for 76% of the variation. Further, a lack of association between candidate SNPs for both the above traits failed to help explain their role in the Arabidopsis distribution across the latitudinal gradient. The need to engage in more local populations to estimate the actual contributions of key genomic regions to flowering time with lesser polygenic interference is proposed. On the other hand, using 950 diverse rice accessions, classified into rice indica and japonics sub-species, it was concluded that a larger sample increased the power of detecting traits including flowering time through GWAS analysis (Huang et al., 2012). The lower phenotypic variation observed by Li et al. (2013b) could be explained by either the alteration in flowering time with increased temperature or too small population. Conversely, two independent studies have exploited the early TOA trait from O. rufipogon (Thanh et al., 2010) and O. officinalis (Ishimaru et al., 2010) and stable QTLs on chromosome 4, 5, 10 and 3, 8, respectively have been identified. The lack of co-localizing of identified QTLs indicates the role of environmental factors such as radiation, photoperiod and relative humidity, indicating a strong genotype x environment interaction as proposed by Kobayashi et al. (2010). Phenotyping for these traits in breeding programs needs to be rigorous in order to avoid or minimize artifacts of these environment interactions.
In conclusion, temperature is by far the most important climate change factor affecting flowering time, with warmer temperatures predominantly advancing flowering. The molecular pathways and the genes involved in flowering time under optimal conditions are well documented. However, information on their response to high temperature and interactions with other environmental factors such as e[CO2], water-deficit stress and photoperiod, is limited and warrants detailed investigation. More studies under realistic field conditions, such as in FACE and T-FACE experiments, are needed.
Some of the key knowledge gaps and opportunities to overcome adverse impacts of climate change on flowering time and processes include:
1. Precise and accurate predictions of changes in phenology, flowering time and time of day of flowering (TOA) using crop simulation models can be attained by: (i) employing canopy/floral tissue temperature rather than ambient air temperature, (ii) incorporating the differential impact of day and night temperature amplitude, and (iii) considering the key interactions between environmental factors such as temperature and RH (VPD).
2. Genetic outputs obtained using global core accessions have and will result in identifying genomic regions (QTLs/SNPs/candidate genes) responsible for changes in flowering time using experimental and natural temperature regimes. The applicability of the candidates identified needs validation at smaller spatial scale to demonstrate the advantage in overcoming yield losses caused due to changes in the timing of flowering related events.
3. Traits such as early-morning flowering, as demonstrated in rice, need to be explored in other crop species or plants as well. A more careful examination and documentation of TOA across landraces and wild relatives of crops could provide novel and pragmatic solutions for adapting crop production to rising temperatures.
4. Deciphering differential regulation of flowering genes by TFs such as bHLH PIFs (PIF3, PIF4, PIF5) and microRNAs that might be involved in integrating diverse environmental signals (light, temperature and possibly CO2) to regulate flower initiation and development is needed. Additionally, novel unknown regulators of flowering and their interconnections or independencies with other already known pathways under changing climates constitute future direction of research on flowering time response to environmental stimuli.
All authors listed, have made substantial, direct and intellectual contribution to the work, and approved it for publication.
The authors declare that the research was conducted in the absence of any commercial or financial relationships that could be construed as a potential conflict of interest.
We express our special thanks to the Federal Ministry for Economic Cooperation and Development, Germany, for supporting SJ and RB working on climate change-related aspects at IRRI. Bill Hardy is thanked for editing the manuscript. The third author (MD) thanks Tamil Nadu Agricultural University, India, for permitting him to take up post-doctoral research at Kansas State University, USA. Contribution no. 14-355-J from the Kansas Agricultural Experiment Station.
The Supplementary Material for this article can be found online at: http://journal.frontiersin.org/article/10.3389/fpls.2016.00913
Ainsworth, E. A., and Long, S. P. (2004). What have we learned from 15 years of free-air CO2 enrichment (FACE)? A meta-analytic review of the responses of photosynthesis, canopy properties and plant production to rising CO2. New Phytol. 165, 351–372. doi: 10.1111/j.1469-8137.2004.01224.x
Amano, T., Smithers, R. J., Sparks, T. H., and Sutherland, W. J. (2010). A 250-year index of first flowering dates and its response to temperature changes. Proc. R. Soc. Biol. Sci. 277, 2451–2457. doi: 10.1098/rspb.2010.0291
Amasino, R. (2010). Seasonal and developmental timing of flowering. Plant J. 61, 1001–1013. doi: 10.1111/j.1365-313X.2010.04148.x
Amasino, R. M., and Michaels, S. D. (2010). The timing of flowering. Plant Physiol. 154, 516–520. doi: 10.1104/pp.110.161653
Asseng, S., Ewert, F., Martre, P., Rötter, R. P., Lobell, D. B., Cammarano, D., et al. (2015). Rising temperatures reduce global wheat production. Nat. Clim. Change 5, 143–147. doi: 10.1038/nclimate2470
Bae, H. H., and Sicher, R. (2004). Changes of soluble protein expression and leaf metabolite levels in Arabidopsis thaliana grown in elevated atmospheric carbon dioxide. Field Crops Res. 90, 61–73. doi: 10.1016/j.fcr.2004.07.005
Bahuguna, R. N., and Jagadish, S. V. K. (2015). Temperature regulation of plant phenological development. Environ. Exp. Bot. 111, 83–90. doi: 10.1016/j.envexpbot.2014.10.007
Bahuguna, R. N., Jha, J., Madan, P., Shah, D., Lawas, M. L., Khetarpal, S., et al. (2015). Physiological and biochemical characterization of NERICA-L 44: a novel source of heat tolerance at the vegetative and reproductive stages in rice. Physiol. Plant. 154, 543–559. doi: 10.1111/ppl.12299
Blázquez, M. A., Ahn, J. H., and Weigel, D. (2003). A thermosensory pathway controlling flowering time in Arabidopsis thaliana. Nat. Genet. 33, 168–171. doi: 10.1038/ng1085
Bloor, J. M. G., Pichon, P., Falcimangne, R., Leadley, P., and Soussana, J. F. (2010). Effects of warming, summer drought, and CO2 enrichment on aboveground biomass production, flowering phenology, and community structure in an upland grassland ecosystem. Ecosystems. 13, 888–900. doi: 10.1007/s10021-010-9363-0
Bowers, J. E. (2007). Has climatic warming altered spring flowering date of Sonoran Desert shrubs? Southwest. Naturalist 52, 347–355. doi: 10.1894/0038-4909(2007)52[347:HCWASF]2.0.CO;2
Bunce, J. A. (2015). Elevated carbon dioxide effects on reproductive phenology and seed yield among soybean cultivars. Crop Sci. 55, 339–343. doi: 10.2135/cropsci2014.04.0273
Carmona, M. J., Calonje, M., and Martínez-Zapater, J. M. (2007). The FT/TFL1 gene family in grapevine. Plant Mol. Biol. 63, 637–650. doi: 10.1007/s11103-006-9113-z
Cho, J. (1956). Double fertilization in Oryza sativa L. and development of the endosperm with special reference to the aleurone layer. Bull. Nat. Inst. Agri. Sci. 6, 61–101.
Christensen, J. H., Hewitson, B., Busuioc, A., Chen, A., Gao, X., Held, I., et al. (2007). “Regional climate projections,” in Climate Change 2007: The Physical Science Basis. Contribution of Working Group I to the Fourth Assessment Report of the Intergovernmental Panel on Climate, eds S. Solomon, D. Qin, M. Manning, Z. Chen, M. Marquis, K. B. Averyt, et al. (Cambridge, UK; New York, NY: Cambridge University Press), 847–940.
Cleland, E. E., Chiariello, N. R., Loarie, S. R., Mooney, H. A., and Field, C. B. (2006). Diverse responses of phenology to global changes in a grassland ecosystem. Proc. Nat. Acad. Sci. U.S.A 103, 13740–13744. doi: 10.1073/pnas.0600815103
Cleland, E. E., Chuine, I., Menzel, A., Mooney, H. A., and Schwartz, M. D. (2007). Shifting plant phenology in response to global change. Trends Ecol. Evol. 22, 357–365. doi: 10.1016/j.tree.2007.04.003
Craufurd, P. Q., Flower, D. J., and Peacock, J. M. (1993). Effect of heat and drought stress on sorghum (Sorghum bicolor). I. Panicle development and leaf appearance. Exp. Agric. 29, 61–76. doi: 10.1017/S001447970002041X
Craufurd, P. Q., and Wheeler, T. R. (2009). Climate change and the flowering time of annual crops. J. Exp. Bot. 60, 2529–2539. doi: 10.1093/jxb/erp196
Crimmins, T., Crimmins, M., and Bertelsen, C. D. (2011). Onset of summer flowering in a ‘Sky Island’ is driven by monsoon moisture. New Phytol. 191, 468–479. doi: 10.1111/j.1469-8137.2011.03705.x
Dale, V. H., Joyce, L. A., McNulty, S., Neilson, R. P., Ayres, M. P., Flannigan, M. D., et al. (2001). Climate change and forest disturbances. BioScience 51, 723–734. doi: 10.1641/0006-3568(2001)051[0723:CCAFD]2.0.CO;2
Davis, S. J., Caldeira, K., and Matthews, H. D. (2010). Future CO2 emissions and climate change from existing energy infrastructure. Science 329, 1330–1333. doi: 10.1126/science.1188566
Dias de Oliveira, E., Bramley, H., Siddique, K. H. M., Henty, S., Berger, J. D., and Palta, J. A. (2013). Can elevated CO2 combined with high temperature ameliorate the effect of terminal drought in wheat? Funct. Plant Biol. 40, 160–171. doi: 10.1071/FP12206
Dias de Oliveira, E., Palta, J. A., Bramley, H., Stefanova, K., and Siddique, K. (2015b). Elevated CO2 reduced floret death in wheat under warmer average temperatures and terminal drought. Front. Plant Sci. 6:1010. doi: 10.3389/fpls.2015.01010
Dias de Oliveira, E. A., Siddique, K. H. M., Bramley, H., Stefanova, K., and Palta, J. A. (2015a). Response of wheat restricted-tillering and vigorous growth traits to variables of climate change. Glob. Chang. Biol. 21, 857–873. doi: 10.1111/gcb.12769
Doney, S. C., Ruckelshaus, M., Duffy, J. E., Barry, J. P., Chan, F., English, C. A., et al. (2012). Climate change impacts on marine ecosystems. Ann. Rev. Mar. Sci. 4, 11–37. doi: 10.1146/annurev-marine-041911-111611
Duchene, E., Butterlin, G., Dumas, V., and Merdinoglu, D. (2012). Towards the adaptation of grapevine varieties to climate change: QTLs and candidate genes for developmental stages. Theo. App. Gen. 124, 623–635. doi: 10.1007/s00122-011-1734-1
Garbutt, K., and Bazzaz, F. A. (1984). The effects of elevated CO2 on plants. New Phytol. 98, 433–446. doi: 10.1111/j.1469-8137.1984.tb04136.x
Garbutt, K., Williams, W. E., and Bazzaz, F. A. (1990). Analysis of the differential response of 5 annuals to elevated CO2 during growth. Ecology 71, 1185–1194. doi: 10.2307/1937386
Grodzinski, B., Jiao, J., and Leonardos, E. D. (1998). Estimating photosynthesis and concurrent export rates in C3 and C4 species at ambient and elevated CO2. Plant Physiol. 117, 207–215. doi: 10.1104/pp.117.1.207
Guo, Y. M., Chen, S., Nelson, M. N., Cowling, W., and Turner, N. C. (2013). Delayed water loss and temperature rise in floral buds compared with leaves of Brassica rapa subjected to a transient water stress during reproductive development. Funct. Plant Biol. 40, 690–699. doi: 10.1071/FP12335
Heinemann, A. B., Maia, A. H. N., Dourado-Neto, D., Ingram, K. T., and Hoogenboom, G. (2006). Soybean (Glycine max (L) Merr) growth and development response to CO2 enrichment under different temperatures regimes. Eur. J. Agron. 24, 52–61. doi: 10.1016/j.eja.2005.04.005
Hemming, M. N., Walford, S. A., Fieg, S., Dennis, E. S., and Trevaskis, B. (2012). Identification of high-temperature-responsive genes in cereals. Plant Physiol. 158, 439–450. doi: 10.1104/pp.111.192013
Herrmann, D., Barre, P., Santoni, S., and Julier, B. (2010). Association of a CONSTANS-LIKE gene to flowering and height in autotetraploid alfalfa. Theor. App. Gen. 121, 865–876. doi: 10.1007/s00122-010-1356-z
Hirabayashi, H., Sasaki, K., Kambe, T., Gannaban, R. B., Miras, M. A., Mendioro, M. S., et al. (2015). qEMF3, a novel QTL for the early-morning flowering trait from wild rice, Oryza officinalis, to mitigate heat stress damage at flowering in rice, O. sativa. J. Exp. Bot. 66, 1227–1236. doi: 10.1093/jxb/eru474
Hovenden, M. J., Wills, K. E., Schoor, J. K. V., Williams, A. L., and Newton, P. C. D. (2008). Flowering phenology in a species-rich temperate grassland is sensitive to warming but not elevated CO2. New Phytologist 178, 815–822. doi: 10.1111/j.1469-8137.2008.02419.x
Huang, X., Zhao, Y., Wei, X., Li, C., Wang, A., Zhao, Q., et al. (2012). Genome-wide association study of flowering time and grain yield traits in a worldwide collection of rice germplasm. Nat. Gen. 44, 32–39. doi: 10.1038/ng.1018
Hulme, P. E. (2011). Contrasting impacts of climate-driven flowering phenology on changes in alien and native plant species distributions. New Phytol. 189, 272–281. doi: 10.1111/j.1469-8137.2010.03446.x
IPCC (2007). “Summary for policy makers,” in Climate Change 2007: The Physical Science Basis. Contribution of Working Group I to the Fourth Assessment Report of the Intergovernmental Panel on Climate Change, eds S. D. Solomon, M. Qin, Z. Manning, M. Chen, M. Marquis, K. B. Avery, et al. (Cambridge, UK; New York, NY: Cambridge University Press), 1–18.
IPCC (2013). “Summary for policy makers,” in Climate Change 2013: The Physical Science Basis. Working Group I Contribution to the Fifth Assessment Report of the Intergovernmental Panel on Climate Change, eds T. F. Stocker, D. Qin, G. K. Plattner, M. M. B. Tignor, S. K. Allen, J. Boschung, et al. (Cambridge, UK; New York, NY, MA: Cambridge University Press), 1–27.
Ishimaru, T., Hirabayashi, H., Ida, M., Takai, T., San-Oh, Y. A., Yoshinaga, S., et al. (2010). A genetic resource for early-morning flowering trait of wild rice Oryza officinalis to mitigate high temperature-induced spikelet sterility at anthesis. Ann. Bot. 106, 515–520. doi: 10.1093/aob/mcq124
Jablonski, L. M., Wang, X., and Curtis, P. S. (2002). Plant reproduction under elevated CO2 conditions: a meta-analysis of reports on 79 crop and wild species. New Phytol. 156, 9–26. doi: 10.1046/j.1469-8137.2002.00494.x
Jagadish, S. V. K., Cairns, J. E., Kumar, A., Somayanda, I. M., and Craufurd, P. Q. (2011). Does susceptibility to heat stress confound screening for drought tolerance? Funct. Plant Biol. 38, 261–269. doi: 10.1071/FP10224
Jagadish, S. V. K., Muthurajan, R., Oane, R., Wheeler, T. R., Heuer, S., Bennett, J., et al. (2010). Physiological and proteomic approaches to dissect reproductive stage heat tolerance in rice (Oryza sativa L). J. Exp. Bot. 61, 143–156. doi: 10.1093/jxb/erp289
Jain, M., Chourey, P. S., Boote, K. J., and Allen, L. H. Jr. (2010). Short-term high temperature growth conditions during vegetative-to-reproductive phase transition irreversibly compromise cell wall invertase-mediated sucrose catalysis and microspore meiosis in grain sorghum (Sorghum bicolor). J. Plant Physiol. 167, 578–582. doi: 10.1016/j.jplph.2009.11.007
Johnston, A., and Reekie, E. (2008). Regardless of whether rising atmospheric carbon dioxide levels increase air temperature, flowering phenology will be affected. Int. J. Plant Sci. 169, 1210–1218. doi: 10.1086/591978
Julia, C., and Dingkuhn, M. (2012). Variation in time of day of anthesis in rice in different climatic environments. Euro. J. Agron. 43, 166–174. doi: 10.1016/j.eja.2012.06.007
Julia, C., and Dingkuhn, M. (2013). Predicting temperature induced sterility of rice spikelets requires simulation of crop generated microclimate. Euro. J. Agron. 49, 50–60. doi: 10.1016/j.eja.2013.03.006
Kakani, V. G., Reddy, K. R., Koti, S., Wallace, T. P., Prasad, P. V. V., Reddy, V. R., et al. (2005). Differences in in vitro pollen germination and pollen tube growth of cotton cultivars in response to high temperature. Ann. Bot. 96, 59–67. doi: 10.1093/aob/mci149
Kazan, K., and Lyons, R. (2016). The link between flowering time and stress tolerance. J. Exp. Bot. 67, 47–60. doi: 10.1093/jxb/erv441
Kim, W., Ahn, H. J., Chiou, T. J., and Ahn, J. H. (2011). The role of the miR399-PHO2 module in the regulation of flowering time in response to different ambient temperatures in Arabidopsis thaliana. Mol. Cells 32, 83–88. doi: 10.1007/s10059-011-1043-1
Kimball, B. A., Kobayashi, K., and Bindi, M. (2002). Responses of agricultural crops to Free-Air CO2 Enrichment. Adv. Agron. 77, 293–368. doi: 10.1016/S0065-2113(02)77017-X
Kobayashi, K., Matsui, T., Yoshimoto, M., and Hasegawa, T. (2010). Effects of temperature, solar radiation, and vapor-pressure deficit on flower opening time in rice. Plant Produc. Sci. 13, 21–28. doi: 10.1626/pps.13.21
Kumar, S., Chaitanya, B. S., Ghatty, S., and Reddy, A. R. (2014). Growth, reproductive phenology and yield responses of a potential biofuel plant, Jatropha curcas grown under projected 2050 levels of elevated CO2. Physiol. Plant. 152, 501–519. doi: 10.1111/ppl.12195
Kumar, S. V., Lucyshyn, D., Jaeger, K. E., Alós, E., Alvey, E., Harberd, N. P., et al. (2012). Transcription factor PIF4 controls the thermosensory activation of flowering. Nature 484, 242–245. doi: 10.1038/nature10928
Kumar, S. V., and Wigge, P. A. (2010). H2A. Z containing nucleosomes mediate the thermosensory response in Arabidopsis. Cell 140, 136–147. doi: 10.1016/j.cell.2009.11.006
Lee, H., Yoo, S. J., Lee, J. H., Kim, W., Yoo, S. K., Fitzgerald, H., et al. (2010). Genetic framework for flowering-time regulation by ambient temperature-responsive miRNAs in Arabidopsis. Nucleic Acids Res. 38, 3081–3093. doi: 10.1093/nar/gkp1240
Lee, J. S. (2011). Combined effect of elevated CO2 and temperature on the growth and phenology of two annual C3 and C4 weedy species. Agri. Ecosys. Environ. 140, 484–491. doi: 10.1016/j.agee.2011.01.013
Leishman, M. R., Sanbrooke, K. J., and Woodfin, R. M. (1999). The effects of elevated CO2 and light environment on growth and reproductive performance of four annual species. New Phytol. 144, 455–462. doi: 10.1046/j.1469-8137.1999.00544.x
Li, X. F., Jia, L. Y., Xu, J., Deng, X. J., Wang, Y., Zhang, W., et al. (2013a). FT-like NFT1 gene may play a role in flower transition induced by heat accumulation in Narcissus tazetta var. Chinensis. Plant Cell Physiol. 54, 270–281. doi: 10.1093/pcp/pcs181
Li, Y., Cheng, R., Spokas, K. A., Palmer, A. A., and Borevitz, J. O. (2013b). Genetic variation for life history sensitivity to seasonal warming in Arabidopsis thaliana. Genetics 196, 569–577. doi: 10.1534/genetics.113.157628
Linkosalo, T., Hakkinen, R., Terhivuo, J., Tuomenvirta, H., and Hari, P. (2009). The time series of flowering and leaf bud burst of boreal trees (1846-2005) support the direct temperature observations of climatic warming. Agricul. Forest Meteorol. 149, 453–461. doi: 10.1016/j.agrformet.2008.09.006
Liu, Y., Mu, J., Niklas, K. J., Li, G., and Sun, S. (2012). Global warming reduces plant reproductive output for temperate multi-inflorescence species on the Tibetan plateau. New Phytol. 195, 427–436. doi: 10.1111/j.1469-8137.2012.04178.x
Lobell, D., Hammer, G., McLean, G., Messina, C., Roberts, M., and Schlenker, W. (2013). The critical role of extreme heat for maize production in the United States. Nat. Clim. Chang. 3, 497–501. doi: 10.1038/nclimate1832
Lopes, M. S., and Reynolds, M. P. (2010). Partitioning of assimilates to deeper roots is associated with cooler canopies and increased yield under drought in wheat. Funct. Plant Biol. 37, 147–156. doi: 10.1071/FP09121
Luedeling, E., Girvetz, E. H., Semenov, M. A., and Brown, P. H. (2011). Climate change affects winter chill for temperate fruit and nut trees. PLoS ONE 6:e20155. doi: 10.1371/journal.pone.0020155
Madan, P., Jagadish, S. V. K., Craufurd, P. Q., Fitzgerald, M., Lafarge, T., and Wheeler, T. R. (2012). Effect of elevated CO2 and high temperature on seed-set and grain quality of rice. J. Exp. Bot. 63, 3843–3852. doi: 10.1093/jxb/ers077
Mahmud, A., Hossain, M. M., Karim, M. A., Mian, M. A. K., Zakaria, M., and Kadian, M. S. (2016). Plant water relations and canopy temperature depression for assessing water stress tolerance of potato. Ind. J. Plant Physiol. 21, 56–63. doi: 10.1007/s40502-015-0202-3
Marc, J., and Gifford, R. M. (1984). Floral initiation in wheat, sunflower, and sorghum under carbon dioxide enrichment. Can. J. Bot. 62, 9–14. doi: 10.1139/b84-002
Matsui, T., Kobayasi, K., Yoshimoto, M., and Hasegawa, T. (2007). Stability of rice pollination in the field under hot and dry conditions in the Riverina region of New South Wales, Australia. Plant Produc. Sci. 10, 57–63. doi: 10.1626/pps.10.57
McKane, R. B., Grigal, D. F., and Russelle, M. P. (1990). Spatiotemporal differences in 15N uptake and the organization of an old-field plant community. Ecology 71, 1126–1132. doi: 10.2307/1937380
Miller-Rushing, A. J., and Primack, R. B. (2008). Global warming and flowering times in Thoreau's concord: a community perspective. Ecology 89, 332–341. doi: 10.1890/07-0068.1
Nakano, Y., Higuchi, Y., Sumitomo, K., and Hisamatsu, T. (2013). Flowering retardation by high temperature in chrysanthemums: involvement of FLOWERING LOCUS T-like 3 gene repression. J. Exp. Bot. 64, 909–920. doi: 10.1093/jxb/ers370
Norby, R. J., Hartz-Rubin, J. S., and Verbrugge, M. J. (2003). Phenological responses in maple to experimental atmospheric warming and CO2 enrichment. Glob. Change Biol. 9, 1792–1801. doi: 10.1111/j.1365-2486.2003.00714.x
Olesen, J. E., Børgesen, C. D., Elsgaard, L., Palosuo, T., Rötter, R. P., Skjelvåg, A. O., et al. (2012). Changes in time of sowing, flowering and maturity of cereals in Europe under climate change. Food Addit. Contam. A. 29, 1527–1542. doi: 10.1080/19440049.2012.712060
Peng, S., Huang, J., Sheehy, J. E., Laza, R. C., Visperas, R. M., Zhong, X., et al. (2004). Rice yields decline with higher night temperature from global warming. Proc. Natl. Acad. Sci. U.S.A. 101, 9971–9975. doi: 10.1073/pnas.0403720101
Pierre, J. B., Bogard, M., Herrmann, D., Huyghe, C., and Julier, B. (2010). A CONSTANS-like gene candidate that could explain most of the genetic variation for flowering date in Medicago truncatula. Mol. Breed. 28, 25–35. doi: 10.1007/s11032-010-9457-6
Pose, D., Verhage, L., Ott, F., Yant, L., Mathieu, J., Angenent, G. C., et al. (2013). Temperature-dependent regulation of flowering by antagonistic FLM variants. Nature 21, 414–417. doi: 10.1038/nature12633
Potvin, C., and Strain, B. R. (1985). Effects of CO2 enrichment and temperature on growth in two C4 weeds, Echinochloa crusgalli and Eleusine indica. Can. J. Bot. 63, 1495–1499. doi: 10.1139/b85-206
Reekie, E. G., and Bazzaz, F. A. (1991). Phenology and growth in four annual species grown in ambient and elevated CO2. Can. J. Bot. 69, 2475–2481. doi: 10.1139/b91-307
Reekie, J. Y. C., Hicklenton, P. R., and Reekie, E. G. (1994). Effects of elevated CO2 on time of flowering in four short-day and four long-day species. Can. J Bot. 72, 533–538. doi: 10.1139/b94-071
Reynolds, M. P., Pierre, C. S., Saad, A. S. I., Vargas, M., and Condon, A. G. (2007). Evaluating potential genetic gains in wheat associated with stress-adaptive trait expression in elite genetic resources under drought and heat stress. Crop Sci. 47, 172–189. doi: 10.2135/cropsci2007.10.0022IPBS
Rushing, A. J. M., and Primack, R. B. (2008). Global warming and flowering times in Thoreau's concord: a community perspective. Ecology 89, 332–341. doi: 10.1890/07-0068.1
Rusterholz, H. P., and Erhardt, A. (1998). Effects of elevated CO2 on flowering phenology and nectar production of nectar plants important for butterflies of calcareous grasslands. Oecologia 113, 341–349. doi: 10.1007/s004420050385
Seneweera, S., Blakeney, A., Milham, P., Basra, A. S., Barlow, E. W. R., and Conroy, J. (1994). Influence of elevated CO2 and phosphorus nutrition on the growth and yield of a short-duration rice (Oryza sativa L. cv. Jarrah). Aus. J. Plant Physiol. 21, 281–292. doi: 10.1071/PP9940281
Sheehy, J. E., Mabilangan, A. E., Dionora, M. J. A., and Pablico, P. P. (2007). Time of day of flowering in wild species of the genus oryza . Int. Rice Res. Newsl. 32, 12–13.
Sherry, R. A., Zhou, X., Gu, S., Arnone, J. A., Schimel, D. S., Verburg, P. S., et al. (2007). Divergence of reproductive phenology under climate warming. Proc. Natl. Acad. Sci. U.S.A. 104, 198–202. doi: 10.1073/pnas.0605642104
Shi, W., Muthurajan, R., Rahman, H., Selvam, J., Peng, S., Zou, Y., et al. (2013). Source–sink dynamics and proteomic reprogramming under elevated night temperature and their impact on rice yield and grain quality. New Phytol. 197, 825–837. doi: 10.1111/nph.12088
Shimono, H., Hasegawa, T., Moriyama, M., Fugimura, S., and Nagata, T. (2005). Modeling spikelet sterility induced by low temperature in rice. Agron. J. 97, 1524–1536. doi: 10.2134/agronj2005.0043
Shimono, H., Okada, M., Yamakawa, Y., Nakamura, H., Kobayashi, K., and Hasegawa, T. (2009). Genotypic variation in rice yield enhancement by elevated CO2 relates to growth before heading, and not to maturity group. J. Exp. Bot. 60, 523–532. doi: 10.1093/jxb/ern288
Spanudakis, E., and Jackson, S. (2014). The role of microRNAs in the control of flowering time. J. Exp. Bot. 65, 365–380. doi: 10.1093/jxb/ert453
Springer, C. J., Orozco, R., Kelly, J. K., and Ward, J. K. (2008). Elevated CO2 influences the expression of floral-initiation genes in Arabidopsis thaliana. New Phytol. 178, 63–67. doi: 10.1111/j.1469-8137.2008.02387.x
Springer, C. J., and Ward, J. K. (2007). Flowering time and elevated atmospheric CO2. New Phytol. 176, 243–255. doi: 10.1111/j.1469-8137.2007.02196.x
Sreeharsha, R. V., Sekhar, K. M., and Reddy, A. R. (2015). Delayed flowering is associated with lack of photosynthetic acclimation in Pigeon pea (Cajanus cajan L.) grown under elevated CO2. Plant Sci. 231, 82–93. doi: 10.1016/j.plantsci.2014.11.012
Steinmeyer, F. T., Lukac, M., Reynolds, M. P., and Jones, H. E. (2013). Quantifying the relationship between temperature regulation in the ear and floret development stage in wheat (Triticum aestivum L.) under heat and drought stress. Funct. Plant Biol. 40, 700–707. doi: 10.1071/FP12362
Takahashi, N., Isogai, A., Ling, P. P., Kato, Y., and Kurata, K. (2008). Effects of elevated atmospheric carbon dioxide concentration on silica deposition in rice (Oryza sativa L.) panicle. Plant Produc. Sci. 11, 307–315. doi: 10.1626/pps.11.307
Talukder, A., McDonald, G. K., and Gill, G. S. (2013). Effect of short-term heat stress prior to flowering and at early grain set on the utilization of water-soluble carbohydrate by wheat genotypes. Field Crops Res. 147, 1–11. doi: 10.1016/j.fcr.2013.03.013
Teng, N., Jin, B., Wang, Q., Hao, H., and Ceulemans, R. (2009). No detectable maternal effects of elevated CO2 on Arabidopsis thaliana over 15 generations. PLoS ONE 4:e6035. doi: 10.1371/journal.pone.0006035
Thanh, P. T., Phan, P. D. T., Ishikawa, R., and Ishii, T. (2010). QTL analysis for flowering time using backcross population between Oryza sativa nipponbare and O. rufipogon. Genes Genetic Syst. 85, 273–279. doi: 10.1266/ggs.85.273
Thines, B. C., Youn, Y., Duarte, M. I., and Harmon, F. G. (2014). The time of day effects of warm temperature on flowering time involve PIF4 and PIF5. J. Exp. Bot. 65, 1141–1151. doi: 10.1093/jxb/ert487
Tian, X., Matsui, T., Li, S., Yoshimoto, M., Kobayasi, K., and Hasegawa, T. (2010). Heat-induced floret sterility of hybrid rice (Oryza sativa L.) cultivars under humid and low wind conditions in the field of Jianghan Basin, China. Plant Produc. Sci. 13, 243–251. doi: 10.1626/pps.13.243
vanDijken, A. J. H., Schluepmann, H., and Smeekens, S. C. M. (2004). Arabidopsis trehalose-6-phosphate synthase 1 is essential for normal vegetative growth and transition to flowering. Plant Physiol. 135, 969–977. doi: 10.1104/pp.104.039743
Vose, R. S., Easterling, D. R., and Gleason, B. (2005). Maximum and minimum temperature trends for the globe: an update through 2004. Geophys. Res. Lett. 32, L23822. doi: 10.1029/2005GL024379
Wahl, V., Ponnu, J., Schlereth, A., Arrivault, S., Langenecker, T., Franke, A., et al. (2013). Regulation of flowering by trehalose-6-phosphate signaling in Arabidopsis thaliana. Science 339, 704–707. doi: 10.1126/science.1230406
Ward, J. K., Roy, D. S., Chatterjee, I., Bone, C. R., Springer, C. J., and Kelly, J. K. (2012). Identification of a major QTL that alters flowering time at elevated [CO2] in Arabidopsis thaliana. PLoS ONE 7:e49028. doi: 10.1371/journal.pone.0049028
Welch, J. R., Vincent, J. R., Auffhammer, M., Moya, P. F., Dobermann, A., and Dawe, D. (2010). Rice yields in tropical/subtropical Asia exhibit large but opposing sensitivitiesto minimum and maximum temperatures. Proc. Natl. Acad. Sci. U.S.A. 107, 14562–14567. doi: 10.1073/pnas.1001222107
White, J. W., Kimball, B. A., Wall, G. W., Ottman, M. J., and Hunt, L. A. (2011). Responses of time of anthesis and maturity to sowing dates and infrared warming in spring wheat. Field Crops Res. 124, 213–222. doi: 10.1016/j.fcr.2011.06.020
Keywords: climate change, flowering time, flowering regulation, high temperature, elevated CO2, tissue temperature
Citation: Jagadish SVK, Bahuguna RN, Djanaguiraman M, Gamuyao R, Prasad PVV and Craufurd PQ (2016) Implications of High Temperature and Elevated CO2 on Flowering Time in Plants. Front. Plant Sci. 7:913. doi: 10.3389/fpls.2016.00913
Received: 25 August 2015; Accepted: 09 June 2016;
Published: 27 June 2016.
Edited by:
Vasileios Fotopoulos, Cyprus University of Technology, CyprusReviewed by:
Helen Bramley, The University of Sydney, AustraliaCopyright © 2016 Jagadish, Bahuguna, Djanaguiraman, Gamuyao, Prasad and Craufurd. This is an open-access article distributed under the terms of the Creative Commons Attribution License (CC BY). The use, distribution or reproduction in other forums is permitted, provided the original author(s) or licensor are credited and that the original publication in this journal is cited, in accordance with accepted academic practice. No use, distribution or reproduction is permitted which does not comply with these terms.
*Correspondence: Peter Q. Craufurd, cC5jcmF1ZnVyZEBjZ2lhci5vcmc=
†Present Address: Rico Gamuyao, Bioscience and Biotechnology Center, Nagoya University, Nagoya, Japan
‡These authors have contributed equally to this work.
Disclaimer: All claims expressed in this article are solely those of the authors and do not necessarily represent those of their affiliated organizations, or those of the publisher, the editors and the reviewers. Any product that may be evaluated in this article or claim that may be made by its manufacturer is not guaranteed or endorsed by the publisher.
Research integrity at Frontiers
Learn more about the work of our research integrity team to safeguard the quality of each article we publish.