- 1Mendel Centre for Plant Genomics and Proteomics, Central European Institute of Technology, Masaryk University, Brno, Czech Republic
- 2Laboratory of Functional Genomics and Proteomics, National Centre for Biomolecular Research, Faculty of Science, Masaryk University, Brno, Czech Republic
- 3Institute of Biophysics, Academy of Sciences of the Czech Republic, v.v.i., Brno, Czech Republic
Telomeres, as physical ends of linear chromosomes, are targets of a number of specific proteins, including primarily telomerase reverse transcriptase. Access of proteins to the telomere may be affected by a number of diverse factors, e.g., protein interaction partners, local DNA or chromatin structures, subcellular localization/trafficking, or simply protein modification. Knowledge of composition of the functional nucleoprotein complex of plant telomeres is only fragmentary. Moreover, the plant telomeric repeat binding proteins that were characterized recently appear to also be involved in non-telomeric processes, e.g., ribosome biogenesis. This interesting finding was not totally unexpected since non-telomeric functions of yeast or animal telomeric proteins, as well as of telomerase subunits, have been reported for almost a decade. Here we summarize known facts about the architecture of plant telomeres and compare them with the well-described composition of telomeres in other organisms.
Telomeres As Nucleoprotein Structures
Telomeres are nucleoprotein structures at the ends of eukaryotic chromosomes that protect linear chromosomes against damage by endogenous nucleases and erroneous recognition as unrepaired chromosomal breaks. It is now known that telomeric structures are formed by telomeric DNA, histone octamers, and a number of proteins that bind telomeric DNA, either directly or indirectly, and together, form the protein telomere cap (Fajkus and Trifonov, 2001; de Lange, 2005; Bianchi and Shore, 2008; Sfeir, 2012). The telomeric cap proteins of diverse organisms are less conserved than one might expect. Even within a single taxonomic class, such as mammals, telomeric proteins display less conservation than other chromosomal proteins (Linger and Price, 2009). On the other hand, in many plant families, whole-genome duplication events have occurred, resulting in a multitude of genomic changes, such as deletions of large fragments of chromosomes, silencing of duplicate genes, and recombining of homologous chromosomal segments, as was shown, e.g., in crucifer species (Mandakova and Lysak, 2008). Polyploidy can result in increased numbers of genes of the same family (Taylor and Raes, 2004; He and Zhang, 2005; Freeling, 2009), which may show sub-functionalization, neo-functionalization, and partial or full redundancy and complicates assignment of an actual and specific function for individual proteins in vivo. Gene duplications and losses in plant phylogeny can be traced also in telomere associated protein families (e.g., in Arabidopsis thaliana: single myb histone (SMH) family, TRF-like (TRFL) family, or Pot1-like family) (Nelson et al., 2014; Beilstein et al., 2015).
In land plants, the telomere is mostly composed of Arabidopsis-type TTTAGGG repeats (Richards and Ausubel, 1988; Figure 1A). Known exceptions are species in the order Asparagales, starting from divergence of the Iridaceae family, which shares the human-type telomeric repeat (TTAGGG; probably caused by a mutation that altered the RNA template subunit of telomerase ∼80 Mya; Adams et al., 2001; Weiss and Scherthan, 2002; Sykorova et al., 2003). The human-type telomere is also shared by species of the Allioideae subfamily, except for the Allium genus (Sykorova et al., 2006), where novel telomeric sequence (CTCGGTTATGGG) was recently described (Fajkus et al., 2016). An unusual telomeric motif (TTTTTTAGGG) was found in the family Solanaceae, in Cestrum elegans and related species (Peska et al., 2015). Also some of the species from the carnivorous genus Genlisea display, instead normal Arabidopsis-type of telomere, two intermingled sequence variants (TTCAGG and TTTCAGG; Tran et al., 2015).
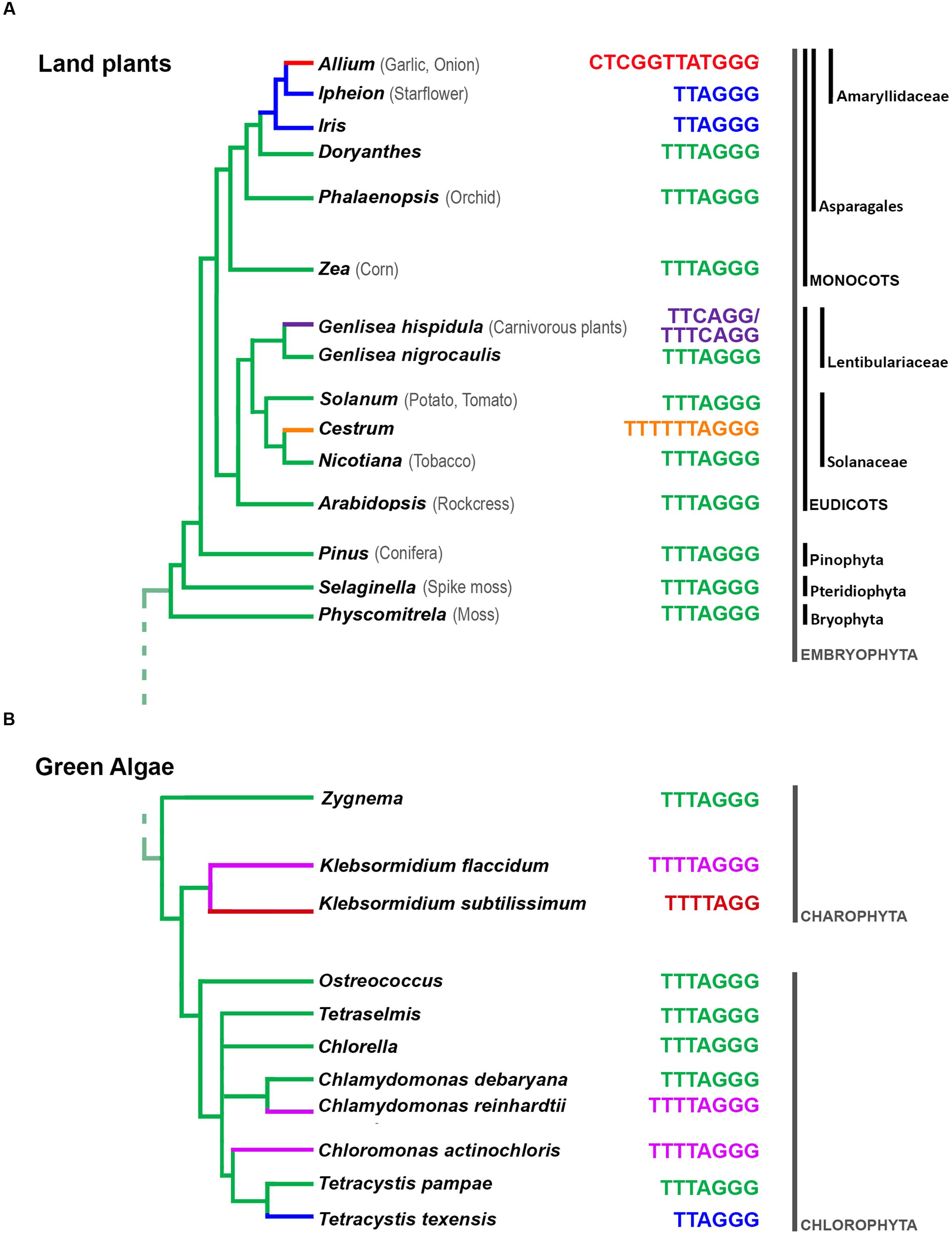
FIGURE 1. Summary of current knowledge on telomere DNA diversity in land plants (A) and green algae (B). The prevalent plant telomeric sequence motif TTTAGGG was first described in A. thaliana (Richards and Ausubel, 1988). Divergent telomeric sequences have been observed in Asparagales (Sykorova et al., 2003), in Cestrum spp. (Peska et al., 2015), in Genlisea (Tran et al., 2015), or in Allium (Fajkus et al., 2016). While Arabidopsis-type telomeric sequence is dominant in “green lineages” of algae Chlorophyta and Streptophyta, this ancestral motif was replaced several times with a novel motifs (reviewed in Fulnečková et al., 2015). (The relationships between families and genera are adapted from the schematic phylogenetic tree presented in Fulnečková et al., 2015 and Fajkus et al., 2016.)
Moreover, across the Plantae kingdom, outside of land plants but including red algae, green algae, and Glaucophytes (Koonin, 2010), telomere types also vary (Figure 1B). For example, in algae, in addition to the Arabidopsis-type of telomeric repeat, the Chlamydomonas-type (TTTTAGGG), human-type (TTAGGG), and a novel TTTTAGG repeat have been described (Fulnecková et al., 2013; Fulnečková et al., 2015).
The length of plant telomeric DNA at a single chromosomal arm can be as small as 500 base pairs (bp) in Physcomitrella patens (Shakirov et al., 2010; Fojtova et al., 2015), as long as 160 kb in Nicotiana tabacum (Fajkus et al., 1995), or 200 kb in Nicotiana sylvestris (Kovarik et al., 1996). Besides the remarkable variation in telomere lengths among diverse plant genera or orders, telomere lengths can also vary at the level of the species or ecotypes: e.g., Arabidopsis telomeres range from 1.5 to 9 kb, depending on the ecotype. Also in the long-living organism Betula pendula, telomeres in different genotypes varied from a minimum length of 5.9–9.6 kb to a maximum length of 15.3–22.8 kb (Shakirov and Shippen, 2004; Maillet et al., 2006; Aronen and Ryynanen, 2014).
Since telomeric DNA serves as a landing pad for a set of proteins, the total length or composition of telomeric tracts could markedly affect the number or selection of telomere-associated proteins and subsequently influence telomere packaging, structural transitions, or launch various biochemical pathways (see below).
Nuclear Localization and Dynamics of Telomeres
In some species during interphase, telomeres, and centromeres could be located at opposite sides of the nucleus, at the nuclear periphery, in limited regions or clusters; this is known as the Rabl organization (Rabl, 1885; for review, see Cowan et al., 2001). The Rabl organization (Wen et al., 2012) was observed in wheat, rye, barley, and oats. Other plant species, such as maize (Zea mays) and sorghum (Sorghum bicolor), despite having fairly large genomes, are not known to exhibit the Rabl configuration (Dong and Jiang, 1998). A recent study among Brachypodium species revealed a positive correlation between Rabl configuration and an increase in DNA content (resulting from replication) and a negative influence of increasing nuclear elongation (Idziak et al., 2015). A rosette-like organization of chromosomes in interphase nuclei was observed in Arabidopsis: telomeres show persistent clustering at the nucleolus while centromeres do not cluster (Armstrong et al., 2001; Tiang et al., 2012). Moreover, during early meiotic prophase, at the leptotene–zygotene transition, telomeres of most plant species cluster to form a bouquet (Bass et al., 1997; Martinez-Perez et al., 1999; Cowan et al., 2002; Corredor and Naranjo, 2007; Higgins et al., 2012; Phillips et al., 2012). Arabidopsis belongs to a small group of species that do not form telomeric bouquets (Armstrong et al., 2001).
Chromatin attachment to the inner nuclear membrane in plants, as well as in other species, is mediated by a well conserved multi-protein complex gathered around SUN (Sad1-UNC-84 homology)-KASH (Klarsicht, ANC-1, and Syne homology) proteins [respectively AtSUN-AtSINE (SUN domain-interacting NE proteins) in A. thaliana; Starr et al., 2001; Zhou et al., 2014; Tamura et al., 2015]. In fission and budding yeasts, interactions during meiosis between telomeres and the nuclear envelope, via interactions between SUN domain proteins and telomere-binding proteins, was described: in Saccharomyces cerevisiae SUN-domain protein yMps3 (monopolar spindle protein 3) is needed for yKu80-mediated telomeric chromatin anchoring (Schober et al., 2009), while in Schizosaccharomyces pombe, interactions between telomeric protein pRap1 (repressor activator protein 1) and pSUN proteins are mediated by pBqt1 and pBqt2 (telomere bouquet protein 1 and 2; Chikashige et al., 2006). The tethering of human telomeres to the nuclear matrix was proposed to depend on an isoform of telomere repeat binding factor 1 (TRF1) interacting partner (hTIN2), named hTIN2L (Kaminker et al., 2009), or an A-type lamin (Ottaviani et al., 2008; for review, see Giraud-Panis et al., 2013). Various homologs of SUN domain proteins were identified in Arabidopsis or in maize. In Arabidopsis, they are also localized to the inner nuclear membrane in somatic cells (Graumann et al., 2010; Tamura et al., 2015), however, homologs of Bqt proteins or TIN2 proteins have not been found in plants and their sequences are poorly conserved.
Telomeres are processed by a telomere-specific machinery that includes telomerase and its regulatory units, as well as nucleases, as exemplified by the exonuclease 1 (AtEXO1) ortholog in Arabidopsis (Kazda et al., 2012; Derboven et al., 2014). In plants, as well as in most of other kingdoms, replication of chromosomal ends results in single-stranded 3′ DNA protrusions (G-overhangs) after degradation of the last RNA primer at the 5′ terminus of a nascent strand. In Silene latifolia or A. thaliana, relatively short (20–30 nucleotides) G-overhangs were detected. Moreover, half of the Silene and Arabidopsis telomeres showed no overhangs or overhangs less than 12 nucleotides in length (Riha et al., 2000; Kazda et al., 2012). These G-overhangs are also thought to be required for chromosome end protection by forming secondary DNA structures such as t-loops (reviewed in Tomaska et al., 2009). Although formation of t-loop structures was demonstrated among plants only in the garden pea (Pisum sativum; Cesare et al., 2003), it is believed that excision from a t-loop in Arabidopsis may result in t-circle formation and in telomere rapid deletion (Watson and Shippen, 2007). In tobacco cell culture, knockdown of one of three human hnRNP homologs, named NgGTBP1 (G-strand specific single-stranded telomere-binding protein 1), led to frequent formation of extrachromosomal t-circles, inhibition of single-stranded invasion into double-stranded telomeric DNA and the loss of protection of telomeres against inter-telomeric recombination (Lee and Kim, 2010, 2013).
As well as in humans, mouse, or Caenorhabditis (Uringa et al., 2011; Vannier et al., 2012), the regulator of telomere elongation helicase 1 (AtRTEL1) plays a putative role in Arabidopsis in the destabilization of DNA loop structures such as t-loops or d-loops (Recker et al., 2014). However, a substantial portion of telomeres in Arabidopsis does not apparently undergo nucleolytic resection, and 3′ ends produced by leading-strand replication remain blunt-ended (Riha et al., 2000). It is believed that blunt-ends in Arabidopsis are specifically recognized and protected by the AtKu70/80 heterodimer although in situ localization of Ku to telomeres remains elusive (Kazda et al., 2012).
Proteins Associated with Telomeric DNA
Telomere-associated proteins can regulate lengths of telomere tracts by modulating access of telomerase or affecting conventional DNA replication machinery. In mammals, telomeric DNA associates with a six-protein complex called shelterin. The specific telomeric dsDNA binding is mediated by TRF1 and TRF2 (Broccoli et al., 1997; Court et al., 2005), through their Myb-like domain with an LKDKWRT amino acid motif that is also conserved in other telobox binding proteins, not only in mammals but also in plants (Bilaud et al., 1996; Feldbrugge et al., 1997). A bridge between proteins directly associated with DNA—TRF1, TRF2, and ssDNA binding protein Pot1 (Protection of telomeres 1)—is mediated by TIN2 and the oligosaccharide/oligonucleotide binding (OB)-fold domain of TPP1 (TINT1, PTOP, PIP1) protein (for review see Schmidt and Cech, 2015; Lazzerini-Denchi and Sfeir, 2016). Moreover, protein Rap1, the last component of shelterin, interacts with TRF2 (Arat and Griffith, 2012) and modulates its recruitment to telomeric DNA (Janouskova et al., 2015). A schematic model of mammalian telomere-associated proteins (Figure 2A) and a proposed model of the telomeric complex in A. thaliana (Figure 2B) summarizes recent knowledge in mammalian and plant telomere biology and provides a clear comparison of conserved structures at chromosome termini. In addition, a general overview of telomere-associated proteins that have been described in plants is given in Table 1. Detailed description of telomeric and putative telomeric dsDNA and ssDNA binding proteins from A. thaliana is shown in Table 2.
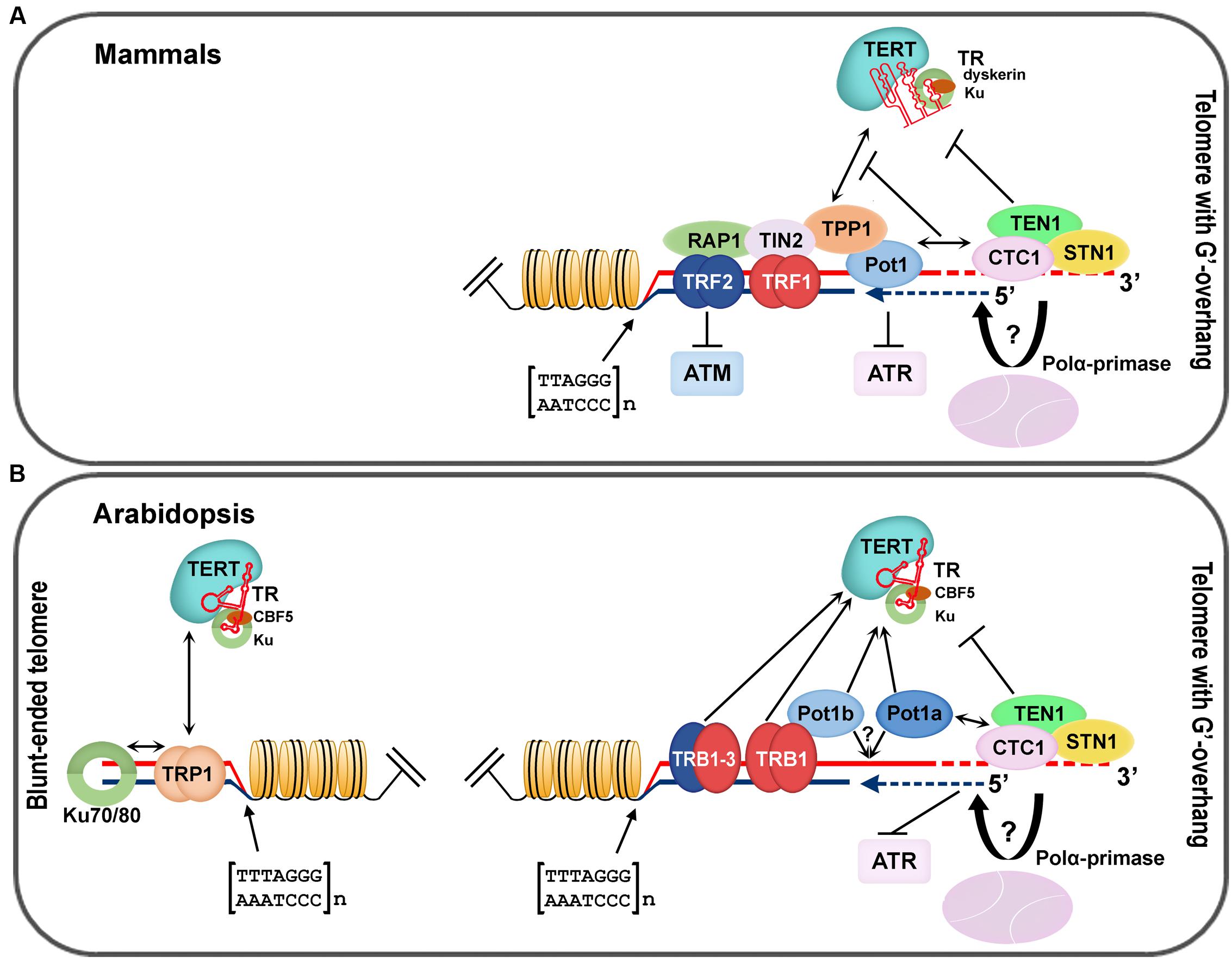
FIGURE 2. Nucleoprotein complexes associated with mammalian and A. thaliana telomeres. (A) Mammalian shelterin proteins (TRF1/2, Rap1, TIN2, TPP, and Pot1) modulate access to the telomerase complex and the ATR/ATM dependent DNA damage response pathway. The CST complex (CTC1–STN1–TEN1) affects telomerase and DNA polymerase α recruitment to the chromosomal termini, and thus coordinates G-overhang extension by telomerase with fill-in synthesis of the complementary C-strand (blue dashed line; figure adopted from Chen and Lingner, 2013). (B) Arabidopsis TRB1, 2 and 3 interact with the telomeric sequence due to the same Myb-like binding domain as mammalian TRF1/2 (Marian et al., 2003; Kuchar and Fajkus, 2004; Schrumpfova et al., 2004). TRB proteins interact with TERT and Pot1b and when localized at chromosomal ends they are eligible to function as components of the plant shelterin complex, mainly at telomeres with a G-overhang (Schrumpfova et al., 2008, 2014). An evolutionary conserved CST complex is suggested to coordinate the unique requirements for efficient replication of telomeric DNA in plants as well as in other organisms (Derboven et al., 2014). Blunt-ended telomeres are specifically recognized and protected by the KU70/80 heterodimer that directly interacts with TRP1, and by extension, with TERT (Kuchar and Fajkus, 2004; Kazda et al., 2012; Schrumpfova et al., 2014).
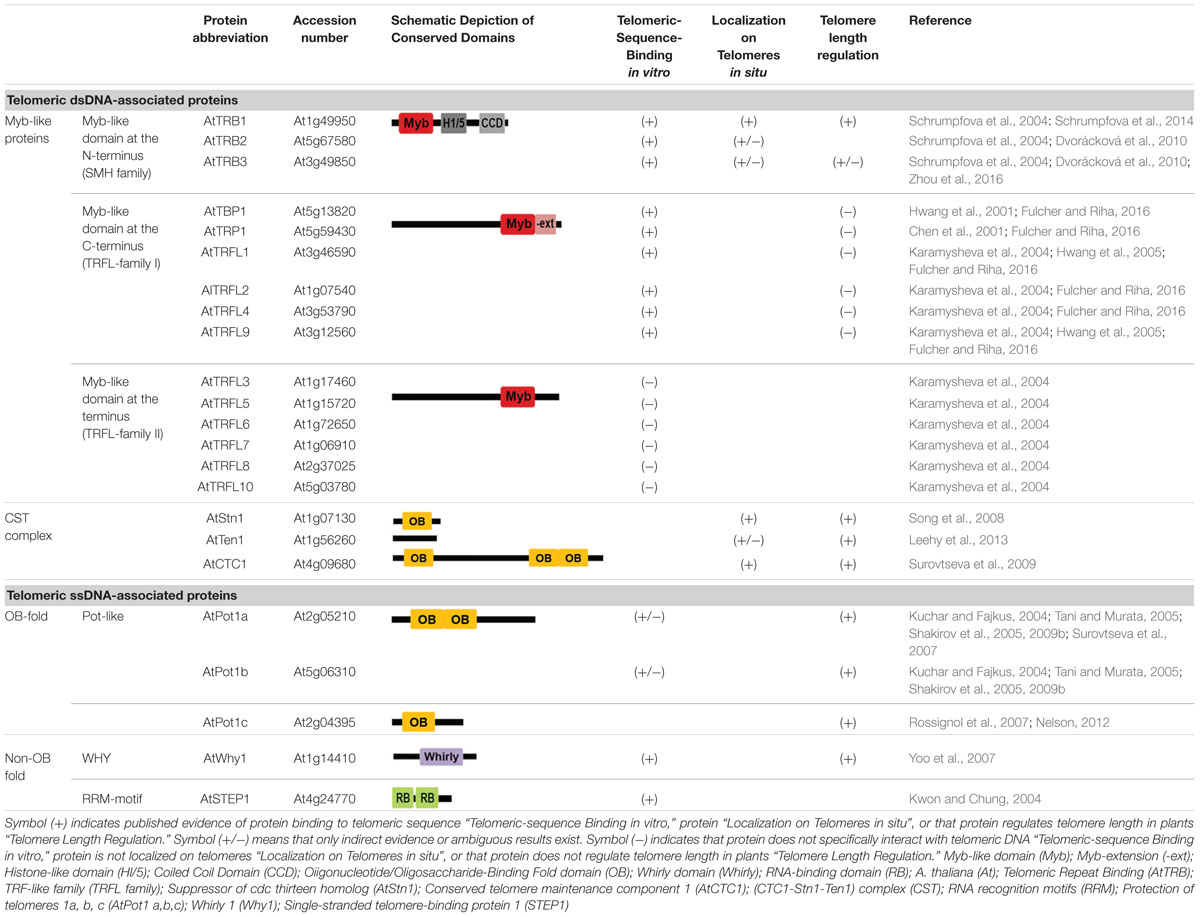
TABLE 2. Telomeric and putative telomeric dsDNA- and ssDNA-binding proteins from Arabidopsis thaliana.
Telomeric dsDNA-Associated Proteins
Myb-like Proteins
In plants, telomeric dsDNA sequence binding proteins with a Myb-like domain of a telobox (short telomeric motif) type can be classified into three main groups: (i) with a Myb-like domain at the N-terminus (SMH family), (ii) with a Myb-like domain at the C-terminus (TRFL family), and (iii) with a Myb-like domain at the C-terminus (AID family; reviewed in Peska et al., 2011; Du et al., 2013).
The first group of proteins, with a Myb-like domain at the N-terminus, also contains a central histone-like domain with homology to the H1 globular domain found in the linker histones H1/H5, and is therefore called the SMH family (Marian et al., 2003; Schrumpfova et al., 2004). Proteins with an SMH motif are plant-specific but are well conserved throughout the plant kingdom (e.g., eudicots, monocots, moss, or red algae; Du et al., 2013). In A. thaliana, there are five members of the SMH family, named telomere repeat binding (AtTRB) proteins (Marian et al., 2003; Schrumpfova et al., 2004). AtTRB1 protein specifically binds plant telomeric repeats through a Myb-like domain in vitro (Mozgova et al., 2008), co-localizes with telomeres in situ, and physically interacts with AtTERT (Figure 2B). Moreover shortening of telomeres was observed in attrb1 knockout mutants (Schrumpfova et al., 2014). Also other members of this family, AtTRB2 and AtTRB3 (previously named AtTBP3 and AtTBP2, respectively; Schrumpfova et al., 2004), bind telomeric dsDNA as well as telomeric ssDNA in vitro as homo- or heteromultimers (Schrumpfova et al., 2004; Mozgova et al., 2008; Hofr et al., 2009; Lee W.K. et al., 2012; Yun et al., 2014). In Arabidopsis, AtTRB1 protein physically interacts via its histone-like domain with AtPot1b (Schrumpfova et al., 2008), an A. thaliana homolog of the G-overhang binding protein Pot1, and a component of an alternative telomerase holoenzyme complex (Tani and Murata, 2005; He et al., 2006; Surovtseva et al., 2007). Also other members of SMH family proteins in land plants show telomeric dsDNA binding capability: e.g., Oryza sativa OsTRBFs (Byun et al., 2008) or Z. mays ZmSMHs (Marian et al., 2003). In addition, proteins with Myb-like domain of a telobox type in plants, adopt distinct non-telomeric functions, e.g., PcMYB1 from Petroselinum crispum acts only as a transcription factor (Feldbrugge et al., 1997). Recently it was shown that AtTRB1 from A. thaliana was not only telomere- and telomerase-binding but was also associated, in vivo, with promoters, mostly with a telo box motif of translation machinery genes (Figure 3; Schrumpfova et al., 2016). The AtTRB1 association with telo box motif was then proven by Zhou et al. (2016). Moreover AtTRB proteins seem to have a new role as chromatin modulators: AtTRB1 competes with LIKE HETEROCHROMATIN PROTEIN 1 (AtLHP1) to maintain downregulation of polycomb group (PcG) target genes (Zhou et al., 2016) and protein AtTRB2 directly interacts with histone deacetylases, HDT4 and HDA6, in vitro and in vivo (Lee and Cho, 2016). Deacetylase activity of HDT4 (Lee and Cho, 2016) and HDA6 (To et al., 2011) against H3K27ac, could be important for subsequent methylations of H3K27me3, that is among others target also for AtLHP1. Taken together, two lines of evidence classify the AtTRB proteins as novel epigenetic regulators that potentially impact transcription status of thousands of genes: (i) association of AtTRB1 with telo box DNA motif (Schrumpfova et al., 2016; Zhou et al., 2016) that is linked with PcG protein pathway (Deng et al., 2013; Wang et al., 2016; Zhou et al., 2016), (ii) involvement of AtTRB proteins in control of H3K27 epigenetic modifications (Lee and Cho, 2016; Zhou et al., 2016), that are also connected with PcG chromatin remodelers.
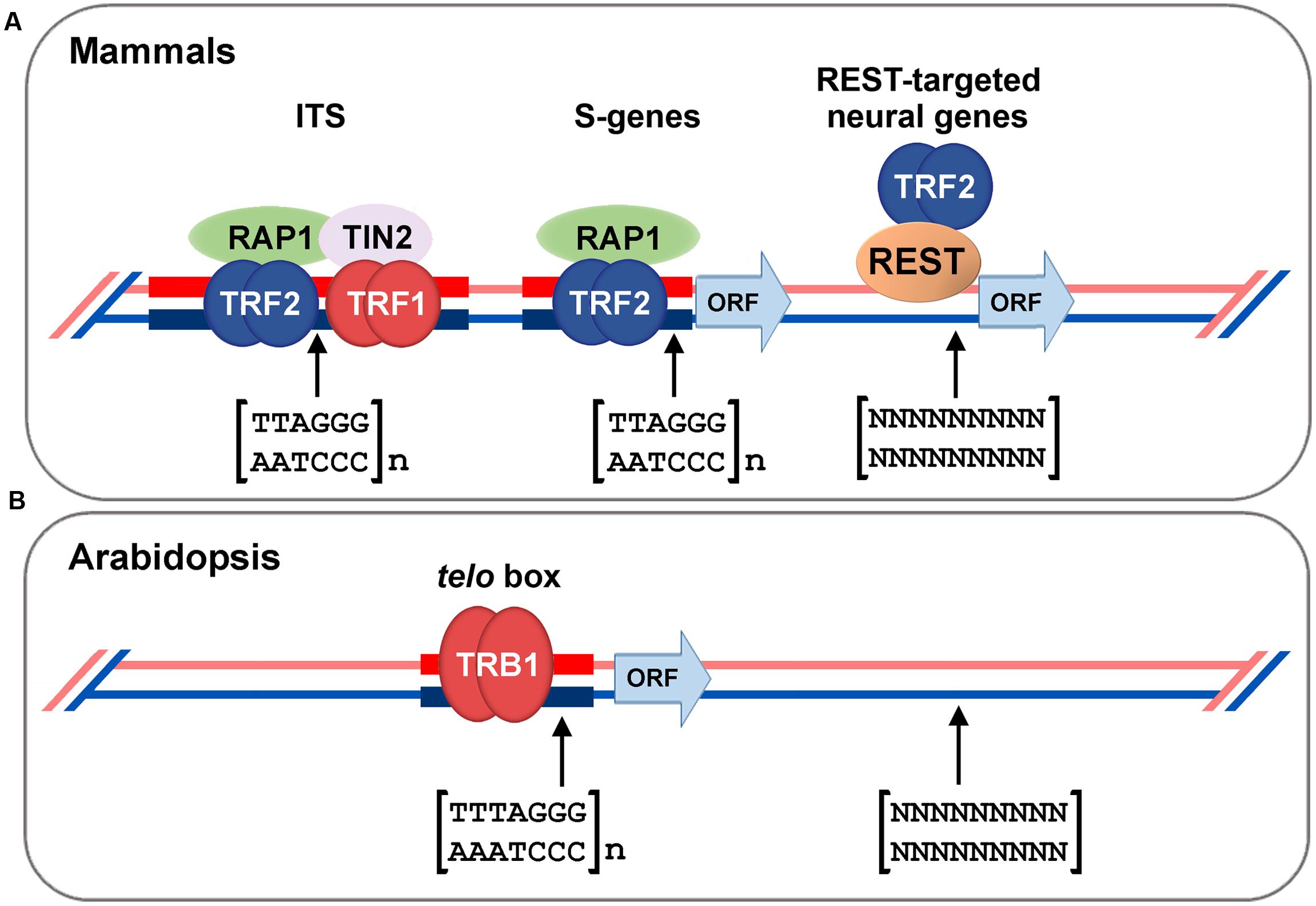
FIGURE 3. Association of shelterin proteins with extra-telomeric sequences. (A) Mammalian telomere-binding proteins TRF1/2, TIN2, or Rap1 associate not only with terminally localized telomeric repeats but also with interstitial telomeric sequences (ITS) and satellite repeats (Krutilina et al., 2001; Mignon-Ravix et al., 2002; Simonet et al., 2011; Yang et al., 2011). Mouse Rap1, together with TRF2, acts as a gene transcription regulator, including subtelomeric-gene (S-gene) silencing, and also binds to non-coding genomic regions enriched with TTAGGG repeats (Martinez et al., 2010). Moreover, TRF2 regulates neuronal genes by interaction of TRF2 with repressor element 1-silencing transcription factor (REST; Zhang et al., 2008). (B) Arabidopsis TRB1 protein was found not only as a component of the telomeric interactome (Schrumpfova et al., 2014), but also as a factor associated with 5′ flanking regions (mostly comprising the telo box) of translation machinery genes (Schrumpfova et al., 2016; Zhou et al., 2016).
The second group of proteins, with a Myb-like domain at the C-terminus, is also named TRFL. However a TRFL Myb-like domain alone is not sufficient for telomere binding and requires a more extended domain—Myb-extension (Myb-ext)—for telomeric dsDNA interactions in vitro (Karamysheva et al., 2004; Ko et al., 2008). Consequently, two families of TRFL can be distinguished: TRFL family 1 with a Myb-ext, whose protein members bind telomeric dsDNA in vitro, and TRFL family 2 without a Myb-ext, whose protein members do not bind telomeric dsDNA specifically in vitro and they are usually not considered as telomeric proteins (Karamysheva et al., 2004). The first identification of a TRFL family protein from O. sativa—telomere-binding protein 1 (OsRTBP1; Yu et al., 2000) was soon followed by numerous other TRFL members: e.g., Nicotiana glutinosa (NgTRF1; Yang et al., 2003), Solanum lycopersicum (LeTBP1; Moriguchi et al., 2006), A. thaliana (AtTBP1, AtTRP1, AtTRFL2-10; Chen et al., 2001; Hwang et al., 2001; Karamysheva et al., 2004), Cestrum parqui (CpTBP; Peska et al., 2011). Even though O. sativa or N. glutinosa mutants for TRFL members exhibited markedly longer telomeres (Yang et al., 2004; Hong et al., 2007), in A. thaliana, a knockout of AtTRP1, member of TRFL family 1 with a Myb-ext, did not change telomere length significantly (Chen et al., 2005). Even multiple knock-out plant, deficient for all six proteins from TRFL family 1 in A. thaliana (AtTBP1, AtTRP1, AtTRFL1, AtTRFL2, AtTRFL4, and AtTRF9) did not exhibit changes in telomere length, or phenotypes associated with telomere dysfunction (Fulcher and Riha, 2016). Thus, although the AtTRFL proteins from A. thaliana specifically bind telomeric DNA in vitro and an interaction between AtTRP1 and AtKu70 was observed, suggesting a putative telomere function (Figure 2B; Kuchar and Fajkus, 2004), no functional evidence exists for their role at telomeres. Another member of this family—ZmIBP2 (initiator-binding protein) protein—binds not only telomeric repeats (Moore, 2009), but was originally identified as a promoter binding ligand (Lugert and Werr, 1994). Moreover, some members of this Myb-like family were identified exclusively based on their ability to bind promoter regions of certain genes: ZmIBP1 (Lugert and Werr, 1994), PcBPF-1 (box P-binding factor) from P. crispum (da Costa e Silva et al., 1993) or CrBPF from Catharanthus roseus (van der Fits et al., 2000).
The third group with a Myb-like domain at the C-terminus (AID family) contains only a few members. The AID family is named according to anther indehiscence 1 (AID) protein from O. sativa—OsAID1 (Zhu et al., 2004). OsAID1 was initially identified as being involved in anther development. Another member of this family—ZmTacs1 (terminal acidic SANT) from Z. mays—may function in chromatin remodeling within the meristem (Marian and Bass, 2005).
In an affinity pull-down technique, 80 proteins from O. sativa were identified for their ability to bind to a telomeric repeat (He et al., 2013). Among them, two of three previously reported proteins from the SMH family–OsTRBF1 and OsTRBF2 (Byun et al., 2008), and one protein with a Myb-domain at the C-terminus (AID family)—OsAID1 (Zhu et al., 2004; Du et al., 2013) were demonstrated, while no member with a Myb-domain at the C-terminus of the TRFL family could be found. From other ribonucleoproteins or RNA-binding proteins with putative telomere association, two homologs of N. tabacum telomeric ssDNA binding protein NtGTBP1 (Os08g0492100 and Os08g0320100), with RNA recognition motifs (RRM; see below; Lee and Kim, 2010), were also identified.
Telomere-binding proteins in budding yeast (yRap1) or in mammals (TRF1, TRF2, Rap1, and TIN2) are associated with extra-telomeric sequences and thus participate in additional roles, e.g., gene activation and repression, DNA replication, heterochromatin boundary-element formation, creation of hotspots for meiotic recombination and chromatin opening (Figure 3A; Morse, 2000; Smogorzewska et al., 2000; Krutilina et al., 2001; Zhang et al., 2008; Martinez et al., 2010; Simonet et al., 2011; Yang et al., 2011; Maï et al., 2014; Ye et al., 2014).
CST Complex
An evolutionary conserved trimeric protein complex named CST (Cdc13/CTC1–Stn1–Ten1) is, similarly to Myb-like proteins, involved in several stages of telomere end formation. In yeast, these OB-fold proteins are required for recruitment of telomerase and DNA polymerase α to the chromosomal termini, and thus coordinate G-overhang extension by telomerase with the fill-in synthesis of the complementary C-strand (Qi and Zakian, 2000; Grossi et al., 2004; Giraud-Panis et al., 2010; Wellinger and Zakian, 2012). In mammals, CST is primarily involved in the rescue of stalled replication forks at the telomere and elsewhere in the genome, and limits telomerase action at individual telomeres to approximately one binding and extension event per cell cycle (Figure 2A; Chen et al., 2012; Stewart et al., 2012; Chen and Lingner, 2013; Kasbek et al., 2013).
In A. thaliana, a mutation in any CST subunits leads to severe morphological defects and is accompanied by a decrease in telomere length, single-strand G-overhang elongation, mostly subtelomere–subtelomere chromosomal fusions and the appearance of extra-chromosomal telomeric circles. Plants lacking Suppressor of cdc thirteen homolog (AtStn1) or Conserved telomere maintenance component 1 (AtCTC1) exhibit no change in telomerase activity whereas telomerase activity was elevated in atten1 mutants (Song et al., 2008; Surovtseva et al., 2009; Leehy et al., 2013). Although circumstantial evidence indicates that CST in plants is needed for telomere integrity, clear evidence is absent that would show any direct physical interaction of any component of the CST complex with plant telomeric DNA. As Arabidopsis AtCTC1 interacts with the catalytic subunit of DNA polymerase α (ICU2) in vitro (Price et al., 2010) and atstn1 mutant phenotypes can be partially phenocopied by impairment of DNA polymerase α, it was recently suggested that seemingly specific function(s) of CST in telomere protection may rather represent unique requirements for efficient replication of telomeric DNA (Figure 2B; Derboven et al., 2014). It seems that the CST complex controls access of telomerase, end-joining recombination and the ATR-dependent (ATM and Rad3-related) DNA damage response pathway at the chromosomal ends in wild-type plants (Boltz et al., 2012; Leehy et al., 2013; Amiard et al., 2014).
Telomeric ssDNA-Associated Proteins
Proteins with OB-fold
The telomeric G-rich overhang is evolutionarily conserved and is a substrate for ssDNA binding proteins. The majority of ssDNA binding proteins bind through OB motifs (OB-fold) and are required for both chromosomal end protection and regulation of telomere length, e.g., telomere-binding protein subunit alpha/beta (TEBPαβ) from Oxytricha nova (telomere end binding protein; Price and Cech, 1987), Cell division cycle 13 (Cdc13p) from S. cerevisiae (Garvik et al., 1995) and Pot1, are present in diverse organisms including human, mouse, chicken, or S. pombe (Figure 2A; Baumann and Cech, 2001; Lei et al., 2002; Wei and Price, 2004; Wu et al., 2006). In A. thaliana, three Pot-like proteins have been named AtPot1a, AtPot1b, AtPot1c (Kuchar and Fajkus, 2004; Rossignol et al., 2007; previously also named as AtPOT1-1, AtPOT1-2 (Tani and Murata, 2005) or AtPot1, AtPot2 (Shakirov et al., 2005; see Rotkova et al., 2009 for an overview). However, descriptions of their functions and binding properties are not unanimously agreed. While a very weak, but specific affinity of AtPot1a and AtPot1b for plant telomeric ssDNA was originally described (Shakirov et al., 2005), later these authors could not demonstrate AtPot1a and AtPot1b binding to telomeric ssDNA in vitro (Shakirov et al., 2009a,b). Nevertheless, stable telomeric ssDNA binding was observed for two full-length plant Pot1 proteins: OlPot1 from the green alga Ostreococcus lucimarinus as well as for ZmPot1b from Z. mays (Shakirov et al., 2009b). Although Pot1 proteins from plant species as diverse as Hordeum vulgare (HvPot1; barley), Populus trichocarpa (poplar), Helianthus argophyllus (sunflower), Selaginella moellendorffii (spikemoss), Gossypium hirsutum (cotton), Pinus taeda (pine), Solanum tuberosum (StPot1; potato), Asparagus officinalis and Z. mays (ZmPot1a) failed to bind telomeric DNA when expressed in a rabbit reticulocyte lysate expression system in vitro and subjected to an electrophoretic mobility shift assay (Shakirov et al., 2009b), binding of plant Pot1 proteins to telomeric DNA under native conditions cannot be excluded. Plants expressing AtPot1a truncated by an N-terminal OB-fold, showed progressive loss of telomeric DNA. In contrast, telomere length was unperturbed in plants expressing analogously trimmed AtPot1b, although overexpression of C-terminally truncated AtPot1b resulted in telomere shortening (Shakirov et al., 2005).
AtPot1a binds AtStn1 and AtCTC1 proteins (Figure 2B; Renfrew et al., 2014), associates with an N-terminally spliced variant of AtTERT (AtTERT-V(I8)) (Rossignol et al., 2007), TER1, one of the RNA subunits of Arabidopsis telomerase, and is required for maintenance of telomere length in vivo (Surovtseva et al., 2007). AtPot1b directly interacts with Myb-like proteins AtTRB1-3 from the SMH family (Schrumpfova et al., 2008), and associates with TER2 and TER2s, putative alternative RNA subunits of telomerase that negatively regulate the function of active telomerase particles (TER1-AtTERT; Cifuentes-Rojas et al., 2012). Nevertheless, AtPot1b does not seem to substantially contribute to telomere maintenance (Cifuentes-Rojas et al., 2012). Pot1-like proteins were also identified in plants with unusual telomeres (e.g., CpPot1 protein in C. parqui; Peska et al., 2008).
Non-OB-fold Telomeric ssDNA Binding Proteins
The transcriptional activator protein Whirly 1 (Why1), from a small protein family found mainly in land plants (Desveaux et al., 2000, 2002; Krause et al., 2005), was also identified in a fraction of telomere-binding proteins in A. thaliana, and an atwhy1 knockout mutant appeared to have shorter telomeres (Yoo et al., 2007). While proteins from A. thaliana (AtWhy1; Yoo et al., 2007) and from H. vulgare (HvWhy1; Grabowski et al., 2008) were found to bind plant telomeric repeat sequences in vitro, diverse organelle localization of other Why family members from O. sativa, A. thaliana, S. tuberosum (Krause et al., 2005; Schwacke et al., 2007) and proposed binding to ssDNA of melted promoter regions (Desveaux et al., 2002), rather indicate a role in communication between plastid and nuclear genes encoding photosynthetic proteins (Foyer et al., 2014; Comadira et al., 2015).
A truncated derivative of chloroplast RNA-binding protein (AtCP31) with RRMs from A. thaliana, named AtSTEP1 (single-stranded telomere-binding protein 1), localizes exclusively to the nucleus, specifically binding single-stranded G-rich plant telomeric DNA sequences and inhibiting telomerase-mediated telomere extension (Kwon and Chung, 2004).
A protein identified by gel mobility shift assay that specifically binds the G-strand of telomeric ssDNA from N. tabacum (NtGTBP1) also contains a tandem pair of RRMs (Hirata et al., 2004). NtGTBP1 is not only associated with telomeric sequences, as well as two additional GTBP paralogs (NtGTBP2 and NtGTBP3), but also inhibits telomeric strand invasion in vitro and leaves of knockdown tobacco plants contained longer telomeres with frequent formation of extrachromosomal t-circles (Lee and Kim, 2010). These observations correspond to a previously detected protein from tobacco nuclei that binds G-rich telomeric strands and reduces accessibility to telomerase or terminal transferase (Fulneckova and Fajkus, 2000).
In addition to the above described proteins, various telomeric ssDNA binding proteins have also been reported in nuclear extracts from Glycine max, A. thaliana, O. sativa, or Vigna radiata (Zentgraf, 1995; Kim et al., 1998; Lee et al., 2000; Kwon et al., 2004). However, precise characterization of these proteins, identified by gel mobility shift assay, is mostly missing.
DNA Repair Proteins and Telomeres
Ku in plants, as well as in other eukaryotes, is a highly conserved complex, consisting of two polypeptides (Ku70 and Ku80; Mimori et al., 1981). Due to its high affinity for DNA ends, Ku has a generally conserved role across species in protecting DNA from nucleolytic degradation. Ku is important for several cellular mechanisms: the DNA double-stranded break (DSB) repair pathway by the Ku-dependent non-homologous end-joining (NHEJ) pathway, the DNA damage response machinery, or protection of telomere ends from being recognized as DSBs, thereby preventing their recombination and degradation (reviewed in Fell and Schild-Poulter, 2015). Human Ku directly interacts not only with the shelterin proteins hTRF1, hTRF2, and hRap1, but also with telomerase subunits hTERT and hTR (RNA template; reviewed in Fell and Schild-Poulter, 2015). In contrast to a massive loss of telomeric DNA that was observed in human cells (Wang et al., 2009), mutations in Ku70 and Ku80 in the dicotyledonous A. thaliana, as well as in the monocotyledonous O. sativa, resulted in longer telomeres, suggesting their conserved role in the negative regulation of plant telomerase (Bundock et al., 2002; Riha et al., 2002; Gallego et al., 2003; Hong et al., 2010). On the other hand, severe developmental defects were observed in O. sativa osku70 knockout mutants, but a similar mutation in A. thaliana atku70 showed no effect on plant development (Bundock et al., 2002; Hong et al., 2010). In S. latifolia and A. thaliana, Ku contributes to the integrity of blunt-ended telomeres by protecting them from nucleolytic resection (Kazda et al., 2012). AtKu specifically interacts with AtTRP1 protein (see above; Figure 2B; Kuchar and Fajkus, 2004) and also assembles with TER2 and TER2S into alternative telomerase complexes that cannot sustain telomere repeats on chromosomal ends (Cifuentes-Rojas et al., 2012).
The mammalian shelterin complex is involved in the repression of the primary signal transducers of DNA breakage, two phosphatidylinositol-3-kinase-like (PI3K) protein kinases: ataxia telangiectasia mutated (ATM) and ATM- and RAD3-related (ATR) kinases. Mice TRF2 acts mainly to protect telomeres against ATM activation (Celli and de Lange, 2005) and POT1 is principally involved in repression of the ATR pathway (Denchi and de Lange, 2007; Guo et al., 2007). Short telomeres in telomerase-deficient plants activate both the AtATM and AtATR, whereas absence of members of the CST complex initiates only AtATR-dependent, but not AtATM-dependent DNA damage response (Amiard et al., 2011; Boltz et al., 2012). In mammals as well as in other organisms, DSBs activate ATM kinase in a manner dependent on the meiotic recombination 11 (Mre11), DNA repair protein 50 (Rad50), and Nijmegen breakage syndrome 1 (Nbs1) named MRN complex. The MRN complex has been found to associate with telomeres and contributes to their maintenance (reviewed in Lamarche et al., 2010). A. thaliana AtRad50 mutant plant cells show a progressive shortening of telomeric DNA (Gallego and White, 2001), while in AtMre11 mutant plants, telomere lengthening was observed (Bundock and Hooykaas, 2002). Contrary to these observations, the absence of the third MRN subunit, AtNbs1, does not affect the length of telomeres (Najdekrova and Siroky, 2012).
A. thaliana plants mutated in XPF (xeroderma pigmentosum group F-complementing) and ERCC1 (excision repair cross-complementation group 1) orthologs that form a structure-specific endonuclease essential for nucleotide excision repair (known as AtRad1 and AtERCC1), develop normally and show wild-type telomere length. However, in the absence of telomerase, mutations in either of these genes induce a significantly earlier onset of chromosomal instability, thus indicating a protective role of AtERCC1/AtRad1 against a 3′ G-strand overhang invasion of interstitial telomeric repeats (Vannier et al., 2009). In addition to the Ku proteins that are involved in Ku-dependent NHEJ, an alternative Ku-independent NHEJ pathway was described (reviewed in Decottignies, 2013). Members of the poly(ADP-ribose) polymerase family play a role not only in the base excision repair pathway and the backup-NHEJ KU-independent pathway (Decottignies, 2013) but were also studied in the context of telomere maintenance, association with shelterin proteins or modulation of telomerase activity (Smith et al., 1998; Cook et al., 2002; Beneke et al., 2008). However, analysis of Arabidopsis orthologs AtPARP1/AtPARP2 (poly(ADP-Ribose) polymerase) has revealed that, unlike in humans, AtPARPs play a minor role in telomere biology (Boltz et al., 2014). It was proposed that DSB repair pathways in A. thaliana are hierarchically organized and the Ku-dependent NHEJ restricts access and action of other DSB repair processes (Charbonnel et al., 2010, 2011). Furthermore the end-joining recombination proteins (AtKU80, AtXRCC1, AtRad1) restrict telomerase activity at deprotected telomeres (Amiard et al., 2014). It was found recently that structure-specific endonucleases AtMUS81 (MMS and UV-sensitive protein 81) and AtSEND1 (single-strand DNA endonuclease 1), which presumably act to repair potentially toxic structures produced by DNA replication and recombination, are essential for telomere stability in Arabidopsis. Combined absence of these endonucleases results in increased occurrence of histone γ-H2AX foci in S-phase and in loss of telomeric DNA (Olivier et al., 2016).
Plant Telomerase
Telomere length in plants and various other organisms is maintained by telomerase, a specialized reverse transcriptase which, in addition to its catalytic subunit (TERT), carries its own RNA template (TR) and elongates telomeric tracts at the chromosomal terminus (Blackburn and Gall, 1978; Fajkus et al., 1996).
TERT subunits consist of an N-terminal portion with telomerase-specific motifs important for binding the telomerase RNA subunit, catalytic domains with the telomerase reverse transcriptase (RT) motifs essential for enzyme activity, and the C-terminal extension, which is highly conserved among plants as well as vertebrates (Sykorova and Fajkus, 2009). Although most eukaryotes harbor only a single TERT gene, in the allotetraploid N. tabacum there are three NtTERT gene variants inherited from its diploid progenitor species N. sylvestris and Nicotiana tomentosiformis. All three NtTERT gene variants are transcribed.
Alternative splicing provides a major source of protein diversity within a given organism. Alternatively spliced variants of TERT transcripts with out-of-frame and/or in-frame mutations were identified not only in humans, mouse, chicken, or Xenopus (reviewed in Hrdlickova et al., 2006), but also in many plant species, e.g., A. thaliana, Z. mays (ZmTERT), O. sativa (OsTERT), Iris tectorum, and tobacco [with human-type (TTAGGG) telomere motif; reviewed in Sykorova and Fajkus, 2009; Sykorova et al., 2012]. Isoforms generated by alternative splicing may show changes or loss of specific function(s) or subcellular localization of the respective product, or could be functionally important, as was suggested for the A. thaliana variant AtTERT V(I8) that exclusively interacts with AtPot1a (Rossignol et al., 2007).
It has been proposed that human telomerase is subjected to posttranslational regulation such as phosphorylation (Kang et al., 1999). Putative phosphorylation sites were detected in the OsTERT sequences from O. sativa (Oguchi et al., 2004) or N. tabacum BY-2 cells (Yang et al., 2002) but not in AtTERT from A. thaliana (Oguchi et al., 2004).
Telomerase-Associated Proteins
Rich protein interactomes of yeast, mammalian or Ciliate TERT have been described, including the Ku heterodimer (Chai et al., 2002), HSP90 (heat-shock protein of 90 kDa; Holt et al., 1999; Grandin and Charbonneau, 2001), ATPases pontin and reptin (Venteicher et al., 2008), TEP1 (telomere protein 1; Harrington et al., 1997), and many others, in a broad study (Fu and Collins, 2007) and reviewed in a constantly updated telomerase database (Podlevsky et al., 2008).
In AtTERT, a mitochondrial targeting signal, multiple nuclear localization signals or a nuclear export signal have been reported (Zachova et al., 2013). As AtTERT protein and its domains localize mainly within the nucleus and the nucleolus (Zachova et al., 2013), it can be assumed that most interacting protein partners relevant to telomeric functions will be found among nuclear or nucleolar proteins.
In plants, a limited number of proteins that directly interact with TERT have been described. It was demonstrated by various direct methods that AtTRB proteins, a group of plant homologs of human TRF proteins with a Myb-domain at the N-terminus (see above), physically interact with N-terminal domains of AtTERT (Figure 2B; Schrumpfova et al., 2014). A mediated interaction between AtTRP1 protein that belongs to the TRFL family, and AtTERT, was also observed (Schrumpfova et al., 2014). Moreover, the N-terminal part of AtTERT exclusively interacts with AtPot1a but not AtPot1b (Rossignol et al., 2007). Also various proteins with an RRM-motif (AtRRM), an ARM-motif (armadillo/β-catenin-like repeat-containing protein; AtARM), metallothionein-like (AtMT2A), or RNA-binding (AtG2p) motifs were found as AtTERT interacting partners in A. thaliana (Lee L.Y. et al., 2012; Dokládal et al., 2015).
Indirect regulation of TERT by various proteins or hormones was further described in plants. In tobacco cell culture, phytohormones such as auxin or abscisic acid regulate phosphorylation of telomerase protein, which is required for the generation of a functional telomerase complex (Tamura et al., 1999; Yang et al., 2002). In A. thaliana, reduced endogenous concentrations of auxin in telomerase activator 1 (AtTAC1) mutant plants blocks the ability of this zinc-finger protein to induce AtTERT. However, AtTAC1 does not directly bind the AtTERT promoter (Ren et al., 2004, 2007). A minimal promoter region for AtTERT was proposed using a set of T-insertion mutant lines in the protein-coding region of the AtTERT gene or in lines with insertions at the 5′ end of AtTERT (Fojtova et al., 2011). Moreover T-DNA insertions in the region upstream of the ATG start of AtTERT also led to the activation of putative regulatory elements (Fojtova et al., 2011).
In vertebrates, only one TR per organism was described. The folding of the TR molecule offers interaction sites for various associating cofactors such as dyskerin, Ku, nucleolar protein 10 (NOP10), H/ACA ribonucleoprotein complex subunit 1 (GAR1), or subunit 2 (NHP2; Ting et al., 2005; for review, see Kiss et al., 2010). A single TR was also described among Brassicaceae family plants. However, in A. thaliana, two TRs were detected—TER1 and TER2, and the latter may be alternately spliced to a TER2s form (Beilstein et al., 2012). The Arabidopsis homolog of human dyskerin, named AtCBF5 (alias AtNAP57), is located within nucleoli and Cajal bodies (Lermontova et al., 2007), associates with active telomerase, and weakly with AtPOT1a, but not AtTERT or AtKu70 (Kannan et al., 2008).
Telomerase-Independent Processes in Plant Telomere Dynamics
Compared to the human model, knowledge of individual protein contributions to the maintenance of telomere length/ accessibility/folding in plants or telomerase biogenesis/regulation is still very limited. The process of telomere maintenance is complicated by the fact that besides the widespread system of telomere maintenance by telomerase (Fajkus et al., 1996; Heller et al., 1996) in plants as well as in other organisms, in the absence of telomerase, telomeres can be elongated by recombination-dependent and telomerase-independent alternative telomere lengthening (ALT) mechanisms (Fajkus et al., 2005). Moreover, in plants, the ALT events appear to participate in early plant development (Ruckova et al., 2008). It was shown that AtKu70 deficiency facilitates engagement of ALT lengthening in A. thaliana (Zellinger et al., 2007) and that ALT was suppressed in the absence of ATM protein (Vespa et al., 2007).
Telomeric DNA of higher eukaryotes, including plants, is associated not only with specific proteins, but also with histone complexes that form nucleosomes (Figure 2; reviewed in Dvořáčková et al., 2015). In various organisms, as well as in plants, telomeric nucleosomes display an unusually short periodicity (157 bp in length), usually 20–40 bp shorter than bulk nucleosomes of the corresponding organism (Fajkus et al., 1995; Fajkus and Trifonov, 2001; reviewed in Pisano et al., 2008). Moreover, the plant telomeric repeat (CCCTAAA) is a natural target for plant-specific asymmetric methylation (Cokus et al., 2008) that was shown to be mediated by an siRNA pathway (Vrbsky et al., 2010). Analysis of telomeres in A. thaliana (Vrbsky et al., 2010) and N. tabacum (Majerova et al., 2011) has demonstrated that telomeric histones were associated with both heterochromatin- and euchromatin-specific marks. Recent data strongly support the involvement of various epigenetic mechanisms (DNA methylation, posttranslational modifications of histones, nucleosome assembly or levels of telomere-repeat containing RNA) in maintenance of telomere stability (reviewed in Dvořáčková et al., 2015) thus demonstrating complexity of telomere regulation.
Conclusion
The need for protection of chromosomal termini remains conserved across most species. Nevertheless, an extraordinary plasticity of mechanisms protecting telomeres has been described among various organisms (reviewed in Giraud-Panis et al., 2013). While individual capping proteins can differ greatly, common features such as homologous binding domains, structures, or interacting partners exist between seemingly different capping systems. Plant systems show certain distinct features of telomere maintenance, including the reversible regulation of telomerase in somatic cells and the absence of developmental telomere shortening (Fajkus et al., 1998; Riha et al., 1998). These distinctions promote further efforts to elucidate plant telomere interactomes. Only recently the first complexes of telomere-binding proteins were demonstrated and meanwhile it seems that the plant telomere-maintenance system shares similarities with that described in mammals. For example, in A. thaliana, one of the most studied plant model systems: (i) the core plant telomeric dsDNA binding proteins (AtTRBs, AtTRP, etc.) contain similar Myb-domains which are also present in human TRF1 or TRF2 proteins; (ii) homologs of human telomeric ssDNA binding hPot1 (AtPOT1a-c) were described; (iii) cross-species conserved CST complexes (AtCTC1/AtTen1/AtStn1) retain its function in plants. The similarities between plant and mammalian telomeric DNA-associated proteins apply also to their roles in regulation of gene expression, which are independent of their roles in telomere capping (Lee and Cho, 2016; Schrumpfova et al., 2016; Zhou et al., 2016), as was previously described in their mammalian counterparts (reviewed in Maï et al., 2014; Ye et al., 2014). Elucidation of the composition of the plant version of shelterin and molecular dissection of its components and their roles will be important in the near future to assess the conservation and mechanisms of end-protection and end-replication processes in yeasts, plants and animals.
Author Contributions
PPS contributed substantially to the writing of the manuscript, tables and drawing the figures; ŠS participated in preparation of tables; JF edited the manuscript. All authors read and approved the manuscript for publication.
Funding
This research was supported by the Czech Science Foundation (13-06943S and 16-01137S) and by the Ministry of Education, Youth and Sports of the Czech Republic under the project CEITEC 2020 (LQ1601).
Conflict of Interest Statement
The authors declare that the research was conducted in the absence of any commercial or financial relationships that could be construed as a potential conflict of interest.
The handling Editor declared a current collaboration as co-Topic Editor in a Frontiers Research Topic with one of the authors, JF, and states that the process nevertheless met the standards of a fair and objective review. This was also confirmed by the Specialty Chief Editor of section Plant Cell Biology, Simon Gilroy.
References
Adams, S. P., Hartman, T. P., Lim, K. Y., Chase, M. W., Bennett, M. D., Leitch, I. J., et al. (2001). Loss and recovery of Arabidopsis-type telomere repeat sequences 5′-(TTTAGGG)(n)-3′ in the evolution of a major radiation of flowering plants. Proc. Biol. Sci. 268, 1541–1546. doi: 10.1098/rspb.2001.1726
Amiard, S., Depeiges, A., Allain, E., White, C. I., and Gallego, M. E. (2011). Arabidopsis ATM and ATR kinases prevent propagation of genome damage caused by telomere dysfunction. Plant Cell 23, 4254–4265. doi: 10.1105/tpc.111.092387
Amiard, S., Olivier, M., Allain, E., Choi, K., Smith-Unna, R., Henderson, I. R., et al. (2014). Telomere stability and development of ctc1 mutants are rescued by inhibition of EJ recombination pathways in a telomerase-dependent manner. Nucleic Acids Res. 42, 11979–11991. doi: 10.1093/nar/gku897
Arat, N. O., and Griffith, J. D. (2012). Human Rap1 interacts directly with telomeric DNA and regulates TRF2 localization at the telomere. J. Biol. Chem. 287, 41583–41594. doi: 10.1074/jbc.M112.415984
Armstrong, S. J., Franklin, F. C., and Jones, G. H. (2001). Nucleolus-associated telomere clustering and pairing precede meiotic chromosome synapsis in Arabidopsis thaliana. J. Cell Sci. 114, 4207–4217.
Aronen, T., and Ryynanen, L. (2014). Silver birch telomeres shorten in tissue culture. Tree Genet. Genomes 10, 67–74. doi: 10.1007/s11295-013-0662-4
Bass, H. W., Marshall, W. F., Sedat, J. W., Agard, D. A., and Cande, W. Z. (1997). Telomeres cluster de novo before the initiation of synapsis: a three-dimensional spatial analysis of telomere positions before and during meiotic prophase. J. Cell Biol. 137, 5–18. doi: 10.1083/jcb.137.1.5
Baumann, P., and Cech, T. R. (2001). Pot1, the putative telomere end-binding protein in fission yeast and humans. Science 292, 1171–1175. doi: 10.1126/science.1060036
Beilstein, M. A., Brinegar, A. E., and Shippen, D. E. (2012). Evolution of the Arabidopsis telomerase RNA. Front. Genet. 3:188. doi: 10.3389/fgene.2012.00188
Beilstein, M. A., Renfrew, K. B., Song, X., Shakirov, E. V., Zanis, M. J., and Shippen, D. E. (2015). Evolution of the telomere-associated protein POT1a in Arabidopsis thaliana is characterized by positive selection to reinforce protein-protein interaction. Mol. Biol. Evol. 32, 1329–1341. doi: 10.1093/molbev/msv025
Beneke, S., Cohausz, O., Malanga, M., Boukamp, P., Althaus, F., and Burkle, A. (2008). Rapid regulation of telomere length is mediated by poly(ADP-ribose) polymerase-1. Nucleic Acids Res. 36, 6309–6317. doi: 10.1093/nar/gkn615
Bianchi, A., and Shore, D. (2008). How telomerase reaches its end: mechanism of telomerase regulation by the telomeric complex. Mol. Cell. 31, 153–165. doi: 10.1016/j.molcel.2008.06.013
Bilaud, T., Koering, C. E., Binet-Brasselet, E., Ancelin, K., Pollice, A., Gasser, S. M., et al. (1996). The telobox, a Myb-related telomeric DNA binding motif found in proteins from yeast, plants and human. Nucleic Acids Res. 24, 1294–1303. doi: 10.1093/nar/24.7.1294
Blackburn, E. H., and Gall, J. G. (1978). A tandemly repeated sequence at the termini of the extrachromosomal ribosomal RNA genes in Tetrahymena. J. Mol. Biol. 120, 33–53.
Boltz, K. A., Jasti, M., Townley, J. M., and Shippen, D. E. (2014). Analysis of poly(ADP-Ribose) polymerases in Arabidopsis telomere biology. PLoS ONE 9:e88872. doi: 10.1371/journal.pone.0088872
Boltz, K. A., Leehy, K., Song, X., Nelson, A. D., and Shippen, D. E. (2012). ATR cooperates with CTC1 and STN1 to maintain telomeres and genome integrity in Arabidopsis. Mol. Biol. Cell 23, 1558–1568. doi: 10.1091/mbc.E11-12-1002
Broccoli, D., Smogorzewska, A., Chong, L., and de Lange, T. (1997). Human telomeres contain two distinct Myb-related proteins, TRF1 and TRF2. Nat. Genet. 17, 231–235. doi: 10.1038/ng1097-231
Bundock, P., and Hooykaas, P. (2002). Severe developmental defects, hypersensitivity to DNA-damaging agents, and lengthened telomeres in Arabidopsis MRE11 mutants. Plant Cell 14, 2451–2462. doi: 10.1105/tpc.005959
Bundock, P., van Attikum, H., and Hooykaas, P. (2002). Increased telomere length and hypersensitivity to DNA damaging agents in an Arabidopsis KU70 mutant. Nucleic Acids Res. 30, 3395–3400. doi: 10.1093/nar/gkf445
Byun, M. Y., Hong, J. P., and Kim, W. T. (2008). Identification and characterization of three telomere repeat-binding factors in rice. Biochem. Biophys. Res. Commun. 372, 85–90. doi: 10.1016/j.bbrc.2008.04.181
Celli, G. B., and de Lange, T. (2005). DNA processing is not required for ATM-mediated telomere damage response after TRF2 deletion. Nat. Cell Biol. 7, 712–718. doi: 10.1038/ncb1275
Cesare, A. J., Quinney, N., Willcox, S., Subramanian, D., and Griffith, J. D. (2003). Telomere looping in P. sativum (common garden pea). Plant J. 36, 271–279. doi: 10.1046/j.1365-313X.2003.01882.x
Chai, W., Ford, L. P., Lenertz, L., Wright, W. E., and Shay, J. W. (2002). Human Ku70/80 associates physically with telomerase through interaction with hTERT. J. Biol. Chem. 277, 47242–47247. doi: 10.1074/jbc.M208542200
Charbonnel, C., Allain, E., Gallego, M. E., and White, C. I. (2011). Kinetic analysis of DNA double-strand break repair pathways in Arabidopsis. DNA Repair (Amst.) 10, 611–619. doi: 10.1016/j.dnarep.2011.04.002
Charbonnel, C., Gallego, M. E., and White, C. I. (2010). Xrcc1-dependent and Ku-dependent DNA double-strand break repair kinetics in Arabidopsis plants. Plant J. 64, 280–290. doi: 10.1111/j.1365-313X.2010.04331.x
Chen, C. M., Wang, C. T., and Ho, C. H. (2001). A plant gene encoding a Myb-like protein that binds telomeric GGTTTAG repeats in vitro. J. Biol. Chem. 276, 16511–16519. doi: 10.1074/jbc.M009659200
Chen, C. M., Wang, C. T., Kao, Y. H., Chang, G. D., Ho, C. H., Lee, F. M., et al. (2005). Functional redundancy of the duplex telomeric DNA-binding proteins in Arabidopsis. Bot. Bull. Acad. Sin. 46, 315–324.
Chen, L. Y., and Lingner, J. (2013). CST for the grand finale of telomere replication. Nucleus 4, 277–282. doi: 10.4161/nucl.25701
Chen, L. Y., Redon, S., and Lingner, J. (2012). The human CST complex is a terminator of telomerase activity. Nature 488, 540–544. doi: 10.1038/nature11269
Chikashige, Y., Tsutsumi, C., Yamane, M., Okamasa, K., Haraguchi, T., and Hiraoka, Y. (2006). Meiotic proteins bqt1 and bqt2 tether telomeres to form the bouquet arrangement of chromosomes. Cell 125, 59–69. doi: 10.1016/j.cell.2006.01.048
Cifuentes-Rojas, C., Nelson, A. D., Boltz, K. A., Kannan, K., She, X., and Shippen, D. E. (2012). An alternative telomerase RNA in Arabidopsis modulates enzyme activity in response to DNA damage. Genes Dev. 26, 2512–2523. doi: 10.1101/gad.202960.112
Cokus, S. J., Feng, S., Zhang, X., Chen, Z., Merriman, B., Haudenschild, C. D., et al. (2008). Shotgun bisulphite sequencing of the Arabidopsis genome reveals DNA methylation patterning. Nature 452, 215–219. doi: 10.1038/nature06745
Comadira, G., Rasool, B., Kaprinska, B., Garcia, B. M., Morris, J., Verrall, S. R., et al. (2015). WHIRLY1 functions in the control of responses to nitrogen deficiency but not aphid infestation in barley. Plant Physiol. 168, 1140–1151. doi: 10.1104/pp.15.00580
Cook, B. D., Dynek, J. N., Chang, W., Shostak, G., and Smith, S. (2002). Role for the related poly(ADP-Ribose) polymerases tankyrase 1 and 2 at human telomeres. Mol. Cell. Biol. 22, 332–342. doi: 10.1128/MCB.22.1.332-342.2002
Corredor, E., and Naranjo, T. (2007). Effect of colchicine and telocentric chromosome conformation on centromere and telomere dynamics at meiotic prophase I in wheat-rye additions. Chromosome Res. 15, 231–245. doi: 10.1007/s10577-006-1117-7
Court, R., Chapman, L., Fairall, L., and Rhodes, D. (2005). How the human telomeric proteins TRF1 and TRF2 recognize telomeric DNA: a view from high-resolution crystal structures. EMBO Rep. 6, 39–45. doi: 10.1038/sj.embor.7400314
Cowan, C. R., Carlton, P. M., and Cande, W. Z. (2001). The polar arrangement of telomeres in interphase and meiosis. Rabl organization and the bouquet. Plant Physiol. 125, 532–538.
Cowan, C. R., Carlton, P. M., and Cande, W. Z. (2002). Reorganization and polarization of the meiotic bouquet-stage cell can be uncoupled from telomere clustering. J. Cell Sci. 115, 3757–3766. doi: 10.1242/jcs.00054
da Costa e Silva, O., Klein, L., Schmelzer, E., Trezzini, G. F., and Hahlbrock, K. (1993). BPF-1, a pathogen-induced DNA-binding protein involved in the plant defense response. Plant J. 4, 125–135.
de Lange, T. (2005). Shelterin: the protein complex that shapes and safeguards human telomeres. Genes Dev. 19, 2100–2110. doi: 10.1101/gad.1346005
Decottignies, A. (2013). Alternative end-joining mechanisms: a historical perspective. Front. Genet. 4:48. doi: 10.3389/fgene.2013.00048
Denchi, E. L., and de Lange, T. (2007). Protection of telomeres through independent control of ATM and ATR by TRF2 and POT1. Nature 448, 1068–1071. doi: 10.1038/nature06065
Deng, W., Buzas, D. M., Ying, H., Robertson, M., Taylor, J., Peacock, W. J., et al. (2013). Arabidopsis Polycomb Repressive Complex 2 binding sites contain putative GAGA factor binding motifs within coding regions of genes. BMC Genomics 14:593. doi: 10.1186/1471-2164-14-593
Derboven, E., Ekker, H., Kusenda, B., Bulankova, P., and Riha, K. (2014). Role of STN1 and DNA polymerase alpha in telomere stability and genome-wide replication in Arabidopsis. PLoS Genet. 10:e1004682. doi: 10.1371/journal.pgen.1004682
Desveaux, D., Allard, J., Brisson, N., and Sygusch, J. (2002). A new family of plant transcription factors displays a novel ssDNA-binding surface. Nat. Struct. Biol. 9, 512–517. doi: 10.1038/nsb814
Desveaux, D., Despres, C., Joyeux, A., Subramaniam, R., and Brisson, N. (2000). PBF-2 is a novel single-stranded DNA binding factor implicated in PR-10a gene activation in potato. Plant Cell 12, 1477–1489. doi: 10.1105/tpc.12.8.1477
Dokládal, L., Honys, D., Rana, R., Lee, L.-Y., Gelvin, S., and Sýkorová, E. (2015). cDNA library screening identifies protein interactors potentially involved in non-telomeric roles of Arabidopsis telomerase. Front. Plant Sci. 6:985. doi: 10.3389/fpls.2015.00985
Dong, F., and Jiang, J. (1998). Non-Rabl patterns of centromere and telomere distribution in the interphase nuclei of plant cells. Chromosome Res. 6, 551–558. doi: 10.1023/A:1009280425125
Du, H., Wang, Y. B., Xie, Y., Liang, Z., Jiang, S. J., Zhang, S. S., et al. (2013). Genome-wide identification and evolutionary and expression analyses of MYB-related genes in land plants. DNA Res. 20, 437–448. doi: 10.1093/dnares/dst021
Dvořáčková, M., Fojtova, M., and Fajkus, J. (2015). Chromatin dynamics of plant telomeres and ribosomal genes. Plant J. 83, 18–37. doi: 10.1111/tpj.12822
Dvorácková, M., Rossignol, P., Shaw, P. J., Koroleva, O. A., Doonan, J. H., and Fajkus, J. (2010). AtTRB1, a telomeric DNA-binding protein from Arabidopsis, is concentrated in the nucleolus and shows highly dynamic association with chromatin. Plant J. 61, 637–649. doi: 10.1111/j.1365-313X.2009.04094.x
Fajkus, J., Fulneckova, J., Hulanova, M., Berkova, K., Riha, K., and Matyasek, R. (1998). Plant cells express telomerase activity upon transfer to callus culture, without extensively changing telomere lengths. Mol. Gen. Genet. 260, 470–474. doi: 10.1007/s004380050918
Fajkus, J., Kovarik, A., and Kralovics, R. (1996). Telomerase activity in plant cells. FEBS Lett. 391, 307–309. doi: 10.1016/0014-5793(96)00757-0
Fajkus, J., Kovarik, A., Kralovics, R., and Bezdek, M. (1995). Organization of telomeric and subtelomeric chromatin in the higher plant Nicotiana tabacum. Mol. Gen. Genet. 247, 633–638. doi: 10.1007/BF00290355
Fajkus, J., Sykorova, E., and Leitch, A. R. (2005). Telomeres in evolution and evolution of telomeres. Chromosome Res. 13, 469–479. doi: 10.1007/s10577-005-0997-2
Fajkus, J., and Trifonov, E. N. (2001). Columnar packing of telomeric nucleosomes. Biochem. Biophys. Res. Commun. 280, 961–963. doi: 10.1006/bbrc.2000.4208
Fajkus, P., Peska, V., Sitova, Z., Fulnecková, J., Dvorackova, M., Gogela, R., et al. (2016). Allium telomeres unmasked: the unusual telomeric sequence (CTCGGTTATGGG)n is synthesized by telomerase. Plant J. 85, 337–347. doi: 10.1111/tpj.13115
Feldbrugge, M., Sprenger, M., Hahlbrock, K., and Weisshaar, B. (1997). PcMYB1, a novel plant protein containing a DNA-binding domain with one MYB repeat, interacts in vivo with a light-regulatory promoter unit. Plant J. 11, 1079–1093. doi: 10.1046/j.1365-313X.1997.11051079.x
Fell, V. L., and Schild-Poulter, C. (2015). The Ku heterodimer: function in DNA repair and beyond. Mutat. Res. Rev. Mutat. Res. 763, 15–29. doi: 10.1016/j.mrrev.2014.06.002
Fojtova, M., Peska, V., Dobsakova, Z., Mozgova, I., Fajkus, J., and Sykorova, E. (2011). Molecular analysis of T-DNA insertion mutants identified putative regulatory elements in the AtTERT gene. J. Exp. Bot. 62, 5531–5545. doi: 10.1093/jxb/err235
Fojtova, M., Sykorova, E., Najdekrova, L., Polanska, P., Zachova, D., Vagnerova, R., et al. (2015). Telomere dynamics in the lower plant Physcomitrella patens. Plant Mol. Biol. 87, 591–601. doi: 10.1007/s11103-015-0299-9
Foyer, C. H., Karpinska, B., and Krupinska, K. (2014). The functions of WHIRLY1 and REDOX-RESPONSIVE TRANSCRIPTION FACTOR 1 in cross tolerance responses in plants: a hypothesis. Philos. Trans. R. Soc. Lond. B Biol. Sci. 369:20130226. doi: 10.1098/rstb.2013.0226
Freeling, M. (2009). Bias in plant gene content following different sorts of duplication: tandem, whole-genome, segmental, or by transposition. Annu. Rev. Plant Biol. 60, 433–453. doi: 10.1146/annurev.arplant.043008.092122
Fu, D., and Collins, K. (2007). Purification of human telomerase complexes identifies factors involved in telomerase biogenesis and telomere length regulation. Mol. Cell 28, 773–785. doi: 10.1016/j.molcel.2007.09.023
Fulcher, N., and Riha, K. (2016). Using centromere mediated genome elimination to elucidate the funcional redudancy of candidate telomere binding proteins in Arabidopsis thaliana. Front. Genet. 6:349. doi: 10.3389/fgene.2015.00349
Fulneckova, J., and Fajkus, J. (2000). Inhibition of plant telomerase by telomere-binding proteins from nuclei of telomerase-negative tissues. FEBS Lett. 467, 305–310. doi: 10.1016/S0014-5793(00)01178-9
Fulnecková, J., Sevcíková, T., Fajkus, J., Lukesová, A., Lukes, M., Vlcek, C., et al. (2013). A broad phylogenetic survey unveils the diversity and evolution of telomeres in eukaryotes. Genome Biol. Evol. 5, 468–483. doi: 10.1093/gbe/evt019
Fulnečková, J., Ševčikova, T., Lukešová, A., and Sýkorová, E. (2015). Transtitions between the Arabidopsis-type and the human-type telomere sequence in green algae (clade Caudivolvoxa, Chlamydomonadales). Chromosoma. doi: 10.1007/s00412-015-0557-2 [Epub ahead of print].
Gallego, M. E., Jalut, N., and White, C. I. (2003). Telomerase dependence of telomere lengthening in Ku80 mutant Arabidopsis. Plant Cell 15, 782–789. doi: 10.1105/tpc.008623
Gallego, M. E., and White, C. I. (2001). RAD50 function is essential for telomere maintenance in Arabidopsis. Proc. Natl. Acad. Sci. U.S.A. 98, 1711–1716. doi: 10.1073/pnas.98.4.1711
Garvik, B., Carson, M., and Hartwell, L. (1995). Single-stranded DNA arising at telomeres in cdc13 mutants may constitute a specific signal for the RAD9 checkpoint. Mol. Cell. Biol. 15, 6128–6613. doi: 10.1128/MCB.15.11.6128
Giraud-Panis, M. J., Pisano, S., Benarroch-Popivker, D., Pei, B., Le, Du, M. H., et al. (2013). One identity or more for telomeres? Front. Oncol. 3:48. doi: 10.3389/fonc.2013.00048
Giraud-Panis, M. J., Teixeira, M. T., Geli, V., and Gilson, E. (2010). CST meets shelterin to keep telomeres in check. Mol. Cell. 39, 665–676. doi: 10.1016/j.molcel.2010.08.024
Grabowski, E., Miao, Y., Mulisch, M., and Krupinska, K. (2008). Single-stranded DNA-binding protein Whirly1 in barley leaves is located in plastids and the nucleus of the same cell. Plant Physiol. 147, 1800–1804. doi: 10.1104/pp.108.122796
Grandin, N., and Charbonneau, M. (2001). Hsp90 levels affect telomere length in yeast. Mol. Genet. Genom. 265, 126–134. doi: 10.1007/s004380000398
Graumann, K., Runions, J., and Evans, D. E. (2010). Characterization of SUN-domain proteins at the higher plant nuclear envelope. Plant J. 61, 134–144. doi: 10.1111/j.1365-313X.2009.04038.x
Grossi, S., Puglisi, A., Dmitriev, P. V., Lopes, M., and Shore, D. (2004). Pol12, the B subunit of DNA polymerase alpha, functions in both telomere capping and length regulation. Genes Dev. 18, 992–1006. doi: 10.1101/gad.300004
Guo, X., Deng, Y., Lin, Y., Cosme-Blanco, W., Chan, S., He, H., et al. (2007). Dysfunctional telomeres activate an ATM-ATR-dependent DNA damage response to suppress tumorigenesis. EMBO J. 26, 4709–4719.
Harrington, L., McPhail, T., Mar, V., Zhou, W., Oulton, R., Bass, M. B., et al. (1997). A mammalian telomerase-associated protein. Science 275, 973–977. doi: 10.1126/science.275.5302.973
He, H., Multani, A. S., Cosme-Blanco, W., Tahara, H., Ma, J., Pathak, S., et al. (2006). POT1b protects telomeres from end-to-end chromosomal fusions and aberrant homologous recombination. EMBO J. 25, 5180–5190. doi: 10.1038/sj.emboj.7601294
He, Q., Chen, L., Xu, Y., and Yu, W. (2013). Identification of centromeric and telomeric DNA-binding proteins in rice. Proteomics 13, 826–832. doi: 10.1002/pmic.201100416
He, X. L., and Zhang, J. Z. (2005). Gene complexity and gene duplicability. Curr. Biol. 15, 1016–1021. doi: 10.1016/j.cub.2005.04.035
Heller, K., Kilian, A., Piatyszek, M. A., and Kleinhofs, A. (1996). Telomerase activity in plant extracts. Mol. Gen. Genet. 252, 342–345. doi: 10.1007/BF02173780
Higgins, J. D., Perry, R. M., Barakate, A., Ramsay, L., Waugh, R., Halpin, C., et al. (2012). Spatiotemporal asymmetry of the meiotic program underlies the predominantly distal distribution of meiotic crossovers in barley. Plant Cell 24, 4096–4109. doi: 10.1105/tpc.112.102483
Hirata, Y., Suzuki, C., and Sakai, S. (2004). Characterization and gene cloning of telomere-binding protein from tobacco BY-2 cells. Plant Physiol. Biochem. 42, 7–14. doi: 10.1016/j.plaphy.2003.10.002
Hofr, C., Sultesova, P., Zimmermann, M., Mozgova, I., Prochazkova Schrumpfova, P., Wimmerova, M., et al. (2009). Single-Myb-histone proteins from Arabidopsis thaliana: a quantitative study of telomere-binding specificity and kinetics. Biochem. J. 419, 221–228. doi: 10.1042/BJ20082195, 222 p following 228.
Holt, S. E., Aisner, D. L., Baur, J., Tesmer, V. M., Dy, M., Ouellette, M., et al. (1999). Functional requirement of p23 and Hsp90 in telomerase complexes. Genes Dev. 13, 817–826. doi: 10.1101/gad.13.7.817
Hong, J. P., Byun, M. Y., An, K., Yang, S. J., An, G., and Kim, W. T. (2010). OsKu70 is associated with developmental growth and genome stability in rice. Plant Physiol. 152, 374–387. doi: 10.1104/pp.109.150391
Hong, J. P., Byun, M. Y., Koo, D. H., An, K., Bang, J. W., Chung, I. K., et al. (2007). Suppression of RICE TELOMERE BINDING PROTEIN 1 results in severe and gradual developmental defects accompanied by genome instability in rice. Plant Cell 19, 1770–1781. doi: 10.1105/tpc.107.051953
Hrdlickova, R., Nehyba, J., Liss, A. S., and Bose, H. R. Jr. (2006). Mechanism of telomerase activation by v-Rel and its contribution to transformation. J. Virol. 80, 281–295. doi: 10.1128/JVI.80.1.281-295.2006
Hwang, M. G., Chung, I. K., Kang, B. G., and Cho, M. H. (2001). Sequence-specific binding property of Arabidopsis thaliana telomeric DNA binding protein 1 (AtTBP1). FEBS Lett. 503, 35–40. doi: 10.1016/S0014-5793(01)02685-0
Hwang, M. G., Kim, K., Lee, W. K., and Cho, M. H. (2005). AtTBP2 and AtTRP2 in Arabidopsis encode proteins that bind plant telomeric DNA and induce DNA bending in vitro. Mol. Gen. Genom. 273, 66–75. doi: 10.1007/s00438-004-1096-3
Idziak, D., Robaszkiewicz, E., and Hasterok, R. (2015). Spatial distribution of centromeres and telomeres at interphase varies among Brachypodium species. J. Exp. Bot. 66, 6623–6634. doi: 10.1093/jxb/erv369
Janouskova, E., Necasova, I., Pavlouskova, J., Zimmermann, M., Hluchy, M., Marini, V., et al. (2015). Human Rap1 modulates TRF2 attraction to telomeric DNA. Nucleic Acids Res. 43, 2691–2700. doi: 10.1093/nar/gkv097
Kaminker, P. G., Kim, S. H., Desprez, P. Y., and Campisi, J. (2009). A novel form of the telomere-associated protein TIN2 localizes to the nuclear matrix. Cell Cycle 8, 931–939. doi: 10.4161/cc.8.6.7941
Kang, S. S., Kwon, T., Kwon, D. Y., and Do, S. I. (1999). Akt protein kinase enhances human telomerase activity through phosphorylation of telomerase reverse transcriptase subunit. J. Biol. Chem. 274, 13085–13090. doi: 10.1074/jbc.274.19.13085
Kannan, K., Nelson, A. D., and Shippen, D. E. (2008). Dyskerin is a component of the Arabidopsis telomerase RNP required for telomere maintenance. Mol. Cell. Biol. 28, 2332–2341. doi: 10.1128/MCB.01490-07
Karamysheva, Z. N., Surovtseva, Y. V., Vespa, L., Shakirov, E. V., and Shippen, D. E. (2004). A C-terminal Myb extension domain defines a novel family of double-strand telomeric DNA-binding proteins in Arabidopsis. J. Biol. Chem. 279, 47799–47807. doi: 10.1074/jbc.M407938200
Kasbek, C., Wang, F., and Price, C. M. (2013). Human TEN1 maintains telomere integrity and functions in genome-wide replication restart. J. Biol. Chem. 288, 30139–30150. doi: 10.1074/jbc.M113.493478
Kazda, A., Zellinger, B., Rossler, M., Derboven, E., Kusenda, B., and Riha, K. (2012). Chromosome end protection by blunt-ended telomeres. Genes Dev. 26, 1703–1713. doi: 10.1101/gad.194944.112
Kim, J. H., Kim, W. T., and Chung, I. K. (1998). Rice proteins that bind single-stranded G-rich telomere DNA. Plant Mol. Biol. 36, 661–672. doi: 10.1023/A:1005994719175
Kiss, T., Fayet-Lebaron, E., and Jady, B. E. (2010). Box H/ACA small ribonucleoproteins. Mol. Cell. 37, 597–606. doi: 10.1016/j.molcel.2010.01.032
Ko, S., Jun, S. H., Bae, H., Byun, J. S., Han, W., Park, H., et al. (2008). Structure of the DNA-binding domain of NgTRF1 reveals unique features of plant telomere-binding proteins. Nucleic Acids Res. 36, 2739–2755. doi: 10.1093/nar/gkn030
Koonin, E. V. (2010). Preview. The incredible expanding ancestor of eukaryotes. Cell 140, 606–608. doi: 10.1016/j.cell.2010.02.022
Kovarik, A., Fajkus, J., Koukalova, B., and Bezdek, M. (1996). Species-specific evolution of telomeric and rDNA repeats in the tobacco composite genome. Theor. Appl. Genet. 92, 1108–1111. doi: 10.1007/BF00224057
Krause, K., Kilbienski, I., Mulisch, M., Rodiger, A., Schafer, A., and Krupinska, K. (2005). DNA-binding proteins of the Whirly family in Arabidopsis thaliana are targeted to the organelles. FEBS Lett. 579, 3707–3712. doi: 10.1016/j.febslet.2005.05.059
Krutilina, R. I., Oei, S., Buchlow, G., Yau, P. M., Zalensky, A. O., Zalenskaya, I. A., et al. (2001). A negative regulator of telomere-length protein trf1 is associated with interstitial (TTAGGG)n blocks in immortal Chinese hamster ovary cells. Biochem. Biophys. Res. Commun. 280, 471–475. doi: 10.1006/bbrc.2000.4143
Kuchar, M., and Fajkus, J. (2004). Interactions of putative telomere-binding proteins in Arabidopsis thaliana: identification of functional TRF2 homolog in plants. FEBS Lett. 578, 311–315. doi: 10.1016/j.febslet.2004.11.021
Kwon, C., and Chung, I. K. (2004). Interaction of an Arabidopsis RNA-binding protein with plant single-stranded telomeric DNA modulates telomerase activity. J. Biol. Chem. 279, 12812–12818. doi: 10.1074/jbc.M312011200
Kwon, C., Kwon, K., Chung, I. K., Kim, S. Y., Cho, M. H., and Kang, B. G. (2004). Characterization of single stranded telomeric DNA-binding proteins in cultured soybean (Glycine max) cells. Mol. Cells 17, 503–508.
Lamarche, B. J., Orazio, N. I., and Weitzman, M. D. (2010). The MRN complex in double-strand break repair and telomere maintenance. FEBS Lett. 584, 3682–3695. doi: 10.1016/j.febslet.2010.07.029
Lazzerini-Denchi, E., and Sfeir, A., (2016). Stop pulling my strings – what telomeres taught us about the DNA damage response. Nat. Rev. Mol. Cell. Biol. 17, 364–378. doi: 10.1038/nrm.2016.43
Lee, J. H., Kim, J. H., Kim, W. T., Kang, B. G., and Chung, I. K. (2000). Characterization and developmental expression of single-stranded telomeric DNA-binding proteins from mung bean (Vigna radiata). Plant Mol. Biol. 42, 547–557. doi: 10.1023/A:1006373917321
Lee, L. Y., Wu, F. H., Hsu, C. T., Shen, S. C., Yeh, H. Y., Liao, D. C., et al. (2012). Screening a cDNA library for protein-protein interactions directly in planta. Plant Cell 24, 1746–1759. doi: 10.1105/tpc.112.097998
Lee, W. K., and Cho, M. H. (2016). Telomere-binding protein regulates the chromosome ends through the interaction with histone deacetylases in Arabidopsis thaliana. Nucl. Acids Res. 44, 4610–4624. doi: 10.1093/nar/gkw067
Lee, W. K., Yun, J. H., Lee, W., and Cho, M. H. (2012). DNA-binding domain of AtTRB2 reveals unique features of a single Myb histone protein family that binds to both Arabidopsis- and human-type telomeric DNA sequences. Mol. Plant 5, 1406–1408. doi: 10.1093/mp/sss063
Lee, Y. W., and Kim, W. T. (2010). Tobacco GTBP1, a homolog of human heterogeneous nuclear ribonucleoprotein, protects telomeres from aberrant homologous recombination. Plant Cell 22, 2781–2795. doi: 10.1105/tpc.110.076778
Lee, Y. W., and Kim, W. T. (2013). Telomerase-dependent 3′ G-strand overhang maintenance facilitates GTBP1-mediated telomere protection from misplaced homologous recombination. Plant Cell 25, 1329–1342. doi: 10.1105/tpc.112.107573
Leehy, K. A., Lee, J. R., Song, X., Renfrew, K. B., and Shippen, D. E. (2013). MERISTEM DISORGANIZATION1 encodes TEN1, an essential telomere protein that modulates telomerase processivity in Arabidopsis. Plant Cell 25, 1343–1354. doi: 10.1105/tpc.112.107425
Lei, M., Baumann, P., and Cech, T. R. (2002). Cooperative binding of single-stranded telomeric DNA by the Pot1 protein of Schizosaccharomyces pombe. Biochemistry 41, 14560–14568. doi: 10.1021/bi026674z
Lermontova, I., Schubert, V., Bornke, F., Macas, J., and Schubert, I. (2007). Arabidopsis CBF5 interacts with the H/ACA snoRNP assembly factor NAF1. Plant Mol. Biol. 65, 615–626. doi: 10.1007/s11103-007-9226-z
Linger, B. R., and Price, C. M. (2009). Conservation of telomere protein complexes: shuffling through evolution. Crit. Rev. Biochem. Mol. Biol. 44, 434–446. doi: 10.3109/10409230903307329
Lugert, T., and Werr, W. (1994). A novel DNA-binding domain in the Shrunken initiator-binding protein (IBP1). Plant Mol. Biol. 25, 493–506. doi: 10.1007/BF00043877
Maï, M. E., Wagner, K. D., Michiels, J. F., Ambrosetti, D., Borderie, A., Destree, S., et al. (2014). The telomeric protein TRF2 regulates Angiogenesis by binding and activating the PDGFRβ promoter. Cell Rep. 9, 1047–1060. doi: 10.1016/j.celrep.2014.09.038
Maillet, G., White, C. I., and Gallego, M. E. (2006). Telomere-length regulation in inter-ecotype crosses of Arabidopsis. Plant Mol. Biol. 62, 859–866. doi: 10.1007/s11103-006-9061-7
Majerova, E., Fojtova, M., Mozgova, I., Bittova, M., and Fajkus, J. (2011). Hypomethylating drugs efficiently decrease cytosine methylation in telomeric DNA and activate telomerase without affecting telomere lengths in tobacco cells. Plant Mol. Biol. 77, 371–380. doi: 10.1007/s11103-011-9816-7
Mandakova, T., and Lysak, M. A. (2008). Chromosomal phylogeny and karyotype evolution in x = 7 crucifer species (Brassicaceae). Plant Cell 20, 2559–2570. doi: 10.1105/tpc.108.062166
Marian, C. O., and Bass, H. W. (2005). The terminal acidic SANT 1 (Tacs1) gene of maize is expressed in tissues containing meristems and encodes an acidic SANT domain similar to some chromatin-remodeling complex proteins. Biochim. Biophys. Acta 1727, 81–86. doi: 10.1016/j.bbaexp.2004.12.010
Marian, C. O., Bordoli, S. J., Goltz, M., Santarella, R. A., Jackson, L. P., Danilevskaya, O., et al. (2003). The maize single myb histone 1 gene, Smh1, belongs to a novel gene family and encodes a protein that binds telomere DNA repeats in vitro. Plant Physiol. 133, 1336–1350.
Martinez, P., Thanasoula, M., Carlos, A. R., Gomez-Lopez, G., Tejera, A. M., Schoeftner, S., et al. (2010). Mammalian Rap1 controls telomere function and gene expression through binding to telomeric and extratelomeric sites. Nat. Cell Biol. 12, 768–780. doi: 10.1038/ncb2081
Martinez-Perez, E., Shaw, P., Reader, S., Aragon-Alcaide, L., Miller, T., and Moore, G. (1999). Homologous chromosome pairing in wheat. J. Cell Sci. 112, 1761–1769.
Mignon-Ravix, C., Depetris, D., Delobel, B., Croquette, M. F., and Mattei, M. G. (2002). A human interstitial telomere associates in vivo with specific TRF2 and TIN2 proteins. Eur. J. Hum. Genet. 10, 107–112. doi: 10.1038/sj.ejhg.5200775
Mimori, T., Akizuki, M., Yamagata, H., Inada, S., Yoshida, S., and Homma, M. (1981). Characterization of a high molecular weight acidic nuclear protein recognized by autoantibodies in sera from patients with polymyositis-scleroderma overlap. J. Clin. Invest. 68, 611–620. doi: 10.1172/JCI110295
Moore, J. (2009). Investigating the DNA Binding Properties of the Initiator Binding Protein 2 (IBP2) in Maize (Zea Mays). Master thesis, Florida State University, Tallahassee.
Moriguchi, R., Kanahama, K., and Kanayama, Y. (2006). Characterization and expression analysis of the tomato telomere-binding protein LeTBP1. Plant Sci. 171, 166–174. doi: 10.1016/j.plantsci.2006.03.010
Mozgova, I., Schrumpfova, P. P., Hofr, C., and Fajkus, J. (2008). Functional characterization of domains in AtTRB1, a putative telomere-binding protein in Arabidopsis thaliana. Phytochemistry 69, 1814–1819. doi: 10.1016/j.phytochem.2008.04.001
Najdekrova, L., and Siroky, J. (2012). NBS1 plays a synergistic role with telomerase in the maintenance of telomeres in Arabidopsis thaliana. BMC Plant Biol. 12:167. doi: 10.1186/1471-2229-12-167
Nelson, A. D. J. (2012). Telomerase regulation in Arabidopsis thaliana. Ph.D. thesis, A&M University, Texas.
Nelson, A. D. J., Forsythe, E. S., Gan, X., Tsiantis, M., and Beilstein, M. A. (2014). Extending the model of Arabidopsis telomere length and composition across Brassicaceae. Chromosome Res. 22, 153–166. doi: 10.1007/s10577-014-9423-y
Oguchi, K., Tamura, K., and Takahashi, H. (2004). Characterization of Oryza sativa telomerase reverse transcriptase and possible role of its phosphorylation in the control of telomerase activity. Gene 342, 57–66. doi: 10.1016/j.gene.2004.07.011
Olivier, M., Da Ines, O., Amiard, S., Serra, H., Goubely, C., White, C., et al. (2016). The structure-specific endonucleases MUS81 and SEND1 ere essential for telomere stability in Arabidopsis. Plant Cell 28, 74–86. doi: 10.1105/tpc.15.00898
Ottaviani, A., Gilson, E., and Magdinier, F. (2008). Telomeric position effect: from the yeast paradigm to human pathologies? Biochimie 90, 93–107. doi: 10.1016/j.biochi.2007.07.022
Peska, V., Fajkus, P., Fojtova, M., Dvorackova, M., Hapala, J., Dvoracek, V., et al. (2015). Characterisation of an unusual telomere motif (TTTTTTAGGG)n in the plant Cestrum elegans (Solanaceae), a species with a large genome. Plant J. 82, 644–654. doi: 10.1111/tpj.12839
Peska, V., Schrumpfova, P. P., and Fajkus, J. (2011). Using the telobox to search for plant telomere binding proteins. Curr. Protein Pept. Sci. 12, 75–83. doi: 10.2174/138920311795684968
Peska, V., Sykorova, E., and Fajkus, J. (2008). Two faces of Solanaceae telomeres: a comparison between Nicotiana and Cestrum telomeres and telomere-binding proteins. Cytogenet. Genome. Res. 122, 380–387. doi: 10.1159/000167826
Phillips, D., Nibau, C., Wnetrzak, J., and Jenkins, G. (2012). High resolution analysis of meiotic chromosome structure and behaviour in barley (Hordeum vulgare L.). PLoS ONE 7:e39539. doi: 10.1371/journal.pone.0039539
Pisano, S., Galati, A., and Cacchione, S. (2008). Telomeric nucleosomes: forgotten players at chromosome ends. Cell Mol. Life. Sci. 65, 3553–3563. doi: 10.1007/s00018-008-8307-8
Podlevsky, J. D., Bley, C. J., Omana, R. V., Qi, X., and Chen, J. J. (2008). The telomerase database. Nucleic Acids Res. 36, D339–D343. doi: 10.1093/nar/gkm700
Price, C. M., Boltz, K. A., Chaiken, M. F., Stewart, J. A., Beilstein, M. A., and Shippen, D. E. (2010). Evolution of CST function in telomere maintenance. Cell Cycle 9, 3157–3165. doi: 10.4161/cc.9.16.12547
Price, C. M., and Cech, T. R. (1987). Telomeric DNA-protein interactions of Oxytricha macronuclear DNA. Genes Dev. 1, 783–793. doi: 10.1101/gad.1.8.783
Qi, H., and Zakian, V. A. (2000). The Saccharomyces telomere-binding protein Cdc13p interacts with both the catalytic subunit of DNA polymerase alpha and the telomerase-associated est1 protein. Genes Dev. 14, 1777–1788.
Recker, J., Knoll, A., and Puchta, H. (2014). The Arabidopsis thaliana homolog of the helicase RTEL1 plays multiple roles in preserving genome stability. Plant Cell 26, 4889–4902. doi: 10.1105/tpc.114.132472
Ren, S., Johnston, J. S., Shippen, D. E., and McKnight, T. D. (2004). TELOMERASE ACTIVATOR1 induces telomerase activity and potentiates responses to auxin in Arabidopsis. Plant Cell 16, 2910–2922. doi: 10.1105/tpc.104.025072
Ren, S., Mandadi, K. K., Boedeker, A. L., Rathore, K. S., and McKnight, T. D. (2007). Regulation of telomerase in Arabidopsis by BT2, an apparent target of TELOMERASE ACTIVATOR1. Plant Cell 19, 23–31. doi: 10.1105/tpc.106.044321
Renfrew, K. B., Song, X., Lee, J. R., Arora, A., and Shippen, D. E. (2014). POT1a and components of CST engage telomerase and regulate its activity in Arabidopsis. PLoS Genet. 10:e1004738. doi: 10.1371/journal.pgen.1004738
Richards, E. J., and Ausubel, F. M. (1988). Isolation of a higher eukaryotic telomere from Arabidopsis thaliana. Cell 53, 127–136. doi: 10.1016/0092-8674(88)90494-1
Riha, K., Fajkus, J., Siroky, J., and Vyskot, B. (1998). Developmental control of telomere lengths and telomerase activity in plants. Plant Cell 10, 1691–1698. doi: 10.2307/3870766
Riha, K., McKnight, T. D., Fajkus, J., Vyskot, B., and Shippen, D. E. (2000). Analysis of the G-overhang structures on plant telomeres: evidence for two distinct telomere architectures. Plant J. 23, 633–641. doi: 10.1046/j.1365-313x.2000.00831.x
Riha, K., Watson, J. M., Parkey, J., and Shippen, D. E. (2002). Telomere length deregulation and enhanced sensitivity to genotoxic stress in Arabidopsis mutants deficient in Ku70. EMBO J. 21, 2819–2826. doi: 10.1093/emboj/21.11.2819
Rossignol, P., Collier, S., Bush, M., Shaw, P., and Doonan, J. H. (2007). Arabidopsis POT1A interacts with TERT-V(I8), an N-terminal splicing variant of telomerase. J. Cell Sci. 120, 3678–3687. doi: 10.1242/jcs.004119
Rotkova, G., Sykorova, E., and Fajkus, J. (2009). Protect and regulate: Recent findings on plant POT1-like proteins. Biol. Plant. 53, 1–4. doi: 10.1007/s10535-009-0001-7
Ruckova, E., Friml, J., Prochazkova Schrumpfova, P., and Fajkus, J. (2008). Role of alternative telomere lengthening unmasked in telomerase knock-out mutant plants. Plant Mol. Biol. 66, 637–646. doi: 10.1007/s11103-008-9295-7
Schmidt, J. C., and Cech, T. R. (2015). Human telomerase: biogenesis, trafficking, recruitment, and activation. Genes Dev. 29, 1095–1105. doi: 10.1101/gad.263863.115
Schober, H., Ferreira, H., Kalck, V., Gehlen, L. R., and Gasser, S. M. (2009). Yeast telomerase and the SUN domain protein Mps3 anchor telomeres and repress subtelomeric recombination. Genes Dev. 23, 928–938. doi: 10.1101/gad.1787509
Schrumpfova, P., Kuchar, M., Mikova, G., Skrisovska, L., Kubicarova, T., and Fajkus, J. (2004). Characterization of two Arabidopsis thaliana myb-like proteins showing affinity to telomeric DNA sequence. Genome 47, 316–324. doi: 10.1139/g03-136
Schrumpfova, P. P., Kuchar, M., Palecek, J., and Fajkus, J. (2008). Mapping of interaction domains of putative telomere-binding proteins AtTRB1 and AtPOT1b from Arabidopsis thaliana. FEBS Lett. 582, 1400–1406. doi: 10.1016/j.febslet.2008.03.034
Schrumpfova, P. P., Vychodilova, I., Dvorackova, M., Majerska, J., Dokladal, L., Schorova, S., et al. (2014). Telomere repeat binding proteins are functional components of Arabidopsis telomeres and interact with telomerase. Plant J. 77, 770–781. doi: 10.1111/tpj.12428
Schrumpfova, P. P., Vychodilova, I., Hapala, J., Schorova, S., Dvoracek, V., and Fajkus, J. (2016). Telomere binding protein TRB1 is associated with promoters of translation machinery genes in vivo. Plant. Mol. Biol. 90, 189–206. doi: 10.1007/s11103-015-0409-8
Schwacke, R., Fischer, K., Ketelsen, B., Krupinska, K., and Krause, K. (2007). Comparative survey of plastid and mitochondrial targeting properties of transcription factors in Arabidopsis and rice. Mol. Genet. Genom. 277, 631–646. doi: 10.1007/s00438-007-0214-4
Shakirov, E. V., McKnight, T. D., and Shippen, D. E. (2009a). POT1-independent single-strand telomeric DNA binding activities in Brassicaceae. Plant J. 58, 1004–1015. doi: 10.1111/j.1365-313X.2009.03837.x
Shakirov, E. V., Perroud, P. F., Nelson, A. D., Cannell, M. E., Quatrano, R. S., and Shippen, D. E. (2010). Protection of telomeres 1 is required for telomere integrity in the moss Physcomitrella patens. Plant Cell 22, 1838–1848. doi: 10.1105/tpc.110.075846
Shakirov, E. V., Song, X., Joseph, J. A., and Shippen, D. E. (2009b). POT1 proteins in green algae and land plants: DNA-binding properties and evidence of co-evolution with telomeric DNA. Nucleic Acids Res. 37, 7455–7467. doi: 10.1093/nar/gkp785
Shakirov, E. V., and Shippen, D. E. (2004). Length regulation and dynamics of individual telomere tracts in wild-type Arabidopsis. Plant Cell 16, 1959–1967. doi: 10.1105/tpc.104.023093
Shakirov, E. V., Surovtseva, Y. V., Osbun, N., and Shippen, D. E. (2005). The Arabidopsis Pot1 and Pot2 proteins function in telomere length homeostasis and chromosome end protection. Mol. Cell. Biol. 25, 7725–7733. doi: 10.1128/MCB.25.17.7725-7733.2005
Simonet, T., Zaragosi, L. E., Philippe, C., Lebrigand, K., Schouteden, C., Augereau, A., et al. (2011). The human TTAGGG repeat factors 1 and 2 bind to a subset of interstitial telomeric sequences and satellite repeats. Cell Res. 21, 1028–1038. doi: 10.1038/cr.2011.40
Smith, S., Giriat, I., Schmitt, A., and de Lange, T. (1998). Tankyrase, a poly(ADP-ribose) polymerase at human telomeres. Science 282, 1484–1487. doi: 10.1126/science.282.5393.1484
Smogorzewska, A., van Steensel, B., Bianchi, A., Oelmann, S., Schaefer, M. R., Schnapp, G., et al. (2000). Control of human telomere length by TRF1 and TRF2. Mol. Cell. Biol. 20, 1659–1668. doi: 10.1128/MCB.20.5.1659-1668.2000
Song, X., Leehy, K., Warrington, R. T., Lamb, J. C., Surovtseva, Y. V., and Shippen, D. E. (2008). STN1 protects chromosome ends in Arabidopsis thaliana. Proc. Natl. Acad. Sci. U.S.A. 105, 19815–19820. doi: 10.1073/pnas.0807867105
Starr, D. A., Hermann, G. J., Malone, C. J., Fixsen, W., Priess, J. R., Horvitz, H. R., et al. (2001). unc-83 encodes a novel component of the nuclear envelope and is essential for proper nuclear migration. Development 128, 5039–5050.
Stewart, J. A., Wang, F., Chaiken, M. F., Kasbek, C., Chastain, P. D. II, Wright, W. E., et al. (2012). Human CST promotes telomere duplex replication and general replication restart after fork stalling. EMBO J. 31, 3537–3549. doi: 10.1038/emboj.2012.215
Surovtseva, Y. V., Churikov, D., Boltz, K. A., Song, X., Lamb, J. C., Warrington, R., et al. (2009). Conserved telomere maintenance component 1 interacts with STN1 and maintains chromosome ends in higher eukaryotes. Mol. Cell. 36, 207–218. doi: 10.1016/j.molcel.2009.09.017
Surovtseva, Y. V., Shakirov, E. V., Vespa, L., Osbun, N., Song, X., and Shippen, D. E. (2007). Arabidopsis POT1 associates with the telomerase RNP and is required for telomere maintenance. EMBO J. 26, 3653–3661. doi: 10.1038/sj.emboj.7601792
Sykorova, E., and Fajkus, J. (2009). Structure-function relationships in telomerase genes. Biol. Cell 101, 375–392. doi: 10.1042/BC20080205.
Sykorova, E., Fulneckova, J., Mokros, P., Fajkus, J., Fojtova, M., and Peska, V. (2012). Three TERT genes in Nicotiana tabacum. Chromosome Res. 20, 381–394. doi: 10.1007/s10577-012-9282-3
Sykorova, E., Leitch, A. R., and Fajkus, J. (2006). Asparagales telomerases which synthesize the human type of telomeres. Plant Mol. Biol. 60, 633–646. doi: 10.1007/s11103-005-5091-9
Sykorova, E., Lim, K. Y., Kunicka, Z., Chase, M. W., Bennett, M. D., Fajkus, J., et al. (2003). Telomere variability in the monocotyledonous plant order Asparagales. Proc. Biol. Sci. 270, 1893–1904. doi: 10.1098/rspb.2003.2446
Tamura, K., Goto, C., and Hara-Nishimura, I. (2015). Recent advances in understanding plant nuclear envelope proteins involved in nuclear morphology. J. Exp. Bot. 66, 1641–1647. doi: 10.1093/jxb/erv036
Tamura, K., Liu, H., and Takahashi, H. (1999). Auxin induction of cell cycle regulated activity of tobacco telomerase. J. Biol. Chem. 274, 20997–21002. doi: 10.1074/jbc.274.30.20997
Tani, A., and Murata, M. (2005). Alternative splicing of Pot1 (Protection of telomere)-like genes in Arabidopsis thaliana. Genes Genet. Syst. 80, 41–48. doi: 10.1266/ggs.80.41
Taylor, J. S., and Raes, J. (2004). Duplication and divergence: the evolution of new genes and old ideas. Annu. Rev. Genet. 38, 615–643. doi: 10.1146/annurev.genet.38.072902.092831
Tiang, C. L., He, Y., and Pawlowski, W. P. (2012). Chromosome organization and dynamics during interphase, mitosis, and meiosis in plants. Plant Physiol. 158, 26–34. doi: 10.1104/pp.111.187161
Ting, N. S., Yu, Y., Pohorelic, B., Lees-Miller, S. P., and Beattie, T. L. (2005). Human Ku70/80 interacts directly with hTR, the RNA component of human telomerase. Nucleic Acids Res. 33, 2090–2098. doi: 10.1093/nar/gki342
To, T. K., Kim, J. M., Matsui, A., Kurihara, Y., Morosawa, T., Ishida, J., et al. (2011). Arabidopsis HDA6 regulates locus-directed heterochromatin silencing in cooperation with MET1. PLoS Genet. 7:e1002055. doi: 10.1371/journal.pgen.1002055
Tomaska, L., Nosek, J., Kramara, J., and Griffith, J. D. (2009). Telomeric circles: universal players in telomere maintenance? Nat. Struct. Mol. Biol. 16, 1010–1015. doi: 10.1038/nsmb.1660
Tran, D. T., Cao, H. X., Jovtchev, G., Neumann, P., Novak, P., Fojtova, M., et al. (2015). Centromere and telomere sequence alterations reflect the rapid genome evolution within the carnivorous plant genus Genlisea. Plant J. 84, 1087–1099. doi: 10.1111/tpj.13058
Uringa, E. J., Youds, J. L., Lisaingo, K., Lansdorp, P. M., and Boulton, S. J. (2011). RTEL1: an essential helicase for telomere maintenance and the regulation of homologous recombination. Nucleic Acids Res. 39, 1647–1655. doi: 10.1093/nar/gkq1045
van der Fits, L., Zhang, H., Menke, F. L., Deneka, M., and Memelink, J. (2000). A Catharanthus roseus BPF-1 homologue interacts with an elicitor-responsive region of the secondary metabolite biosynthetic gene Str and is induced by elicitor via a JA-independent signal transduction pathway. Plant Mol. Biol. 44, 675–685. doi: 10.1023/A:1026526522555
Vannier, J. B., Depeiges, A., White, C., and Gallego, M. E. (2009). ERCC1/XPF protects short telomeres from homologous recombination in Arabidopsis thaliana. PLoS Genet. 5:e1000380. doi: 10.1371/journal.pgen.1000380
Vannier, J. B., Pavicic-Kaltenbrunner, V., Petalcorin, M. I., Ding, H., and Boulton, S. J. (2012). RTEL1 dismantles T loops and counteracts telomeric G4-DNA to maintain telomere integrity. Cell 149, 795–806. doi: 10.1016/j.cell.2012.03.030
Venteicher, A. S., Meng, Z., Mason, P. J., Veenstra, T. D., and Artandi, S. E. (2008). Identification of ATPases pontin and reptin as telomerase components essential for holoenzyme assembly. Cell 132, 945–957. doi: 10.1016/j.cell.2008.01.019
Vespa, L., Warrington, R. T., Mokros, P., Siroky, J., and Shippen, D. E. (2007). ATM regulates the length of individual telomere tracts in Arabidopsis. Proc. Natl. Acad. Sci. U.S.A. 104, 18145–18150. doi: 10.1073/pnas.0704466104
Vrbsky, J., Akimcheva, S., Watson, J. M., Turner, T. L., Daxinger, L., Vyskot, B., et al. (2010). siRNA-mediated methylation of Arabidopsis telomeres. PLoS Genet. 6:e1000986. doi: 10.1371/journal.pgen.1000986
Wang, H., Liu, C., Cheng, J., Liu, J., Zhang, L., He, C., et al. (2016). Arabidopsis flower and embryo developmental genes are repressed in seedlings by different combinations of polycomb group proteins in association with distinct sets of cis-regulatory elements. PLoS Genet. 12:e1005771. doi: 10.1371/journal.pgen.1005771
Wang, Y., Ghosh, G., and Hendrickson, E. A. (2009). Ku86 represses lethal telomere deletion events in human somatic cells. Proc. Natl. Acad. Sci. U.S.A. 106, 12430–12435. doi: 10.1073/pnas.0903362106
Watson, J. M., and Shippen, D. E. (2007). Telomere rapid deletion regulates telomere length in Arabidopsis thaliana. Mol. Cell. Biol. 27, 1706–1715. doi: 10.1128/MCB.02059-06
Wei, C., and Price, C. M. (2004). Cell cycle localization, dimerization, and binding domain architecture of the telomere protein cPot1. Mol. Cell. Biol. 24, 2091–2102. doi: 10.1128/MCB.24.5.2091-2102.2004
Weiss, H., and Scherthan, H. (2002). Aloe spp. - plants with vertebrate-like telomeric sequences. Chromosome Res. 10, 155–164. doi: 10.1023/A:1014905319557
Wellinger, R. J., and Zakian, V. A. (2012). Everything you ever wanted to know about Saccharomyces cerevisiae telomeres: beginning to end. Genetics 191, 1073–1105. doi: 10.1534/genetics.111.137851
Wen, R., Moore, G., and Shaw, P. J. (2012). Centromeres cluster de novo at the beginning of meiosis in Brachypodium distachyon. PLoS ONE 7:e44681. doi: 10.1371/journal.pone.0044681
Wu, L., Multani, A. S., He, H., Cosme-Blanco, W., Deng, Y., Deng, J. M., et al. (2006). Pot1 deficiency initiates DNA damage checkpoint activation and aberrant homologous recombination at telomeres. Cell 126, 49–62. doi: 10.1016/j.cell.2006.05.037
Yang, D., Xiong, Y., Kim, H., He, Q., Li, Y., Chen, R., et al. (2011). Human telomeric proteins occupy selective interstitial sites. Cell Res. 21, 1013–1027. doi: 10.1038/cr.2011.39
Yang, S. W., Jin, E., Chung, I. K., and Kim, W. T. (2002). Cell cycle-dependent regulation of telomerase activity by auxin, abscisic acid and protein phosphorylation in tobacco BY-2 suspension culture cells. Plant J. 29, 617–626. doi: 10.1046/j.0960-7412.2001.01244.x
Yang, S. W., Kim, D. H., Lee, J. J., Chun, Y. J., Lee, J. H., Kim, Y. J., et al. (2003). Expression of the telomeric repeat binding factor gene NgTRF1 is closely coordinated with the cell division program in tobacco BY-2 suspension culture cells. J. Biol. Chem. 278, 21395–21407. doi: 10.1074/jbc.M209973200
Yang, S. W., Kim, S. K., and Kim, W. T. (2004). Perturbation of NgTRF1 expression induces apoptosis-like cell death in tobacco BY-2 cells and implicates NgTRF1 in the control of telomere length and stability. Plant Cell 16, 3370–3385. doi: 10.1105/tpc.104.026278
Ye, J., Renault, V. M., Jamet, K., and Gilson, E. (2014). Transcriptional outcome of telomere signalling. Nat. Rev. Genet. 15, 491–503. doi: 10.1038/nrg3743
Yoo, H. H., Kwon, C., Lee, M. M., and Chung, I. K. (2007). Single-stranded DNA binding factor AtWHY1 modulates telomere length homeostasis in Arabidopsis. Plant J. 49, 442–451. doi: 10.1111/j.1365-313X.2006.02974.x
Yu, E. Y., Kim, S. E., Kim, J. H., Ko, J. H., Cho, M. H., and Chung, I. K. (2000). Sequence-specific DNA recognition by the Myb-like domain of plant telomeric protein RTBP1. J. Biol. Chem. 275, 24208–24214. doi: 10.1074/jbc.M003250200
Yun, J. H., Lee, W. K., Kim, H., Kim, E., Cheong, C., Cho, M. H., et al. (2014). Solution structure of telomere binding domain of AtTRB2 derived from Arabidopsis thaliana. Biochem. Biophys. Res. Commun. 452, 436–442. doi: 10.1016/j.bbrc.2014.08.095
Zachova, D., Fojtova, M., Dvorackova, M., Mozgova, I., Lermontova, I., Peska, V., et al. (2013). Structure-function relationships during transgenic telomerase expression in Arabidopsis. Physiol. Plant. 149, 114–126. doi: 10.1111/ppl.12021
Zellinger, B., Akimcheva, S., Puizina, J., Schirato, M., and Riha, K. (2007). Ku suppresses formation of telomeric circles and alternative telomere lengthening in Arabidopsis. Mol. Cell. 27, 163–169. doi: 10.1016/j.molcel.2007.05.025
Zentgraf, U. (1995). Telomere-binding proteins of Arabidopsis thaliana. Plant Mol. Biol. 27, 467–475. doi: 10.1007/BF00019314
Zhang, P., Pazin, M. J., Schwartz, C. M., Becker, K. G., Wersto, R. P., Dilley, C. M., et al. (2008). Nontelomeric TRF2-REST interaction modulates neuronal gene silencing and fate of tumor and stem cells. Curr. Biol. 18, 1489–1494. doi: 10.1016/j.cub.2008.08.048
Zhou, X., Graumann, K., Wirthmueller, L., Jones, J. D., and Meier, I. (2014). Identification of unique SUN-interacting nuclear envelope proteins with diverse functions in plants. J. Cell Biol. 205, 677–692. doi: 10.1083/jcb.201401138
Zhou, Y., Hartwig, B., James, G. V., Schneeberger, K., and Turck, F. (2016). Complementary activities of TELOMERE REPEAT BINDING proteins and polycomb group complexes in transcriptional regulation of target genes. Plant Cell 28, 87–101. doi: 10.1105/tpc.15.00787
Keywords: telomere, telomerase, telomeric proteins, shelterin, telomeric repeat binding (TRB), plant
Citation: Procházková Schrumpfová P, Schořová Š and Fajkus J (2016) Telomere- and Telomerase-Associated Proteins and Their Functions in the Plant Cell. Front. Plant Sci. 7:851. doi: 10.3389/fpls.2016.00851
Received: 12 November 2015; Accepted: 31 May 2016;
Published: 28 June 2016.
Edited by:
Anne-Catherine Schmit, Institut de Biologie Moléculaire des Plantes, CNRS UPR2357, FranceReviewed by:
Takashi Murata, National Institute for Basic Biology, JapanFranziska Katharina Turck, Max Planck Society, Germany
Copyright © 2016 Procházková Schrumpfová, Schořová and Fajkus. This is an open-access article distributed under the terms of the Creative Commons Attribution License (CC BY). The use, distribution or reproduction in other forums is permitted, provided the original author(s) or licensor are credited and that the original publication in this journal is cited, in accordance with accepted academic practice. No use, distribution or reproduction is permitted which does not comply with these terms.
*Correspondence: Petra Procházková Schrumpfová, schpetra@centrum.cz