- 1Unit of Functional Plant Biology, Institute of Botany, University of Innsbruck, Innsbruck, Austria
- 2Department of Botany, Faculty of Science, Charles University in Prague, Prague, Czech Republic
Charophyte green algae are a paraphyletic group of freshwater and terrestrial green algae, comprising the classes of Chlorokybophyceae, Coleochaetophyceae, Klebsormidiophyceae, Zygnematophyceae, Mesostigmatophyceae, and Charo- phyceae. Zygnematophyceae (Conjugating green algae) are considered to be closest algal relatives to land plants (Embryophyta). Therefore, they are ideal model organisms for studying stress tolerance mechanisms connected with transition to land, one of the most important events in plant evolution and the Earth’s history. In Zygnematophyceae, but also in Coleochaetophyceae, Chlorokybophyceae, and Klebsormidiophyceae terrestrial members are found which are frequently exposed to naturally occurring abiotic stress scenarios like desiccation, freezing and high photosynthetic active (PAR) as well as ultraviolet (UV) irradiation. Here, we summarize current knowledge about various stress tolerance mechanisms including insight provided by pioneer transcriptomic and proteomic studies. While formation of dormant spores is a typical strategy of freshwater classes, true terrestrial groups are stress tolerant in vegetative state. Aggregation of cells, flexible cell walls, mucilage production and accumulation of osmotically active compounds are the most common desiccation tolerance strategies. In addition, high photophysiological plasticity and accumulation of UV-screening compounds are important protective mechanisms in conditions with high irradiation. Now a shift from classical chemical analysis to next-generation genome sequencing, gene reconstruction and annotation, genome-scale molecular analysis using omics technologies followed by computer-assisted analysis will give new insights in a systems biology approach. For example, changes in transcriptome and role of phytohormone signaling in Klebsormidium during desiccation were recently described. Application of these modern approaches will deeply enhance our understanding of stress reactions in an unbiased non-targeted view in an evolutionary context.
Charophyte Algae in Terrestrial Environments
Charophyte green algae are a diverse paraphyletic assemblage of strictly freshwater algae (Leliaert et al., 2012) comprising about 100 genera. We can distinguish ‘advanced charophytes’ (Zygnematophyceae, Coleochaetophyceae, Charophyceae) recently designated as ZCC clade (de Vries et al., 2016) and ‘basal charophytes’ (Klebsormidiophyceae, Chlorokybophyceae, Mesostigmatophyceae), designated as KCM clade (de Vries et al., 2016). However, charophyte green algae are not only restricted to aquatic habitats. Terrestrial forms occur in the classes Chlorokybophyceae (Lewis and McCourt, 2004), the Klebsormidiophyceae (e.g., Karsten et al., 2010; Karsten and Holzinger, 2012), the Zygnematophyceae (e.g., Lewis and McCourt, 2004; Holzinger et al., 2009, 2010) and the Coleochaetophyceae (Graham et al., 2012, 2013) and viable airborne cells of various charophyte algae were also reported (Sharma et al., 2007).
Colonization of moderately moist habitats in the proximity of water by the charophyte algal ancestor of land plants and its gradual transition to drylands has been suggested by Becker and Marin (2009). Recently, several reports have demonstrated the close relationship of land plants and Zygnematophyceae that are currently viewed as sister lineages (Becker and Marin, 2009; Wodniok et al., 2011; Timme et al., 2012; Becker, 2013; Zhong et al., 2014, 2015; Delaux et al., 2015). At present, Zygnematophyceae dominate in various stressful habitats. For example, desmids are typically found in acidic bogs (Štástný, 2010), Zygogonium ericetorum is a common member of temperate biological soil crust (Hoppert et al., 2004) and Mesotaenium berggrenii and Ancylonema nordenskiöldii live on the surface of glaciers on bare ice (Remias et al., 2009, 2012a,b).
Transition to terrestrial habitats is connected with frequent exposure to naturally occurring abiotic stress scenarios like desiccation, freezing and high PAR and UV radiation. The effects of these stresses on ultrastructure, photosynthesis and ecology in green algae have recently been reviewed (Holzinger and Lütz, 2006; Holzinger and Karsten, 2013; Karsten and Holzinger, 2014). In the present review, we mainly focus on the current knowledge about abiotic stress tolerance mechanisms known in terrestrial members of the charophyte algae as model systems to study terrestrialization events. We include important classical studies based on traditional methods as well as new understanding derived from recent transcriptomic and genomic datasets. In general, stress tolerance strategies are summarized in a simplified schema (Figure 1).
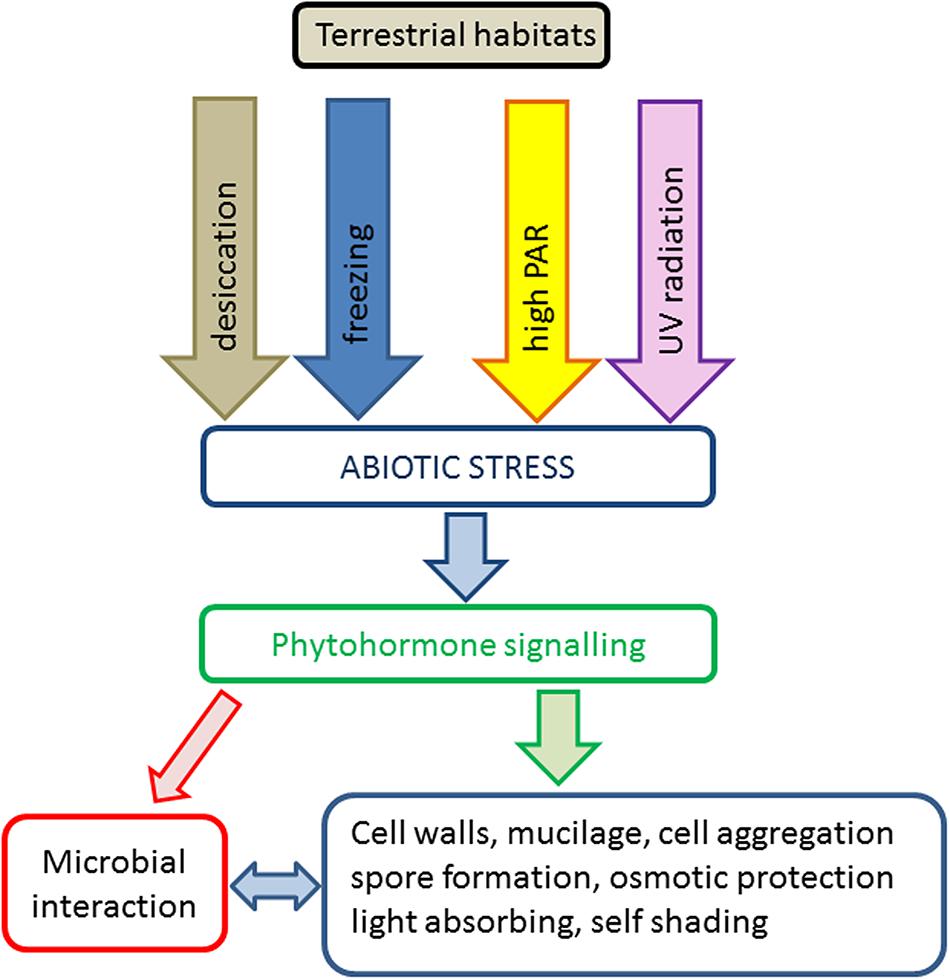
FIGURE 1. Schematic representation of abiotic stress factors and tolerance strategies in charophyte green algae in terrestrial habitats.
Self-Protection
The morphologically most complex group of charophyte algae, the class Charophyceae, is restricted solely to aquatic environment (Leliaert et al., 2012). On the other hand, terrestrial charophytes are characterized by a very simple thallus, unicellular or filamentous which, however, usually aggregate into colonies, multi-layered mats or biofilms. This growth pattern belongs to common stress avoidance strategies and provides protection from multiple stresses at the same time. While the outer layers are fully exposed to the environment and susceptible to damage, at the same time they efficiently protect the cells underneath by their water-holding and screening capacity. In addition, aeroterrestrial algae are also components of biological soil crusts, microecosystems containing also bacteria, Cyanobacteria, fungi, lichens, mosses, and anorganic particles where the whole community can profit from protection provided by an individual member (Belnap and Lange, 2001).
Chlorokybus atmophyticus, the only known member of the class Chlorokybophyceae, occurs in subaerial habitats and is characterized by sarcinoid colonies with groups of cells embedded in soft mucilage (Škaloud, 2009).
Freshwater Coleochaete usually forms flat epiphytic disk or cushion-like thalli, composed by densely branched filaments. However, when grown in aero-terrestrial conditions, namely on agar or sand, it markedly changes its morphology and growth habitus. It forms multistratose clusters of thick walled cells with acetolysis resistant autofluorescent cell wall components (Graham et al., 2012).
Aeroterrestrial members of the class Klebsormidiophyceae form multi-layered biofilms on soil or other aeroterrestrial substrata (Karsten and Rindi, 2010; Karsten et al., 2013). This provides above all self-shading and photoprotection of individual filaments inside the mat which can be even enhanced by soil particles interwoven within the mats (Karsten and Holzinger, 2012; Karsten et al., 2013). The importance of self-shading is reflected by generally low light requirements for photosynthesis in Klebsormidium that were repeatedly shown (Karsten and Rindi, 2010; Karsten et al., 2013).
Mat-forming growth is also typical for filamentous Zygnematophyceae. Filaments usually start to grow at the bottom of a pool and when enough biomass is produced, oxygen bubbles trapped within it carry the mat to the surface of the pool (Graham et al., 1995). The top layers are then fully exposed to solar radiation which leads to their bleaching. Berry and Lembi (2000) measured irradiance below the Spirogyra mat to be more than 30 times lower than at the mat surface. Moreover, the photosynthetic rate measured under experimental conditions was higher at lower irradiance showing that underlying filaments were exposed to more optimal irradiances (Berry and Lembi, 2000). Similarly, the low light adaptation in Zygnema is also usually explained by photoprotection provided by multi-layered mats (Holzinger et al., 2009; Herburger et al., 2015).
Spirogyra mats were even shown to be able of phototactic movement at low light conditions. The filaments align toward the light source and when they touch other filaments, they glide along each other, form bundles and move toward the light source by repeated rolling and stretching (Kim et al., 2005).
The differentiation of mat layers is best developed in terrestrial filamentous conjugating green alga Z. ericetorum. Zygogonium ericetorum produces two types of cells termed green and purple morphs (Figures 2A,B), (Aigner et al., 2013). The purple morph of the top layers is better protected from high irradiation and helps to shade the green morph underneath which is in turn less sensitive to desiccation (Holzinger et al., 2010; Aigner et al., 2013).
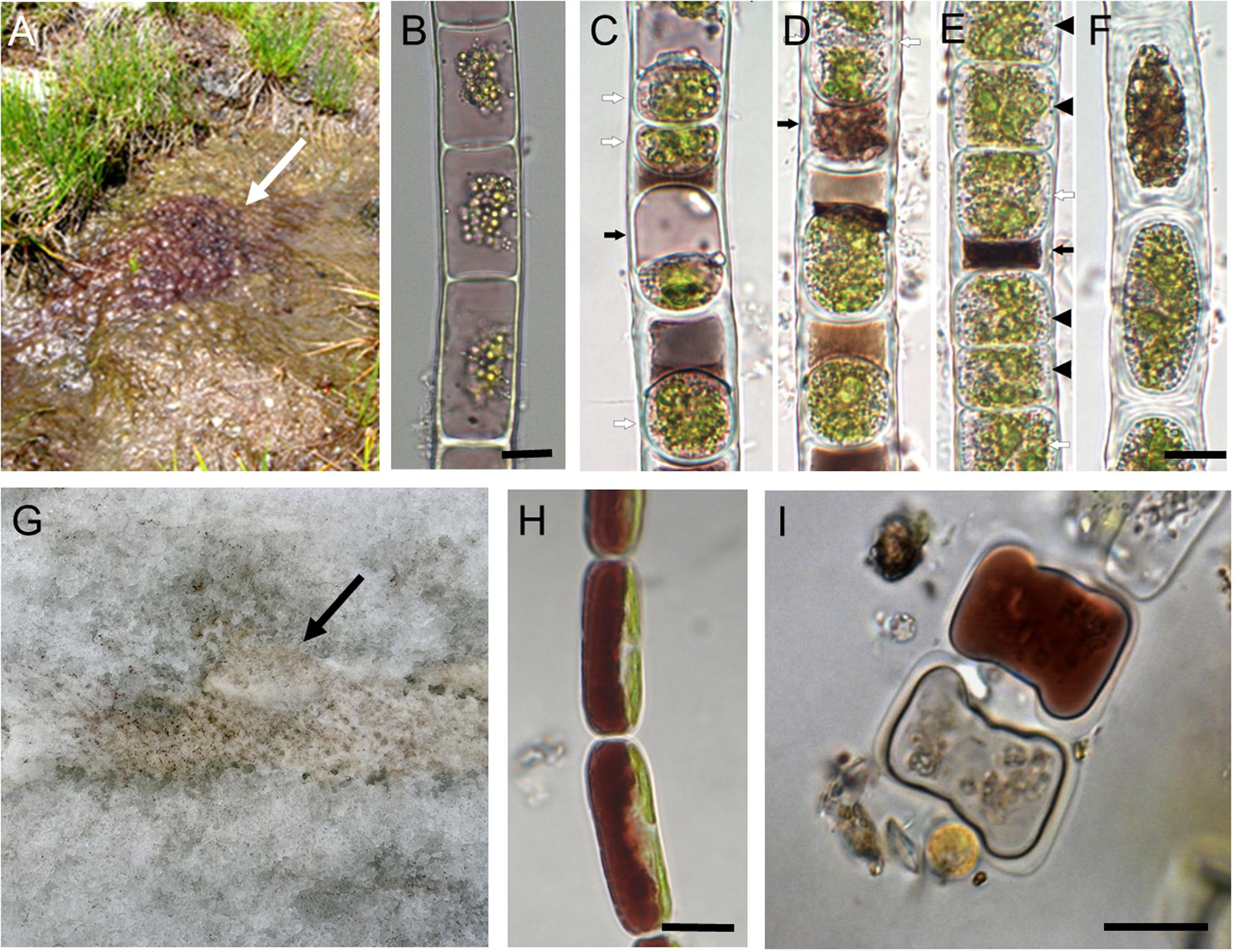
FIGURE 2. Zygnematophyceae with purple vacuolar pigmentation. (A–F) Zygogonium ericetorum; (G–I) Ancylonema nordenskiöldii. (A) Habitat showing purple coloration (arrow), (B) vegetative filament, (C–E) aplanospore formation, white arrows indicate enlarged aplanospores with cell wall cleavage, black arrows point to compacted brownish residues, black arrowheads illustrate germinated aplanospores, (F) thick walled akinetes, (G) habitat on the surface of a glacier, arrow indicates coloration by algae (H) vegetative filament with purple coloration of the vacuole, (I) conjugation stage. Bars (B–F,H,I) 10 μm. (A,B) Reprinted from Aigner et al. (2013), (C–F) reprinted from Stancheva et al. (2014), (G–I) reprinted from Remias et al. (2012a) with permission of Springer Business Media.
Formation of Specialized Cells
Formation of specialized stress-tolerant cells as a part of the life cycle is a widespread strategy for survival of unfavorable conditions and is widely known from many different groups of algae and other protists.
The first type of stress tolerant cells are dormant zygotes, i.e., resting cells that are developed as a result of sexual reproduction. Within Charophyta, such cells have been described in Coleochaetophyceae, Charophyceae, and Zygnematophyceae. Dormant zygotes usually require a period of dormancy before germination. Dormancy can be broken by a change in environmental conditions (temperature, light) or by addition of gibberellic acid (Sederias and Colman, 2007; Agrawal, 2009). Possible roles in phytohormone stress sensing and signal transduction are discussed below.
In Charophyceae, the dormant zygotes are termed oospores. Oospores are the overwintering stages that can survive anoxic conditions on lake bottoms. They are the only desiccation tolerant stages in the life cycle of Charophyceae and thus guarantee their survival in habitats that dry out for several years (Proctor, 1967; Leliaert et al., 2012). The young zygote secretes a thick cell wall and the inner walls of cortical cells thicken and become encrusted with lime. Calcified envelopes of the zygotes are known as “gyrogonites” (Leliaert et al., 2012).
Dormant zygotes, termed zygospores, that contain acetolysis resistant material are typical feature of the life cycle of Coleochaetophyceae (Delwiche et al., 1989) and Zygnematophyceae (e.g., Zygnema sp.: Stancheva et al., 2012, Spirogyra sp.: Stancheva et al., 2013 and Z. ericetorum: Stancheva et al., 2016). Cell walls of Zygnematophyceae zygospores consist of three major layers. Endospore is the colorless inner layer. The middle layer, termed mesospore, is crucial for stress tolerance as it contains enzyme- and acetolysis-resistant sporopollenin-like material. Exospore is at least partially enzyme-soluble, colorless and sometimes also sculptured (Ashraf and Godward, 1980). Zygospores can be spherical, ellipsoid, rectangular, or lenticular and may be colored yellow, brown, purple, blue, or black (Kadlubowska, 1984). Most of the morphological characters important for traditional species determination are on the mesospore, where a blue vs. brown mesospores were suggested to separate different phylogenetic clades (Stancheva et al., 2012).
The other type of specialized cells is not formed during the sexual process, even though morphologically they can be very similar to the sexual spores. In Zygnematophyceae, mostly in filamentous Zygnematales, several such cell types were described. Parthenospores result from incomplete conjugation; they form directly from gametes that failed to find a compatible sexual partner (Kadlubowska, 1984). They are smaller and more rounded than zygospores (Poulíčková et al., 2007). Aplanospores are formed inside vegetative cells when protoplasts shrink and are enclosed by a complete new cell wall, independent of the original wall (Kadlubowska, 1984). Their formation was recently described in Z. ericetorum (Figures 2C–F), (Stancheva et al., 2014). Finally, akinetes develop directly from vegetative cells by thickening of the cell wall by deposition of wall material at the inner surface of the vegetative cell wall (Kadlubowska, 1984). In some species of Zygnema, the cell walls of mature akinetes can have a similar structure, ornamentation, and coloration to that of the zygospores (Kadlubowska, 1984). For morphology and light microscopy images of zygospores and akinetes, see Stancheva et al. (2012).
In contrast, many algae from stressful environments do not form any specialized stages and survive environmental stresses in vegetative state (Agrawal, 2009). Such cells are not dormant, so they can resume their physiological activity and growth immediately under restoring of favorable conditions (Agrawal, 2009). Nevertheless, vegetative cells in natural or experimentally induced stressful conditions differ from growing, non-stressed vegetative cells in many ways. They are usually characterized by storage product accumulation, thick cell wall, reduced physiological activity and ceased cellular division. Traditionally, such cells were sometimes termed ‘akinetes’ or even ‘winter forms’ in Micrasterias (Meindl et al., 1989). To avoid the confusion with ‘akinetes’ in Zygnema which are true specialized cells with zygospore-like cell walls, the term ‘pre-akinete’ or ‘mature cell’ has also been introduced by some authors for these hardened, stress tolerant vegetative cells (Fuller, 2013; Pichrtová et al., 2014a,b, 2016a; Herburger et al., 2015). The term ‘mature cell’ refers to the typical occurrence of such cells in old, starved cultures.
Mature cells (pre-akinetes) are key elements for survival of Zygnema in stressful environment where sexual reproduction and zygospore formation is very rare. Pre-akinetes of Arctic and Antarctic Zygnema survived when exposed to osmotic stress (Pichrtová et al., 2014a), desiccation (Pichrtová et al., 2014b), UV radiation (Holzinger et al., 2009), freezing (Hawes, 1990), and they were even observed to be the overwintering stages (Pichrtová et al., 2016b). Formation of mature cells (pre-akinetes) during aging of the cultures was tracked in cultures of Alpine strains of Zygnema (Figure 3), (Herburger et al., 2015); old cultures survived desiccation at 84% RH and had in general lower rETR max (Herburger et al., 2015). However, only after previous acclimation by slow desiccation (induced either by controlled desiccation at high relative humidity or by pre-cultivation on agar), the pre-akinetes were able to survive rapid desiccation in air at a relative humidity of 10% (Pichrtová et al., 2014b).
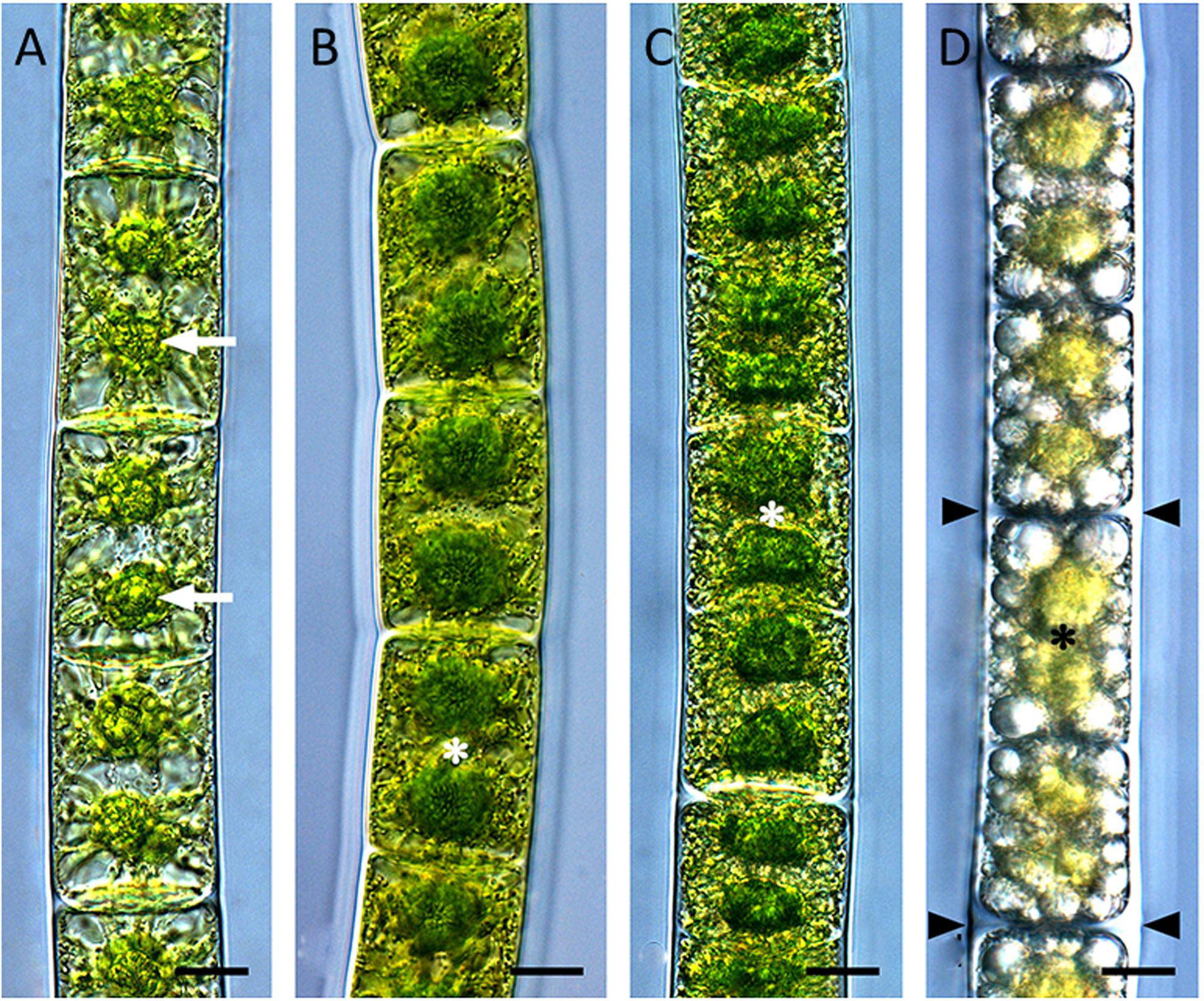
FIGURE 3. Pre-akinete formation in Zygnema sp. Saalach. (A) 1 month, (B) 6 months, (C) 9 months, (D) 15 months old cultures. Arrows: pyrenoids, asterisks: nuclei, arrowheads: thickened cell walls. Bars 10 μm. Reprinted from Herburger et al. (2015).
Glacier surface belongs to the most extreme habitats on Earth and it is interesting that the only eukaryotic algae that live there belong to the class Zygnematophyceae. Field populations of these algae, namely species A. nordenskioeldii (Figures 2G–I), (Remias et al., 2012a) and M. berggrenii, are also composed of vegetative cells; in the field no spores or cysts are produced even for overwintering (Remias et al., 2009, 2012a), however, conjugation has been observed in A. nordenskioeldii in field collected samples that were transferred to the laboratory (Figure 2I).
Members of the class Klebsormidiophyceae are also stress tolerant in their vegetative cells. Morison and Sheath (1985) showed a change in cellular morphology in desiccation tolerant K. rivulare resembling pre-akinete formation in Zygnema. Upon desiccation, carbohydrate and lipid contents increased and protein content decreased (Morison and Sheath, 1985). Many papers have recently been published that investigated various strains of Klebsormidium, Interfilum, Hormidiella, and Entransia in relation to desiccation stress (Karsten et al., 2010, 2013, 2014; Karsten and Holzinger, 2012; Herburger et al., 2016a). All species investigated were able to survive experimental desiccation and even the aquatic Entransia coped with low water availability better than other freshwater algae (Herburger et al., 2016a). Alpine strains of Klebsormidium were also markedly resistant to osmotic stress (Kaplan et al., 2012). Moreover, most of the investigated Klebsormidium sp. strains also survived freezing at -40°C (Elster et al., 2008).
Cell Wall Structure and Composition in Relation to Stress Tolerance
Cell wall composition of charophyte green algae has recently been investigated extensively, particularly before the background that they are thought to be closest living relatives to land plants (Mikkelsen et al., 2014). Charophyte green algae have cell walls containing polymers with remarkable similarity to cellulose, pectins, hemicelluloses, arabinogalactan proteins (AGPs), extensin, and lignin present in embryophyte walls (Sørensen et al., 2011; Domozych et al., 2012). Charophyte green algae contain orthologous genes in the cellulose synthetase (cesA) family, as well as in the cesA-like families cslC and cslD as analyzed by a survey of genomes and transcriptomes (Yin et al., 2014). Recent review articles summarize cell wall composition of charophytes (Popper and Tuohy, 2010; Popper et al., 2011, 2014; Domozych et al., 2012; Sørensen et al., 2012; Domozych and Domozych, 2014). We will focus on the cell wall components that are involved in desiccation and irradiation stress tolerance. The cell walls of streptophytic green algae have been suggested to be a defining structure that enabled green algal ancestors to colonize land (Mikkelsen et al., 2014). However, what are the traits that enabled this colonization? All charophyte green algae are poikilohydric organisms, they equilibrate with the surrounding relative air humidity, and do not have mechanisms that protect from desiccation. However, some cell wall components might have the sole function of prolonging the water holding capacities for algae that were exposed to desiccating conditions.
One of the major differences in early-divergent chlorophyte and prasinophyte algae genomes is the occurrence of a low number of glycosyl transferases (GTs), whereas land plants contain hundreds of GTs. Now there is genetic evidence that many of the core cell wall polysaccharides have their origin in charophyte green algae (Domozych et al., 2012). These include mannan, xyloglucan, xylan, pectin, and arabino-galactan proteins (Mikkelsen et al., 2014). Most important for stress tolerance are pectic proteins, which have been extensively studied in the mesotaeniaceae Netrium and the desmidiacean green algae Closterium, Penium, and Micrasterias (e.g., Domozych and Rogers-Domozych, 1993; Brosch-Salomon et al., 1998; Domozych et al., 2007; Eder et al., 2008; Eder and Lütz-Meindl, 2010). While the chemical structure of the detected homogalacturonans (α-D-1,4-galacturonic acid) might vary in the degree of methyl esterification, relevant for the function of these compounds during developmental processes. When incorporated into mucilage, particularly the water holding capacities of these layers are ecologically relevant in habitats with fluctuating water regimes. Formation of mucilage layers is widespread in Zygnematophyceae. For example, in Zygnema irregulare formation of secondary pectic layers was observed during prolonged cultivation on solid medium (Fuller, 2013). In the desmid Micrasterias denticulata mucilage layers have been described and characterized (e.g., Oertel et al., 2004; Wanner et al., 2013). Additionally, pectic layers have another great benefit in charophyte green algae – allowing to form multicellular organisms, a clear prerequisite of success for plants on land (Domozych and Domozych, 2014). Moreover, a mucilage layer has also been suggested to play a significant role in UV protection (Lütz et al., 1997), however, UV screening compounds have not directly been identified in the mucilage. Recently, homogalacturonans have been described as ancient streptophyte feature, albeit secondarily lost in Klebsormidium (O’Rourke et al., 2015). Members of the Klebsormidiales have indeed many differences concerning their cell walls (Mikhailyuk et al., 2014), when compared to later branching charophytes.
The localization and function of callose, a β-D-1,3-glucan has been investigated in Klebsormidium and Zygnema (Herburger and Holzinger, 2015). Callose as a cell wall flexibilizing compound can protect cell walls from desiccation induced damage, when they follow the shrinkage of the protoplasts. It was previously shown by biochemical methods, that a high abundance of this compound was found in the early branching charophyte Klebsormidium (Sørensen et al., 2011). Upon localization this flexibilizing compound is predominately found in mechanically strained areas of the cell wall (Herburger and Holzinger, 2015). In Klebsormidium the absolute content of callose is significantly increased upon desiccation stress applied for up to 3 h. Localization of callose in Klebsormidium demonstrated that particularly the cross cell walls and the cell walls of the terminal cells contain high amounts of this compound, and thus can fold and follow the desiccation induced volume reduction (Figures 4A–F), (Herburger and Holzinger, 2015). In contrast, the later branching Zygnema, did not change the callose content upon desiccation stress. However, callose can still be localized in the cell walls of Zygnema, particularly in the cell corners, that are exposed to the most severe strain upon desiccation stress (Figures 4G–L). Desiccation induced volume changes have recently been investigated in Klebsormidium and Zygnema at different relative humidities, where Zygnema showed a more drastic volume reduction at the lowest RH tested (Lajos et al., 2016). Interestingly the more rigid, cellulose-rich secondary walls of Zygnema tolerate this stress without being damaged.
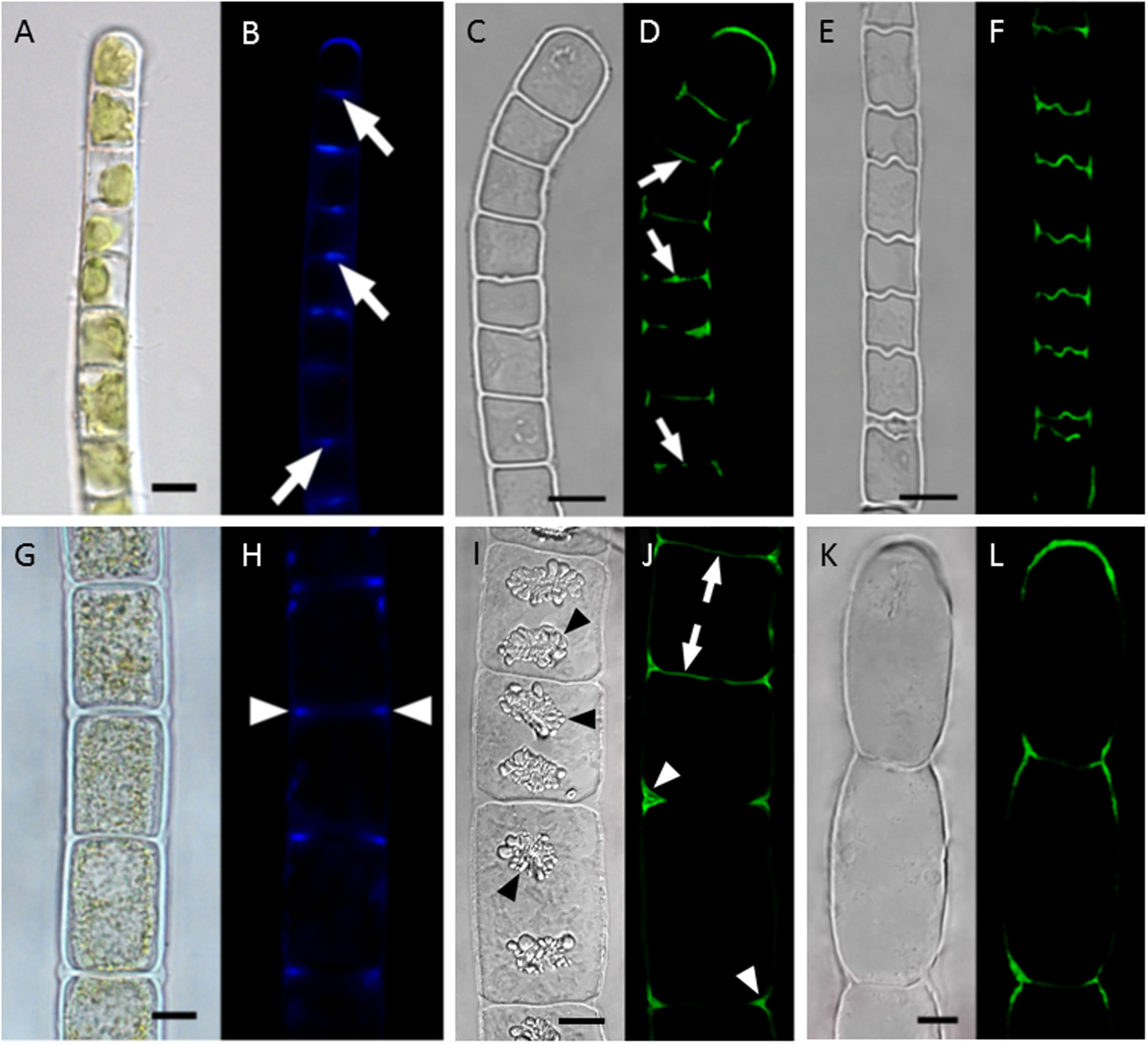
FIGURE 4. Localization of callose in cell walls of Klebsormidium crenulatum (A–F) and Zygnema sp. Saalach (G,H). (A,B) Aniline blue staining, (C,D) staining of turgescent cell with antibody 400-2, (E,F) staining of desiccated cells with antibody 400-2, (G,H) aniline blue staining, (I,J) staining of turgescent cells with antibody 400-2, (K,L) staining of desiccated cell with antibody 400-2. Arrows: cross walls, arrowheads: cell corners. Bars 10 μm. reprinted from Herburger and Holzinger (2015).
The occurrence of fossilizable biomacromolecules resistant to chemical processes in spores of charophyte green algae has been reported repeatedly (Versteegh and Blokker, 2004). The green autofluorescence of Zygnematophyceae zygospores is characteristic for sporopollenin-like material (algaenan) that differs from true sporopollenin of pollen grains in chemical composition and in biochemical pathway leading to its production (Versteegh and Blokker, 2004; Poulíčková et al., 2007). While sporopollenin is synthesized in phenylpropanoid pathway, the acetate–malate pathway leads to algaenans (Versteegh and Blokker, 2004). Similarly, in Coleochaete the inner zygote cell wall layers have been described to contain material similar in ultrastructural appearance and chemistry to sporopollenin (Delwiche et al., 1989; Kroken et al., 1996). Sporopollenin is polymerized from hydroxylated fatty acids and phenolics, its occurrence predated lignin (summarized in Weng and Chapple, 2010). Sporopollenin and algaenans in the cell walls provides protection from both desiccation and UV radiation. The occurrence of ‘lignin-like’ phenolic compounds were described in walls of vegetative cells of Coleochaete (Delwiche et al., 1989). However, many of these early findings were based on crude detection methods and have to be discussed critically (Weng and Chapple, 2010).
Physiological Protection of the Photosynthetic Apparatus
One of the key targets of radiation (high PAR and UV) as well as desiccation and temperature stress is photosynthesis. The effects of desiccation on photosynthesis of green algae were recently summarized (Holzinger and Karsten, 2013; Karsten and Holzinger, 2014). Photosynthetic strategies of desiccation tolerant organisms were recently summarized by Fernandez-Marin et al. (2016). It has been shown, that dehydration directly affects the electron transport chains in the thylakoid membranes. The loss of water in the presence of light increases the risk of chlorophyll overexcitation, which can in turn generate oxygen radicals and reactive oxygen species (ROS). These compounds have various negative effects that will not be further discussed in this review. To compensate these effects, tolerant organisms up-regulate photoprotection mechanisms (Fernandez-Marin et al., 2016). The regulation of the photosynthetic electron transport rates and protection through photoinhibition is crucial for survival (e.g., Roach and Krieger-Liszkay, 2014). UV-B radiation has been shown to induce similar photo-oxidative stress in charophyte green algae (Germ et al., 2009; Karsten and Holzinger, 2014). The shift form LHCSR (light-harvesting complex stress-related) in chlorophytes to PSBS (photosystem II subunit S)-dependent non-photochemical quenching (NPQ) in charophytes has been demonstrated in ZCC-clade genera (Chara, Zygenma, and Cosmarium; Gerotto and Morosinotto, 2013). While Zygnema and Cosmarium showed a fast NPQ induction upon light exposure, Klebsormidium and Interfilum as representatives of the KCM-clade had a much slower induction. The only exception was Mesotaenium, where little NPQ induction was found (Gerotto and Morosinotto, 2013), however, in this genus different strategies like abundant protection by phenolic compounds are established (see below).
Numerous publications have investigated photosynthetic performance under laboratory controlled conditions in Klebsormidiophyceae (Karsten et al., 2010, 2013, 2014, 2015; Karsten and Holzinger, 2012) and in Zygnematophyceae (Pichrtová et al., 2013, 2014b; Herburger et al., 2015). Some information on photosynthesis parameters and light acclimation are available in Characeae (Küster et al., 2000; Vieira and Necchi, 2003). In contrast, little is known on other charophyte green algae according their photosynthetic performance.
Many physiological data are available for the genus Klebsormidium (Karsten et al., 2010, 2013, 2015; Karsten and Holzinger, 2012). Regardless of strain and clade, Klebsormidium shows a broad light amplitude, the relative electron transport rate did not show photoinhibition up to 500 μmol photons m2 s-1 in Klebsormidium crenulatum (Figure 5A), (Karsten et al., 2010). Very low light compensation points in the range 1.8–5.7 μmol photons m2 s-1 were found for different Klebsormidium species (Karsten et al., 2010; Karsten and Holzinger, 2012). The maximum photosynthetic oxygen production ranged from 37.9 μmol O2 h-1 mg-1 chl a in K. crenulatum (Karsten et al., 2010), 75.9 μmol O2 h-1 mg-1 chl a in K. flaccidum (Karsten et al., 2013) to 87.0 μmol O2 h-1 mg-1 chl a in K. dissectum.
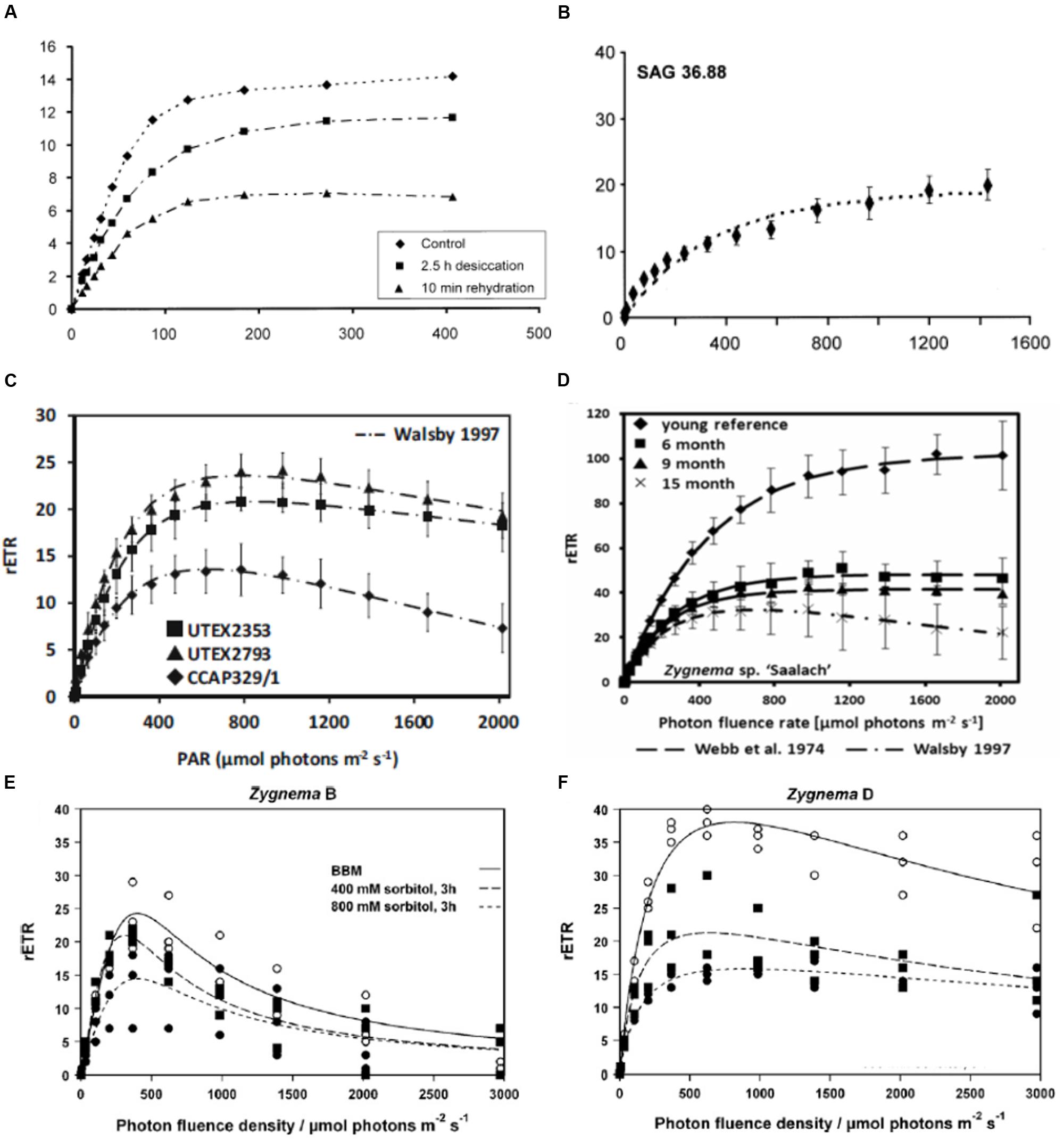
FIGURE 5. Relative electron transport rates in different charophyte green algae. (A) Klebsormidium crenulatum, (SAG 2415, desiccation and rehydration, reprinted from Karsten et al., 2010 with permission of Wiley and Sons.), (B) Interfilum (SAG 36.88), reprinted from Karsten et al. (2014), (C) Entransia fimbriata (UTEX2353, UTEX2793) and Hormidiella attenuata (CCAP329/1) reprinted from Herburger et al. (2016a), (D) Zygnema sp. Saalach (SAG 2419, various culture ages, reprinted from Herburger et al., 2015), (E) arctic Zygnema sp. ‘B’ (CCALA976, (F) Antarctic Zygnema sp. ‘D’ (E,F) reprinted from Kaplan et al. (2013).
The Klebsormidiophyceae genus Interfilum showed different kinetics of decrease in effective quantum yield under desiccation conditions in relation to their morphological characteristics (Karsten et al., 2014). While single celled strains exhibited a faster desiccation rate, cell packet forming strains showed slower desiccation rates (Karsten et al., 2014). In general, all investigated Interfilum strains exhibited optimum photosynthesis under low photon fluence rates, with no signs of photoinhibition under high light conditions up to 1500 μmol photons m2 s-1 (Figure 5B), (Karsten et al., 2014). In the additionally investigated Klebsormidiophyceaen genera Entransia and Hormidiella similar reactions to desiccation were observed (Figure 5C), (Herburger et al., 2016a). All these data suggest that Klebsormidiophyceae have low light requirements, coupled with no photoinhibition under higher irradiation, corroborating a high photophysiological plasticity.
In contrast, in Zygnematophyceae rETR curves show much higher initial saturation irradiance (Ik values). In the desmidiaceae Cosmarium (Stamenković and Hanelt, 2011), in the range of 1200 μmol photons m2 s-1 for tropical and cosmopolitan strains, somewhat lower in polar taxons (around 700 μmol photons m2 s-1). In a polar strain of Zygnema Ik values around 450 μmol photons m2 s-1 were observed (Holzinger et al., 2009). Ik values of Zygnema sp. collected in the Austrian Alps (~150 μmol photons m2 s-1) and in a lowland strain of Zygnema at ~450 μmol photons m2 s-1 (Herburger et al., 2015). The rETR curves showed significant difference in relation to the culture age, where the oldest cultures had a significant drop in the maximum electron transport rate (rETRmax; Figure 5D), (Herburger et al., 2015). Signs of photoinhibition were observed in arctic and Antarctic Zygnema sp. strains, and the rETRmax values were drastically reduced upon osmotic stress (Figures 5E,F), (Kaplan et al., 2013). However, in general, the values determined for Zygnematophyceae point toward a higher light adaptation, and moreover remarkable resistance against UV irradiation has been observed (summarized in Holzinger and Lütz, 2006). How can this adaptation be realized?
The xanthophyll cycle pool size may contribute substantially to a well-developed photo protection process, in this way dissipating excessive irradiation (Stamenković et al., 2014). There is evidence for the increase in zeaxanthin content of the PS II antenna in high light tolerating Cosmarium strains (Stamenković et al., 2014). In Zygnema strains from arctic and Antarctic habitatas, a considerable amount of antheraxanthin and zeaxanthin was found (Pichrtová et al., 2013). The highest de-epoxidation state was found in an arctic strain, which went along with the highest concentration of phenolic compounds (Pichrtová et al., 2013).
These studies match well with the findings of high resistance to photoinhibition in an Antarctic strain of Zygnema sp. (Thangaraj, 2016). In this study the cells have been exposed to 400, 1400, 2100, and 3500 μmol photons m-2 s-1. After an initial drop of the Fv/Fm value, the cells showed a rapid tendency to recover, even after unrealistically high irradiation. The authors claim some constitutive and genetically fixed preservation mechanisms responsible for the high tolerance in the Antarctic Zygnema sp. strain. Interestingly, dFo did not recover to pre-treatment values, which indicated rearrangements of the LHC II complex.
Zygnematophyceae have shown remarkable stress tolerance to experimental UV radiation (Meindl and Lütz, 1996; Lütz et al., 1997). UV-effects in algae were summarized in Holzinger and Lütz (2006). In a study with field collected samples of Zygnema sp. from Svalbard, virtually no effect on the ultrastructure but a significant decrease of the Fv/Fm value after 24 h UV A, but not after UV AB exposures was found (Holzinger et al., 2009). Similar results were gained by Pichrtová et al. (2013), albeit significant reduction in Fv/Fm were observed in an arctic and an Antarctic strain of Zygnema sp. after a predominant UV A treatment for 8 days. Germ et al. (2009) did not find a decrease in Fv/Fm after UV B exposure in an arctic Zygnema strain. In Cosmarium UV A radiation caused a twofold larger depression of Fv/Fm when compared to that under PAR irradiation (Stamenković and Hanelt, 2014). The observation that in several of the investigated Zygnematophyceae UV A lead to more pronounced photoinhibition, might be ecologically relevant, as in natural sunlight the proportion of UVA is at least ten times higher than UV B, and moreover this waveband is not blocked by the ozone layer.
Production of Protective Substances
Algae produce a vast spectrum of metabolites that help them to protect the cells against environmental stress. Some are produced as a result of stress but some are also produced constitutively. Precise classification according to their function is difficult, because very often one substance is involved in various processes. Here, we focus on two major protective mechanisms provided by accumulated compounds – osmotic acclimation, which is important for tolerating limiting water availability and UV (PAR) screening that protects exposed cells.
Accumulation of Organic Osmolytes
Preventing water loss and maintaining homeostasis under stress conditions is essential for normal cellular function. Increasing salinity, desiccation, and freezing lead to osmotic dehydration and a reduced cellular water potential (Bisson and Kirst, 1995). Therefore, the physiological effects of these stresses are similar and the acclimation during exposure to an individual stress can result in resistance to other stresses (Morison and Sheath, 1985; Welsh, 2000; Vilumbrales et al., 2013). Nevertheless, these stresses are complex and components other than osmotic dehydration play a role in their effects (e.g., ionic component of the salt stress, Darehshouri and Lütz-Meindl, 2010). Recently osmotic stress induced ultrastructural changes leading to degradation of dictyosomes were investigated by TEM and FIB-SEM tomography in the charophyte green algae Micrasterias and Nitella (Lütz-Meindl et al., 2015). During the osmotic acclimation in response to long term hyperosmotic conditions, algae synthesize and accumulate various substances that increase the cellular osmotic value, leading to a negative osmotic potential (Bisson and Kirst, 1995). Organic osmolytes also play other roles in cellular protection. They can act as compatible solutes that are highly soluble, accumulate to high concentrations without interacting with cellular functions, protect proteins and stabilize membranes (Bisson and Kirst, 1995; Yancey, 2005). They can also act as antioxidants, cryoprotectants and heat protectants and can be rapidly degraded and used as respiratory substrates (Welsh, 2000; Yancey, 2005). Organic osmolytes are chemically diverse, comprising sugars, polyols, amino acids, and their derivatives (Welsh, 2000; Yancey, 2005).
Several studies reported osmolyte accumulation in various species of Klebsormidium. Sucrose and glutamic acid were detected as major osmoregulatory compounds of K. flaccidum and K. sterile subjected to osmotic stress in artificial seawater (Brown and Hellebust, 1980). K. flaccidum was capable to increase freezing tolerance during cold acclimation in a similar way to higher land plants (Nagao et al., 2008). The authors showed accumulation of sugars (mainly sucrose and glucose), but also of other compounds, such as amino acids and an unknown glycoside (Nagao et al., 2008). Kaplan et al. (2012) detected various soluble carbohydrates in two species of Klebsormidium (Table 1). The major proportion of oligosaccharides remained unidentified, the rest was dominated by raffinose, sucrose, glucose, xylose, galactose, mannose, inositol, fructose, glycerol, mannitol, and sorbitol (Kaplan et al., 2012). However, the total content of soluble carbohydrates was only approximately 1.2% of the dry weight (Kaplan et al., 2012). Karsten and Rindi (2010) reported sucrose as the major organic osmolyte of Klebsormidium sp. isolated from an urban wall in Germany. Sucrose accumulated in the cells with increasing salinity, in the highest salinity levels tested, however, the sucrose content and maximum quantum yield of PSII rapidly declined. This rather stenohaline response can be explained by the inability of Streptophyta to accumulate polyols as organic osmolytes (in contrast to most aeroterrestrial Chlorophyta, e.g., Gustavs et al., 2010), because sucrose cannot be accumulated to such a high content (Karsten and Rindi, 2010; Karsten et al., 2010). The only known report of sorbitol accumulation to high cellular concentrations by K. marinum (Brown and Hellebust, 1980) has to be viewed critically as molecular phylogenetic analyses revealed its position under Chlorophyta which resulted in its reclassification as Stichococcus deasonii (Neustupa et al., 2007).
Recent transcriptomic study of K. crenulatum showed up-regulation of sucrose synthase and sucrose phosphate synthase after desiccation stress (Holzinger et al., 2014) even though this enzyme has a different structure than in plants (Nagao and Uemura, 2012). In addition, several enzymes involved in the biosynthesis of the raffinose family of oligosaccharides were also up-regulated (Holzinger et al., 2014).
Low cellular osmotic potentials (-0.8 to -1.67 MPa) and tolerance to osmotic stress was also reported in arctic and Antarctic Zygnema spp. (Kaplan et al., 2013; Pichrtová et al., 2014a). Soluble sugars accounted for 9.9 ± 0.8% ash-free dry weight of field collected Antarctic Zygnema and 95% of the extracted sugars were identified as sucrose, with traces of glucose, fructose, and mannitol (Hawes, 1990). However, the author argued that this concentration is still too low to significantly depress the freezing point of the cell contents (Hawes, 1990).
Even though many streptophytes were shown to tolerate osmotic, desiccation and freezing stress, production of organic osmolytes within this group remains largely unknown or it seems that the detected organic osmolytes do not accumulate to high concentrations enough to fully explain the alga’s stress tolerance (Hawes, 1990; Kaplan et al., 2012). Therefore, it seems that accumulation of soluble carbohydrates alone cannot explain the high osmotic values and water stress tolerance of aeroterrestrial streptophytic algae.
UV (and PAR) Screening Compounds
Strictly freshwater Charophyceae were found to be sensitive to UV B radiation, but at the same time they do not produce any UV absorbing compounds. They have to rely on natural UV attenuation provided by water and substances dissolved within (de Bakker et al., 2005).
Mycosporine-like amino acids (MAAs) are the most widespread compounds with UV screening function, commonly accumulating in many groups of algae and other organisms. They are colorless, water-soluble substances with peak absorbances between 310 and 360 nm (Cockell and Knowland, 1999; Shick and Dunlap, 2002). They can function also as antioxidants (Coba et al., 2009; Carreto and Carignan, 2011) and are involved in osmotic regulation (Shick and Dunlap, 2002; Oren, 2007). Within Charophyta, their production has been so far proven only in Klebsormidiophyceae. K. fluitans was shown to synthesize a so far unidentified MAA with absorption maximum at 324 nm (Table 1). After UV exposure, this compound was accumulated up to more than 1% DW (Kitzing et al., 2014). A closely related genus Hormidiella that occurs in similar habitats as Klebsormidium contains the same compound, while this compound was not present in Entransia, an aquatic genus from the same class. Entransia occurs in peat bogs and humic lakes where UV screening is provided by dissolved organic carbon of the water (Kitzing and Karsten, 2015).
Another group of substances with UV screening function are phenolic substances. They are produced by only few algal classes and within charophytes their production has been so far proven only in Zygnematophyceae (Table 1), which chemotaxonomically support their close relationship to land plants. Like MAAs, phenolics are water soluble and natural UV screens because of their aromatic groups. In addition, some phenolic substances cause strong pigmentation of the vacuoles and so they protect photosynthetic apparatus also from excessive PAR irradiation in a similar way as secondary carotenoids of some chlorophyte algae. In contrast to MAAs they do not contain nitrogen which makes their synthesis “cheaper” and therefore advantageous in extreme habitats with nutrient deficiency (Carreto and Carignan, 2011). This is probably the main reason why Zygnematophyceae are able to thrive in extreme environments like glacier surface where strong irradiation is coupled with very oligotrophic conditions. Phenolics also act as antioxidants and may play a role also against herbivores or parasites. Antibacterial activity of methanol extract of Spirogyra varians is also attributed to phenolics (Cannell et al., 1988).
Ancylonema nordenskioeldii and M. berggrenii, common zygnematophytes of both Alpine and Arctic glaciers, have dark purple brownish peripheral vacuoles containing phenolic pigments (Figures 2H,I). Phenolic compounds of M. berggrenii were determined as purpurogallin derivates (Remias et al., 2009, 2012b). The pigments cause even macroscopically visible coloration that significantly decreases the albedo of the ice and thus potentially promotes the melting of the glacier, with consequences for the whole ecosystem and global climate (Stibal et al., 2012).
Purple morph of Z. ericetorum similarly accumulates several unusual phenolic substances in vacuoles (Figures 2B–F), (Aigner et al., 2013). These components are mainly gallic acid derivatives, and their structure has recently been investigated (Newsome et al., 2013). In this way, Zygogonium has gained a high tolerance to iron, as well as aluminum (Herburger et al., 2016b). Various gallotannins were also detected in a Spirogyra sp. (Nishizawa et al., 1985). Spirogyra pratensis was able to produce purple pigmentation in the presence of iron (Allen and Alston, 1959). A whole range of unspecified, phenolics with UV screening ability but lacking typical dark color were detected also in other zygnematophytes, such as Zygnema (Pichrtová et al., 2013) and Zygnemopsis (Figueroa et al., 2009).
Molecular Investigations of Stress Related Processes
At the moment, only a few publications are available using ‘omics’ techniques to address the molecular pathways behind stress tolerance and adaptation in charophytes. Comparisons of plant molecular processes between the charophyte green algae Coleochaete orbicularis and Spirogyra pratensis were performed and revealed a closer similarity to Arabidopsis thaliana than to the chlorophyte Chlamydomonas reinhardtii (Timme and Delwiche, 2010). The authors used a list of genes identified by Graham et al. (2000) to be important for colonization of land and found solid hits and even true orthologs in Coleochaete and Spirogyra including, e.g., cellulosic cell walls and cytokinetic phragmoplasts. According to the body plan no hit for asymmetric cell division was found in Spirogyra, but two hits were obtained in Coleochaete (Timme and Delwiche, 2010). A transcriptomic study of severe desiccation stress in K. crenulatum demonstrated that most of the highest upregulated transcripts do not show any similarity to known proteins (Holzinger et al., 2014). Major physiological shifts upon desiccation were the up-regulation of transcripts for photosynthesis, energy production and ROS metabolism (Holzinger et al., 2014). Classical desiccation responses, like late embryogenesis abundant proteins (LEA) or proteins involved in early response to desiccation (ERD) as well as osmolyte production (raffinose family oligosaccharides RFO) were upregulated. In contrast, transcripts of cell division, DNA replication, cofactor biosynthesis, and amino acid biosynthesis were strictly down-regulated as a consequence of desiccation stress (Holzinger et al., 2014).
Recently the effect of ionizing radiation was investigated in an arctic strain of Zygnema sp. (Choi et al., 2015). The photosynthetic efficiency markedly decreased with a 5 kGy dose, and the cells showed serious damage. However, an increase in DPPH radical scavenging activity was found in gamma-irradiated cells. The study is insofar interesting, as changes in the protein expression levels were investigated by a proteomics approach (Choi et al., 2015). Photosynthesis related proteins were up- or down-regulated, confirming the impact of gamma irradiation on the photosynthetic process. Proteins related to DNA repair, quinone oxidoreductase, regulation of microtubules and cell wall biogenesis were found to be upregulated. This means, that arctic Zygnema cells showed the capacity to actively repair damages to DNA, which is likely an effect of ionizing radiation. The ubiquinone oxidoreductase 13-kD-like subunit was also upregulated upon gamma irradiation. This protein is a coenzyme of the mitochondrial NADP-dehydrogenase, essential for NAPH oxidation. Interestingly, the regulation of cytoskeletal elements, in the particular case through an armadillo repeat-containing kinesin protein, that is involved in the ATP-binding mechanism (Choi et al., 2015). This might interact with organelle positioning and cell wall deposition.
In contrast, energy metabolism, isoprene biosynthesis and protein biosynthesis related proteins were down-regulated with the applied gamma-irradiation (Choi et al., 2015). This can be considered as a protection mechanism.
Algae and Microbe Interaction
There is increasing knowledge that charophytes serve as hosts for microbial communities that occur within surface mucilage matrices (Knack et al., 2015). These microbial associations were suggested to nurture the earliest plants and influence their biogeochemical roles. Microbiomes are known to be closely related to host organisms from certain lineages, it is hypothesized that microbiomes of early-diverging modern bryophytes and closely related charophyte green algae have commonalities reflecting the ancestral traits (Knack et al., 2015). Metagenomic sequence data were used from the late-diverging charophytes Chaetosphaeridium and Coleochaete, as well as the liverwort Conocephalum to infer bacteria and fungi for comparisons with the microbiome of an outgroup chlorophytic green alga Cladophora. It was found that the streptophyte (charophyte green algae and liverwort) microbiomes contained N-fixing cyanobacteria (NifH genes indicating nitrogen fixation), methanotrophs (pMMo genes indicating methane oxidation) and early diverging fungi. They were much more similar to each other than to the Cladophora microbiota (Knack et al., 2015).
Symbiotic interactions were also tested in a large scale transcriptomic analysis comparing 259 transcriptomes (10 green algal and basal land plant genomes) and were suggested to be involved in colonizing land by plants (Delaux et al., 2015). The appearance of a key regulator, a calcium and calmodulin-dependent protein kinase, suggested as symbiotic signaling pathway predated the conquering of land (Delaux et al., 2015). In their study, they tested the newly generated deep transcriptomes of the charophyte complex Closterium peracerosum-strigosum-littorale and the draft genome of Spirogyra sp. as well as transcriptomes of liverworts, mosses and hornworts. They report, that potential homologs of DMI1 and CCaMK (‘symbiotic genes’ belonging to the signaling module) were found in charophytes and even in chlorophytes (Delaux et al., 2015). This is insofar not surprising, as virtually all lichen forming algae (besides cyanophyta) belong to the chlorophytic lineage. Despite being very successful on land by tolerating extreme desiccation (e.g., Kranner et al., 2008), lichens did not give rise to further phylogenetic developments on land like the charophytes. The mutualistic fungal partners (Mucoromycotina/Glomeromycota), acting in some sort of primitive mycorrhizal symbiosis in early land plants, have recently been reviewed by Field et al. (2015).
Function of Plant Hormones
Phytohormones play a critical role in signal transduction upon stress reactions. Recently transcriptomic and genomic datasets became available in the early branching Klebsormidium (Holzinger et al., 2014; Hori et al., 2014), which allow to analyze the role of phytohormones in stress sensing (Figure 6). With the KEGG pathway reconstruction tool, almost complete pathways were found for cytokinin signaling, abscisic acid (ABA) signaling and ethylene response in K. crenulatum (Holzinger and Becker, 2015). In the draft sequence of K. flaccidum genome, signaling pathways for auxin, ABA, cytokinin, salicylic acid, and JA were found (Hori et al., 2014). Particularly the ABA response is well known in abiotic stress reactions. When K. crenulatum cells are exposed to severe desiccation stress ABA signaling components PP2C and SnRK2 were significantly increased, together with the nuclear AREB protein (Holzinger and Becker, 2015). There is a 70% overlap in differential gene expression profiles between plants that have been stressed with desiccation and high light, and it has to be stated that the majority of the ABA is synthesized in the plastids itself (de Vries et al., 2016). However, experimental ABA application did not change cold tolerance in K. flaccidum (Nagao et al., 2008). Also, orthologs for the cytokinin receptors CRE1/AHK (in the plasma membrane), cytoplasmic AHP and the transcription factor A-ARP were upregulated as a consequence of desiccation stress in K. crenulatum (Holzinger and Becker, 2015). The abiotic stress of severe desiccation regulates the expression of three classical phytohormone pathways in K. crenulatum, corroborating that these early branching charophyte algae have the prerequisites for living on land (Holzinger and Becker, 2015). For successful terrestrialization, mechanisms to sense the external environment are crucial.
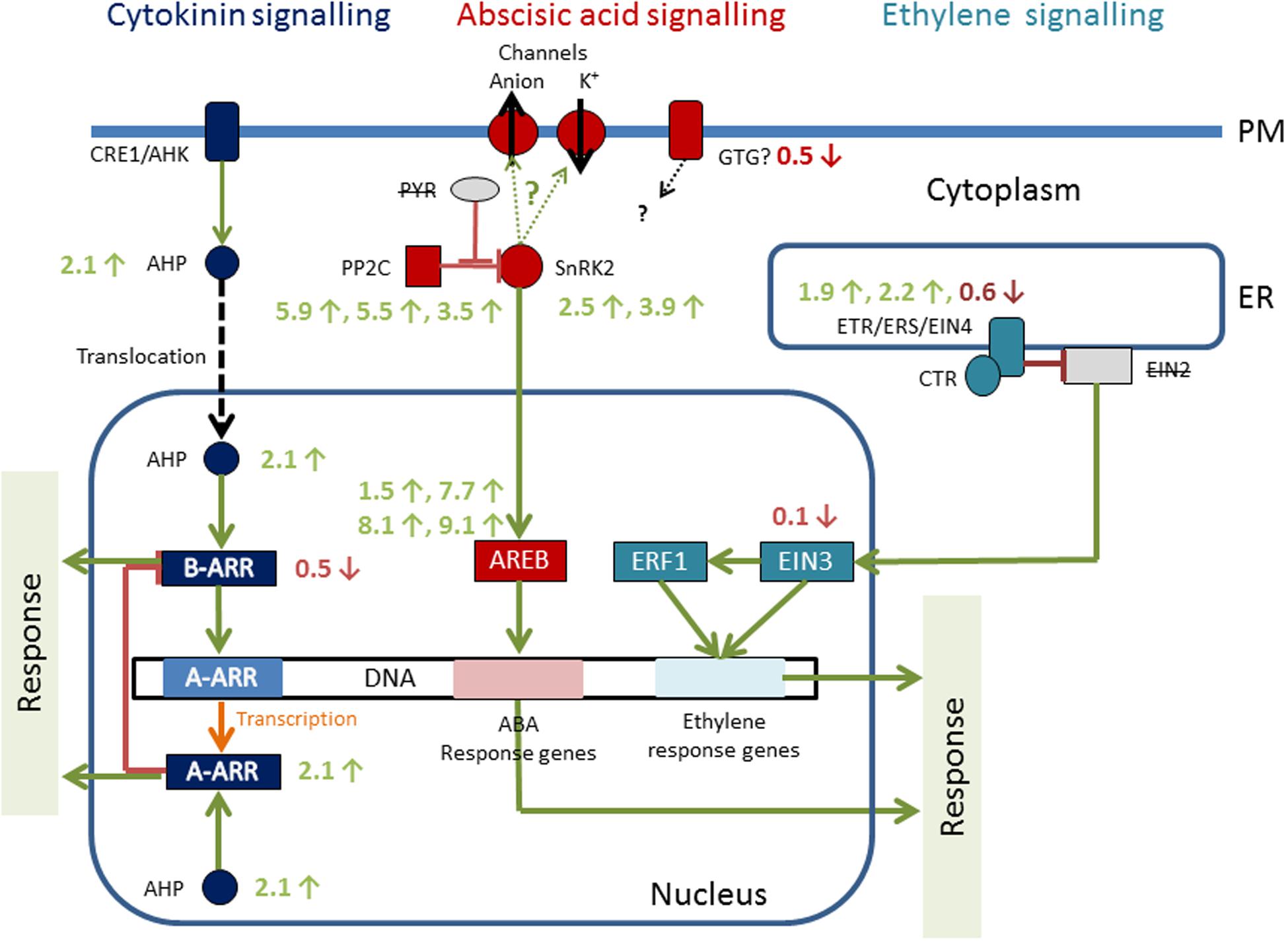
FIGURE 6. Hormone signaling pathways of Klebsormidium crenulatum; numbers are transcription changes (-fold) up or down regulation upon severe desiccation stress. Reprinted from Holzinger and Becker (2015).
First evidence for ethylene biosynthesis and pathway genes in charophyte green algae have been given by Timme and Delwiche (2010). Ethylene response appears to be highly conserved as a plant hormone for the past 450 mio years (Ju et al., 2015). It was shown, that Spirogyra ethylene-signaling homologs have the capacity to partially rescue Arabidopsis defective mutants, indicating homologous ethylene-signaling pathways, implying that the common aquatic ancestor possessed this pathway already prior to the colonization of land (Ju et al., 2015).
Strategies in an Evolutionary Point of View
In a recent review, Delwiche and Cooper (2015) give an outstanding overview on the evolutionary background of the origin of the terrestrial flora. The discrepancy between single-gene and morphological analysis and genome-scale phylogenetic analysis are discussed, the latter clearly indicating the Zygnematophyceae as sister taxon to land plants (Timme and Delwiche, 2010; Wodniok et al., 2011; Timme et al., 2012; Wickett et al., 2014; Zhong et al., 2014, 2015). Recently this view has additionally been supported by analysis of plastid evolution (de Vries et al., 2016). While many prerequisites for the terrestrial life-style are common in several groups of charophytes, only zygnematophyceaen algae share with land plants the transfer of a few plastid genes to the nucleus (de Vries et al. (2016). Chloroplasts became more submissive to nuclear control by key genes involved in the organelle division machinery (de Vries et al., 2016). Only basal charophyta (and some chlorophyta) possess the plastid division genes ftsI, ftsW, and minD. In contrast, ZCC-clade algae transferred these genes to the nucleus or lost them (de Vries et al., 2016). Interestingly, only the genus Zygnema possess cysA and cysT (encoding components in the sulfate ABC transporter system). These genes were not observed in any other zygnematophyceaen plastid genomes. Thus, the Zygnema plastid genome coding capacity was considered most similar to the hypothetical land plant ancestor (de Vries et al., 2016).
The link to understand evolution of terrestrial organisms from aquatic ones is to remember that life remains a fundamentally aquatic process (Delwiche and Cooper, 2015). The ancestral habitat for all charophyte lineages is freshwater, so terrestrial and semi-terrestrial environments are intermittently available to freshwater organisms. With the unpredictable changes of water availability organisms conquering land require desiccation tolerance mechanisms. Harholt et al. (2016) go even a step further, hypothesizing that charophyceaen green algal ancestors were already living on land for some time before the emergence of land plants. Their hypothesis is based on the idea that ancestral charophytes evolved their cell walls in response to the terrestrial selection pressure. However, this has to be seen critically, as strictly aquatic algae including chlorophytes have also evolved a complex cell wall modification machinery (e.g., Domozych et al., 2012). The occurrence of complex cell walls can thus not only be seen in connection with terrestrialization. Moreover, terrestrialization is not unique for charophytes evolutionary close to land plants, but also phylogenetic basal lineages contain terrestrial forms (e.g., chlorophytes like Stichococcus, Trentepohlia, Apatococcus, Prasiola).
Ecological differentiation has been suggested to trigger the formation of cryptic species in Klebsormidiales (Škaloud and Rindi, 2013). In this study, 14 lineages could be resolved based on concatenated ITS rDNA + rbcL data sets with clear preferences for either natural subaerial substrata, artificial subaerial substrata or aquatic habitats. These results were interpreted as an evidence for the existence of a high number of cryptic species within morphospecies (Škaloud and Rindi, 2013). These investigations were expanded to a global scale study in the same genus, where in addition to the global ubiquity strong biogeographic patterns were revealed on a local scale (Ryšánek et al., 2015). Again, for the local fine-scale structuring of the genotypes habitat differentiation was suggested. In a recent study by Ryšánek et al. (2016) one of these ecological factors – the pH value (pH 4 to pH 8) of the substrate was investigated, and hypothesized as a potential important factor for sympatric speciation.
Survival in vegetative state, e.g., in form of ‘pre-akinetes’ in Zygnema (Holzinger et al., 2009; Pichrtová et al., 2014a; Herburger et al., 2015) is important prerequisite for terrestrial life style. But also morphological features support this survival; One example is the holdfast of Spirogyra cells, which is preceded by callose deposition at the tip of developing rhizoids (Yamada et al., 2003). Branching is common in Z. ericetorum (Stancheva et al., 2014), and more complex morphologies can be observed in Coleochaete, still having the advantage of a small thallus (Leliaert et al., 2012). Structural simplicity might be advantageous in shallow and transient aquatic habitats, because even a thin film of water can provide enough room for coverage (Delwiche and Cooper, 2015). In addition, many species produce large amounts of mucilage with water holding capacities. The ultimate adaptation strategy to a terrestrial lifestyle is the sexual reproduction of Zygnematophyceae, which completely lacks flagellate stages and in the conjugation tube only gliding motility is necessary (Ikegaya et al., 2012).
In the present review, knowledge about stress tolerance mechanisms in Charophyta connected with life in terrestrial environment was summarized. We aimed to include insights derived from recent studies using omics techniques to investigate charophyte stress responses. A combination of methods will in future help to elucidate the stress tolerance that allows survival of this algal group under unfavorable conditions, and which key elements were responsible for the final transition to land.
Author Contributions
All authors listed, have substantial, direct and intellectual contribution to the work, and approved it for publication.
Conflict of Interest Statement
The authors declare that the research was conducted in the absence of any commercial or financial relationships that could be construed as a potential conflict of interest.
Acknowledgment
This review article was supported by Austrian Science Fund (FWF) Project P 24242-B16 and FWF project I 1951-B16 to AH and by The Czech Science Foundation grant 15-34645 L to MP.
References
Agrawal, S. C. (2009). Factors affecting spore germination in algae - review. Folia Microbiol. 54, 273–302. doi: 10.1007/s12223-009-0047-0
Aigner, S., Remias, D., Karsten, U., and Holzinger, A. (2013). Unusual phenolic compounds contribute to the ecophysiological performance in the purple-colored green alga Zygogonium ericetorum (Zygnematophyceae, Streptophyta) from a high-alpine habitat. J. Phycol. 49, 648–660. doi: 10.1111/jpy.12075
Allen, A., and Alston, R. E. (1959). Formation of purple pigment in Spirogyra pratensis cultures. Nature 183, 1064–1065. doi: 10.1038/1831064b0
Ashraf, M., and Godward, M. B. E. (1980). Ultrastructure and chemistry of the zygospore wall of Spirogyra. Ann. Bot. 46, 485–487.
Becker, B. (2013). Snowball earth and the split of Streptophyta and Chlorophyta. Trends Plant Sci. 18, 180–183. doi: 10.1016/j.tplants.2012.09.010
Becker, B., and Marin, B. (2009). Streptophyte algae and the origin of embryophytes. Ann. Bot. 103, 999–1004. doi: 10.1093/aob/mcp044
Belnap, J., and Lange, O. L. (2001). Biological Soil Crusts: Structure, Function and Management. Berlin: Springer.
Berry, H. A., and Lembi, C. A. (2000). Effects of temperature and irradiance on the seasonal variation of a Spirogyra (Chlorophyta) population in a midwestern lake (U.S.A.). J. Phycol. 36, 841–851. doi: 10.1046/j.1529-8817.2000.99138.x
Bisson, M. A., and Kirst, G. O. (1995). Osmotic acclimation and turgor pressure regulation in algae. Naturwissenschaften 82, 461–471. doi: 10.1007/BF01131597
Brosch-Salomon, S., Höftberger, M., Holzinger, A., and Lütz-Meindl, U. (1998). Ultrastructural localization of polysaccharides and N-acetyl-D-galactosamine in the secondary pathway of green algae (Desmidiaceae). J. Exp. Bot. 49, 145–153. doi: 10.1093/jxb/49.319.145
Brown, L. M., and Hellebust, J. A. (1980). The contribution of organic solutes to osmotic balance in some green and eustigmatophyte algae. J. Phycol. 16, 265–270. doi: 10.1111/j.0022-3646.1980.00265.x
Cannell, R. J., Farmer, P., and Walker, J. M. (1988). Purification and characterization of pentagalloylglucose, and alpha-glucosidase inhibitor/antibiotic from the freshwater green alga Spirogyra varians. Biochem. J. 255, 937–941. doi: 10.1042/bj2550937
Carreto, J. I., and Carignan, M. O. (2011). Mycosporine-like amino acids: relevant secondary metabolites. Chemical and ecological aspects. Marine Drugs 9, 387–446. doi: 10.3390/md9030387
Choi, J.-I., Yoon, M., Lim, S., Kim, G. H., and Park, H. (2015). Effect of gamma irradiation on physiological and proteomic changes of arctic Zygnema sp. (Chlorophyta, Zygnematales). Phycologia 54, 333–341. doi: 10.2216/14-106.1
Coba, F., Aguilera, J., Figueroa, F. L., Gálvez, M. V., and Herrera, E. (2009). Antioxidant activity of mycosporine-like amino acids isolated from three red macroalgae and one marine lichen. J. Appl. Phycol. 21, 161–169. doi: 10.1007/s10811-008-9345-1
Cockell, C. S., and Knowland, J. (1999). Ultraviolet radiation screening compounds. Biol. Rev. 74, 311–345. doi: 10.1111/j.1469-185X.1999.tb00189.x
Darehshouri, A., and Lütz-Meindl, U. (2010). H2O2 localization in the green alga Micrasterias after salt and osmotic stress by TEM-coupled electron energy loss spectroscopy. Protoplasma 239, 49–56. doi: 10.1007/s00709-009-0081-4
de Bakker, N. V. J., van Bodegom, P. M., van de Poll, W. H., Boelen, P., Nat, E., Rozema, J., et al. (2005). Is UV-B radiation affecting charophycean algae in shallow freshwater systems? New Phytol. 166, 957–966. doi: 10.1111/j.1469-8137.2005.01377.x
de Vries, J., Stanton, A., Archibald, J. M., and Gould, S. B. (2016). Streptophyte terrestrialization in light of plastid evolution. Trends Plant Sci doi: 10.1016/j.tplants.2016.01.021 [Epub ahead of print].
Delaux, P.-M., Radhakrishnan, G. V., Jayaraman, D., Cheema, J., Malbreil, M., Volkening, J. D., et al. (2015). Algal ancestors of land plants was prepared for symbiosis. Proc. Natl. Acad. Sci. U.S.A. 112, 13390–13395. doi: 10.1073/pnas.1515426112
Delwiche, C. F., and Cooper, E. D. (2015). The evolutionary origin of a terrestrial flora. Curr. Biol. 25, R899–R910. doi: 10.1016/j.cub.2015.08.029
Delwiche, C. F., Graham, L. E., and Thomson, N. (1989). Lignin-like compounds and sporopollenin in Coleochaete, an algal model for land plant ancestry. Science 245, 399–401. doi: 10.1126/science.245.4916.399
Domozych, D. S., Ciancia, M., Fangel, J. U., Mikkelsen, M. D., Ulvskov, P., and Willats, W. G. T. (2012). The cell walls of green algae: a journey through evolution and diversity. Front. Plant Sci. 3:82. doi: 10.3389/fpls.2012.0008210.3389/fpls.2012.00082
Domozych, D. S., and Domozych, C. E. (2014). Multicellularity in green algae: upsizing in a walled complex. Front. Plant Sci. 5:649. doi: 10.3389/fpls.2014.00649
Domozych, D. S., and Rogers-Domozych, C. (1993). Mucilage processing and secretion in the green alga Closterium II. Ultrastructure and immunocytochemistry. J. Phycol. 29, 659–667. doi: 10.1111/j.0022-3646.1993.00659.x
Domozych, D. S., Serfis, A., Kiemle, S. N., and Gretz, M. R. (2007). The structure and biochemistry of charophycean cell walls. I. Pectins of Penium margaritaceum. Protoplasma 230, 99–115. doi: 10.1007/s00709-006-0197-8
Eder, M., and Lütz-Meindl, U. (2010). Analyses and localization of pectin- like carbohydrates in cell wall and mucilage of the green alga Netrium digitus. Protoplasma 243, 25–38. doi: 10.1007/s00709-009-0040-0
Eder, M., Tenhaken, R., Driouich, A., and Lütz-Meindl, U. (2008). Occurrence and characterization of arabinogalactan-like proteins and hemicelluloses in Micrasterias (Streptophyta). J. Phycol. 44, 1221–1234. doi: 10.1111/j.1529-8817.2008.00576.x
Elster, J., Degma, P., Kovacik, L., Valentova, L., Sramkova, K., and Pereira, B. (2008). Freezing and desiccation injury resistance in the filamentous green alga Klebsormidium from Antarctic, Arctic Slovakia. Biologie. 63, 839–847.
Fernandez-Marin, B., Holzinger, A., and Garcia-Plazaola, J. I. (2016). “Photosynthetic strategies of desiccation-tolerant organisms,” in Handbook of Photosynthesis, 3rd Edn, Chap. 38, ed. M. Pessarakli (Boca Raton, FL: CRC Press), 719–737.
Field, K. J., Pressel, S., Duckett, J. G., Rimington, W. R., and Bidartondo, M. I. (2015). Symbiotic options for the conquest of land. Trends Ecol. Evol. 30, 477–486. doi: 10.1016/j.tree.2015.05.007
Figueroa, F. L., Korbee, N., Carillo, P., Medina-Sanchez, J. M., Mata, M., Bonomi, J., et al. (2009). The effects of UV radiation on photosynthesis estimated as chlorophyll fluorescence in Zygnemopsis decussata (Chlorophyta) growing in a high mountain lake (Sierra Nevada. Southern Spain). J. Limnol. 68, 206–216.
Fuller, C. (2013). Examining Morphological and Physiological Changes in Zygnema Irregulare During A Desiccation and Recovery Period. Master’s thesis, California State University, San Marcos San Marcos CA.
Germ, M., Kreft, I., and Gaberšćik, A. (2009). UV-B radiation and selenium affected energy availability in green alga Zygnema. Biologia 64, 676–679. doi: 10.2478/s11756-009-0062-2
Gerotto, C., and Morosinotto, T. (2013). Evolution of photoprotection mechanisms upon land colonization: evidences of PSBS dependent NPQ in late Streptophyte algae. Physiol. Plant 149, 583–598. doi: 10.1111/ppl.12070
Graham, J. M., Lembi, C. A., Adrian, H. L., and Spencer, D. F. (1995). Physiological responses to temperature and irradiance in Spirogyra (Zygnematales. Charophyceae). J. Phycol. 31, 531–540. doi: 10.1111/j.1529-8817.1995.tb02546.x
Graham, L. E., Arancibia-Avila, P., Taylor, W. A., Strother, P. K., and Cook, M. E. (2012). Aeroterrestrial Coleochaete (Streptophyta, Coleochaetales) models early plant adaptation to land. Am. J. Bot. 99, 130–144. doi: 10.3732/ajb.1100245
Graham, L. E., Cook, M. E., and Busse, J. S. (2000). The origin of plants: body plan changes contributing to a major evolutionary radiation. Proc. Natl. Acad Sci. U.S.A. 97, 4535–4540. doi: 10.1073/pnas.97.9.4535
Graham, L. E., Lewis, L. A., Taylor, W., Wellman, C., and Cook, M. E. (2013). “Early terrestrialization: transition from algal to bryophyte grade,” in Advances in Photosynthesis and Respiration, eds Hanson and S. Rice (Dordecht: Springer), 9–28.
Gustavs, L., Eggert, A., Michalik, D., and Karsten, U. (2010). Physiological and biochemical responses of green microalgae from different habitats to osmotic and matric stress. Protoplasma 243, 3–14. doi: 10.1007/s00709-009-0060-9
Harholt, J., Moestrup, O., and Ulvskov, P. (2016). Why plants were terrestrial from the beginning. Trends Plant Sci. 21, 96–101. doi: 10.1016/j.tplants.2015.11.010
Hawes, I. (1990). Effects of freezing and thawing on a species of Zygnema (Chlorophyta) from the Antarctic. Phycologia 29, 326–331. doi: 10.2216/i0031-8884-29-3-326.1
Herburger, K., and Holzinger, A. (2015). Localization and quantification of callose in the streptophyte green algae Zygnema and Klebsormidium: correlation with desiccation tolerance. Plant Cell Physiol. 56, 2259–2270. doi: 10.1093/pcp/pcv139
Herburger, K., Karsten, U., and Holzinger, A. (2016a). Entransia and Hormidiella, sister lineages of Klebsormidium (Streptophyta), respond differently to light, temperature and desiccation stress. Protoplasma doi: 10.1007/s00709-015-0889-z [Epub ahead of print].
Herburger, K., Remias, D., and Holzinger, A. (2016b). The green alga Zygogonium ericetorum (Zygnematophyceae, Charophyta) shows high iron and aluminium tolerance: protection mechanisms and photosynthetic performance. FEMS Microbiol. Ecol.
Herburger, K., Lewis, L. A., and Holzinger, A. (2015). Photosynthetic efficiency, desiccation tolerance and ultrastructure in two phylogenetically distinct strains of alpine Zygnema sp. (Zygnematophyceae, Streptophyta): role of pre-akinete formation. Protoplasma 252, 571–589. doi: 10.1007/s00709-014-0703-3
Holzinger, A., and Becker, B. (2015). Desiccation tolerance in the streptophyte green alga Klebsormidium: the role of phytohormones. Comm. Integr. Biol. 8:e1059978. doi: 10.1080/19420889.2015.1059978
Holzinger, A., Kaplan, F., Blaas, K., Zechmann, B., Komsic-Buchmann, K., and Becker, B. (2014). Transcriptomics of desiccation tolerance in the streptophyte green alga Klebsormidium reveal a land plant-like defense. PLoS ONE 9:e110630. doi: 10.1371/journal.pone.0110630
Holzinger, A., and Karsten, U. (2013). Desiccation stress and tolerance in green algae: consequences for ultrastructure, physiological and molecular mechanisms. Front. Plant Sci. 4:327. doi: 10.3389/fpls.2013.00327
Holzinger, A., and Lütz, C. (2006). Algae and UV irradiation: effects on ultrastructure and related metabolic functions. Micron 37, 190–207. doi: 10.1016/j.micron.2005.10.015
Holzinger, A., Roleda, M. Y., and Lütz, C. (2009). The vegetative arctic green alga Zygnema is insensitive to experimental UV exposure. Micron 40, 831–838. doi: 10.1016/j.micron.2009.06.008
Holzinger, A., Tschaikner, A., and Remias, D. (2010). Cytoarchitecture of the desiccation-tolerant green alga Zygogonium ericetorum. Protoplasma 243, 15–24. doi: 10.1007/s00709-009-0048-5
Hoppert, M., Reimer, R., Kemmling, A., Schröder, A., Günzl, B., and Heinken, T. (2004). Structure and reactivity of a biological soil crust from a xeric sandy soil in central Europe. Geomicrobiol. J. 21, 183–191. doi: 10.1080/01490450490275433
Hori, K., Maruyama, F., Fujisawa, T., Togashi, T., Yamamoto, N., Seo, M., et al. (2014). Klebsormidium flaccidum genome reveals primary factors for plant terrestrial adaptation. Nat. Comm. 5:3978. doi: 10.1038/ncomms4978
Ikegaya, H., Nakase, T., Iwata, K., Tsuchida, H., Sonobe, S., and Shimmen, T. (2012). Studies on conjugation of Spirogyra using monoclonal culture. J. Plant Res. 125, 457–464. doi: 10.1007/s10265-011-0457-3
Ju, C., Van de Poel, B., Cooper, E. D., Thierer, J. H., Gibbons, T. R., Delwiche, C. F., et al. (2015). Conservation of ethylene as a plant hormone over 450 million years of evolution. Nat. Plants 1:14004. doi: 10.1038/nplants.2014.4
Kadlubowska, J. Z. (1984). “Conjugatophyceae I: chlorophyta VIII: zygnemales,” in Süsswasserflora von Mitteleuropa, Band 16, eds H. Ettl, J. Gerloff, H. Heynig, and D. Mollenhauer (Jena: Gustav Fisher), 1–532.
Kaplan, F., Lewis, L. A., Herburger, K., and Holzinger, A. (2013). Osmotic stress in the arctic and antarctic green alga Zygnema sp. (Zygnemtales, Streptophyta): effects on photosynthesis and ultrastructure. Micron 44, 317–330. doi: 10.1016/j.micron.2012.08.004
Kaplan, F., Lewis, L. A., Wastian, J., and Holzinger, A. (2012). Plasmolysis effects and osmotic potential of two phylogenetically distinct alpine strains of Klebsormidium (Streptophyta). Protoplasma 249, 789–804. doi: 10.1007/s00709-011-0324-z
Karsten, U., Herburger, K., and Holzinger, A. (2014). Dehydration, temperature and lighttolerance in members of the aeroterrestrial green algal genus Interfilum (Streptophyta) from biogeographically different temperate soils. J. Phycol. 50, 804–816. doi: 10.1111/jpy.12210
Karsten, U., Herburger, K., and Holzinger, A. (2015). Living in biological soil crust communities of African deserts – physiological traits of green algal Klebsormidium species (Streptophyta) to cope with desiccation, light and temperature gradients. J. Plant Physiol. doi: 10.1016/j.jplph.2015.09.002 [Epub ahead of print].
Karsten, U., and Holzinger, A. (2012). Light, temperature and desiccation effects onphotosynthetic activity, and drought-induced ultrastructural changes in the green alga Klebsormidium dissectum (Streptophyta) from a high alpine soil crust. Microb. Ecol. 63, 51–63. doi: 10.1007/s00248-011-9924-6
Karsten, U., and Holzinger, A. (2014). Green algae in alpine biological soil crust communities: acclimation strategies against ultraviolet radiation and dehydration. Biodivers. Conserv. 23, 1845–1858. doi: 10.1007/s10531-014-0653-2
Karsten, U., Lütz, C., and Holzinger, A. (2010). Ecophysiological performance of the aeroterrestrial green alga Klebsormidium crenulatum (Klebsormidiophyceae, Streptophyta) isolated from an alpine soil crust with an emphasis on desiccation stress. J. Phycol. 46, 1187–1197. doi: 10.1111/j.1529-8817.2010.00921.x
Karsten, U., Pröschold, T., Mikhailyuk, T., and Holzinger, A. (2013). Photosynthetic performance of different genotypes of the green alga Klebsormidium sp. (Streptophyta) isolated from biological soil crusts of the Alps. Algol. Stud. 142, 45–62. doi: 10.1127/1864-1318/2013/0102
Karsten, U., and Rindi, F. (2010). Ecophysiological performance of an urban strain of the aeroterrestrial green alga Klebsormidium sp. (Klebsormidiales, Klebsormidiophyceae). Eur. J. Phycol. 45, 426–435. doi: 10.1080/09670262.2010.498587
Kim, G. H., Yoon, M., and Klochkova, T. A. (2005). A moving mat: phototaxis in the filamentous green algae Spirogyra (Chlorophyta, Zygnemataceae). J. Phycol. 41, 232–237. doi: 10.1111/j.1529-8817.2005.03234.x
Kitzing, C., and Karsten, U. (2015). UV-induced effects on optimum quantum yield andsunscreen contents in members of the genera Interfilum, Klebsormidium, Hormidiella and Entransia (Klebsormidiophyceae, Streptophyta). Eur. J. Phycol. 50, 279–287. doi: 10.1080/09670262.2015.1031190
Kitzing, C., Pröschold, T., and Karsten, U. (2014). UV-induced effects on growth, photosynthetic performance and sunscreen contents in different populations of the green alga Klebsormidium fluitans (Streptophyta) from alpine soil crusts. Microb. Ecol. 67, 327–340. doi: 10.1007/s00248-013-0317-x
Knack, J. J., Wilcox, L. W., Delaux, P.-M., Ané, J.-M., Piotrowski, M. J., Cook, M. E., et al. (2015). Microbiomes of streptophyte algae and bryophytes suggest that a functional suite of microbiota fostered plant colonization of land. Int. J. Plant Sci. 176, 405–420. doi: 10.1086/681161
Kranner, I., Beckett, R., Hochman, A., and Nash, T. H. I. I. I. (2008). Desiccation-tolerance in lichens: a review. Bryologist 111, 576–593. doi: 10.1639/0007-2745-111.4.576
Kroken, S. B., Graham, L. E., and Cook, M. E. (1996). Occurrence and evolutionary significance of resistant cell walls in charophytes and bryophytes. Am. J. Bot. 83, 1241–1254. doi: 10.2307/2446108
Küster, A., Schaible, R., and Schubert, H. (2000). Light acclimation of the charophyte Lamprothamnium papulosum. Aquat. Bot. 68, 205–216. doi: 10.1016/S0304-3770(00)00122-4
Lajos, K., Mayr, S., Buchner, O., and Holzinger, A. (2016). A new microscopic method to analyse desiccation induced volume changes in aeroterrestrial green algae. J. Microsc. doi: 10.1111/jmi.12409 [Epub ahead of print].
Leliaert, F., Smith, D. R., Moreau, H., Herron, M. D., Verbruggen, H., Delchiche, C. F., et al. (2012). Phylogeny and molecular evolution of green algae. Crit. Rev. Plant Sci. 31, 1–46. doi: 10.1080/07352689.2011.615705
Lewis, L. A., and McCourt, R. M. (2004). Green algae and the origin of land plants. Am. J. Bot. 91, 1535–1556. doi: 10.3732/ajb.91.10.1535
Lütz, C., Seidlitz, H. K., and Meindl, U. (1997). Physiological and structural changes in the chloroplast of the green alga Micrasterias denticulata induced by UV-B simulation. Plant Ecol. 128, 54–64.
Lütz-Meindl, U., Luckner, M., Andosch, A., and Wanner, G. (2015). Structural stress responses and degradation of dictyosomes in algae analysed by TEM and FIB-SEM tomography. J. Microsc. doi: 10.1111/jmi.12369 [Epub ahead of print].
Meindl, U., and Lütz, C. (1996). Effects of UV irradiation on cell development and ultrastructure of the green alga Micrasterias. J. Photochem. Photobiol. B Biol. 36, 285–292. doi: 10.1016/S1011-1344(96)07395-2
Meindl, U., Wittmann-Pinegger, D., and Kiermayer, O. (1989). Cell multiplication and ultrastructure of Micrasterias denticulata (Desmidiaceae) grown under salt stress. Plant Syst. Evol. 164, 197–208. doi: 10.1007/BF00940437
Mikhailyuk, T., Holzinger, A., Massalski, A., and Karsten, U. (2014). Morphology and ultrastructure of Interfilum and Klebsormidium (Klebsormidiales, Streptophyta) with special reference to cell division and thallus formation. Eur. J. Phycol. 49, 395–412. doi: 10.1080/09670262.2014.949308
Mikkelsen, M. D., Harholt, J., Ulvskov, P., Johansen, I. E., Fangel, J. U., Doblin, M. S., et al. (2014). Evidence for land plant cell wall biosynthetic mechanisms in charophyte green algae. Ann. Bot. 114, 1217–1236. doi: 10.1093/aob/mcu171
Morison, M., and Sheath, R. (1985). Response to desiccation stress by Klebsormidium rivulare (Ulotrichales, Chlorophyta) from a rhode Island stream. Phycologia 24, 129–145. doi: 10.2216/i0031-8884-24-2-129.1
Nagao, M., Matsui, K., and Uemura, M. (2008). Klebsormidium flaccidum, a charophycean green alga, exhibits cold acclimation that is closely associated with compatible solute accumulation and ultrastructural changes. Plant Cell Environ. 31, 872–885. doi: 10.1111/j.1365-3040.2008.01804.x
Nagao, M., and Uemura, M. (2012). Sucrose phosphate phosphatase in the green alga Klebsormidium flaccidum (Streptophyta) lacks an extensive C-terminal domain and differs from that of land plants. Planta 235, 851–861. doi: 10.1007/s00425-011-1550-5
Neustupa, J., Eliáš, M., and Šejnohová, L. (2007). A taxonomic study of two Stichococcus species (Trebouxiophyceae, Chlorophyta) with a starch-enveloped pyrenoid. Nova Hedwigia 84, 51–63. doi: 10.1127/0029-5035/2007/0084-0051
Newsome, A. G., Murphy, B. T., and van Breemen, R. B. (2013). Isolation and characterization of natural blue pigments from underexplored sources. Phys. Method Food Anal. 1138, 105–125. doi: 10.1021/bk-2013-1138.ch008
Nishizawa, M., Yamagishi, T., Nonaka, G.-I., Nishioka, I., and Ragan, M. A. (1985). Gallotannins of the freshwater green alga Spirogyra sp. Phytochemistry 24, 2411–2413. doi: 10.1016/S0031-9422(00)83053-8
Oertel, A., Aichinger, N., Hochreiter, R., Thalhammer, J., and Lütz-Meindl, U. (2004). Analysis of mucillage secretion and excretion in Micrasterias (Chlorophyta) by means of immunoelectron microscopy and digital time lapse video microscoy. J. Phycol. 40, 711–720. doi: 10.1111/j.1529-8817.2004.03222.x
Oren, A. (2007). “Diversity of organic osmotic compounds and osmotic adaptation in cyanobacteria and algae,” in Algae and Cyanobacteria in Extreme Environments, ed. J. Seckbach (Berlin: Springer), 639–655.
O’Rourke, C., Gregson, T., Murray, L., Sadler, I. H., and Fry, S. C. (2015). Sugar composition of the pectic polysaccharides of charophytes, the closest algal relatives of land-plants: presence of 3-O-methyl-D-galactose residues. Ann. Bot. 116, 225–236. doi: 10.1093/aob/mcv089
Pichrtová, M., Arc, E., Stöggl, W., Kranner, I., Hájek, T., Hackl, H., et al. (2016a). Formation of lipid bodies and fatty acid composition changes upon pre-akinete formation in arctic and Antarctic Zygnema (Zygnematophyceae, Streptophyta) strains. FEMS Micobiol. Ecol. doi: 10.1093/femsec/fiw096
Pichrtová, M., Hájek, T., and Elster, J. (2014a). Osmotic stress and recovery in field populations of Zygnema sp. (Zygnematophyceae, Streptophyta) on Svalbard (High Arctic) subjected to natural desiccation. FEMS Microbiol. Ecol. 89, 270–280. doi: 10.1111/1574-6941.12288
Pichrtová, M., Hájek, T., and Elster, J. (2016b). Annual development of mat-forming conjugating green algae Zygnema spp. in hydroterrestrial habitats in the Arctic. Polar Biol.
Pichrtová, M., Kulichová, J., and Holzinger, A. (2014b). Nitrogen limitation and slow drying induce desiccation tolerance in conjugating green algae (Zygnematophyceae) from polar habitats. PLoS ONE 9:e113137. doi: 10.1371/journal.pone.0113137
Pichrtová, M., Remias, D., Lewis, L., and Holzinger, A. (2013). Changes in phenolic compounds and cellular ultrastructure of Arctic and Antarctic strains of Zygnema (Zygnematophyceae, Streptophyta) after exposure to experimentally enhanced UV to PAR ratio. Micorb. Ecol. 65, 68–83. doi: 10.1007/s00248-012-0096-9
Popper, Z. A., Michel, G., Herve, C., Domozych, D. S., Willats, W. G. T., Tuohy, M. G., et al. (2011). Evolution and diversity of plant cell walls: from algae to flowering plants. Annu. Rev. Plant Biol. 62, 567–590. doi: 10.1146/annurev-arplant-042110-103809
Popper, Z. A., Ralet, M. C., and Domozych, D. S. (2014). Plant and algal cell walls: diversity and functionality. Ann. Bot. 114, 1043–1048. doi: 10.1093/aob/mcu214
Popper, Z. A., and Tuohy, M. G. (2010). Beyond the green: understanding the evolutionary puzzle of plant and algal cell walls. Plant Physiol. 153, 373–383. doi: 10.1104/pp.110.158055
Poulíčková, A., Žižka, Z., Hašler, P., and Benada, O. (2007). Zygnematalean zygospores: morphological features and use in species identification. Folia Microbiol. 52, 135–145. doi: 10.1007/BF02932152
Proctor, V. W. (1967). Storage and germination of Chara oospores. J. Phycol. 3, 90–92. doi: 10.1111/j.1529-8817.1967.tb04638.x
Remias, D., Holzinger, A., Aigner, S., and Lütz, C. (2012a). Ecophysiology and ultrastructure of Ancylonema nordenskiöldii (Zygnematales, Streptophyta), causing brown ice on glaciers in Svalbard (high arctic). Polar Biol. 35, 899–908. doi: 10.1007/s00300-011-1135-6
Remias, D., Holzinger, A., and Lütz, C. (2009). Ultrastructure and physiological characterization of the ice alga Mesotaenium berggrenii (Zygnemaphyceae, Chlorophyta) from glaciers in the European Alps. Phycologia 48, 302–312. doi: 10.2216/08-13.1
Remias, D., Schwaiger, S., Aigner, S., Leya, T., Stuppner, H., and Lütz, C. (2012b). Characterization of an UV- and VIS-absorbing, purpurogallin-derived secondary pigment new to algae and highly abundant in Mesotaenium berggrenii (Zygnematophyceae, Chlorophyta), an extremophyte living on glaciers. FEMS Microbiol. Ecol. 79, 638–648. doi: 10.1111/j.1574-6941.2011.01245.x
Roach, T., and Krieger-Liszkay, A. (2014). Regulation of photosynthtic electron transport and photoinhibition. Curr. Prot. Pept. Sci. 15, 351–362. doi: 10.2174/1389203715666140327105143
Ryšánek, D., Holzinger, A., and Škaloud, P. (2016). Influence of substrate and pH on the diversity of aeroterrestrial alga Klebsormidium (Klebsormidiales, Streptohyta): a potentially important factor for sympatric speciation. Phycologia doi: 10.2216/15-110.1
Ryšánek, D., Hrèková, K., and Škaloud, P. (2015). Global ubiquity and local endemism of free-living terrestrial protists: phylogeographic assessment of the streptophyte alga Klebsormidium. Enviorn. Microbiol. 17, 689–698. doi: 10.1111/1462-2920.12501
Sederias, J., and Colman, B. (2007). The interaction of light and low temperature on breaking the dormancy of Chara vulgaris oospores. Aquat. Bot. 87, 229–234. doi: 10.1016/j.aquabot.2007.06.008
Sharma, N., Rai, A., Singh, S., and Brown, R. (2007). Airborne algae: their present status and relevance. J. Phycol. 43, 615–627. doi: 10.1111/j.1529-8817.2007.00373.x
Shick, J. M., and Dunlap, W. C. (2002). Mycosporine-like amino acids and related gadusols: biosynthesis, acumulation, and UV-protective functions in aquatic organisms. Ann. Rev. Physiol. 64, 223–262. doi: 10.1146/annurev.physiol.64.081501.155802
Škaloud, P. (2009). Species composition and diversity of aero-terrestrial algae and cyanobacteria of the Boreè Hill ventaroles. Fottea 9, 65–80.
Škaloud, P., and Rindi, F. (2013). Ecological differentiation of cryptic species within an asexual protist morphospecies:a case study of filamentous green alga Klebsormidium (Streptophyta). J. Eukaryot. Microbiol. 60, 350–362. doi: 10.1111/jeu.12040
Sørensen, I., Pettolino, F. A., Bacic, A., Ralph, J., Lu, F., O’Neill, M. A., et al. (2011). The charophycean green algae provide insights into the early origins of plant cell walls. Plant J. 68, 201–211. doi: 10.1111/j.1365-313X.2011.04686.x
Sørensen, I., Rose, J. K. C., Doyle, J. D., Domozych, D. S., and Willats, G. T. (2012). The charophycean green algae as model systems to study plant cell walls and other evolutionary adaptations that gave rise to land plants. Plant Sign. Behav. 7, 1–3. doi: 10.4161/psb.7.1.18574
Stamenković, M., Bischof, K., and Hanelt, D. (2014). Xanthophyll cycle pool size and composition in several Cosmarium strains (Zygnematophyceae, Streptophyta) are related to their geographic distribution patterns. Protoist 165, 14–30. doi: 10.1016/j.protis.2013.10.002
Stamenković, M., and Hanelt, D. (2011). Growth and photosynthetic characteristics of several Cosmarium strains (Zygnematophyceae, Streptophyta) isolated from various geographic regions under a constant light-temperature regime. Aquat. Ecol. 45, 455–472. doi: 10.1007/s10452-011-9367-7
Stamenković, M., and Hanelt, D. (2014). Sensitivity of photosynthesis to UV radiation in sensitivity of photosynthesis to UV radiation in several Cosmarium strains (Zygnematophyceae, Streptophyta) is related to their geographic distribution. Photochem. Photobiol. Sci. 13, 1066–1081. doi: 10.1039/c3pp50192b
Stancheva, R., Hall, J. D., Herburger, K., Lewis, L. A., McCourt, R. M., Sheath, R. G., et al. (2014). Phylogenetic position of Zygogonium ericetorum (Zygnematophyceae, Charophyta) from a high alpine habitat and ultrastructural characterization of unusual aplanospores. J. Phycol. 50, 790–803. doi: 10.1111/jpy.12229
Stancheva, R., Hall, J. D., McCourt, R. M., and Sheath, R. G. (2013). Identity and phylogenetic placement of Spirogyra species (Zygnematophyceae, Charophyta) from California streams and elsewhere. J. Phycol. 49, 588–607. doi: 10.1111/jpy.12070
Stancheva, R., Herburger, K., Sheath, R. G., and Holzinger, A. (2016). Conjugation morphology of Zygogonium ericetorum (Zygnematophyceae, Charophyta) from a high alpine habitat. J. Phycol. 52, 131–134. doi: 10.1111/jpy.12363
Stancheva, R., Sheath, R. G., and Hall, J. D. (2012). Systematics of the genus Zygnema (Zygnematophyceae, Charophyta) from Californian watersheds. J. Phycol. 48, 409–422. doi: 10.1111/j.1529-8817.2012.01127.x
Štástný, J. (2010). Desmids (Conjugatophyceae, Viridiplantae) from the Czech Republic; new and rare taxa, distribution, ecology. Fottea 10, 1–74.
Stibal, M., Šabacká, M., and Žárský, J. (2012). Biological processes on glacier and ice sheet surfaces. Nature Geoscience 5, 771–774. doi: 10.1038/ngeo1611
Thangaraj, G. (2016). Antarctic strain of green filamentous alga Zygnema sp. shows a high resistance to photoinhibition under simulated polar conditions. Czech Polar Rep. 5, 176–184. doi: 10.5817/CPR2015-2-15
Timme, R. E., Bachvaroff, T. R., and Delwiche, C. F. (2012). Broad phylogenomic sampling and the sister lineage of land plants. PLoS ONE 7:e29696. doi: 10.1371/journal.pone.0029696
Timme, R. E., and Delwiche, C. F. (2010). Uncovering the evolutionary origin of plant molecular processes: comparison of Coleochaete (Coleochaetales) and Spirogyra (Zygnematales) transcriptomes. BMC Plant Biol. 10:96. doi: 10.1186/1471-2229-10-96
Versteegh, G. J. M., and Blokker, P. (2004). Resistant macromolecules of extant and fossil microalgae. Phycol. Res. 52, 325–339. doi: 10.1111/j.1440-1835.2004.tb00342.x
Vieira, J., and Necchi, O. (2003). Photosynthetic characteristics of charophytes from tropical lotic ecosystems. Phycol. Res. 51, 51–60. doi: 10.1111/j.1440-1835.2003.tb00170.x
Vilumbrales, D. M., Skácelová, K., and Barták, M. (2013). Sensitivity of Antarctic freshwater algae to salt stress assessed by fast chlorophyll fluorescence transient. Czech Polar Rep. 3, 163–172. doi: 10.5817/CPR2013-2-17
Wanner, G., Schäfer, T., and Lütz-Meindl, U. (2013). 3-D analysis of dictyosomes and multivesicular bodies in the green alga Micrasterias denticulata by FIB/SEM tomography. J. Struct. Biol. 184, 203–211. doi: 10.1016/j.jsb.2013.10.003
Welsh, D. (2000). Ecological significance of compatible solute accumulation by micro organisms: from single cells to global climate. FEMS Microbiol. Rev. 24, 263–290. doi: 10.1111/j.1574-6976.2000.tb00542.x
Weng, J.-K., and Chapple, C. (2010). The origin and evolution of lignin biosynthesis. New Phytol. 187, 273–285. doi: 10.1111/j.1469-8137.2010.03327.x
Wickett, N. J., Mirarab, S., Ngyuyen, N., Warnow, T., Carpenter, E., Matasci, N., et al. (2014). Phylotrascriptomic analysis of the origin and early diversification of land plants. Proc. Natl. Acad. Sci. U.S.A. 111, E4859–E4868. doi: 10.1073/pnas.1323926111
Wodniok, S., Brinkmann, H., Glöckner, G., Heidel, A. J., Philippe, H., Melkonian, M., et al. (2011). Origin of land plants: do conjugating green algae hold the key? BMC Evol. Biol. 11:104. doi: 10.1186/1471-2148-11-104
Yamada, S., Sonobe, S., and Shimmen, T. (2003). Synthesis of a callosic substance during rhizoid differentiation in Spirogyra. Plant Cell Physiol. 44, 1225–1228. doi: 10.1093/pcp/pcg152
Yancey, P. (2005). Organic osmolytes as compatible, metabolic and counteracting cytoprotectants in high osmolarity and other stresses. J. Exp. Biol. 208, 2819–2830. doi: 10.1242/jeb.01730
Yin, Y., John, M. A., Cao, H., and Rupani, M. (2014). A survey of plant and algal genomes and transcriptomes reveals new insights into the evolution and function of the cellulose synthase superfamily. BMC Genomics 15:260. doi: 10.1186/1471-2164-15-260
Zhong, B., Sun, L., and Penny, D. (2015). The origin of land plants: a phylogenomic perspective. Evol. Bioinform. 11, 137–141. doi: 10.4137/EBO.S29089
Keywords: transcriptomics, proteomics, metabolomics, UV irradiation, desiccation, phylogenomic analysis
Citation: Holzinger A and Pichrtová M (2016) Abiotic Stress Tolerance of Charophyte Green Algae: New Challenges for Omics Techniques. Front. Plant Sci. 7:678. doi: 10.3389/fpls.2016.00678
Received: 24 March 2016; Accepted: 02 May 2016;
Published: 20 May 2016.
Edited by:
Zoë A. Popper, National University of Ireland, IrelandReviewed by:
John Moore, Stellenbosch University, South AfricaSven B. Gould, Heinrich-Heine-Universität Düsseldorf, Germany
Copyright © 2016 Holzinger and Pichrtová. This is an open-access article distributed under the terms of the Creative Commons Attribution License (CC BY). The use, distribution or reproduction in other forums is permitted, provided the original author(s) or licensor are credited and that the original publication in this journal is cited, in accordance with accepted academic practice. No use, distribution or reproduction is permitted which does not comply with these terms.
*Correspondence: Andreas Holzinger, YW5kcmVhcy5ob2x6aW5nZXJAdWliay5hYy5hdA==