- 1Key Laboratory of Economic Plants and Biotechnology, Kunming Institute of Botany, Chinese Academy of Sciences, Kunming, China
- 2Yunnan Key Laboratory for Wild Plant Resources, Kunming, China
- 3University of Chinese Academy of Sciences, Beijing, China
Due to the fluctuating water availability in the arboreal habitat, epiphytic plants are considered vulnerable to climate change and anthropogenic disturbances. Although co-occurring taxa have been observed divergent adaptive performances in response to drought, the underlying physiological and morphological mechanisms by which epiphyte species cope with water stress remain poorly understood. In the present study, two co-occurring epiphytic orchids with different phenologies were selected to investigate their drought-resistance performances. We compared their functional traits, and monitored their physiological performances in a 25-days of drought treatment. In contrast to the deciduous species Pleione albiflora, the evergreen species Coelogyne corymbosa had different root anatomical structures and higher values for saturated water content of pseudobulbs. Moreover, plants of C. corymbosa had thicker leaves and epidermis, denser veins and stomata, and higher values for leaf mass per unit area and the time required to dry saturated leaves to 70% relative water content. However, samples from that species had lower values for net photosynthetic rate (An), stomatal length and chlorophyll content per unit dry mass. Nevertheless, due to greater capacity for water storage and conservation, C. corymbosa maintained higher An, stomatal conductance (gs), and instantaneous water-use efficiency during severe drought period, and their values for leaf water potential were higher after the water stress treatment. By Day 10 after irrigation was restarted, only C. corymbosa plants recovered their values for An and gs to levels close to those calculated prior to the imposition of water stress. Our results suggest that the different performance responding to drought and re-watering in two co-occurring epiphytic orchids is related to water-related traits and these two species have divergent adaptive mechanisms. Overall, C. corymbosa demonstrates drought avoidance by enhancing water uptake and storage, and by reducing water losses while P. albiflora employs a drought escape strategy by fixing more carbon during growing season and shedding leaves and roots at dry season, leaving a dormant pseudobulb to minimize transpiration. These findings may improve our understanding of the potential effects that climate change can have on the population dynamics of different epiphytic taxa.
Introduction
Vascular epiphytes use other plants as a support for growth but do not parasitize hosts (Benzing, 1990). This taxonomically heterogeneous group, comprising over 27,000 species in 73 families, accounts for approximately 9% of all extant vascular plant species (Zotz, 2013). Vascular epiphytes are most impressive in tropical rainforests, and their abundance and diversity are noticeably decreased outside the tropic zone. Some noteworthy exceptions include temperate rain forests of Chile and New Zealand, or montane forests in the Himalayas, where the epiphyte biomass and diversity are comparable to that of many tropical forests (Zotz, 2005).
Epiphytic plants represent a substantial proportion of the entire biomass in tropical montane cloud forests (Hofstede et al., 1993; Nadkarni et al., 2004) and temperate rain forests (Diaz et al., 2010). Both epiphytes and the humus they generate and hold in the canopy can influence the processes of water/nutrient uptake or release. Therefore this plant group plays a key role in hydrology and nutrient cycling within forest ecosystems (Veneklaas et al., 1990; Hofstede et al., 1993; Nadkarni et al., 2004; Pypker et al., 2006; Diaz et al., 2010). In addition, epiphytes facilitate animal life in tree canopies by providing abundant and diverse resources, e.g., food and shelters (Treseder et al., 1995; Bluthgen et al., 2000; Ellwood et al., 2002).
Although epiphytes are essential components of these ecosystems, this plant group is currently considered more vulnerable to global climate change and anthropogenic disturbances when compared with co-occurring plant types such as supporting trees (Benzing, 1998; Zotz and Bader, 2009; Mondragón et al., 2015). This is probably because arboreal habitats cause epiphytes tightly coupled to atmospheric inputs whereas terrestrial plants are in contact with the soil, from which they can obtain stable supplies of water and nutrient (Benzing, 1998). Among all the abiotic factors, water appears to be one of the greatest limitations to the growth of vascular epiphytes among all the abiotic factors (Laube and Zotz, 2003; Zotz et al., 2010). Furthermore, the diversity and abundance of epiphytic species respond more emphatically to water gradients than any other life forms, including trees, shrubs, and terrestrial herbs (Gentry and Dodson, 1987; Benzing, 1990). The high mortality rate for juvenile epiphytic plants is probably caused by drought or low air humidity (Zotz and Hietz, 2001). Unlike a terrestrial habitat, the supply of moisture in a tree canopy is intermittent rather than continuous. Rainless periods of a few hours may be sufficient to induce drought stress (Zotz and Hietz, 2001). However, even in the wet season, rainless periods of a few days are rather common (Zotz and Tyree, 1996; Engelbrecht et al., 2006). Epiphytic species endure at least moderate drought stress over most of the year (Zotz and Tyree, 1996). Therefore, multiple morphological and physiological adaptations have evolved to cope with the challenges of a fluctuating external water supply. Examples include water-impounding foliage of bromeliads (Zotz and Thomas, 1999), succulent pseudobulbs produced by orchids (Ng and Hew, 2000), and the water-saving crassulacean acid metabolism, or CAM (Zotz and Hietz, 2001; Lüttge, 2004). However, when one considers how the frequency of drought events is increasing in certain regions (IPCC, 2013), along with more variable precipitation patterns (Allan and Soden, 2008) and rapid deforestation/fragmentation in both tropical and temperate forests (Echeverria et al., 2006; Broadbent et al., 2008), one must ask whether these adaptions are enough to support the survival of epiphytes in our changing world.
The answer seems ambiguous. For example, epiphyte species richness is much lower in a secondary forest than in an adjacent primary forest, and the number of species within some taxa declined dramatically while the tallies of others remained flat (Krömer and Gradstein, 2003). Some taxa have benefited from anthropogenic disturbances even if the entire epiphytic species diversity has been dramatically affected (Barthlott et al., 2001; Cascante-Marin et al., 2006; Toledo-Aceves et al., 2012). Epiphytic taxa exhibit divergent patterns of growth, survival, and the establishment of new seedlings on isolated remnant trees after they are exposed to a shift in microclimate due to logging, with mesic taxa tending to be more severely affected and substituted by more xerophytic ones (Padmawathe et al., 2004; Werner and Gradstein, 2008; Werner, 2011). These reports indicate that various epiphytic species differed in their responses to environmental changes, with even slight shifts in their microclimate producing “winners” and “losers” (Zotz and Bader, 2009). However, it is unclear whether these differences in sensitivity are related to the way in which they adapt to water stress.
Three commonly recognized adaptive strategies for a plant to water stress are drought escape, drought avoidance, and drought tolerance (Levitt, 1980; Farooq et al., 2012). Drought escape means that a plant to completes its life cycle and goes dormant before the onset of the dry season. Plants that utilize drought avoidance maintain a high water status by enhancing their water uptake and reducing water losses. By contrast, drought-tolerant plants continue their metabolic activities even at low tissue water potential. The boundaries between types of strategies are not clear-cut because they are not mutually exclusive (Levitt, 1980). Plants with different drought adaptive strategies may coexist within the same habitat, especially in some arid and semi-arid ecosystems (Chaves et al., 2002; Galmés et al., 2007; Hernández et al., 2010).
Although these divergent performances have been widely reported among co-occurring epiphytic taxa (Padmawathe et al., 2004; Werner and Gradstein, 2008; Werner, 2011; Toledo-Aceves et al., 2012), little is known about the physiological and morphological mechanisms behind this phenomenon. With almost 19,000 epiphytic species, the family Orchidaceae alone accounts for 68% of all epiphytes (Zotz, 2013). This family displays a spectacular array of adaptations and rapid speciations with numerous adaptive characteristics (Silvera et al., 2009). For this present study, we compared two co-occurring epiphytic orchids with different phenologies: the evergreen Coelogyne corymbosa and deciduous Pleione albiflora. Their functional traits (including those of the root, pseudobulb, and leaf) were compared with regard to plant water relations, and their physiological performances were monitored over 25 days of drought treatment, followed by 10-days of recovery after re- irrigation. The main aims were to explore their respective adaptive strategies and investigate any possible links with performance in response to drought conditions. Our goal was to improve our understanding of the population dynamics of different types of epiphytes in a changing world so that we can preserve this vulnerable group. We tested three hypotheses:
(1) With thick, evergreen leaves, plants of C. corymbosa employ a drought avoidance strategy while the deciduous P. albiflora escape the effects of drought by shedding its leaves and roots during the dry season.
(2) Plants of C. corymbosa are better able to resist drought while P. albiflora shows weaker capacity for drought-resistance but stronger photosynthetic performance during favorable growing periods.
(3) Because of their enhanced capacity for water conservation, epiphytic species that utilize a drought avoidance strategy will be the “winners” if their local environment becomes drier or an extreme drought event occurs.
Materials and Methods
Plant Materials and Growth Conditions
Although C. corymbosa and P. albiflora are both perennial herbs they have distinct morphologies and phenologies. While the former has two evergreen leaves on each perennial pseudobulb, the latter features only one leaf on its annual renewed pseudobulb. According to their carbon isotope ratios (-28.23% for C. corymbosa and -29.47% for P. albiflora), leaves of both species use C3 photosynthetic CO2 fixation. We did not test the photosynthetic modes of green pseudobulbs in these two species, but CAM photosynthetic pathway may be presented in green pseudobulbs of some orchids (Ng and Hew, 2000). When the growth of P. albiflora begins in the spring, fresh roots are produced from the base of the new shoot at the side of the pseudobulb. The new shoot swells and the old pseudobulb shrinks during the growth of the plant. As winter approaches, the single leaf withers, the old pseudobulb shrivels, and the roots die, leaving the new pseudobulb to survive the seasonal cold and dry conditions (Cribb and Butterfield, 1999). Contrary to the annual renewed nature of pseudobulb and leaf in P. albiflora, Plants of C. corymbosa persist leaves and pseudobulbs for years (even the leaves dropped, the leafless pseudobulbs survive) and lack a dormant period (Cribb and Butterfield, 1999). Plants of C. corymbosa are wide distributed from Nepal to southwest China while those of P. albiflora are relatively limited to western portions of Yunnan Province in southwest China and adjacent northern Myanmar (Chen et al., 2009). The two orchids examined here were found on the trunks of Illicium sp., Lithocarpus sp., and Rhododendron delavayi trees in an evergreen broad-leaved forest in the Xiaoheishan Nature Reserve in Yunnan Province, at the elevation of 2500 m. Samples of each were collected in April 2014, at the end of the dry season, when plants of P. albiflora were still in dormancy (leafless and rootless) and the new shoots of C. corymbosa were also dormant.
The plants were grown in a greenhouse at Kunming Institute of Botany, CAS (1990 m, E102°41′, N25°01′) in baskets (30 cm × 40 cm) containing a mixture of 70% bark (1 cm × 1 cm), 20% moss, and 10% humus. The light intensity was controlled with shade nets to provide a level approximating 40% of full sunlight (800 μmol m-2 s-1). A humidification and ventilation system was used to maintain the ambient temperature at 20 to 30°C and the relative humidity above 60% throughout the growing season. Before the treatment began, plants were watered normally at 3-days intervals. Plants grew in the greenhouse for 3 months, and the current year’s fully expanded leaves from each species were used in our analysis.
Functional Traits of Leaf, Pseudobulb, and Root
Six leaves and newly mature pseudobulbs were sampled from different plants, and immediately weighed to obtain their fresh weight (FW). Leaf area were measured with a leaf area meter (3100, Li-Cor, USA). Afterward, those materials with cutting edges were saturated overnight in distilled water before recording values for saturated weight (SW). The samples were then oven-dried at 80°C for 48 h before determining dry weight (DW). Relative water content (RWC) was calculated as (FW-DW)/(SW-DW) × 100. The saturated water content (SWC) of the pseudobulbs, an indicator of water storage capacity, was computed as (SW-DW)/DW (Stratton et al., 2000). Specific leaf weight was expressed as leaf dry mass per unit area (LMA). Chlorophyll was extracted in 10 mL of N, N-dimethylformamide in volumetric flasks for 48 h under darkness. Absorbance was measured at 647 and 664 nm with a spectrophotometer (UV-2550; Shimadzu, Japan) to calculate total chlorophyll content, following the method of Inskeep and Bloom (1985).
The rate of water loss after excision was monitored from the undamaged, mature leaves that were first saturated overnight to obtain SW values. The leaves were then placed on a lab bench and their cut petioles were sealed with Parafilm. FWs were measured periodically for 10 days on a digital balance before the leaves were oven-dried at 80°C for 48 h to obtain DW. As a threshold for physiological damage, the time needed to dry saturated leaves to 70% RWC (T70) was determined by regressing RWC against the time interval from leaf excision to each measurement of FW (Hao et al., 2010).
For each species, the middle portions of their sampled leaves, pseudobulbs, and maturation zone of roots were first fixed in FAA solution (formalin, acetic acid, ethanol, and distilled water; 10:5:50:35, v:v:v:v) for a month before transverse sections of each organ were made with a cryostat microtome (CM3050S; Leica, Germany). They were then photographed under a light microscope (DM2500; Leica, Germany). Leaf traits of thickness (LT), upper cuticle thickness (UCT), upper epidermal thickness (UET), lower epidermal thickness (LET), and mesophyll thickness (MT) were then measured from those digital photographs with the Image J software (National Institutes of Health, USA).
The adaxial and abaxial epidermises were peeled from the leaves, and images were made under the DM2500 light microscope. Stomatal parameters were measured from those digital photographs with the Image J software. For each leaf, stomata were tallied in six randomly selected fields. Stomatal density (SD) was calculated as the number per unit leaf area. Stomatal length (SL) was measured from 20 stomata selected randomly. For determining vein density (VD), leaves were boiled for 5 min in a 1% NaOH solution and rinsed in distilled water before being photographed. Total vein length was measured by Image J software and VD was calculated as total vein length per area. For each species, six leaves from different plants were used for anatomical observations.
Drought and Re-watering Experiments
During July and August of 2014, 25-days drought and 10-days re-watering experiments were performed. The net photosynthetic rate (An) and maximal photochemical efficiency of Photosystem II (Fv/Fm) of the leaves as well as the water potential of the culture substrate (Ψcs), were measured at 5-days intervals during the drought period and on Days 1, 3, and 10 after normal irrigation resumed. Pre-dawn water potential of leaves (Ψleaf) from each species was measured at the beginning and the end of the drought period. A group of well-watered plants was set as a control to compare the lifespan of leaves.
Values for An and Fv/Fm were obtained with a portable photosynthesis system equipped with a fluorescence chamber (6400-40; Li-Cor, USA). While the photosynthetic measurements were being made, the light level was set at a saturation intensity of 600 μmol m-2 s-1 (based on photosynthetic light response curves, data not shown) and the atmospheric CO2 concentration was kept at 400 μmol mol-1 by a CO2 injector system (6400-01; Li-Cor, USA). Measurements of An were recorded from 09:00 to 11:30, when CO2 uptake was maximal. Values for stomatal conductance (gs) and transpiration rate (E) were obtained simultaneously during the measurements of An. Instantaneous water use efficiency (WUEi) was expressed as An/E. Measurements of Fv/Fm were conducted at night after the leaves had adapted to darkness for at least 3 h. Both Ψcs and Ψleaf were determined with WP4C Dew point PotentiaMeter (Decagon, USA). Five to six leaves from different plants were used in the drought and re-watering experiments.
Data Analysis
Differences in leaf traits between species were assessed by independent t-tests. One-way ANOVA was used to test for significant differences before and after drought stress for both species, with means discriminated using Tukey’s multiple comparison test. The results were accepted as significant at p < 0.05. All statistical analyses were conducted with the SPSS 16.0 program (SPSS Inc., USA).
Results
Root cross-sections are shown in Figures 1A,B. Those of C. corymbosa were covered with a velamen consisting of four layers of dead cells while the epidermis of P. albiflora roots had a single cell layer. Structural cross-section indicated that velamen radicum was present for C. corymbosa but absence for P. albiflora. Roots of the former also exhibited a suberized exodermis.
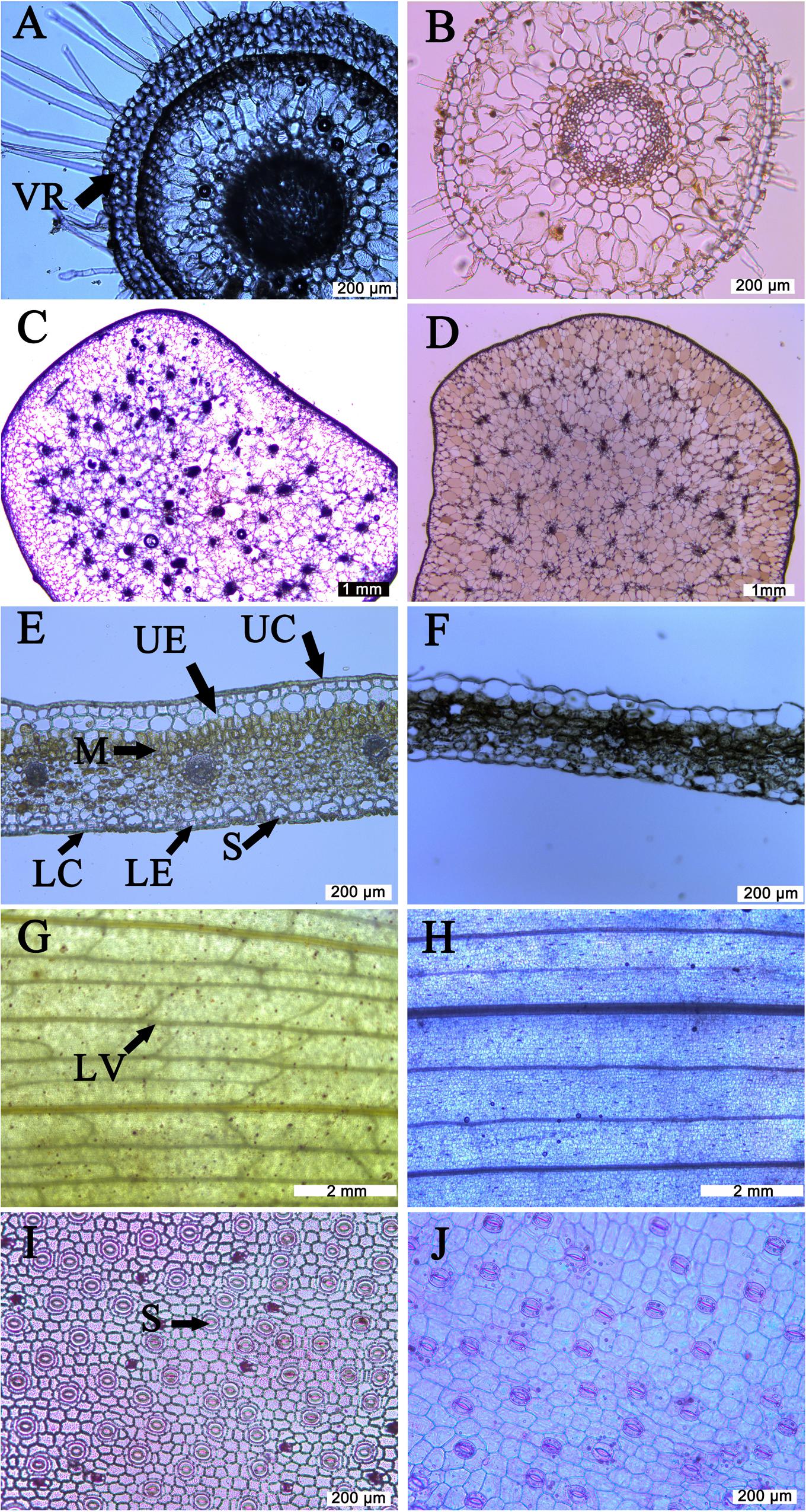
FIGURE 1. Anatomic observation of different organs from Coelogyne corymbosa and Pleione albiflora. (A) Root cross section from C. corymbosa; (B) root cross section from P. albiflora; (C) pseudobulb cross section from C. corymbosa; (D) pseudobulb cross section from P. albiflora; (E) leaf cross section from C. corymbosa; (F) leaf cross section from P. albiflora; (G) leaf veins from C. corymbosa; (H) leaf veins from P. albiflora; (I) lower epidermis from leaf of C. corymbosa; (J) lower epidermis from leaf of P. albiflora. VR, velamen radicum; UC, upper cuticle; UE, upper epidermis; M, mesophyll; LC, lower cuticle; LE, lower epidermis; S, stomata; LV, leaf vein.
The pseudobulbs from both species were anatomically similar, comprising a thick epidermis, multiple parenchyma cells, and vascular bundles (Figures 1C,D). Although there was no significant difference in FW (data not shown), C. corymbosa had a significantly higher SWC of pseudobulb (Table 1), indicating the pseudobulb of the former had a greater capacity for water storage.
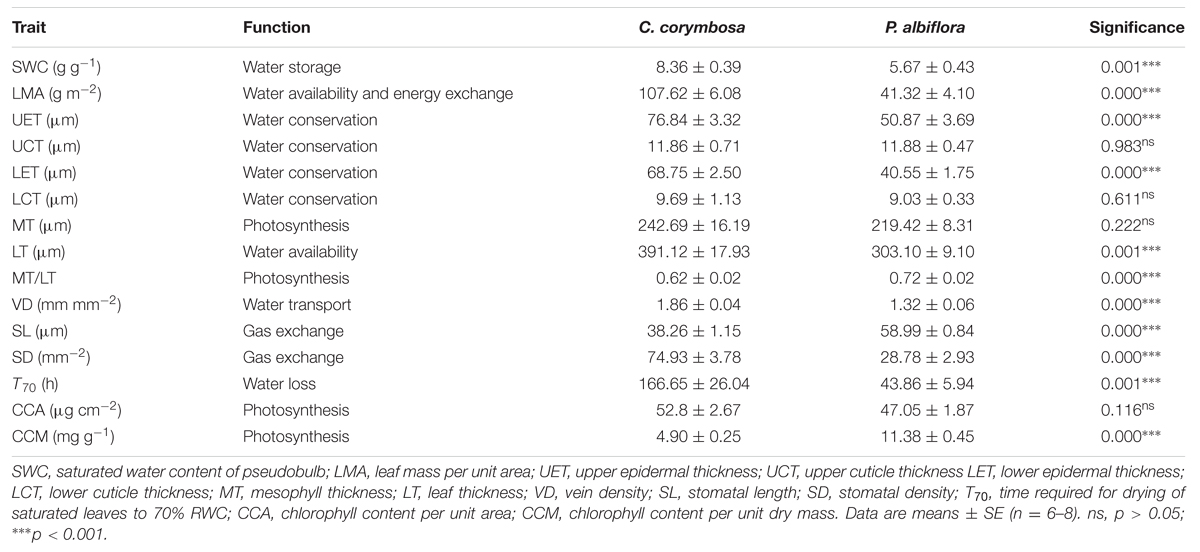
TABLE 1. Functional traits of leaves and pesudobulbs from Coelogyne corymbosa and Pleione albiflora.
Most leaf traits also differed significantly between species. Compared with P. albiflora, leaves of C. corymbosa were thicker and had denser veins as well as denser but smaller stomata (Figures 1E–J; Table 1). These variations were manifested by their higher values for LMA, UET, LET, LT, VD, SD, and T70, but lower values for SL, and CCM (Figure 2; Table 1). However, values calculated for UCT, LCT, MT, and CCA did not differ significantly between species (Table 1). Thus, the thicker leaf of C. corymbosa was mainly caused by its thicker epidermis.
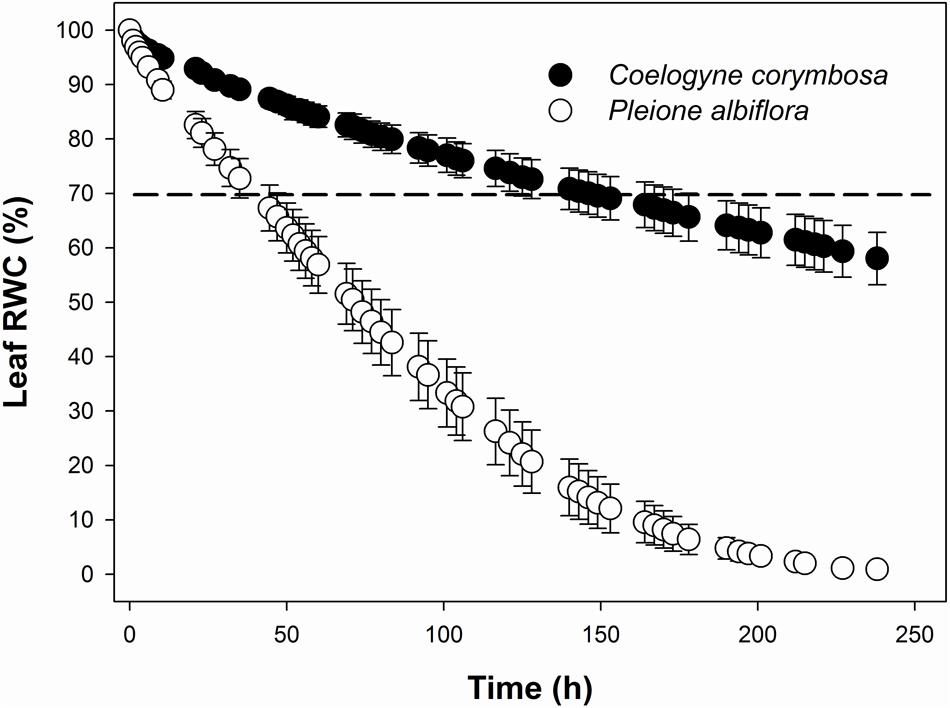
FIGURE 2. Water loss curves for leaves from C. corymbosa and P. albiflora, showing changes in relative water content (RWC) with time after excision. Dashed line indicates time required for drying of saturated leaves to 70% RWC (T70). Data are means ± SE (n = 6).
From the beginning of the drought treatment, Ψcs decreased continuously and dropped dramatically after 10 days of imposed stress. However, those values quickly rebounded to a very high level when the plants were re-watered (Figure 3A). Drought conditions decreased the pre-dawn Ψleaf readings for both species. Although P. albiflora showed comparable Ψleaf to C. corymbosa before irrigation was initially withheld, that species had significantly lower values after 25 days of drought stress (Figure 3B).
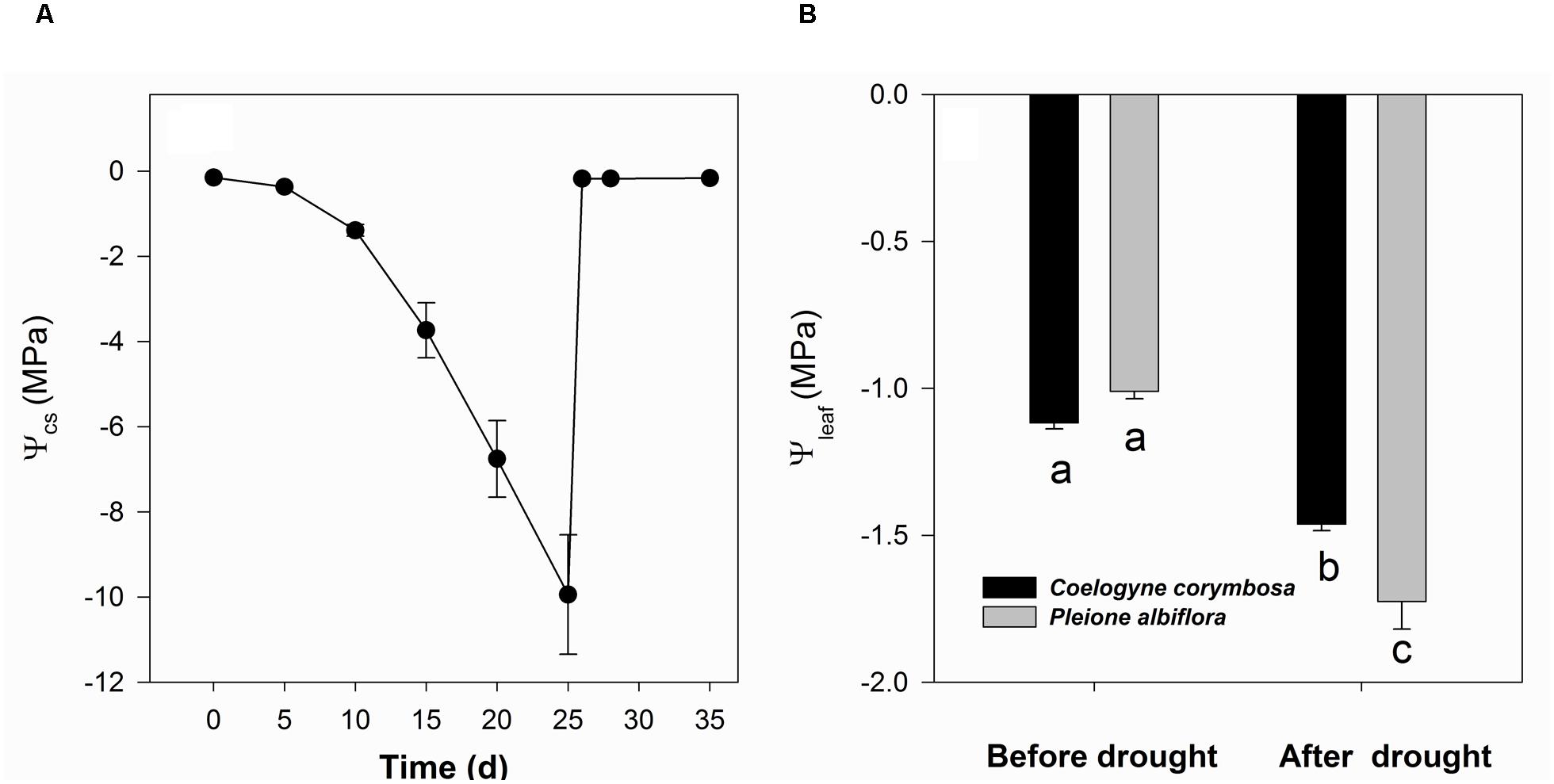
FIGURE 3. Water potential in culture substrate (Ψcs; A) and pre-dawn water potential of leaves (Ψleaf; B) from C. corymbosa and P. albiflora. Different letters indicate significant differences at p < 0.05 between species and treatments. Data are means ± SE (n = 5–6).
Under well-watered conditions, values of An were significantly higher in P. albiflora than in C. corymbosa (Figure 4A). For both species, An and gs decreased as drought stress intensified although those values were significantly higher in C. corymbosa than in P. albiflora after 15 days of drought treatment (Figures 4A,B). The WUEi in P. albiflora was high under well-watered conditions and moderate drought (i.e., Days 5 and 10 after irrigation was stopped), but was decreased dramatically under severe drought (after 15 days of treatment). By contrast, C. corymbosa showed relatively higher WUEi under severe drought (Figure 4C). After 25 days of treatments, Fv/Fm values were significantly decreased in both species. However, all Fv/Fm values were relatively stable (close to 0.8) under drought stress (Figure 4D). When the plants were re-watered, values of An, gs, WUEi, and Fv/Fm increased in both species. After 10 days of recovery, levels of these photosynthesis representing parameters in C. corymbosa were nearly as high as those measured before the drought treatment. However, P. albiflora were still significantly lower than those calculated prior to the imposition of drought. Meanwhile, when compared with leaves from well-watered plants, 25 days of water stress significantly decreased the lifespan of leaves in P. albiflora (121 ± 3.7 days vs. 160 ± 2.8 days; n = 6).
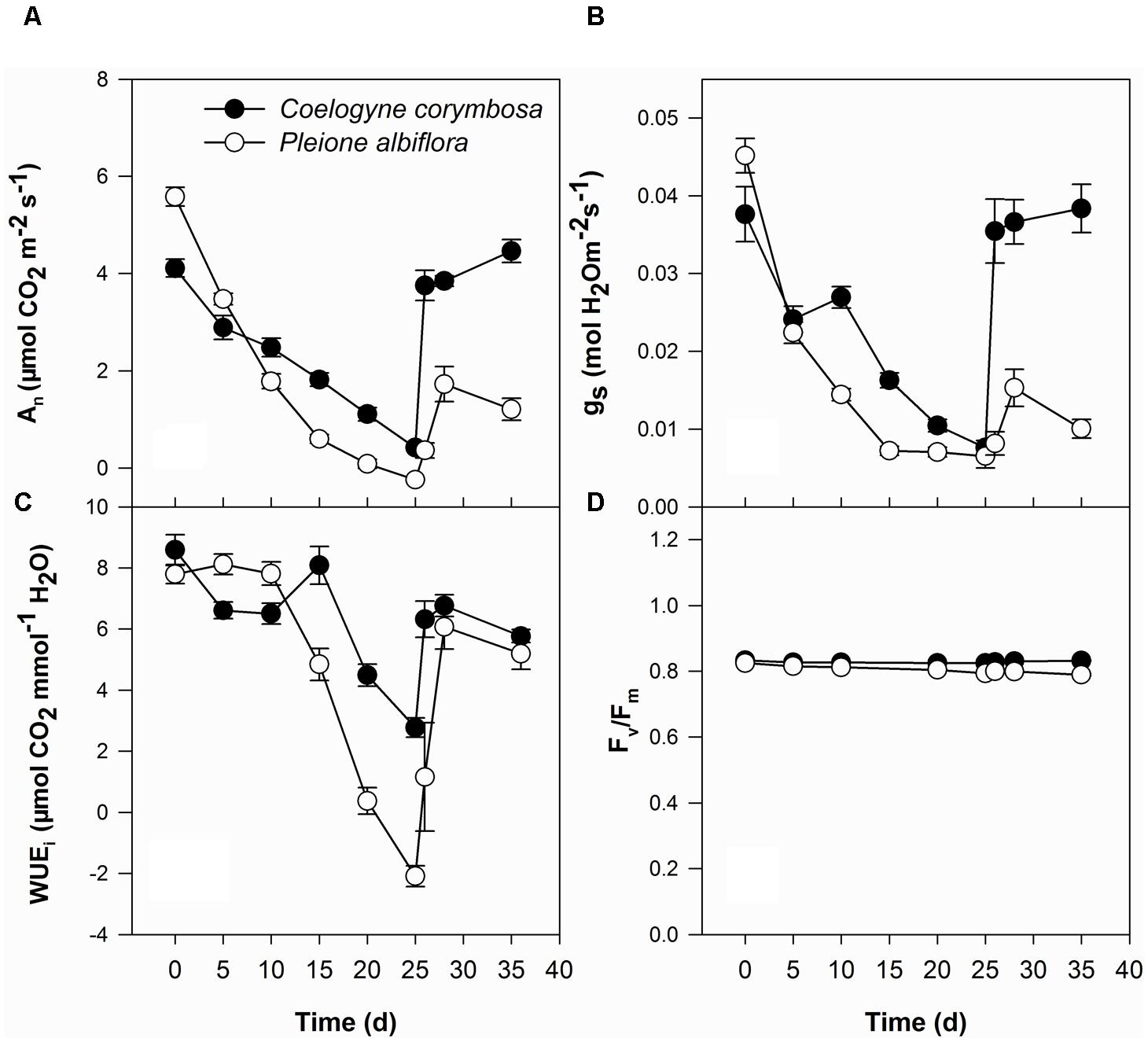
FIGURE 4. Net photosynthetic rate (An; A), stomatal conductance (gs; B), instantaneous water use efficiency (WUEi; C), and maximal photochemical efficiency of photosystem II (Fv/Fm; D) for C. corymbosa and P. albiflora under drought treatment (Days 0–25) and after re-watering (Days 26–35). Data are means ± SE (n = 6).
Discussion
Our study revealed that these two co-occurring epiphytic orchids differ significantly in morphological and physiological traits related to the uptake, storage, transport, and loss of water. Furthermore, the photosynthetic performance during drought treatment and recovery differed significantly between them. These results indicate that the two species have divergent adaptive strategies for coping with drought stress.
Velamen radicum and suberized exodermis were present in C. corymbosa but absence in P. albiflora. The velamen radicum is an important adaptive structure for orchids in epiphytic habitats because it allows for rapid water-absorption under wet conditions but also provides a barrier to transpirational water loss from the internal cells of the roots when the environment is dry (Benzing et al., 1982; Zotz and Winkler, 2013). Moreover, the presence of suberized cell layers at the periphery of the roots plays an important role in preventing water loss under condition of extreme drought (Hose et al., 2001). A thicker velamen radicum and suberized exodermis have also been observed in epiphytic orchids that grow in drier environment (Moreira et al., 2013). Therefore the presence of such structures in C. corymbosa may facilitate the maintenance of a suitable water balance for the entire plant, especially in dry seasons. By contrast, the lack of such structures in P. albiflora is connected with the phenology of this species, which precisely fits the timing of the Asian monsoon, i.e., producing roots at the beginning of the wet season and shedding them as the dry season approaches (Cribb and Butterfield, 1999). This phenomenon is also observed in species in other arid or semi-arid areas, such as the desert succulent Agave deserti (Hunt and Nobel, 1987). On account of the fact that hydraulics of root systems are more sensitive to drought than the leaves (Domec et al., 2009), and a few days without rainfall can be rather common even in the wet season (Zotz and Tyree, 1996; Engelbrecht et al., 2006), by not having to support a velamen radicum and suberized exodermis, plants of P. albiflora avoid over-investment in disposable roots as well as risk of dysfunction within the root hydraulic system that result from unexpected droughty periods occurring even during wet season in epiphytic habitats.
As an organ that stores water, carbohydrates and minerals, the pseudobulbs are of central importance to the growth and survival of epiphytic orchids (Ng and Hew, 2000). Similar to pseudobulbs in other orchids (Ng and Hew, 2000), those of our test species featured an impervious epidermis and multiple water-storing cells. However, those sampled pseudobulbs from C. corymbosa had significantly higher SWC values, indicating that this species has greater capacity to store water (Stratton et al., 2000). In addition, compared to the annual renewed pseudobulbs of P. albiflora, the pseudobulbs of C. corymbosa survive much longer than needed to support its foliage. In fact, in some epiphytic orchid species the pseudobulbs can survive as long as 8 years after their leaves dropped (Zotz, 1998). These old pseudobulbs can significantly increase the water storage capacity of a plant, thereby playing an essential role in helping species survive in prolonged periods of drought in epiphytic habitats, where availability of water is often severely limited (Zotz and Tyree, 1996; Zotz, 1999).
Leaf morphological traits play an important role in maintaining water balances in epiphytes (Zhang et al., 2015). Although phylogenetically related (Gravendeel et al., 2001) and sharing the same habitat, the two orchids examined here have contrasting leaf phenologies, leaf morphological traits, and leaf physiologies. The leaf lifespan of P. albiflora is 5–6 months under well-water conditions while plants of C. corymbosa can sustain their leaves for more than 4 years. These two species exhibit different trade-offs between CO2 acquisition and water loss. C. corymbosa invests more resources in the functions of water transport and reduction of water losses by constructing a thicker leaf epidermis and denser veins, whereas P. albiflora tends to fix more carbon during short, favorable growing seasons so that higher An and gs values were found in P. albiflora under well-watered condition.
Leaf venation facilitates mechanical support, carbohydrate transport, and replacement of water lost via transpiration (Niklas, 1999; Roth-Nebelsick et al., 2001; Sack and Frole, 2006). The particular density of those veins can serve as a proxy for climate or habitat (Uhl and Mosbrugger, 1999; Zhang et al., 2015). Drought stress often leads to higher VD (Uhl and Mosbrugger, 1999; Dunbar-Co et al., 2009), which in turn influences the degree of plant drought resistance (Scoffoni et al., 2011). Therefore, the denser veins associated with C. corymbosa may help the evergreen leaves to survive the harsh dry season.
Although leaf cuticle is critical for protecting plants against water losses, its thickness is not always correlated to the cuticular water permeability (Riederer and Schreiber, 2001). Because leaf cuticle is more efficient in evergreen epiphytes than in deciduous epiphytes for preventing water losses after stomatal closure (Helbsing et al., 2000), we might speculate that other physical properties of the cuticle and the thickness of epidermis, rather than cuticle thickness, are responsible for the different T70 values for these two species.
Smaller and denser stomata enable plants to respond more quickly to environmental changes or to the decrease in leaf water potential, and they also promote greater diffusive conductance under favorable conditions (Aasamaa et al., 2001; Drake et al., 2013). These stomatal attributes are essential for the survival of C. corymbosa under conditions of fluctuating water availability. Although, by comparison, the large stomata of P. albiflora are slower to close and less able to prevent a hydraulic dysfunction in dry conditions, this lag in response may be advantageous in cool, moist, or shaded environments (Aasamaa et al., 2001; Hodgson et al., 2010) during the wet season.
Plants of C. corymbosa invested vast resources toward traits of different organs related to water supply and maintenance. These different strategies for resource investment affect their physiological performances under drought stress. More drought-resistant species control their stomatal functioning to allow for some carbon fixation at water stress, thus improving their WUEi (Yordanov et al., 2000). Our study results suggest that the higher water storage capacity by the pseudobulbs of C. corymbosa facilitates this process, such that the leaf maintains a relatively higher water potential even when the water potential of the culture substrate becomes quite low after 25 days of imposed drought. The performances of these two orchids under stress are similar to those reported for co-occurring Mediterranean woody species. That is, plants with thin, high assimilation rate leaves are more vulnerable to drought while evergreen species, with thicker leaves and low assimilation rates, had the longest drought survival time (Lopez-Iglesias et al., 2014).
The genera Pleione and Coelogyne belong to the monophyletic subtribe Coelogyninae, and the deciduous Pleione diverged early to one of the three major clades (Gravendeel et al., 2001). In addition to their coexistence described here, other Pleione species have been recorded as co-existing with evergreen members in subtribe Coelogyninae, especially Coelogyne species (Cribb and Butterfield, 1999). Therefore, we speculate that a hydrological niche segregation (Silvertown et al., 2015) occurs between these two genera. The most common way for coexisting plants to achieve this type of segregation is by employing different strategies of water acquisition, such as through contrasts in their phenologies or rooting depths (Silvertown et al., 2015). For example, in seasonal tropical dry forests, evergreen trees can access soil water at greater depths than coexisting deciduous species during the dry season (Meinzer et al., 1999; Hasselquist et al., 2010). Because deep soil water is not available within an epiphytic habitat, epiphytes can survive the dry season only by using their stored water or by a very efficient water uptake during sporadic rainfall events (Zotz and Tyree, 1996). Previous researches in tropical ecosystems have predicted that increased drought would favor an increase in deciduous trees and a reduction in evergreen trees (Enquist and Enquist, 2011; Fauset et al., 2012). But these studies focused on annual precipitation rather than precipitation frequency, while the former is of lower relevance for epiphytic plants (Zotz and Bader, 2009). In contrast, our results suggest that evergreen epiphytes are better able to cope with drought events in the growing season. Thus, a change in precipitation frequency would have a larger impact on deciduous epiphytes than evergreen ones.
Zotz and Winter (1994) have shown that the annual carbon gain of three co-occurring epiphytic species is similar regardless their leaf phenology (evergreen vs. deciduous) or photosynthetic pathway (C3 vs. CAM). This indicates that the strategies of stress escape or stress avoidance in an epiphytic habitat can be equally beneficial in terms of annual leaf carbon gain. We found that 25-days of stress decreased the lifespan of leaves in P. albiflora, which inevitably led to the decrease of annual leaf carbon gain in this species. Therefore, the “winners” and “losers” of epiphytes after microclimate shifts (Padmawathe et al., 2004; Werner and Gradstein, 2008; Werner, 2011) probably reflect the different adaptive strategies utilized by epiphytic taxa in response to environmental disturbances.
Conclusion
The two species investigated here differ significantly in their water-related traits, with plants of C. corymbosa having higher capacity for water uptake and storage and greater ability to reduce water losses. By comparison, plants of P. albiflora have higher photosynthetic capacity under well-watered conditions. The different photosynthetic performance responding to drought and re-watering in these two co-occurring epiphytic orchids is related to water-related traits. The divergence in morphology and physiology reflects fundamentally different adaptive strategies in the same epiphytic habitat. The drought escape plants are more vulnerable to the fluctuating water availability in an epiphytic habitat in the global climate change and anthropogenic disturbances. Therefore, more attention should be focused on such species in the conservation of epiphyte diversity in the changing world.
Author Contributions
S-BZ and HH designed experiments. WZ carried out experiments and analyzed experimental results. WZ and S-BZ wrote the manuscript.
Conflict of Interest Statement
The authors declare that the research was conducted in the absence of any commercial or financial relationships that could be construed as a potential conflict of interest.
Acknowledgment
This work is financially supported by the National Natural Science Foundation of China (31170315, 31370362, 31400289) and the Natural Science Foundation of Yunnan Province (2013FA044).
References
Aasamaa, K., Sober, A., and Rahi, M. (2001). Leaf anatomical characteristics associated with shoot hydraulic conductance, stomatal conductance and stomatal sensitivity to changes of leaf water status in temperate deciduous trees. Aust. J. Plant Physiol. 28, 765–774. doi: 10.1071/PP00157
Allan, R. P., and Soden, B. J. (2008). Atmospheric warming and the amplification of precipitation extremes. Science 321, 1481–1484. doi: 10.1126/science.1160787
Barthlott, W., Schmit-Neuerburg, V., Nieder, J., and Engwald, S. (2001). Diversity and abundance of vascular epiphytes: a comparison of secondary vegetation and primary montane rain forest in the Venezuelan Andes. Plant Ecol. 152, 145–156. doi: 10.1023/A:1011483901452
Benzing, D. H. (1990). Vascular Epiphytes. General Biology and Related Biota. Cambridge: Cambridge University Press. doi: 10.1017/CBO9780511525438
Benzing, D. H. (1998). Vulnerabilities of tropical forests to climate change: the significance of resident epiphytes. Clim. Change 39, 519–540. doi: 10.1023/A:1005312307709
Benzing, D. H., Ott, D. W., and Friedman, W. E. (1982). Roots of Sobralia macrantha (Orchidaceae): structure and function of the velamen-exodermis complex. Am. J. Bot. 69, 608–614. doi: 10.2307/2443070
Bluthgen, N., Verhaagh, M., Goitia, W., Jaffe, K., Morawetz, W., and Barthlott, W. (2000). How plants shape the ant community in the Amazonian rainforest canopy: the key role of extrafloral nectaries and homopteran honeydew. Oecologia 125, 229–240. doi: 10.1007/s004420000449
Broadbent, E. N., Asner, G. P., Keller, M., Knapp, D. E., Oliveira, P. J. C., and Silva, J. N. (2008). Forest fragmentation and edge effects from deforestation and selective logging in the Brazilian Amazon. Biol. Conserv. 141, 1745–1757. doi: 10.1016/j.biocon.2008.04.024
Cascante-Marin, A., Wolf, J. H. D., Oostermeijer, J. G. B., den Nijs, J. C. M., Sanahuja, O., and Duran-Apuy, A. (2006). Epiphytic bromeliad communities in secondary and mature forest in a tropical premontane area. Basic Appl. Ecol. 7, 520–532. doi: 10.1016/j.baae.2005.10.005
Chaves, M. M., Pereira, J. S., Maroco, J., Rodrigues, M. L., Ricardo, C. P. P., Osorio, M. L., et al. (2002). How plants cope with water stress in the field. Photosynthesis and growth. Ann. Bot. 89, 907–916. doi: 10.1093/aob/mcf105
Chen, X. Q., Liu, Z. J., Zhu, G. H., Lang, K. Y., Ji, Z. H., Luo, Y. B., et al. (2009). “Orchidaceae,” in Flora of China, eds Z. Y. Wu, P. H. Raven, and D. Y. Hong (St. Louis, MO: Missouri Botanical Garden Press).
Diaz, I. A., Sieving, K. E., Pena-Foxon, M. E., Larrain, J., and Armesto, J. J. (2010). Epiphyte diversity and biomass loads of canopy emergent trees in Chilean temperate rain forests: a neglected functional component. For. Ecol. Manage. 259, 1490–1501. doi: 10.1016/j.foreco.2010.01.025
Domec, J. C., Noormets, A., King, J. S., Sun, G., McNulty, S. G., Gavazzi, M. J., et al. (2009). Decoupling the influence of leaf and root hydraulic conductances on stomatal conductance and its sensitivity to vapour pressure deficit as soil dries in a drained loblolly pine plantation. Plant Cell Environ. 32, 980–991. doi: 10.1111/j.1365-3040.2009.01981.x
Drake, P. L., Froend, R. H., and Franks, P. J. (2013). Smaller, faster stomata: scaling of stomatal size, rate of response, and stomatal conductance. J. Exp. Bot. 64, 495–505. doi: 10.1093/jxb/ers347
Dunbar-Co, S., Sporck, M. J., and Sack, L. (2009). Leaf trait diversification and design in seven rare taxa of the Hawaiian Plantago radiation. Int. J. Plant Sci. 170, 61–75. doi: 10.1086/593111
Echeverria, C., Coomes, D., Salas, J., Rey-Benayas, J. M., Lara, A., and Newton, A. (2006). Rapid deforestation and fragmentation of Chilean Temperate Forests. Biol. Conserv. 130, 481–494. doi: 10.1016/j.biocon.2006.01.017
Ellwood, M. D. F., Jones, D. T., and Foster, W. A. (2002). Canopy ferns in lowland dipterocarp forest support a prolific abundance of ants, termites, and other invertebrates. Biotropica 34, 575–583. doi: 10.1111/j.1744-7429.2002.tb00576.x
Engelbrecht, B. M. J., Dalling, J. W., Pearson, T. R. H., Wolf, R. L., Galvez, D. A., Koehler, T., et al. (2006). Short dry spells in the wet season increase mortality of tropical pioneer seedlings. Oecologia 148, 258–269. doi: 10.1007/s00442-006-0368-5
Enquist, B. J., and Enquist, C. A. F. (2011). Long-term change within a Neotropical forest: assessing differential functional and floristic responses to disturbance and drought. Glob. Change Biol. 17, 1408–1424. doi: 10.1111/j.1365-2486.2010.02326.x
Farooq, M., Hussain, M., Wahid, A., and Siddique, K. (2012). “Drought stress in plants: an overview,” in Plant Responses to Drought Stress, ed. R. Aroca (Heidelberg: Springer-Verlag), 1–33. doi: 10.1007/978-3-642-32653-0_1
Fauset, S., Baker, T. R., Lewis, S. L., Feldpausch, T. R., Affum-Baffoe, K., Foli, E. G., et al. (2012). Drought-induced shifts in the floristic and functional composition of tropical forests in Ghana. Ecol. Lett. 15, 1120–1129. doi: 10.1111/j.1461-0248.2012.01834.x
Galmés, J., Flexas, J., Savé, R., and Medrano, H. (2007). Water relations and stomatal characteristics of Mediterranean plants with different growth forms and leaf habits: responses to water stress and recovery. Plant Soil 290, 139–155. doi: 10.1007/s11104-006-9148-6
Gentry, A. H., and Dodson, C. H. (1987). Diversity and biogeography of Neotropical vascular epiphytes. Ann. Mo. Bot. Gdns. 74, 205–233. doi: 10.2307/2399395
Gravendeel, B., Chase, M. W., De Vogel, E. F., Roos, M. C., Mes, T. H. M., and Bachmann, K. (2001). Molecular phylogeny of Coelogyne (Epidendroideae; Orchidaceae) based on plastid RFLPS, matK, and nuclear ribosomal its sequences: evidence for polyphyly. Am. J. Bot. 88, 1915–1927. doi: 10.2307/3558367
Hao, G. Y., Sack, L., Wang, A. Y., Cao, K. F., and Goldstein, G. (2010). Differentiation of leaf water flux and drought tolerance traits in hemiepiphytic and non-hemiepiphytic Ficus tree species. Funct. Ecol. 24, 731–740. doi: 10.1111/j.1365-2435.2010.01724.x
Hasselquist, N. J., Allen, M. F., and Santiago, L. S. (2010). Water relations of evergreen and drought-deciduous trees along a seasonally dry tropical forest chronosequence. Oecologia 164, 881–890. doi: 10.1007/s00442-010-1725-y
Helbsing, S., Riederer, M., and Zotz, G. (2000). Cuticles of vascular epiphytes:efficient barriers for water loss after stomatal closure? Ann. Bot. 86, 765–769. doi: 10.1006/anbo.2000.1239
Hernández, E. I., Vilagrosa, A., Pausas, J. G., and Bellot, J. (2010). Morphological traits and water use strategies in seedlings of Mediterranean coexisting species. Plant Ecol. 207, 233–244. doi: 10.1007/s11258-009-9668-2
Hodgson, J. G., Sharafi, M., Jalili, A., Diaz, S., Montserrat-Marti, G., Palmer, C., et al. (2010). Stomatal vs. genome size in angiosperms: the somatic tail wagging the genomic dog? Ann. Bot. 105, 573–584. doi: 10.1093/aob/mcq011
Hofstede, R. G. M., Wolf, J. H. D., and Benzing, D. H. (1993). Epiphytic biomassand nutrient status of a Colombian upper Montane rain forest. Selbyana 14, 37–45.
Hose, E., Clarkson, D. T., Steudle, E., Schreiber, L., and Hartung, W. (2001). The exodermis: a variable apoplastic barrier. J. Exp. Bot. 52, 2245–2264. doi: 10.1093/jexbot/52.365.2245
Hunt, E. R., and Nobel, P. S. (1987). Allometric root shoot relationships and predicted water-uptake for desert succulents. Ann. Bot. 59, 571–577.
Inskeep, W. P., and Bloom, P. R. (1985). Extinction coefficients of chlorophyll a and b in N,N-dimethylformamide and 80% acetone. Plant Physiol. 77, 483–485. doi: 10.1104/Pp.77.2.483
IPCC (2013). Climate Change 2013: The Physical Science Basis. Cambridge: Cambridge University Press.
Krömer, T., and Gradstein, S. R. (2003). Species richness of vascular epiphytes in two primary forests and fallows in the Bolivian Andes. Selbyana 24, 190–195.
Laube, S., and Zotz, G. (2003). Which abiotic factors limit vegetative growth in a vascular epiphyte? Funct. Ecol. 17, 598–604. doi: 10.1046/j.1365-2435.2003.00760.x
Levitt, J. (1980). Responses of Plants to Environmental Stresses. Water, Radiation, Salt, and Other Stresses, Vol. II. New York, NY: Academic Press.
Lopez-Iglesias, B., Villar, R., and Poorter, L. (2014). Functional traits predict drought performance and distribution of Mediterranean woody species. Acta Oecol. 56, 10–18. doi: 10.1016/j.actao.2014.01.003
Lüttge, U. (2004). Ecophysiology of crassulacean acid metabolism (CAM). Ann. Bot. 93, 629–652. doi: 10.1093/aob/mch087
Meinzer, F. C., Andrade, J. L., Goldstein, G., Holbrook, N. M., Cavelier, J., and Wright, S. J. (1999). Partitioning of soil water among canopy trees in a seasonally dry tropical forest. Oecologia 121, 293–301. doi: 10.1007/s004420050931
Mondragón, D., Valverde, T., and Hernández-Apolinar, M. (2015). Population ecology of epiphytic angiosperms: a review. Trop. Ecol. 56, 1–39. doi: 10.13140/2.1.4043.5849
Moreira, A. S. F. P., de Lemos, J. P., and Isaias, R. M. D. (2013). Structural adaptations of two sympatric epiphytic orchids (Orchidaceae) to a cloudy forest environment in rocky outcrops of Southeast Brazil. Rev. Biol. Trop. 61, 1053–1065.
Nadkarni, N. M., Schaefer, D., Matelson, T. J., and Solano, R. (2004). Biomass and nutrient pools of canopy and terrestrial components in a primary and a secondary montane cloud forest, Costa Rica. For. Ecol. Manage. 198, 223–236. doi: 10.1016/j.foreco.2004.04.011
Ng, C. K. Y., and Hew, C. S. (2000). Orchid pseudobulbs - ‘false’ bulbs with a genuine importance in orchid growth and survival! Sci. Hortic. 83, 165–172. doi: 10.1016/S0304-4238(99)00084-9
Niklas, K. J. (1999). A mechanical perspective on foliage leaf form and function. New Phytol. 143, 19–31. doi: 10.1046/j.1469-8137.1999.00441.x
Padmawathe, R., Qureshi, Q., and Rawat, G. S. (2004). Effects of selective logging on vascular epiphyte diversity in a moist lowland forest of Eastern Himalaya, India. Biol. Conserv. 119, 81–92. doi: 10.1016/j.biocon.2003.10.024
Pypker, T. G., Unsworth, M. H., and Bond, B. J. (2006). The role of epiphytes in rainfall interception by forests in the Pacific Northwest. II. Field measurements at the branch and canopy scale. Can. J. For. Res. 36, 819–832. doi: 10.1139/X05-286
Riederer, M., and Schreiber, L. (2001). Protecting against water loss: analysis of the barrier properties of plant cuticles. J. Exp. Bot. 52, 2023–2032. doi: 10.1093/jexbot/52.363.2023
Roth-Nebelsick, A., Uhl, D., Mosbrugger, V., and Kerp, H. (2001). Evolution and function of leaf venation architecture: a review. Ann. Bot. 87, 553–566. doi: 10.1006/anbo.2001.1391
Sack, L., and Frole, K. (2006). Leaf structural diversity is related to hydraulic capacity in tropical rain forest trees. Ecology 87, 483–491. doi: 10.1890/05-0710
Scoffoni, C., Rawls, M., McKown, A., Cochard, H., and Sack, L. (2011). Decline of leaf hydraulic conductance with dehydration: relationship to leaf size and venation architecture. Plant Physiol. 156, 832–843. doi: 10.1104/pp.111.173856
Silvera, K., Santiago, L. S., Cushman, J. C., and Winter, K. (2009). Crassulacean acid metabolism and epiphytism linked to adaptive radiations in the Orchidaceae. Plant Physiol. 149, 1838–1847. doi: 10.1104/pp.108.132555
Silvertown, J., Araya, Y., and Gowing, D. (2015). Hydrological niches in terrestrial plant communities: a review. J. Ecol. 103, 93–108. doi: 10.1111/1365-2745.12332
Stratton, L., Goldstein, G., and Meinzer, F. C. (2000). Stem water storage capacity and efficiency of water transport: their functional significance in a Hawaiian dry forest. Plant Cell Environ. 23, 99–106. doi: 10.1046/j.1365-3040.2000.00533.x
Toledo-Aceves, T., Garcia-Franco, J. G., Hernandez-Rojas, A., and MacMillan, K. (2012). Recolonization of vascular epiphytes in a shaded coffee agroecosystem. Appl. Veg. Sci. 15, 99–107. doi: 10.1111/j.1654-109X.2011.01140.x
Treseder, K. K., Davidson, D. W., and Ehleringer, J. R. (1995). Absorption of ant-provided carbon dioxide and nitrogen by a tropical epiphyte. Nature 375, 137–139. doi: 10.1038/375137a0
Uhl, D., and Mosbrugger, V. (1999). Leaf venation density as a climate and environmental proxy: a critical review and new data. Palaeogeogr. Palaeoclimatol. Palaeoecol. 149, 15–26. doi: 10.1016/S0031-0182(98)00189-8
Veneklaas, E. J., Zagt, R. J., Vanleerdam, A., Vanek, R., Broekhoven, A. J., and Vangenderen, M. (1990). Hydrological properties of the epiphyte mass of a montane tropical rain forest, Colombia. Vegetatio 89, 183–192. doi: 10.1007/Bf00032170
Werner, F. A. (2011). Reduced growth and survival of vascular epiphytes on isolated remnant trees in a recent tropical montane forest clear-cut. Basic Appl. Ecol. 12, 172–181. doi: 10.1016/j.baae.2010.11.002
Werner, F. A., and Gradstein, S. R. (2008). Seedling establishment of vascular epiphytes on isolated and enclosed forest trees in an Andean landscape, Ecuador. Biodivers. Conserv. 17, 3195–3207. doi: 10.1007/s10531-008-9421-5
Yordanov, I., Velikova, V., and Tsonev, T. (2000). Plant responses to drought, acclimation, and stress tolerance. Photosynthetica 38, 171–186. doi: 10.1023/a:1007201411474
Zhang, S. B., Dai, Y., Hao, G. Y., Li, J. W., Fu, X. W., and Zhang, J. L. (2015). Differentiation of water-related traits in terrestrial and epiphytic Cymbidium species. Front. Plant Sci. 6:260. doi: 10.3389/fpls.2015.00260
Zotz, G. (1998). Demography of the epiphytic orchid, Dimerandra emarginata. J. Trop. Ecol. 14, 725–741. doi: 10.1017/S0266467498000534
Zotz, G. (1999). What are backshoots good for? Seasonal changes in mineral, carbohydrate and water content of different organs of the epiphytic orchid, Dimerandra emarginata. Ann. Bot. 84, 791–798. doi: 10.1006/anbo.1999.0983
Zotz, G. (2005). Vascular epiphytes in the temperate zones – a review. Plant Ecol. 176, 173–183. doi: 10.1007/s11258-004-0066-5
Zotz, G. (2013). The systematic distribution of vascular epiphytes a critical update. Bot. J. Linn. Soc. 171, 453–481. doi: 10.1111/boj.12010
Zotz, G., and Bader, M. Y. (2009). “Epiphytic plants in a changing world-global: change effects on vascular and non-vascular epiphytes,” in Progress in Botany, eds U. Lüttge, W. Beyschlag, B. Budel, and D. Francis (Heidelberger: Springer-Verlag), 147–170.
Zotz, G., Bogusch, W., Hietz, P., and Ketteler, N. (2010). Growth of epiphytic bromeliads in a changing world: the effects of CO2, water and nutrient supply. Acta Oecol. 36, 659–665. doi: 10.1016/j.actao.2010.10.003
Zotz, G., and Hietz, P. (2001). The physiological ecology of vascular epiphytes: current knowledge, open questions. J. Exp. Bot. 52, 2067–2078. doi: 10.1093/jexbot/52.364.2067
Zotz, G., and Thomas, V. (1999). How much water is in the tank? Model calculations for two epiphytic bromeliads. Ann. Bot. 83, 183–192. doi: 10.1006/anbo.1998.0809
Zotz, G., and Tyree, M. T. (1996). Water stress in the epiphytic orchid, Dimerandra emarginata (G. Meyer) Hoehne. Oecologia 107, 151–159. doi: 10.1007/Bf00327898
Zotz, G., and Winkler, U. (2013). Aerial roots of epiphytic orchids: the velamen radicum and its role in water and nutrient uptake. Oecologia 171, 733–741. doi: 10.1007/s00442-012-2575-6
Keywords: epiphyte, drought, coexistence, adaptive strategy, water relations, photosynthesis, leaf phenology, functional trait
Citation: Zhang W, Hu H and Zhang S-B (2016) Divergent Adaptive Strategies by Two Co-occurring Epiphytic Orchids to Water Stress: Escape or Avoidance?. Front. Plant Sci. 7:588. doi: 10.3389/fpls.2016.00588
Received: 12 January 2016; Accepted: 18 April 2016;
Published: 03 May 2016.
Edited by:
Boris Rewald, University of Natural Resources and Life Sciences, Vienna, AustriaReviewed by:
Jie He, Nanyang Technological University, SingaporeMarisa Santos, Federal University of Santa Catarina, Brazil
Copyright © 2016 Zhang, Hu and Zhang. This is an open-access article distributed under the terms of the Creative Commons Attribution License (CC BY). The use, distribution or reproduction in other forums is permitted, provided the original author(s) or licensor are credited and that the original publication in this journal is cited, in accordance with accepted academic practice. No use, distribution or reproduction is permitted which does not comply with these terms.
*Correspondence: Shi-Bao Zhang, c2J6aGFuZ0BtYWlsLmtpYi5hYy5jbg==