- Institut Sophia Agrobiotech, UMR INRA Université Nice Sophia Antipolis CNRS, Sophia Antipolis, France
The symbiotic interaction between legumes and nitrogen-fixing rhizobium bacteria leads to the formation of a new organ, the nodule. Early steps of the interaction are characterized by the production of bacterial Nod factors, the reorientation of root-hair tip growth, the formation of an infection thread (IT) in the root hair, and the induction of cell division in inner cortical cells of the root, leading to a nodule primordium formation. Reactive oxygen species (ROS) and nitric oxide (NO) have been detected in early steps of the interaction. ROS/NO are determinant signals to arbitrate the specificity of this mutualistic association and modifications in their content impair the development of the symbiotic association. The decrease of ROS level prevents root hair curling and ITs formation, and that of NO conducts to delayed nodule formation. In root hairs, NADPH oxidases were shown to produce ROS which could be involved in the hair tip growth process. The use of enzyme inhibitors suggests that nitrate reductase and NO synthase-like enzymes are the main route for NO production during the early steps of the interaction. Transcriptomic analyses point to the involvement of ROS and NO in the success of the infection process, the induction of early nodulin gene expression, and the repression of plant defense, thereby favoring the establishment of the symbiosis. The occurrence of an interplay between ROS and NO was further supported by the finding of both S-sulfenylated and S-nitrosylated proteins during early symbiotic interaction, linking ROS/NO production to a redox-based regulation of the symbiotic process.
Introduction
Symbiosis describes a situation in which two or more organisms belonging to different species live together for an extended period of time (De Bary, 1879). Both partners can influence the fate of symbiosis, from the host side by the intensity of the immune response, and from the symbiont side by the degree of biological perturbations inflicted on the host (Moné et al., 2014). An important factor for the evolution of symbiosis is the control of redox environment (Hentschel et al., 2000). Redox homeostasis must be tightly controlled to stay under the situation of oxidative stress and to act as signaling pathway. The chemical instability of reactive oxygen species (ROS) and reactive nitrogen species in living organisms is an important property which explains their multi-faceted roles in biology, particularly in plant–microbe interactions (Mittler et al., 2011; Puppo et al., 2013; Hichri et al., 2015; Meilhoc et al., 2015).
The symbiotic associations between Legumes (Fabaceae) and bacteria of Rhizobium type implies a recognition step which ultimately leads to the formation of nitrogen-fixing structures called nodules (Oldroyd and Downie, 2008). The interaction starts with the secretion of flavonoids by the plant roots. The perception of the flavonoids by the bacteria leads to the production of bacterial lipochito-oligosaccharides (Nod factors, NF), their specific recognition by the plant, and the induction of nodulation genes in both partners (Oldroyd and Downie, 2008). Increasing evidence support the critical role of ROS and nitric oxide (NO) in the recognition, signaling and immunity processes during the first steps of the symbiotic association between the two partners (Puppo et al., 2013). Both ROS and NO accumulate in roots and growing nodules according to specific spatiotemporal patterns, and regulate the expression of numerous genes that govern the development and the set-up of mature nodules. Several comprehensive reviews highlight the different functions played by these molecules in the nitrogen-fixing symbiosis (NFS) depending on the bacterial or plant origin (Boscari et al., 2013b; Puppo et al., 2013; Hichri et al., 2015; Meilhoc et al., 2015). In the present review, much attention will be paid on recent advance in the occurrence and the function of ROS and NO during the initial steps of NFS, and particularly on the specific role of NADPH oxidases, nitrate reductases (NRs) and hemoglobins (Hbs) in the control of the balance between ROS/NO production and catabolism.
Role of ROS in the Establishment of the Symbiotic Interaction
Involvement of NADPH Oxidase
Reactive oxygen species are transiently produced during rhizobial infection (Peleg-Grossman et al., 2009, 2012). The inhibition of ROS production by the NAD(P)H oxidase inhibitor diphenyleneiodonium (DPI; Peleg-Grossman et al., 2007), and the correlation between ROS accumulation and transcript accumulation of two NADPH oxidase genes in response to NF in Medicago truncatula roots (Lohar et al., 2007), argue for the involvement of NADPH oxidases in ROS generation. NADPH oxidase genes, also named respiratory burst oxidase homologs (Rbohs), were recently identified and characterized in legume genomes (Lohar et al., 2007; Marino et al., 2011; Montiel et al., 2012). In Phaseolus vulgaris, nine Rboh genes have been identified (Montiel et al., 2012). PvRbohB is particularly accumulated in shoots, roots and nodules. Promoter activity of PvRbohB was detected during infection thread (IT) progression and nodule development. Transgenic roots knocked-down for PvRbohB by RNA interference (RNAi), Montiel et al. (2012) showed a reduced ROS production with concomitant reduction of nodulation. Microscopy analysis revealed that progression of the ITs was affected in PvRbohB-RNAi roots indicating that RbohB could play a key role in successful rhizobial colonization and proper IT growth and shape (Montiel et al., 2012). Ten Rboh genes were identified in M. truncatula genome (Marino et al., 2012). Their involvement in H2O2 synthesis during root infection by Sinorhizobium meliloti needs to be fully evidenced, but downregulation of legume Rbohs leads to decreased nodulation efficiency and an impairment of nitrogen fixation (Marino et al., 2011; Puppo et al., 2013).
The involvement of other potential enzymatic ROS sources cannot be excluded. Type III peroxidases (Prx-III), which were implicated in generation of apoplastic ROS are good candidates (Martinez et al., 1998; Bindschedler et al., 2006). They were reported to promote cell wall hardening (Passardi et al., 2004) and rigidification of IT cell wall and matrix (Wisniewski et al., 2000). Whole transcriptome analyses reveal that Prx-III genes, which were named rhizobial induced peroxidases (Rip1-10), are inducible by rhizobia and NFs in root hairs (Breakspear et al., 2014), suggesting that they could be involved in root hair ROS production during plant-symbiont recognition.
ROS in Root Hair Response to Rhizobium and NF
A rise of ROS was observed in root cortical cells of inoculated M. truncatula plants, which peaked at 24 h after inoculation and remained high after 48 h (Peleg-Grossman et al., 2009, 2012). During the infection process, production of superoxide anion (O2-) and H2O2 was localized in IT and infected cells (Santos et al., 2001; Ramu et al., 2002; Rubio et al., 2004). In P. vulgaris, a transient increase of ROS was detected at the tip of root hairs within seconds after NF addition (Cardenas et al., 2008). However, after several minutes H2O2 production appears to be inhibited by NF (Shaw and Long, 2003; Lohar et al., 2007). ROS production was not observed in M. truncatula plants inoculated with a S. meliloti nodD1ABC mutant unable to produce NF, indicating that the oxidative burst is activated downstream NF perception (Ramu et al., 2002). Furthermore, suppression of immune responses (ROS production, SA accumulation) was observed in M. truncatula and M. sativa roots upon addition of NF (Martínez-Abarca et al., 1998; Shaw and Long, 2003). It was suggested that ROS production is necessary for infection initiation, but prolonged and elevated levels are detrimental to nodulation (Toth and Stacey, 2015). The subsequent hypothesis is that NF may activate a first ROS production wave involved in nodule development, and inhibit a second one involved in defense reactions. The first wave would modulate the expression of plant genes and/or the redox status of proteins involved in root hair deformation (Lohar et al., 2007), IT progression and nodule formation (Montiel et al., 2012; Puppo et al., 2013).
Moreover, H2O2 appears to control a key step of the interaction. An S. meliloti strain, overexpressing a catalase gene, showed a delayed nodulation phenotype associated with aberrant IT (Jamet et al., 2007). The catalase overexpression probably decreased the internal H2O2 concentration of the bacteria progressing inside the IT, as observed in free-living conditions. Thus, a positive role for H2O2 during IT elongation was proposed that could be related to IT rigidity (Rathbun et al., 2002), or to a cytoplasmic signal used by the bacteria to regulate symbiotic function (Pauly et al., 2006). Alternatively, specific posttranslational H2O2 protein modifications might occur in IT as observed for nitrogen fixing bacteroids (Oger et al., 2012).
Role of NO in the Establishment of the Symbiotic Interaction
NO Production
Several possible pathways of NO synthesis have been reported in plants which can be divided into oxidative (NO synthase like –NOS-like, polyamine-mediated, hydroxylamine-mediated) and reductive (NR, plasma membrane-bound nitrite NO reductase, mitochondrial electron transfer chain, xanthine oxidoreductase) pathways (Gupta et al., 2011; Mur et al., 2013). Two studies investigated potential NO source in the first step of NFS. In the first one, the treatment of soybean roots inoculated with Bradyrhizobium japonicum with the NOS inhibitor Nw-nitro-L-arginine (L-NNA) resulted in a 70% reduction of nodule number, suggesting the contribution of NOS-like enzyme in NO production (Leach et al., 2010). The other report shows that treatment of M. truncatula inoculated roots with tungstate, a NR inhibitor, mimics the addition of NO scavenger on the transcriptional regulation of genes involved in the nodulation process, whereas treatment with the NOS inhibitor L-NG-nitroarginine methyl ester (L-NAME) is ineffective (Boscari et al., 2013a). M. truncatula genome possess 3 NR genes, MtNR3 being only expressed during the nodulation process (Puppo et al., 2013). MtNR1 and MtNR2 are strongly induced during nodulation process (Figure 1). These results suggest a specific role of these enzymes, as a NO source, during symbiosis establishment. The potential involvement of the other plant NO sources was not yet investigated.
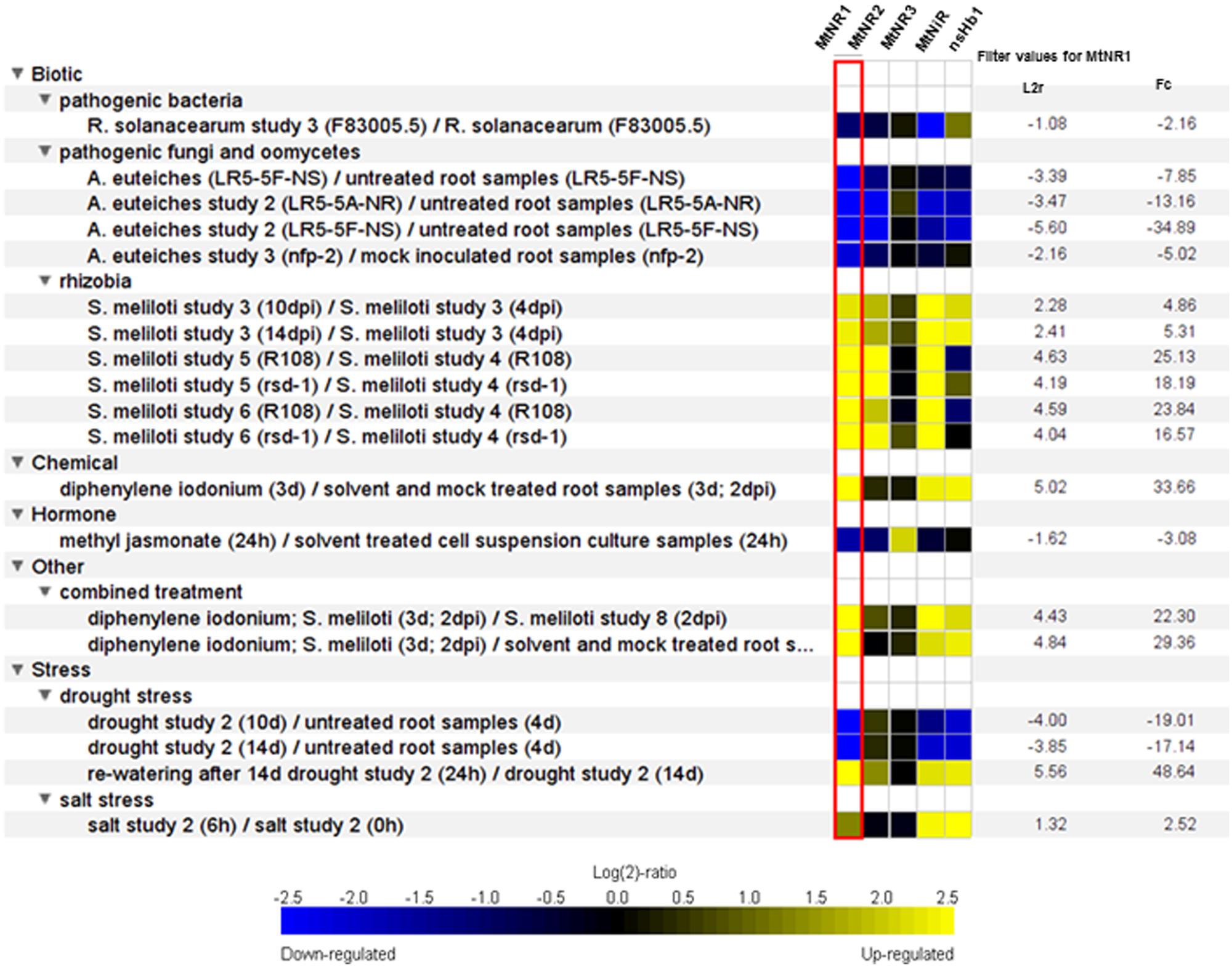
FIGURE 1. Screenshot of the results obtained with the Genevestigator Perturbation tool for the Medicago truncatula MtNR1 (MTR_3g073170), MtNR2 (MTR_5g059820), MtNR3 (MTR_3g073150), MtNiR (MTR_4g086020), ns-Hb1 (MTR_4g068860) genes on the Affymetrix 61K Medicago GeneChip. The “Heatmap-List” view is presented here. Perturbations are ordered according to the expression log2-ratios of MtNR1 and are filtered to keep only the perturbations leading to a atleast twofold-change and a p-value of maximum 0.05. Perturbations having the highest log2 ratio are listed first. R. solanacearum, Ralstonia solanacearum; A. euteiches, Aphanomyces euteiches; S. meliloti, Sinorhizobium meliloti.
In symbiotic bacteria, the main route for NO production is the denitrification pathway, which occurs both in free living bacteria under microoxic conditions and in nodules (Meilhoc et al., 2011). The other way for NO production could be NOS-like enzymes which activity was reported in many bacteria (Sudhamsu and Crane, 2009). In S. meliloti, a L-arginine-dependent NO production was reported in free-living cells, although no corresponding gene was found in its genome (Pii et al., 2007). To date, there is no evidence for an involvement of the bacterial partner in NO production during symbiosis establishment.
NO Metabolism
Toxic or signaling effects of NO depend on its concentration at the site of action (Mur et al., 2013). Therefore, NO concentration should be regulated to allow signaling functions to occur and to limit toxic effects. Hbs are known to act as NO scavengers in plants (Gupta et al., 2011). Based on their sequence homology and affinity for O2, plant Hbs have been classified into non-symbiotic hemoglobins (ns-Hbs), leghemoglobins, (Lbs), and truncated hemoglobins (tr-Hbs) (Gupta et al., 2011; Hill, 2012). The three Hb types are expressed in legumes (Nagata et al., 2008; Bustos-Sanmamed et al., 2011). Due to their very high affinity for O2 and NO (Km in the range of 1–20 nM), ns-Hbs and Lbs are able to scavenge O2 and NO traces to convert them to nitrate (Hill, 2012). Lbs, which accumulate to millimolar concentration in infected nodule cells (Appleby, 1992), are thought to buffer free O2 in the nanomolar range, avoiding inactivation of nitrogenase while maintaining high O2 flux for respiration (Ott et al., 2005). Tr-Hbs are induced in M. truncatula (Vieweg et al., 2005), and Frankia spp. (Niemann and Tisa, 2008; Coats et al., 2009) during NFS. Based on their expression pattern, it was also suggested that they could be involved in NO homeostasis. Other NO metabolizing pathways such as S-nitrosoglutathione reductase (GSNOR), glutathione peroxidase and thioredoxin systems, which are known to also regulate NO level in plants (Leterrier et al., 2011; Correa-Aragunde et al., 2015) have been evidenced in legumes (Renard et al., 2011; Matamoros et al., 2015), but their respective contributions to NO balance remains to be investigated in NFS.
Three Hb classes have been also described in bacteria: flavo-Hb (Hmp), single-domain Hb (sd-Hb), and truncated Hb (tr-Hb), which exhibit NO scavenging and detoxification activity (Sanchez et al., 2011). The lower competitiveness of S. meliloti overexpressing hmp strains as compared to the WT is an argument in favor of a Hmp role in NO control during the infection process (del Giudice et al., 2011). The respiratory NO reductase (Nor) and two proteins of the NnrS family (NnrS1 and NnrS2) were shown to be also involved in NO degradation and to be essential in maintaining efficient NFS (Cam et al., 2012; Meilhoc et al., 2013; Blanquet et al., 2015). However, their role in NO control during symbiosis establishment was not yet investigated.
NO in the Recognition of Rhizobium Partner
Numerous findings support the hypothesis that NO signaling plays a role in plant microbe recognition. Treatments of soybean roots with L-NNA reduced nodule number during interaction with B. japonicum, a phenotype reverted by the addition of the NO-donor diethylenetriamine NONOate (Leach et al., 2010). In the same way, the decrease of the NO content by 2-[4-carboxyphenyl]-4,4,5,5-tetramethyl imidazoline-1-oxyl-3-oxide (cPTIO) treatment and by hmp overexpression in the plant partner delayed nodulation in the M. truncatula – S. meliloti interaction, indicating that NO is required for an optimal establishment of the symbiotic process (del Giudice et al., 2011). Several reports underlined the role of plant Hbs in regulating NO level during symbiosis establishment. First, upon Lotus japonicus inoculation with Mesorhizobium loti, a transient production of NO was observed at the root surface 4 h post-inoculation (hpi), which then decreased to its basal level 10 hpi (Nagata et al., 2008). However, when L. japonicus was infected with the plant pathogens Ralstonia solanacearum and Pseudomonas syringae, NO was continuously produced for at least 24 hpi (Nagata et al., 2008). The decrease in NO level after its transient accumulation following infection with M. loti was assigned to LjHB1 which gene expression was upregulated by the symbiont, but not by the pathogens (Nagata et al., 2008, 2009; Murakami et al., 2011). In addition, NO was shown to up-regulate ns-Hb expression in different plant species (Shimoda et al., 2005; Sasakura et al., 2006; Nagata et al., 2008). These observations suggest that at early step of symbiotic interaction, the initial burst of NO induces the expression of ns-Hb that, in return, scavenges NO and down-regulates its level to lower plant defense response and allow the reception of the symbiont. Experimental evidence showed that lipopolysaccharides from M. loti induced LjHB1 expression and NO production in L. japonicus roots (Nagata et al., 2009; Murakami et al., 2011). They showed the polymer distributed on the outer membrane of Gram-negative bacteria play a major role in the recognition and establishment of symbiosis.
Nitric oxide production was also observed in the infection pockets, along the ITs, and in dividing cortical cells of the nodule primordia (del Giudice et al., 2011). The presence of NO in nodule primordia present high similarity with the local NO increase observed in lateral root primordia (Correa-Aragunde et al., 2004; Lanteri et al., 2006). In both studies, authors reported that NO could modulate the expression of cell cycle regulatory genes (Correa-Aragunde et al., 2006; del Giudice et al., 2011). It was notably observed in M. truncatula that NO scavenging provokes the down regulation of plant genes involved in nodule development, such as MtCRE1 and MtCCS52A (del Giudice et al., 2011). Furthermore, transcriptomic analysis of cPTIO-treated inoculated roots of M. truncatula showed that NO is involved in the regulation of many family of genes related to cell cycle process and protein synthesis in nodule primordia (Boscari et al., 2013a), which reinforces the hypothesis that NO plays a similar function in nodule and lateral root organogenesis.
Interestingly, the control of NO homeostasis through the spatiotemporal coordination of NR and Hb gene expression was recently hypothesized to participate to nitrate sensing in maize roots (Trevisan et al., 2015). Moreover, Frungillo et al. (2015) demonstrate that NO is at the center of nitrogen homeostasis in Arabidopsis plants. They demonstrated that NO derived from nitrate assimilation inhibits the activity of GSNOR, which controls the cellular levels of GSNO, by S-nitrosylation (addition of a NO group to cysteine thiols) of some of its cysteine residues. They observed that inhibition of GSNOR is necessary to amplify a SNO signal, which in turn feedback regulates nitrate assimilation (Figure 2). It is noteworthy that nodulation efficiency is as well finely tuned according to the nitrate availability (Streeter, 1988).
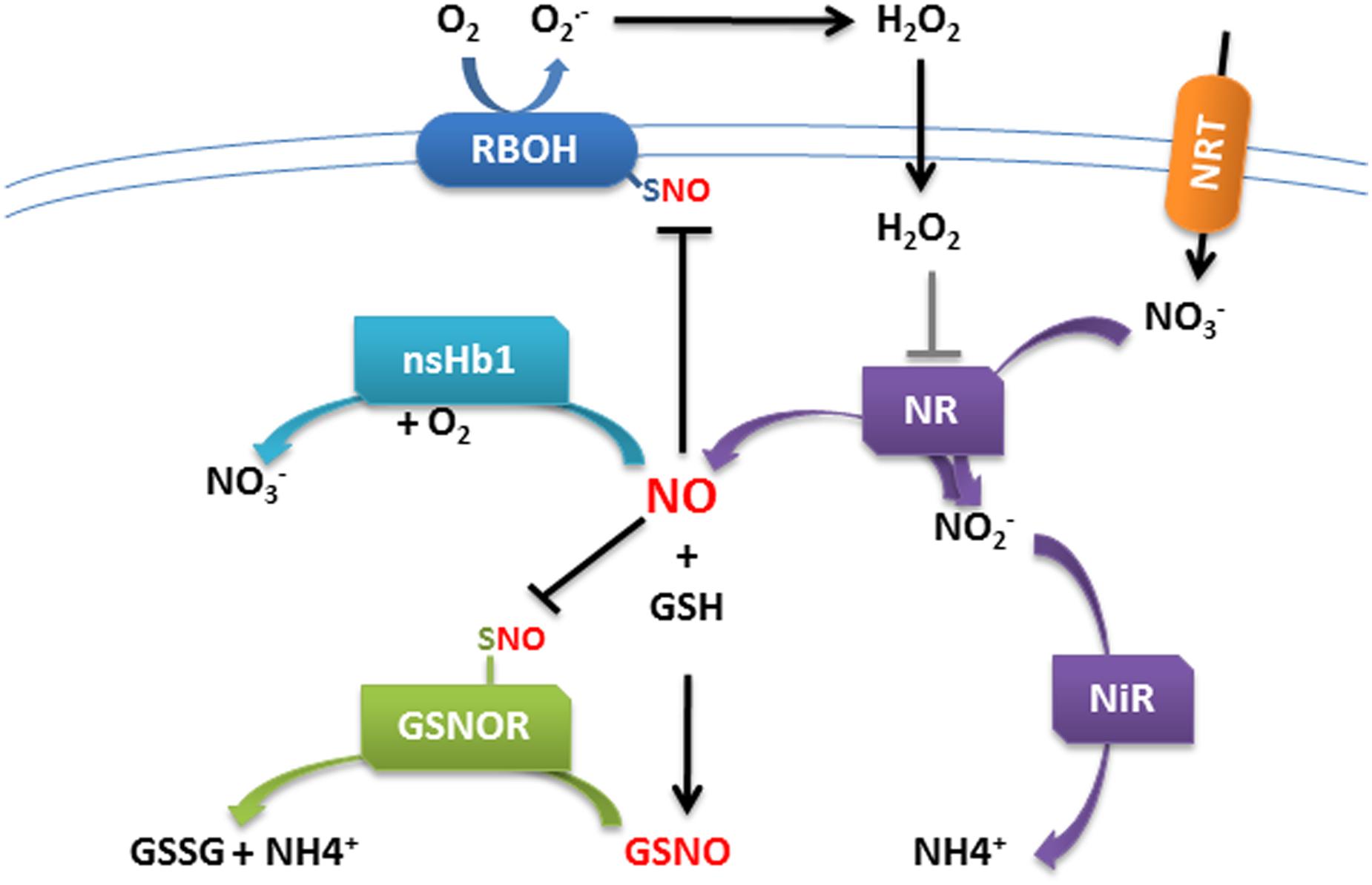
FIGURE 2. Schematic model of cross-talk regulation between ROS and NO in plant cells. H2O2 produced by NADPH oxidase leads to the activation of nitrate reductase (NR) and concomitant NO production. NO accumulation in turn blunts NADPH oxidase activity by S-nitrosylation, preventing accumulation of excess ROS. In the nitrogen assimilation pathway, nitrate (NO3-) is taken up by nitrate transporters (NRT) and reduced to nitrite (NO2-) by NR. Nitrite is reduced to NO by NR. NO reacts with reduced glutathione (GSH) in the presence of O2 to form S-nitrosoglutathione (GSNO). GSNO is converted by the enzyme GSNO reductase (GSNOR) into oxidized glutathione (GSSG) and NH3. NO accumulation leads to GSNOR S-nitrosylation and inhibition preventing GSNO degradation.
NO and ROS Interplay
Overall, it appears that ROS and NO present a fine-tuned spatio-temporal modulation which plays a critical role in signaling and immunity in the associations between Legumes and rhizobia. The occurrence of an interplay between ROS and NO was supported by the finding of both S-sulfenylated and S-nitrosylated proteins posttranslational modification during NFS (Oger et al., 2012; Puppo et al., 2013), linking ROS/NO production to a redox-based regulation of the symbiotic process. Peroxynitrite (ONOO-) is a signaling molecule formed when NO reacts with O2-. Its function may be mediated by the selective nitration of Tyr residues in a small number of proteins. Blanquet et al. (2015) reported that glutamine synthetase GS1a, a key enzyme in N2-fixation, is nitrated in nodules. Such NO/ONOO--mediated posttranslational inactivation of GS provides a direct link between NO/O2- signaling and nitrogen metabolism in root nodules. Whether these molecules work in synergy in early symbiosis steps is not determined yet, however, the comparison of M. truncatula inoculated roots treated with either the NADPH oxidase inhibitor (Andrio et al., 2012), or the NO scavenger (Boscari et al., 2013a), reveals a strong overlap in the signaling pathways triggered by either molecule. Furthermore 75% of the 316 differentially regulated genes identified in both analyses are similarly up- or down-regulated (Puppo et al., 2013). Amongst the up-regulated genes, some gene families involved in plant defense and secondary metabolism were notably identified, as previously highlighted during cell death induction (Zago et al., 2006). Moreover, in transcriptomic analysis of DPI-treated M. truncatula roots (Andrio et al., 2012), MtNR1, MtNiR, and Mtns-Hb1 were found strongly up-regulated (Figure 1), suggesting that H2O2 could control the transcriptional regulation of enzymes involved in NO homeostasis. In the same way, it was recently reported that an elevated ROS concentration during plant–pathogen interaction results in the activation of NR (Wang et al., 2010; Lin et al., 2012). Interestingly, in Arabidopsis leaves, NR activation leads to increased NR-mediated NO production, and to the subsequent inactivation of NADPH oxidase activity by S-nitrosylation during pathogen infection (Yun et al., 2011). These results evidence the overlapping connection between NO and ROS production with a negative feedback loop of the NO on ROS production (Figure 2). However, such type of ROS/NO interplay still has to be clearly elucidated in roots and during establishment of NFS.
Conclusions and Future Directions
We showed that spatio-temporal accumulations of ROS and NO are critical for the specificity of their function throughout the successive steps of symbiosis initiation. NADPH oxidases were identified as major source of ROS production, and NR and NOS-like have been evidenced as NO sources during the early steps of the interaction. Importance of plant and bacterial Hbs in NO balance was particularly highlighted. However, the involvement of alternative systems like catalase, glutathione peroxidase, GSNOR and thioredoxins remains to be investigated to decipher their respective contribution in ROS and NO balance. Transcriptomic analyses point to the involvement of ROS and NO in the success of the infection process notably by the repression of plant defense responses favoring the establishment of the symbiosis Further investigations will aim to decipher the possible regulation of nodule NADPH oxidase activity by NO and the transcriptional regulation of genes involved in NO homeostasis by H2O2 in symbiosis establishment.
Author Contributions
ID, NP, and AP contribute to the writing of the different paragraph about ROS. RB and AB contribute to the writing of the different paragraph about NO and interplay ROS/NO.
Conflict of Interest Statement
The authors declare that the research was conducted in the absence of any commercial or financial relationships that could be construed as a potential conflict of interest.
Acknowledgments
This work was supported by the “Institut National de la Recherche Agronomique,” the “Centre National de la Recherche Scientifique,” the University of Nice–Sophia Antipolis and the French Government (National Research Agency, ANR) through the LABEX SIGNALIFE program (reference # ANR-11-LABX-0028-01). ID was supported by a post-doctoral fellowship from the ANR (Projet CAROLS : ANR-11-BSV7-010-02).
References
Andrio, E., Marino, D., Marmeys, A., Dunoyer, De Segonzac, M., Damiani, I., et al. (2012). Hydrogen peroxide-regulated genes in the Medicago truncatula – Sinorhizobium meliloti symbiosis. New Phytol. 198, 179–189. doi: 10.1111/nph.12120
Appleby, C. A. (1992). The origin and functions of haemoglobinin Plants. Sci. Prog. 76, 365–398. doi: 10.1093/molbev/mss164
Bindschedler, L. V., Dewdney, J., Blee, K. A., Stone, J. M., Asai, T., Plotnikov, J., et al. (2006). Peroxidase-dependent apoplastic oxidative burst in Arabidopsis required for pathogen resistance. Plant J. Mol. Biol. 47, 851–863. doi: 10.1111/j.1365-313X.2006.02837.x
Blanquet, P., Silva, L., Catrice, O., Bruand, C., Carvalho, H., and Meilhoc, E. (2015). Sinorhizobium meliloti controls nitric oxide-mediated post-translational modification of a medicago truncatula nodule protein. Mol. Plant Microbe Interact. 28, 1353–1363. doi: 10.1094/MPMI-05-15-0118-R
Boscari, A., Del Giudice, J., Ferrarini, A., Venturini, L., Zaffini, A. L., Delledonne, M., et al. (2013a). Expression dynamics of the Medicago truncatula transcriptome during the symbiotic interaction with Sinorhizobium meliloti: which role for nitric oxide? Plant Physiol. 161, 425–439. doi: 10.1104/pp.112.208538
Boscari, A., Meilhoc, E., Castella, C., Bruand, C., Puppo, A., and Brouquisse, R. (2013b). Which role for nitric oxide in symbiotic N2-fixing nodules: toxic by-product or useful signaling/metabolic intermediate? Front. Plant Sci. 4:384. doi: 10.3389/fpls.2013.00384
Breakspear, A., Liu, C., Roy, S., Stacey, N., Rogers, C., Trick, M., et al. (2014). The root hair “infectome” of Medicago truncatula uncovers changes in cell cycle genes and reveals a requirement for Auxin signaling in rhizobial infection. Plant Cell 26, 4680–4701. doi: 10.1105/tpc.114.133496
Bustos-Sanmamed, P., Tovar-Mendez, A., Crespi, M., Sato, S., Tabata, S., and Becana, M. (2011). Regulation of nonsymbiotic and truncated hemoglobin genes of Lotus japonicus in plant organs and in response to nitric oxide and hormones. New Phytol. 189, 765–776. doi: 10.1111/j.1469-8137.2010.03527.x
Cam, Y., Pierre, O., Boncompagni, E., Herouart, D., Meilhoc, E., and Bruand, C. (2012). Nitric oxide (NO): a key player in the senescence of Medicago truncatula root nodules. New Phytol. 196, 548–560. doi: 10.1111/j.1469-8137.2012.04282.x
Cardenas, L., Martinez, A., Sanchez, F., and Quinto, C. (2008). Fast, transient and specific intracellular ROS changes in living root hair cells responding to Nod factors (NFs). Plant J. 56, 802–813. doi: 10.1111/j.1365-313X.2008.03644.x
Coats, V., Schwintzer, C. R., and Tjepkema, J. D. (2009). Truncated hemoglobins in Frankia CcI3: effects of nitrogen source, oxygen concentration, and nitric oxide. Can. J. Micobiol. 55, 867–873. doi: 10.1139/w09-042
Correa-Aragunde, N., Foresi, N., and Lamattina, L. (2015). Nitric oxide is a ubiquitous signal for maintaining redox balance in plant cells: regulation of ascorbate peroxidase as a case study. J. Exp. Bot. 66, 2913–2921. doi: 10.1093/jxb/erv073
Correa-Aragunde, N., Graziano, M., Chevalier, C., and Lamattina, L. (2006). Nitric oxide modulates the expression of cell cycle regulatory genes during lateral root formation in tomato. J. Exp. Bot. 57, 581–588. doi: 10.1093/jxb/erj045
Correa-Aragunde, N., Graziano, M., and Lamattina, L. (2004). Nitric oxide plays a central role in determining lateral root development in tomato. Planta 218, 900–905. doi: 10.1007/s00425-003-1172-7
del Giudice, J., Cam, Y., Damiani, I., Fung-Chat, F., Meilhoc, E., Bruand, C., et al. (2011). Nitric oxide is required for an optimal establishment of the Medicago truncatula-Sinorhizobium meliloti symbiosis. New Phytol. 191, 405–417. doi: 10.1111/j.1469-8137.2011.03693.x
Frungillo, L., Skelly, M. J., Loake, G. J., Spoel, S. H., and Salgado, I. (2015). S-nitrosothiols regulate nitric oxide production and storage in plants through the nitrogen assimilation pathway. Nat. Commun. 5:5401. doi: 10.1038/ncomms6401
Gupta, K. J., Fernie, A. R., Kaiser, W. M., and Van Dongen, J. T. (2011). On the origins of nitric oxide. Trends Plant Sci. 16, 160–168. doi: 10.1016/j.tplants.2010.11.007
Hentschel, U., Steinert, M., and Hacker, J. (2000). Common molecular mechanisms of symbiosis and pathogenesis. Trends Microbiol. 8, 226–231. doi: 10.1016/S0966-842X(00)01758-3
Hichri, I., Boscari, A., Castella, C., Rovere, M., Puppo, A., and Brouquisse, R. (2015). Nitric oxide: a multifaceted regulator of the nitrogen-fixing symbiosis. J. Exp. Bot. 66, 2877–2887. doi: 10.1093/jxb/erv051
Hill, R. D. (2012). Non-symbiotic haemoglobins-What’s happening beyond nitric oxide scavenging? AoB Plants 2012:ls004. doi: 10.1093/aobpla/pls004
Jamet, A., Mandon, K., Puppo, A., and Herouart, D. (2007). H2O2 is required for optimal establishment of the Medicago sativa/Sinorhizobium meliloti symbiosis. J. Bacteriol. 189, 8741–8745. doi: 10.1128/JB.01130-07
Lanteri, M. L., Pagnussat, G. C., and Lamattina, L. (2006). Calcium and calcium-dependent protein kinases are involved in nitric oxide- and auxin-induced adventitious root formation in cucumber. J. Exp. Bot. 57, 1341–1351. doi: 10.1093/jxb/erj109
Leach, J., Keyster, M., Du Plessis, M., and Ludidi, N. (2010). Nitric oxide synthase activity is required for development of functional nodules in soybean. J. Plant Physiol. 167, 1584–1591. doi: 10.1016/j.jplph.2010.06.019
Leterrier, M., Chaki, M., Airaki, M., Valderrama, R., Palma, J. M., Barroso, J. B., et al. (2011). Function of S-nitrosoglutathione reductase (GSNOR) in plant development and under biotic/abiotic stress. Plant Signal. Behav. 6, 789–793. doi: 10.4161/psb.6.6.15161
Lin, A., Wang, Y., Tang, J., Xue, P., Li, C., Liu, L., et al. (2012). Nitric oxide and protein S-nitrosylation are integral to hydrogen peroxide-induced leaf cell death in rice. Plant Physiol. 158, 451–464. doi: 10.1104/pp.111.184531
Lohar, D. P., Haridas, S., Gantt, J. S., and Vandenbosch, K. A. (2007). A transient decrease in reactive oxygen species in roots leads to root hair deformation in the legume–rhizobia symbiosis. New Phytologist 173, 39–49. doi: 10.1111/j.1469-8137.2006.01901.x
Marino, D., Andrio, E., Danchin, E. G. J., Oger, E., Gucciardo, S., Lambert, A., et al. (2011). A Medicago truncatula NADPH oxidase is involved in symbiotic nodule functioning. New Phytol. 189, 580–592. doi: 10.1111/j.1469-8137.2010.03509.x
Marino, D., Dunand, C., Puppo, A., and Pauly, N. (2012). A burst of plant NADPH oxidases. Trends Plant Sci. 17, 9–15. doi: 10.1016/j.tplants.2011.10.001
Martinez, C., Montillet, J. L., Bresson, E., Agnel, J. P., Dai, G. H., Daniel, J. F., et al. (1998). Apoplastic peroxidase generates superoxide anions in cells of cotton cotyledons undergoing the hypersensitive reaction to Xanthomonas campestris pv. malvacearum Race 18. Mol. Plant Microbe Interact. 11, 1038–1047. doi: 10.1094/MPMI.1998.11.11.1038
Martínez-Abarca, F., Herrera-Cervera, J. A., Bueno, P., Sanjuan, J., Bisseling, T., and Olivares, J. (1998). Involvement of salicylic acid in the establishment of the Rhizobium meliloti - Alfalfa symbiosis. Mol. Plant Microbe Interact. 11, 153–155. doi: 10.1094/MPMI.1998.11.2.153
Matamoros, M. A., Saiz, A., Peñuelas, M., Bustos-Sanmamed, P., Mulet, J. M., Barja, M. V., et al. (2015). Function of glutathione peroxidases in legume root nodules. J. Exp. Bot. 66, 2979–2990. doi: 10.1093/jxb/erv066
Meilhoc, E., Blanquet, P., Cam, Y., and Bruand, C. (2013). Control of NO level in rhizobium-legume root nodules: not only a plant globin story. Plant Signal. Behav. 8:10. doi: 10.4161/psb.25923
Meilhoc, E., Boscari, A., Brouquisse, R., and Bruand, C. (2015). “Multifaceted roles of nitric oxide in legume–rhizobium symbioses,” in Biological Nitrogen Fixation, ed. F. J. de Bruijn (Hoboken, NJ: John Wiley & Sons, Inc.), 637–648.
Meilhoc, E., Boscari, A., Bruand, C., Puppo, A., and Brouquisse, R. (2011). Nitric oxide in legume-rhizobium symbiosis. Plant Sci. 181, 573–581. doi: 10.1016/j.plantsci.2011.04.007
Mittler, R., Vanderauwera, S., Suzuki, N., Miller, G., Tognetti, V. B., Vandepoele, K., et al. (2011). ROS signaling: the new wave? Trends Plant Sci. 16, 300–309. doi: 10.1016/j.tplants.2011.03.007
Moné, Y., Monnin, D., and Kremer, N. (2014). The oxidative environment: a mediator of interspecies communication that drives symbiosis evolution. Proc. Biol. Sci. 281:20133112. doi: 10.1098/rspb.2013.3112
Montiel, J. S., Nava, N., CáRdenas, L., SáNchez-López, R., Arthikala, M.-K., Santana, O., et al. (2012). A Phaseolus vulgaris NADPH oxidase gene is required for root infection by rhizobia. Plant Cell Physiol. 53, 1751–1767. doi: 10.1093/pcp/pcs120
Mur, L. A., Mandon, J., Persijn, S., Cristescu, S. M., Moshkov, I. E., Novikova, G. V., et al. (2013). Nitric oxide in plants: an assessment of the current state of knowledge. AoB Plants 5:ls052. doi: 10.1093/aobpla/pls052
Murakami, E., Nagata, M., Shimoda, Y., Kucho, K., Higashi, S., Abe, M., et al. (2011). Nitric oxide production induced in roots of Lotus japonicus by lipopolysaccharide from Mesorhizobium loti. Plant Cell Physiol. 52, 610–617. doi: 10.1093/pcp/pcr020
Nagata, M., Hashimoto, M., Murakami, E., Shimoda, Y., Shimoda-Sasakura, F., Kucho, K., et al. (2009). A possible role of class 1 plant hemoglobin at the early stage of legume-rhizobium symbiosis. Plant Signal. Behav. 4, 202–204. doi: 10.4161/psb.4.3.7796
Nagata, M., Murakami, E., Shimoda, Y., Shimoda-Sasakura, F., Kucho, K., Suzuki, A., et al. (2008). Expression of a class 1 hemoglobin gene and production of nitric oxide in response to symbiotic and pathogenic bacteria in Lotus japonicus. Mol. Plant Microbe Interact. 21, 1175–1183. doi: 10.1094/MPMI-21-9-1175
Niemann, J., and Tisa, L. S. (2008). Nitric oxide and oxygen regulate truncated hemoglobin gene expression in Frankia strain CcI3. J. Bacteriol. 190, 7864–7867. doi: 10.1128/JB.01100-08
Oger, E., Marino, D., Guigonis, J. M., Pauly, N., and Puppo, A. (2012). Sulfenylated proteins in the Medicago truncatula-Sinorhizobium meliloti symbiosis. J. Proteomics 75, 4102–4113. doi: 10.1016/j.jprot.2012.05.024
Oldroyd, G. E., and Downie, J. A. (2008). Coordinating nodule morphogenesis with rhizobial infection in legumes. Annu. Rev. Plant Biol. 59, 519–546. doi: 10.1146/annurev.arplant.59.032607.092839
Ott, T., Van Dongen, J. T., Gunther, C., Krusell, L., Desbrosses, G., Vigeolas, H., et al. (2005). Symbiotic leghemoglobins are crucial for nitrogen fixation in legume root nodules but not for general plant growth and development. Curr. Biol. 15, 531–535. doi: 10.1016/j.cub.2005.01.042
Passardi, F., Longet, D., Penel, C., and Dunand, C. (2004). The class III peroxidase multigenic family in rice and its evolution in land plants. Phytochemistry 65, 1879–1893. doi: 10.1016/j.phytochem.2004.06.023
Pauly, N., Pucciariello, C., Mandon, K., Innocenti, G., Jamet, A., Baudouin, E., et al. (2006). Reactive oxygen and nitrogen species and glutathione: key players in the legume-Rhizobium symbiosis. J. Exp. Bot. 57, 1769–1776. doi: 10.1093/jxb/erj184
Peleg-Grossman, S., Golani, Y., Kaye, Y., Melamed-Book, N., and Levine, A. (2009). NPR1 protein regulates pathogenic and symbiotic interactions between Rhizobium and legumes and non-legumes. PLoS ONE 4:e8399. doi: 10.1371/journal.pone.0008399
Peleg-Grossman, S., Melamed-Book, N., and Levine, A. (2012). ROS production during symbiotic infection suppresses pathogenesis-related gene expression. Plant Signal. Behav. 7, 409–415. doi: 10.4161/psb.19217
Peleg-Grossman, S., Volpin, H., and Levine, A. (2007). Root hair curling and Rhizobium infection in Medicago truncatula are mediated by phosphatidylinositide-regulated endocytosis and reactive oxygen species. J. Exp. Bot. 58, 1637–1649. doi: 10.1093/jxb/erm013
Pii, Y., Crimi, M., Cremonese, G., Spena, A., and Pandolfini, T. (2007). Auxin and nitric oxide control indeterminate nodule formation. BMC Plant Biol. 7:21. doi: 10.1186/1471-2229-7-21
Puppo, A., Pauly, N., Boscari, A., Mandon, K., and Brouquisse, R. (2013). Hydrogen peroxide and nitric oxide: key regulators of the Legume-Rhizobium and mycorrhizal symbioses. Antioxid Redox Signal. 18, 2202–2219. doi: 10.1089/ars.2012.5136
Ramu, S. K., Peng, H.-M., and Cook, D. R. (2002). Nod factor induction of reactive oxygen species production is correlated with expression of the early nodulin gene rip1 in Medicago truncatula. Mol. Plant Microbe Interact. 15, 522–528. doi: 10.1094/MPMI.2002.15.6.522
Rathbun, E. A., Naldrett, M. J., and Brewin, N. J. (2002). Identification of a family of extensin-like glycoproteins in the lumen of Rhizobium-induced infection threads in Pea root nodules. Mol. Plant Microbe Interact. 15, 350–359. doi: 10.1094/MPMI.2002.15.4.350
Renard, M., Alkhalfioui, F., Schmitt-Keichinger, C., Ritzenthaler, C., and Montrichard, F. (2011). Identification and characterization of thioredoxin h isoforms differentially expressed in germinating seeds of the model legume Medicago truncatula. Plant Physiol. 155, 1113–1126. doi: 10.1104/pp.110.170712
Rubio, M. C., James, E. K., Clemente, M. R., Bucciarelli, B., Fedorova, M., Vance, C. P., et al. (2004). Localization of superoxide dismutases and hydrogen peroxide in legume root nodules. Mol. Plant Microbe Interact. 17, 1294–1305. doi: 10.1094/MPMI.2004.17.12.1294
Sanchez, C., Cabrera, J. J., Gates, A. J., Bedmar, E. J., Richardson, D. J., and Delgado, M. J. (2011). Nitric oxide detoxification in the rhizobia-legume symbiosis. Biochem. Soc. Trans. 39, 184–188. doi: 10.1042/BST0390184
Santos, R., Hérouart, D., Sigaud, S., Touati, D., and Puppo, A. (2001). Oxidative burst in alfalfa-sinorhizobium meliloti symbiotic interaction. Mol. Plant Microbe Interact. 14, 86–89. doi: 10.1094/MPMI.2001.14.1.86
Sasakura, F., Uchiumi, T., Shimoda, Y., Suzuki, A., Takenouchi, K., Higashi, S., et al. (2006). A Class 1 Hemoglobin gene from alnus firma functions in symbiotic and nonsymbiotic tissues to detoxify nitric oxide. Mol. Plant Microbe Interact. 19, 441–450. doi: 10.1094/MPMI-19-0441
Shaw, S. L., and Long, S. R. (2003). Nod factor inhibition of reactive oxygen efflux in a host legume. Plant Physiol. 132, 2196–2204. doi: 10.1104/pp.103.021113
Shimoda, Y., Nagata, M., Suzuki, A., Abe, M., Sato, S., Kato, T., et al. (2005). Symbiotic rhizobium and nitric oxide induce gene expression of non-symbiotic hemoglobin in Lotus japonicus. Plant Cell Physiol. 46, 99–107. doi: 10.1093/pci/pci001
Streeter, J. G. (1988). Inhibition of legume nodule formation and N2 fixation by nitrate. CRC Crit. Rev. Plant Sci. 7, 1–23. doi: 10.1080/07352688809382257
Sudhamsu, J., and Crane, B. R. (2009). Bacterial nitric oxide synthases: what are they good for? Trends Microbiol. 17, 212–218. doi: 10.1016/j.tim.2009.02.003
Toth, K., and Stacey, G. (2015). Does plant immunity play a critical role during initiation of the legume-rhizobium symbiosis? Front. Plant Sci. 6:401. doi: 10.3389/fpls.2015.00401
Trevisan, S., Manoli, A., Ravazzolo, L., Botton, A., Pivato, M., Masi, A., et al. (2015). Nitrate sensing by the maize root apex transition zone: a merged transcriptomic and proteomic survey. J. Exp. Bot. 66, 3699–3715. doi: 10.1093/jxb/erv165
Vieweg, M. F., Hohnjec, N., and Kuster, H. (2005). Two genes encoding different truncated hemoglobins are regulated during root nodule and arbuscular mycorrhiza symbioses of Medicago truncatula. Planta 220, 757–766. doi: 10.1007/s00425-004-1397-0
Wang, P., Du, Y., Li, Y., Ren, D., and Song, C. P. (2010). Hydrogen peroxide-mediated activation of MAP kinase 6 modulates nitric oxide biosynthesis and signal transduction in Arabidopsis. Plant Cell 22, 2981–2998. doi: 10.1105/tpc.109.072959
Wisniewski, J. P., Rathbun, E. A., Knox, J. P., and Brewin, N. J. (2000). Involvement of diamine oxidase and peroxidase in insolubilization of the extracellular matrix: implications for pea nodule initiation by Rhizobium leguminosarum. Mol. Plant Microbe Interact. 13, 413–420. doi: 10.1094/MPMI.2000.13.4.413
Yun, B. W., Feechan, A., Yin, M., Saidi, N. B., Le Bihan, T., Yu, M., et al. (2011). S-nitrosylation of NADPH oxidase regulates cell death in plant immunity. Nature 478, 264–268. doi: 10.1038/nature10427
Keywords: legume, nitric oxide, nitrogen fixation, Rhizobium, symbiosis
Citation: Damiani I, Pauly N, Puppo A, Brouquisse R and Boscari A (2016) Reactive Oxygen Species and Nitric Oxide Control Early Steps of the Legume – Rhizobium Symbiotic Interaction. Front. Plant Sci. 7:454. doi: 10.3389/fpls.2016.00454
Received: 23 December 2015; Accepted: 23 March 2016;
Published: 08 April 2016.
Edited by:
Jeremy Astier, Helmholtz Zentrum München, GermanyReviewed by:
Alberto A. Iglesias, Universidad Nacional del Litoral – Consejo Nacional de Investigaciones Científicas y Técnicas, ArgentinaAndreas Niebel, Centre National de la Recherche Scientifique, France
Copyright © 2016 Damiani, Pauly, Puppo, Brouquisse and Boscari. This is an open-access article distributed under the terms of the Creative Commons Attribution License (CC BY). The use, distribution or reproduction in other forums is permitted, provided the original author(s) or licensor are credited and that the original publication in this journal is cited, in accordance with accepted academic practice. No use, distribution or reproduction is permitted which does not comply with these terms.
*Correspondence: Alexandre Boscari, YWxleGFuZHJlLmJvc2NhcmlAc29waGlhLmlucmEuZnI=