- 1National Key Laboratory of Crop Genetic Improvement, National Center of Oil Crop Improvement (Wuhan), College of Plant Science and Technology, Huazhong Agricultural University, Wuhan, China
- 2College of Chemistry and Life Science, Hubei University of Education, Wuhan, China
Allopolyploidization with the merger of the genomes from different species has been shown to be associated with genetic and epigenetic changes. But the maintenance of such alterations related to one parental species after the genome is extracted from the allopolyploid remains to be detected. In this study, the genome of Brassica napus L. (2n = 38, genomes AACC) was extracted from its intergeneric allohexaploid (2n = 62, genomes AACCOO) with another crucifer Orychophragmus violaceus (2n = 24, genome OO), by backcrossing and development of alien addition lines. B. napus-type plants identified in the self-pollinated progenies of nine monosomic additions were analyzed by the methods of amplified fragment length polymorphism, sequence-specific amplified polymorphism, and methylation-sensitive amplified polymorphism. They showed modifications to certain extents in genomic components (loss and gain of DNA segments and transposons, introgression of alien DNA segments) and DNA methylation, compared with B. napus donor. The significant differences in the changes between the B. napus types extracted from these additions likely resulted from the different effects of individual alien chromosomes. Particularly, the additions which harbored the O. violaceus chromosome carrying dominant rRNA genes over those of B. napus tended to result in the development of plants which showed fewer changes, suggesting a role of the expression levels of alien rRNA genes in genomic stability. These results provided new cues for the genetic alterations in one parental genome that are maintained even after the genome becomes independent.
Introduction
Allopolyploidization with combination of two or more divergent genomes through interspecific hybridizations is a shaping force in the plant speciation (Ramsey and Schemske, 1998), as it lead to immediate evolutionary advantage, such as adaptation to novel niches and changing environment (Parisod et al., 2010b). At chromosomal and genomic levels, polyploidy can lead to gene redundancy (Adams and Wendel, 2005; Moore and Purugganan, 2005), translocation and transposition (interchromosomal exchanges), unequal crossing over, transposon-mediated event, mutation, sequence deletion and insertion (illegitimate recombination) (Chen, 2007; Doyle et al., 2008). Studies on serial resynthesized polyploids (e.g., Arabidopsis, Brassica, Gossypium, Nicotiana, Triticum, and Triticale) and natural polyploid species have revealed genomic changes in nascent polyploid taxa, including deletion events, gene conversion events, rDNA loci changes, transposon activation, chromosomal rearrangements, and epigenetic phenomena (Comai, 2005; Chen, 2007; Doyle et al., 2008). Also, allopolyploidy is considered to play a major role in gene expression especially non-additive expression of homoeologous genes (Chen, 2007; Li et al., 2014).
In contrast to allopolyploidy, aneuploidy refers to karyotypic abnormalities with the gain or loss of one or more chromosomes from the normal number (2n) in the cells, which disrupts the balanced complement and has detrimental effects on various aspects of growth and developments in all organisms studied (Siegel and Amon, 2012). The abnormalities in phenotype and development are attributable to the genome-wide gene expression perturbation revealed in plants and animals (Letourneau et al., 2014; Zhu et al., 2015). Aneuploidy may increase genomic instability (increased rates of chromosome mis-segregation, mitotic recombination, mutation, increased DNA damage), resulting in the reduced cell fitness (Janssen et al., 2011; Crasta et al., 2012). However, plants appear to be remarkably resistant to the adverse effects of ploidy and show high viability (Makarevitch and Harris, 2010); aneuploidy may play a role in both speciation and genome evolution (Ramsey and Schemske, 2002).
Monosomic alien addition lines are another type of aneuploids that have one chromosome added to their genome from an alien species. A complete set of MAALs, each line carrying a chromosome of an alien species can be developed by recurrent backcrossing of the natural or synthesized allopolyploids with one of the parental species, which results in the dissection of the genome of the donor species. The complete set of MAALs is widely used for studying the genome structure, genetic mapping and breeding bridge to transfer target genes and traits from the alien chromosome to the recipient genome (Zhao et al., 2008; Prakash et al., 2009; Geleta et al., 2012; Ding et al., 2013; Kang et al., 2014). But how the individual alien chromosomes impact the host genome remain to be elucidated. The extent of the genetic and the epigenetic changes in the genome of one parent effected by allopolyploidization/aneuploidization can be determined by comparing the parental species among the progenies resulting from self-pollination of the MAALs.
The globally important oilseed rape (Brassica napus L.) is a young allotetraploid species (2n = 4x = 38, genomes AACC); it originated ∼7500 years ago from natural interspecific hybridization between B. rapa L. (2n = 20, genome AA) and B. oleracea L. (2n = 18, genome CC) (Chalhoub et al., 2014). B. napus has been one of the model system for studying genetic and phenotypic changes associated with allopolyploid formation at genomic, transcriptomic and proteomic levels (Song et al., 1995; Albertin et al., 2006; Lukens et al., 2006; Gaeta et al., 2007, 2009; Xu et al., 2009; Ksiazczyk et al., 2011; Xiong et al., 2011; Zhou et al., 2011; Cui et al., 2012, 2013), together with another model system Arabidopsis suecica (Pontes et al., 2004; Chen, 2007). A set of nine MAALs of B. napus with one of the different chromosome from another crucifer Orychophragmus violaceus (L.) O. E. Schulz (2n = 24, genome OO) have been obtained and characterized for their phenotype and cytology (Ding et al., 2013). These MAALs were developed by producing the intergeneric somatic hybrid/allohexaploid with the sum of the chromosomes from these two species (2n = 6x = 62, genomes AACCOO) and then recurrent backcrossing of the allohexaploid with B. napus (Zhao et al., 2008; Ding et al., 2013). These lines were distinguishable from each other, because they expressed the phenotypes and chromosome markers specific for O. violaceus. In particular, the rDNA loci on three O. violaceus chromosomes were active and showed variable degrees of expression dominance over those of B. napus, i.e., the epigenetic phenomenon of nucleolar dominance (ND) (Chen, 2007). Some phenotypic deviations from the original B. napus genotype were presented by the self-pollinated B. napus-type progenies (2n = 38) from these MAALs, which were likely attributable to the alien genetic introgression from O. violaceus, to the genetic changes during the process of intergeneric hybridization/allopolyploidization/aneuploidization. In this study, the B. napus-type progenies derived from nine MAALs were investigated and compared by methods of AFLP, SSAP and MSAP, to quantify the genomic changes, retrotransposon mobilization and alterations in cytosine methylation. These results have provided novel insights into the genetic and epigenetic modifications that have persisted even after the genome was restituted from the allopolyploids.
Materials and Methods
Plant Materials
The intergeneric somatic hybrid No. 101 (2n = 62, genomes AACCOO) of Brassica napus L. cv. Huashuang No. 3 (2n = 38, genomes AACC) and O. violaceus (L.) O. E. Schulz (2n = 24, genome OO) was pollinated with Huashuang No. 3. The backcrossed progeny produced (2n = 50, genomes AACCO) was female sterile but male fertile (Zhao et al., 2008). Among the further backcrossed progenies (Huashuang No. 3 as the female parent), nine different monosomic (i.e., 2n = 39, AACC + 1O; where 1O represented a single O. violaceus chromosome) or disomic additional lines (i.e., 2n = 40, AACC + 2O; where 2O represented a pair of O. violaceus chromosomes) were established (designated as M1–M9) (Figure 1). Eight of them reported previously were distinguishable from each other on the basis of their phenotype and cytology (Ding et al., 2013). Five plants having the same number of chromosomes as B. napus (2n = 38) among the selfed progenies from each of these additional lines except the female sterile one M7 were identified to study the genetic changes induced during the process of allopolyploidization and aneuploidization, in comparison with parental B. napus. Because M7 was female sterile but male fertile, B. napus-type progenies were produced by pollinating the parental Huashuang No. 3. The selfed seeds of these B. napus-type plants were used to obtain the plants for DNA extraction, for the investigations of phenotype, fertility and seed quality. The parental Huashuang No. 3 was also selfed in each generation in parallel with the somatic hybrids and progenies.
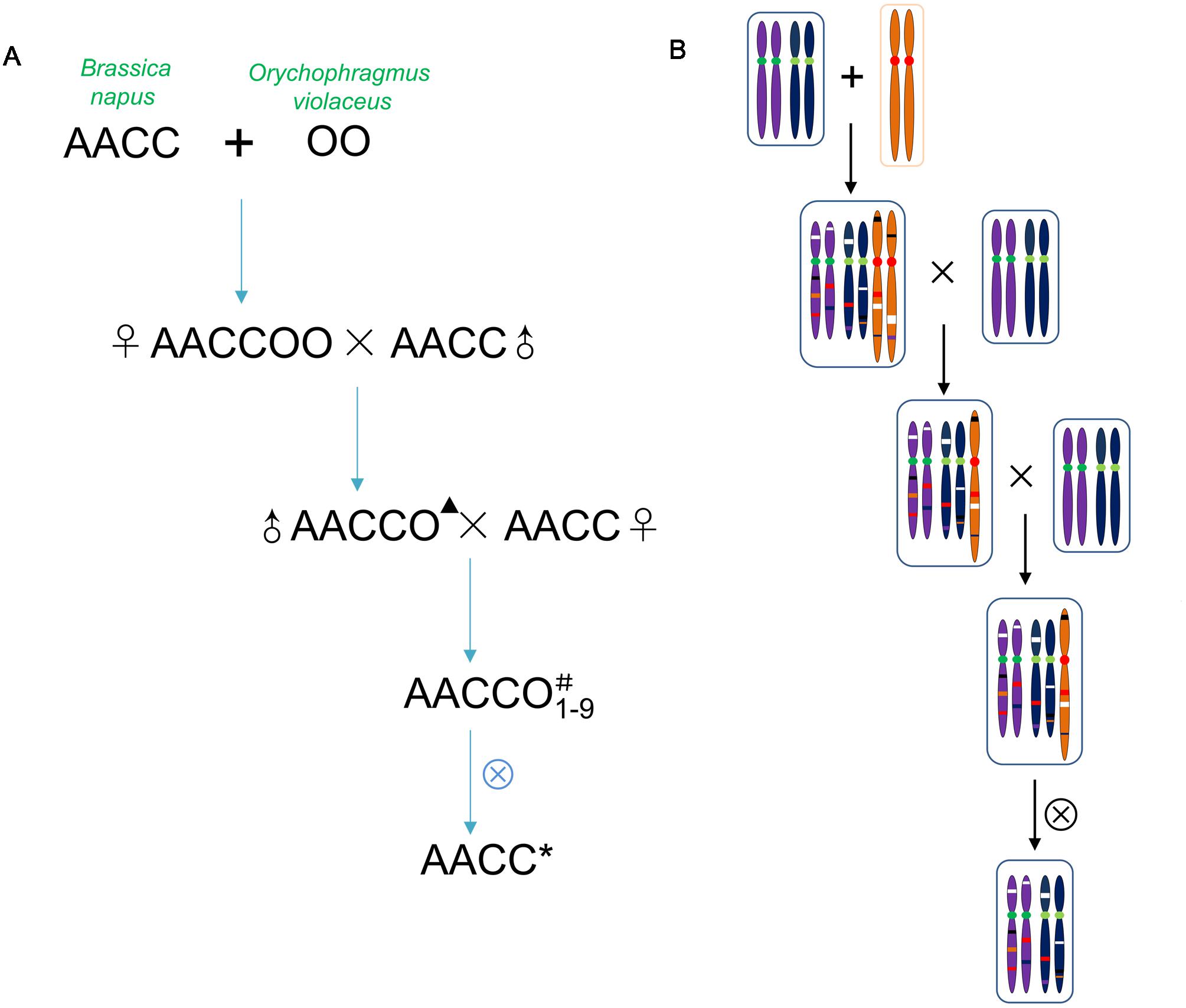
FIGURE 1. Extraction procedure of Brassica napus-type progeny from the allohexaploid (A) and presence of the genetic changes on the chromosomes (B). One pair of chromosomes for each genome was shown. The genetic changes include exchanged segments between genomes of two parents. The changes represented by different colors, lost bands (white), new bands (red) and the epigenetic changes (black). ▴Represented the haploid genome of O. violaceus. #Represented one chromosome of O. violaceus. ∗Represented the extracted B. napus with the genetic and the epigenetic changes.
Cytology and Pollen Fertility
The chromosome complement of 45 individuals of the B. napus-type progeny (2n = 38) raised from nine selfed addition lines was determined by chromosome counting of the ovaries from young flower buds and by the meiotic pairing and segregation in pollen mother cells (PMCs). The immature ovaries of young flower buds were treated with 2 mM 8-hydroxyquinoline for 3–4 h at room temperature and then fixed in acetic acid: ethanol 1:3 (v/v) and used for counting the number of chromosomes according to the procedure of Li et al. (1995). For studying meiotic analysis in PMCs, young flower buds were fixed and stored at -20°C. At anthesis, pollen samples were collected closed to noon time on sunny days and stained with 1% acetic carmine to score pollen stainability as an indicator of pollen fertility.
Amplified Fragment Length Polymorphisms
The AFLP procedure was performed with parent plants and with progenies resulting from selfing, according to the protocol of Vos et al. (1995). Genomic DNA was extracted from young fresh leaves by the cetyltrimethyl-ammonium bromide procedure (Kidwell and Osborn, 1992). In total, 32 pairs of AFLP primers (Supplementary Table S2) were randomly selected and used for AFLP fingerprint analysis. Each AFLP gel was run twice. The upper part and lower part of the gel, where resolution was not satisfactory, was not used for band scoring. Only high-resolution, middle-part of the gel, which was reproducible in all cases were used for scoring.
Sequence-Specific Amplification Polymorphisms
In the present investigation, the SSAP strategy was mainly used following the protocol of Pouilly et al. (2008) which was based on previously described protocols of Waugh et al. (1997) and Ellis et al. (1998) with some modifications (Zou et al., 2011; Zhang et al., 2013) to design the primer sequences (Supplementary Table S3). The endonuclease enzyme EcoRI was used to restrict genomic DNA, which was employed following ligation to adaptors, as the template for a two-step SSAP reaction including pre-amplification and selective amplification. We surveyed three contrasting Brassica transposons, Athila-like retrotransposon, BraSto, and Bot1.
Methylation-Sensitive Amplification Polymorphisms
The methylation-sensitive amplified fragment length polymorphism (MSAP) protocol (Xiong et al., 1999; Xu et al., 2000) was used as described by Zhang et al. (2013). The 23 pairs of randomly selected primers (Supplementary Table S4) were used to detect the DNA methylation alteration patterns; only the clear and reproducible bands were scored. MSAP involved the use of two isoschizomers, HpaII and MspI which can recognize the similar CCGG sites that have different sensitivity to the methylation states of the cytosine. HpaII is inactive if one or both strands of cytosine are fully methylated, but it cleaves hemi-methylated sequences (i.e., if only a single DNA strand is methylated), or neither strand is methylated; MspI digests methylation of double-stranded DNA, or non-methylated sequences. Based on the presence (+) or absence (-) of a band in the EcoRI/HpaII-digested (H) or EcoRI/MspI-digested (M) genomic DNA, the methylation status of the specific loci was decided.
Fatty Acid Analyses
Seed oil was extracted and its composition was analyzed using gas chromatography (HP 6890, Germany). The content of fatty acid and glucosinolate were estimated using near infrared reflectance spectroscopy (NIRS) (Vector 22/N, Bruker, Germany).
Statistical Analyses
Two-by-two chi-square contingency tests were used to test the significance of the genetic and the epigenetic changes among the B. napus-type progenies and the Pearson correlations were performed among the observed changes.
Results
Phenotype and Fertility of B. napus Plants Extracted from Alien Additions
Among the self-pollinated progenies of each of nine B. napus–O. violaceus addition lines (M1–M9) (Figure 1), five B. napus-type plants (2n = 38) were identified for further study by cytological investigations including, chromosome counting in the somatic cells and normal meiotic pairing (19 bivalents) and 19:19 segregation in PMCs. All these 45 plants showed high pollen fertility (90.5–96.8% stainability) and good seed set after selfing, while the parental B. napus cv. Huashuang No. 3 showed expectedly normal pollen fertility and seed-setting. They showed the phenotype similar to Huashuang No. 3, except for minor differences in leaf shape and color. Nine lines derived from the 45 plants largely maintained the phenotype of Huashuang No. 3, but some differences in shape and color of leaves, flowering time and fertility were discernible between the lines from the same or different additions. The individuals from M9 showed delayed flowering and low seed set. The selfed seeds of these nine lines exhibited low content of erucic acid (0.47–3.31%) and variable contents of glucosinolates (11.82–65.51 μmol/g ∼30 μmol/g), as in Huashuang No. 3 which also showed low contents of erucic acid (∼1%) and glucosinolates (∼30 μmol/g) in seeds.
AFLP Analysis of Genomic Changes in the Extracted B. napus Plants
The genomic components of these 45 B. napus-type individuals were analyzed using 32 primer combinations of EA (EcoRI) and MC (MseI). In all, 5,534 polymorphic bands were scored; this comprised 3,537 (63.9%) bands lost in the parent B. napus but present in the progenies, 937 (16.9%) new bands unobserved in both parent, and 1,060 (19.2%) bands specific to O. violaceus. The number of bands lost ranged from 13 in line M5 to 256 in line M6, that of the novel bands ranged from 4 in line M8 to 31 in line M3, and that of the O. violaceus-specific bands was from 6 in line M6 and M9 to 33 in line M3. The average of the lost bands (78) was much higher than the averages of the novel bands (21) and O. violaceus-specific bands (23). Among the five plants from the same addition line, the number and percentage of each kind of polymorphic band varied only narrowly for most additions, except the line M6 and M8; in M6 the number of the bands that were lost ranged from 60 to 256, and that of the O. violaceus-specific bands from 6 to 25. In M8 the number of the novel bands ranged from 4 to 24. These data indicated that the lost bands varied much more widely among the plants.
The average rates of all the three kinds of bands for the five plants within each addition showed no significant differences for M2, M3, M4, M5 (Supplementary Table S1). Compared to the M2–M5, the average rate of the lost band was significantly higher than the average rate of the new bands as well as that of the O. violaceus-specific bands in lines M1, M6, M8, and only significantly higher than that of new bands but not than that of O. violaceus-specific bands in line M7. Additionally, the average rate of the lost band was significantly higher than the average rate of the new bands as well as that of the O. violaceus-specific bands in lines M1, M6, M8, and M9, this possibly contributed to the higher total rates (2.58–3.40%) in these four lines. Unlike these four lines, the other four lines M2, M3, M4, and M7 were only marginally higher. Line M5 was an exception; the average rate of the lost band was lower than the average rate of other two types of bands (Supplementary Table S1). The plants from each addition presented the higher rate of lost band but lower rates of both new and specific bands (except for M5) than the others (1.46–1.76).
For the comparison of the average rates of lost bands among plants from different additions (Figure 2), the percentage for line M6 (2.92) was highest but showed no significant differences as compared to M1 (1.90), M8 (2.54), and M9 (2.40); however, these percentages were significantly higher than those of the remaining additions (0.46 for M5 to 0.72 for M3). For the comparison of novel bands, the rates for M2–M5, and M7 were comparable but were higher than those of M6, M8, and M9. As to the specific bands, the rates for M2–M5, and M7 were similar but significantly higher than those of M6 and M9.
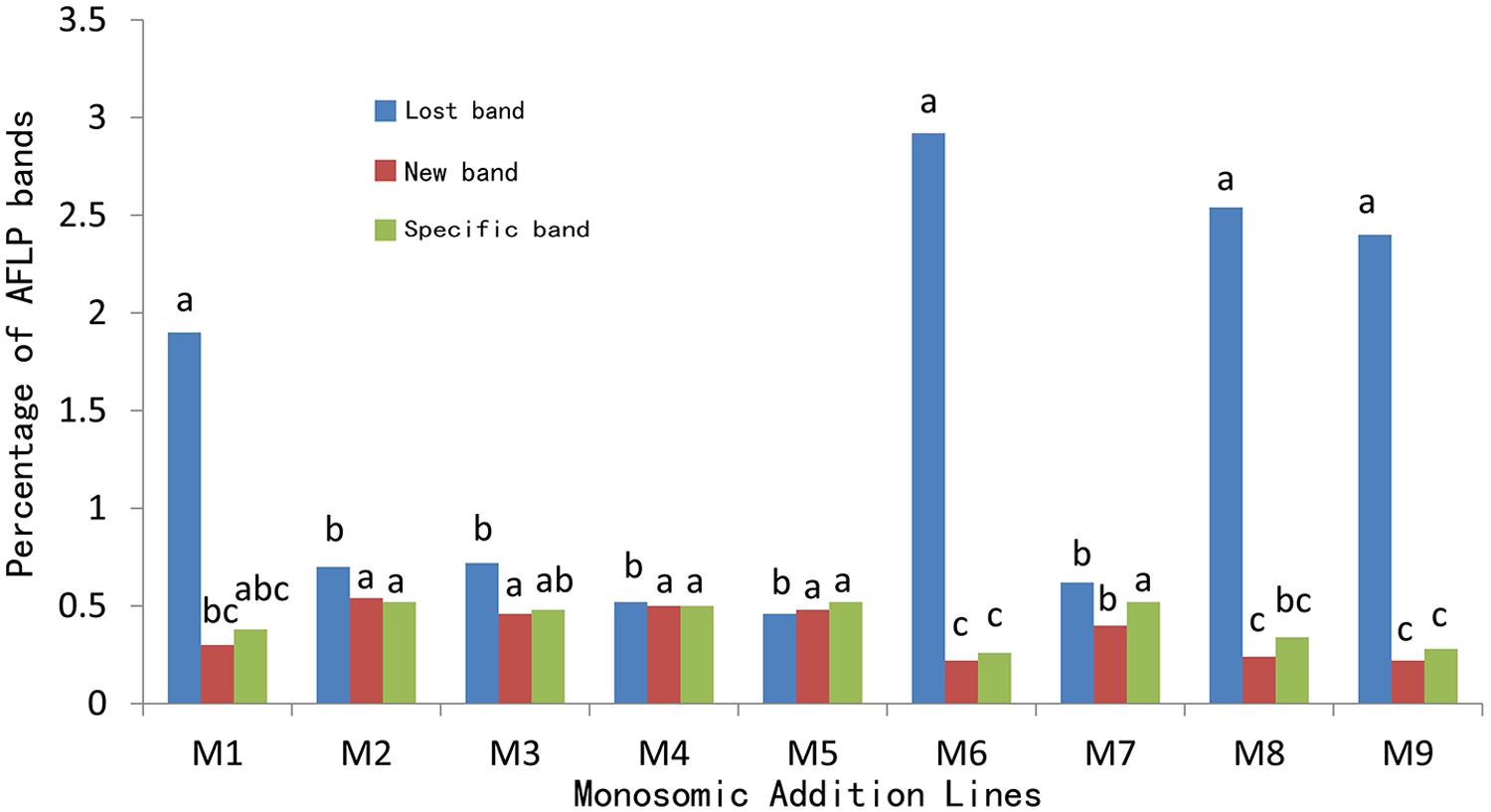
FIGURE 2. Average percentages of AFLP bands in the extracted B. napus-type plants. The percentages represented by the bars of the same color marked with identical letter (s) are not significant (p < 0.05).
SSAP Analysis of Transposon Variations in the Extracted B. napus Plants
Sequence-specific amplified polymorphism markers were used to detect the variations in three contrasting transposable elements, namely Athila-like retrotransposons, Bot1, and BraSto in the 45 B. napus-type individuals from the nine addition lines (Table 1). SSAP polymorphism appeared as lost bands as well as new bands, but the percent of the new bands was rather low; in fact no new band appeared for Bot1 in any progenies. Like the AFLP bands, the number of SSAP lost bands varied not only among the lines but also among the five individuals within a given lines. We found that the number of lost bands among the 45 plants varied from 1 to 46 for Athila-like, from 1 to 29 for Bot1, and from 1 to 12 for BraSto, and averaged 16, 7.5, 4.6, respectively. The widest range of the lost bands among the five plants in the same additions were 4–35 for Athila-like and 1–10 for BraSto, both in the line M8, and 7–29 for Bot1 in the line M6.
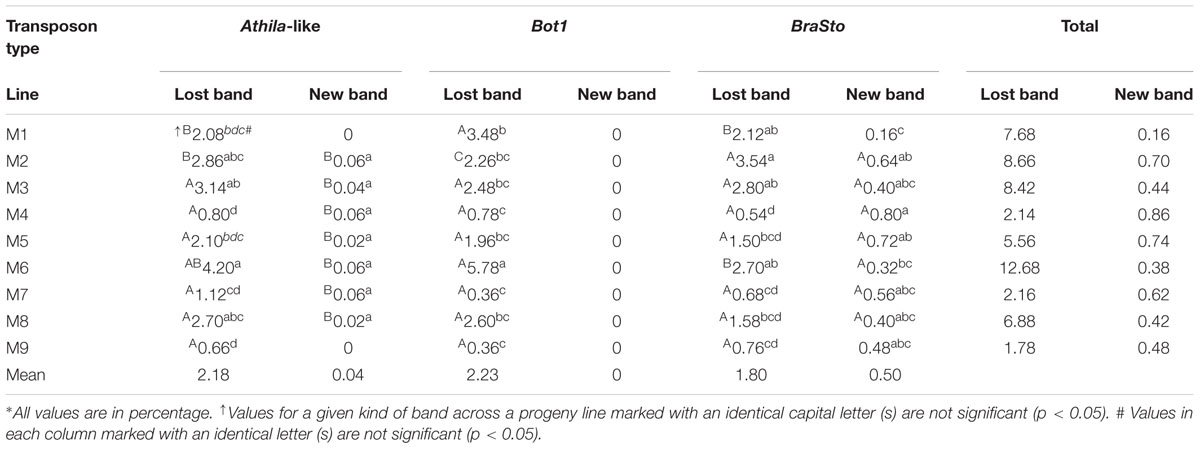
TABLE 1. Statistical analysis of the SSAP bands in Brassica napus-type progenies derived from nine additions∗.
The highest rate of the lost bands of the Athila-like transposons in M6 (4.2) was insignificant with the reference to the percentages in M2 (2.86), M3 (3.14), and M8 (2.70), but significantly higher than those of the remaining five lines, M1 (2.08), M4 (0.80), M5 (2.10), M7 (1.12), and M9 (0.66) (Table 1). The rates of the new bands of the Athila-like transposons were quite low (0–0.02) and had no obvious differences. Of the lost bands of Bot1, the percentage for M6 (5.78) was significantly higher than that of all other lines. The percentages for M1 (3.48), M2 (2.26), M3 (2.48), M5 (1.96), and M8 (2.60) were comparable. Likewise, only the percentage for M1 was significantly higher than those of M4 (0.78), M6 (0.36), and M9 (0.36). Of the lost bands of BraSto, although the percentage for the M2 (3.54) was the highest and it was not significantly higher than the percentages for the line M1 (2.12), M3 (2.80), and M6 (2.70), but it was significantly higher than the percentages for the remaining five lines, M4 (0.54), M5 (1.5), M7 (0.68), M8 (1.58), and M9 (0.76). When the new bands of BraSto were considered, the rates of the new bands of BraSto were low (0.16–0.80) and only the percentage for M4 (0.80) was significantly higher than those of M1 (0.16) and M6 (0.32). For the total rates of absent bands of these three transposons (1.78–12.68), M6 (12.68) was much higher than all others, and M1, M2, M3, M5, and M8 were ranged from 5.56 to 8.66 which was higher than M4, M7, and M9 having range between 1.78 and 2.14. The total rates of new bands of these three transposons were still low (0.16–0.86).
For the comparisons of the frequencies of the absent bands between three transposons within each addition (Table 1), the rate for Bot1 (3.48) in line M1 was significantly higher than those of Athila-like (2.08) and BraSto (2.12). Within line M2, the rate of BraSto (3.54) was significantly higher than that of Athila-like (2.86) which was also significantly higher than that of Bot1 (2.26). For M6, the rate of Bot1 (5.78) was significantly higher than that of BraSto (2.70) but not than that of Athila-like (4.20). Within the remaining six additions, there were no significant differences among three rates. As to the rates of new bands which occurred both for Athila-like and BraSto within seven additions, the rates of BraSto were significantly higher than those of Athila-like transposons.
MSAP Analysis of DNA Methylation Alterations in the Extracted B. napus Plants
As already mentioned, the MSAP protocol described by Zhang et al. (2013) was followed; 23 pairs of primers were used to detect the DNA methylation alteration patterns. The MSAP loci in these B. napus-type progenies were grouped into fifteen patterns and compared with those in the parent B. napus (Table 2). Fifteen patterns of methylation were recognized; three pattern namely ++/++, ++/-- and --/++ were excluded from the MSAP analysis as it was not possible to decide whether the loci were methylated (++/++) or they resulted from the methylation changes, or sequence variation (++/-- and --/++). The remaining 12 patterns were either monomorphic or polymorphic and were grouped into A, B, C, D types (Table 3); monomorphic pattern indicates similar pattern of methylation in the parent as well as the progenies in either HpaII (H) or MspI (M) lane. Three types of DNA methylation—hypermethylation (type A), hypomethylation (type B), and variable pattern of methylation (type C) were observed. Type A referred to the hypermethylation state in the parent B. napus, and Type B to the hypomethylation state in the progenies. Types A and B presented four subtypes each—A1 to A4 and B1 to B4. A1 was the de novo methylation in the parent B. napus, A2 was the de novo methylation in the progeny lines (in either HpaII or MspI lane), A3 was hypermethylation in the parent (in either HpaII or MspI lane), and A4 was the hypermethylation band in the progeny. The rates of Type A within additions were much higher than those of Type B, except for the reverse situation in M2. Therefore, the methylation changes were mainly observed in type A and type B (Table 3). Type C represented varied patterns of methylation; these patterns showed no difference in DNA methylation between the parent and the progenies. Type D showed three subtypes—D1 (no methylation), D2 (hemi-methylation), and D3 (hemi-methylation). The percentage of Type D varied from 41.20 to 66.0 among the 45 plants and showed an average of 53.08–62.42 among additions (Table 3); this suggested that the progeny lines inherited most of the methylation patterns from the parent B. napus. That the percentage for M2 (62.42) was significantly higher than that for M6 (55.32), or M8 (53.08), or M9 (54.96) which indicated the obvious difference in the maintenance of parental methylation situation. The percentage of hypermethylation ranged from 11.7 to 44.9 among the 45 plants and gave an average of 15.98 among the nine lines. The highest value (32.82%) for M8 had no significant differences with those of M6 (29.38%) and M9 (29.14%), but it was significantly higher than M1 (22.84%), M2 (15.98%), M3 (19.90%), M4 (19.96%), M5 (20.76%), and the latter five rates were comparable. The percentage of hypomethylation alterations ranged from 5.50 to 22.70 among the 45 plants and averaged 10.9–19.96 among the lines. The highest percentage for M2 (19.96) was comparable with those for M3 (16.14) and M4 (16.28), but was significantly higher than all the remaining six percentages. The low rates of C type averaged 2.24–5.06 showed no significant differences. The plants from lines M6, M8, and M9 showed higher methylation changes, while those from M2 changed least. This represented that the progenies from different addition lines gave obvious difference in increase or decrease in methylation levels.
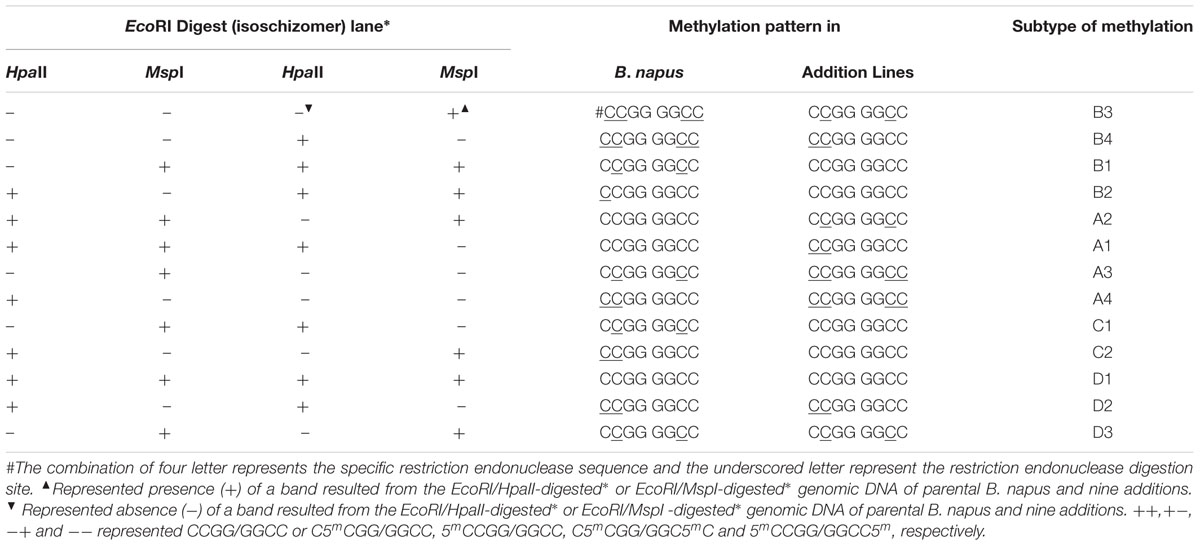
TABLE 2. Methylation-sensitive amplified polymorphism analysis of cytosine methylation patterns in the extracted B. napus.
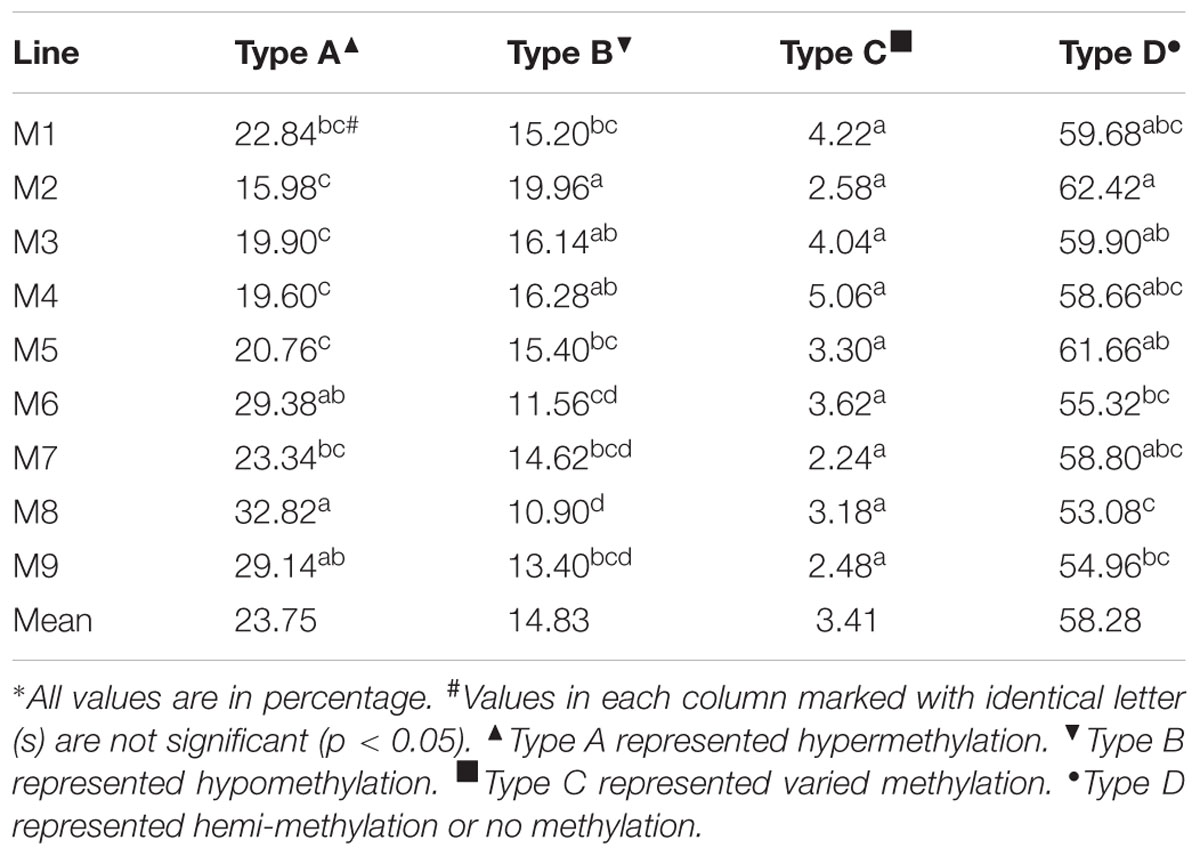
TABLE 3. Methylation-sensitive amplified polymorphism analysis of DNA methylation types in the extracted B. napus∗.
The correlation coefficients between AFLP and SSAP was 0.384 (p = 0.308), between AFLP and MSAP was 0.900 (p = 0.001), and between SSAP and MSAP was 0.057 (p = 0.80) (Figure 3). Therefore, the AFLP and MSAP values were significantly correlated, AFLP and SSAP changes were some but insignificantly correlated, while SSAP and MSAP changes were not correlated.
Discussion
Consequent to the merger of the genomes of B. napus and O. violaceus via somatic hybridization and repeated backcrossing, the genomes of the resultant B. napus-type progenies showed changes in the genomic components in terms of (i) losing and acquiring DNA segments and transposons, (ii) introgression of alien DNA segments, and (iii) DNA methylation (Figures 2 and 3, Tables 1 and 3), which were likely to be induced during the process of allopolyploidization and aneuploidization and to be maintained, even after the genomes became independent. The variations observed in the three types of the genomic changes named above among the B. napus-type individuals extracted from these addition lines (M1–M9) suggested the different effects of individual alien chromosomes from O. violaceus on inducing genetic alterations in the B. napus genomes. Nevertheless, the change rates obtained here should underestimate the actual changes, because the twice backcrossing of the allohexaploid (2n = 62, genomes AACCOO) with parental B. napus would diminish the actual alterations responding to the genome merger. The genomic alteration showed no significant correlation with transposon mobilization but found to have a “very strong positive correlation” with DNA methylation changes; no correlation between transposon mobilization and DNA methylation changes. It warrants further investigation to establish a causal link between the two variables showing a “strong positive correlation.”
Of the genomic changes, the extent of loss and gain of the DNA segments could be attributed to the two species involved in hybridization, the type of hybridization (sexual or somatic), and the number of hybridization events (Gaeta et al., 2007; Xiong et al., 2011). The hybridization pathways as well as the genetic and the epigenetic changes in the present materials were compared with the previous B. napus-type lines derived from sexual cross between B. napus cv. Oro and O. violaceus (Zhang et al., 2013). As the separation of parental genomes occurred during the mitotic divisions of the hybrids (Li et al., 1995; Li and Ge, 2007), the somatic cells with the chromosomes of either parental species entered meiosis, resulting in two types of progenies by self-pollination, mostly parental B. napus and a few mixoploid hybrids. The mixoploid hybrids that again showed the genome separation were successively selected for mixoploidy up to F5 generation. However, the altered chromosome behavior in one F5 plant led to the formation of F6 plants having variable chromosomal complements. Two F6 plants produced further progenies which were advanced to the F20 generation; the plants of the F20 have the karyotype of B. napus but with genomic changes. As a result, the genome of these B. napus-type progenies passed through several rounds of genome merger and separation, but no backcrossing was done for developing the progenies. The different crossing approach and pedigrees might have contributed to the greater genomic changes, for their average proportions of loss and gain were 7.2 and 5.4% (Zhang et al., 2013), much higher than observed in the present investigation 1.42 and 0.37% (Figure 2, Supplementary Table S1). The larger extent of genomic alteration from the sexual cross might be attributed to the occurrence of partial chromosomal complements in F6 progeny and the recovery of the B. napus-type in later generations. In contrast, the completeness of the chromosomal complement for B. napus was maintained in the pedigree from the allohexaploid backcross for the purpose of MAALs. Interestingly, the introgressions derived from the partial hybrids between B. napus and Capsella bursa-pastoris (Zhang et al., 2013), although the hybrids were backcrossed twice with the parent B. napus, revealed genetic and epigenetic changes similar to those in the hybrids from the sexual cross. Of common was that the introgressions were selected from both types of hybrids carrying partial complements of B. napus plus some alien chromosomes or even chromosomal segments; more genetic changes may be envisaged to occur via meiotic recombination during karyotype recovery. Although, the B. napus-type plants from the mixoploid hybrids expressed some traits specific for O. violaceus, but they had no detectable AFLP bands specific to O. violaceus, this was likely attributable to the limited number of primers used and the few sequences introgressed. Additionally, the separation of the genomes from the two species during mitotic divisions of the hybrid cells and subsequent inclusions of the genomes into different cells also decreased the chance for chromosomal exchanges. On the contrary, the sequential formations of the allopolyploid, backcrossing progenies and MAALs should induce introgression of alien DNA segments, although no meiotic homoeologous pairing of chromosomes were observed (Zhao et al., 2008; Ding et al., 2013).
The ‘Genome Shock’ hypothesis (McClintock, 1984) proposes that wide hybridization (including allopolyploidy) is associated with the activity of TEs (transposable elements). Studies on several allopolyploids have suggested that allopolyploidization not always trigger TE mobilization (Madlung et al., 2005; Beaulieu et al., 2009; Parisod et al., 2010a; Petit et al., 2010). Moreover, introduction of small amount of foreign chromatin or DNA has been shown to cause widespread misregulation of TEs, resulting in their mobilization in the genomes of both plants and animals (Remus et al., 1999; Shapiro, 2010). Athila-like reterotransposon, a class I TE, is 10 kb long (Alix and Heslop-Harrison, 2004), mainly concentrated in the pericentromeric regions of the chromosome and represented by c. 400 copies in Brassica oleracea (Alix et al., 2005). Activation of Athila retrotransposon was reported for Arabidopsis (Josefsson et al., 2006). Bot1, a class II CACTA transposon, is also 10 kb long and has c. 1500 copies in B. oleracea and has Brassica C-genome specificity and several rounds of amplification, whereas Brassica A genome is nearly devoid of Bot1 copies (Alix et al., 2008). Bot1 which represented 2.3% of the genome in B. oleracea played a major role in Brassica genome divergence and gene proliferation (Alix et al., 2008). Brassica miniature inverted-repeat transposable element (MITE) BraSto is 250 bp long, and preferentially located in gene space with c. 125 and c. 310 copies in B. oleracea and B. napus, respectively (Sarilar et al., 2011). Introgression of foreign DNA may lead to the mobilization of MITEs which likely affect gene expression and phenotypic variability (Nakazaki et al., 2003). Therefore, both Athila-like reterotransposon and Bot1 have the same length (10 kb) and are much longer than BraSto (250 bp). The average frequency of the SSAP lost bands for these three TEs was rather close (2.18% for Athila-like, 2.23% for Bot1, 1.80% for BraSto) in the B. napus-type individuals, while the average of the SSAP new bands for BraSto (0.50%) was higher than those of Athila-like (0.04%) and for Bot1 (0) (Table 1). Although Bot1 was distributed on C genome only, it was lost at similar rate as the other two TEs on A and C genome; this likely resulted from the higher copy number of Bot1. The lower values of the new bands for Athila-like reterotransposon and Bot1 might be associated with their longer length. In contrast to the SSAP changes of the nine LTR (Copia) retrotransposons identified in B. rapa in the B. napus extracted from the two sexual hybrids mentioned above, the rates were ∼1% lower for both lost and new bands, and the difference was not so obvious as in the AFLP bands. These results from sexual and somatic hybridizations suggested that the genome of B. napus responded to TE mobilization within limited ranges, upon genome merger and alien introgression.
DNA methylation perturbation is observed in various allopolyploids or in the F1 hybrids (Shaked et al., 2001; Lukens et al., 2006; Gaeta et al., 2007; Hegarty et al., 2011). Extensive and genome-wide alterations in DNA methylation patterns were associated with the integration of alien DNA into the host genome of plants and mammals (Remus et al., 1999; Wang et al., 2005). In the B. napus-type progenies from two sexual crosses mentioned above, the rates of methylation changes are similar (33.4–39.8%) and the hypermethylation was more frequent than hypomethylation. Coincidentally, the extent of the total and two degrees of methylation alterations were quite close to those in the present study (Table 2); this represented the general trend of methylation in the B. napus genome induced by interspecific hybridization and incorporation of alien DNA.
The ND in which the rRNA genes from one parent are expressed has been observed in many interspecific hybrids and their allopolyploid derivatives (Chen and Pikaard, 1997). Furthermore, the co-occurrence of ND with the phenotypic and transcriptomic dominance was shown in certain allopolyploids (Chen, 2007). Based on the suggestion that the ND and the genome-specific stability in natural and synthetic Brassica allotetraploids were related, it was proposed that ND possibly played a role in the stabilization of the chromosomes from the parent of the dominant rRNA genes (Ge et al., 2013), given that the rRNAs were the main component of the ribosome for protein synthesis. The rRNA genes from O. violaceus were completely dominant over those of B. napus in their allohexaploids (Ge et al., 2009). The genes on the O. violaceus chromosome in one addition line (M4) expressed complete dominance, while those carried by the alien chromosome in M5 and M6 showed partial dominance (Ding et al., 2013); M6 had the lowest transcription level. It appears that the expression levels of rRNA genes from O. violaceus in the three additions namely, M4, M5, and M6 were positively correlated with the genomic stability. The loss rates of ALFP bands in the B. napus-type plants from M4 (0.52%) and M5 (0.46%) were lowest among all rates and were significantly much lower than that of M6 (2.92%) (Figure 2, Supplementary Table S1). Notably, the rates of lost, new and O. violaceus-specific bands were very close within plants from M4 or M5, but the rate of loss was much higher than the rates of other two from M6. Similarly, the loss rates of three transposons were lowest in plants from M4 and were not significantly lower than those of M5, but the rates from both M4 and M5 were significantly lower than that of M6 (Table 1). The percentage of hypermethylation change in plants from M4 and M5 were quite similar but significantly lower than that of M6, and inversely, the percentage of hypomethylation change in plants from M4 were insignificantly higher than that of M5 but significantly higher than that of M6. The mechanism underlining the relationship between ND and genome stability warrants further investigation.
Conclusion
The extracted B. napus from the intergeneric allopolyploids and additions with another crucifer showed certain alterations in genomic components, retrotransposon mobilization, and DNA methylation. The result firstly indicated the genetic and the epigenetic changes of the genome from one parental species induced by the processes of allopolyploidization and aneuploidization, which were still maintained after the genome was extracted and the parent restituted. The possible effect of the nucleolar dominance on the genomic changes provided novel insights into the genome interaction in the allopolyploids.
Author Contributions
Conceived and design the experiment: ZL, XG, YS, MG. Performed the experiment: YD, MG. Analyzed the data: ZL, XG, MG, YD. Contributed reagent/material/analysis tool: MG, YD, XG. Write the paper: ZL, MG.
Funding
This work was supported by the National Natural Science foundation of China (Grant No. 31375656).
Conflict of Interest Statement
The authors declare that the research was conducted in the absence of any commercial or financial relationships that could be construed as a potential conflict of interest.
Acknowledgment
We thank Dr. Jing Wang for data analysis and Dr. Shengqian Xia for discussion.
Supplementary Material
The Supplementary Material for this article can be found online at: http://journal.frontiersin.org/article/10.3389/fpls.2016.00438
Abbreviations
AFLP, amplified fragment length polymorphism; MAALs, monosomic alien addition lines; MSAP, methylation-sensitive amplified polymorphism; SSAP, sequence-specific amplified polymorphism.
References
Adams, K. L., and Wendel, J. F. (2005). Polyploidy and genome evolution in plants. Curr. Opin. Plant Biol. 8, 135–141. doi: 10.1016/j.pbi.2005.01.001
Albertin, W., Balliau, T., Brabant, P., Chevre, A. M., Eber, F., Malosse, C., et al. (2006). Numerous and rapid nonstochastic modifications of gene products in newly synthesized Brassica napus allotetraploids. Genetics 173, 1101–1113. doi: 10.1534/genetics.106.057554
Alix, K., and Heslop-Harrison, J. S. (2004). The diversity of retroelements in diploid and allotetraploid Brassica species. Plant Mol. Biol. 54, 895–909. doi: 10.1007/s11103-004-0391-z
Alix, K., Joets, J., Ryder, C. D., Moore, J., Barker, G. C., Bailey, J. P., et al. (2008). The CACTA transposon Bot1 played a major role in Brassica genome divergence and gene proliferation. Plant J. 56, 1030–1044. doi: 10.1111/j.1365-313X.2008.03660
Alix, K., Ryder, C. D., Moore, J., King, G. J., and Pat Heslop-Harrison, J. S. (2005). The genomic organization of retrotransposons in Brassica oleracea. Plant Mol. Biol. 59, 839–851. doi: 10.1007/s11103-005-1510-1
Beaulieu, J., Jean, M., and Belzile, F. (2009). The allotetraploid Arabidopsis thaliana-Arabidopsis lyrata subsp. petraea as an alternative model system for the study of polyploidy in plants. Mol. Genet. Genomics 281, 421–435. doi: 10.1007/s00438-008-0421-7
Chalhoub, B., Denoeud, F., Liu, S., Parkin, I. A., Tang, H., Wang, X., et al. (2014). Early allopolyploid evolution in the post-Neolithic Brassica napus oilseed genome. Science 345, 950–953. doi: 10.1126/science.1253435
Chen, Z. J. (2007). Genetic and epigenetic mechanisms for gene expression and phenotypic variation in plant polyploids. Annu. Rev. Plant Biol. 58, 377–406. doi: 10.1146/annurev.arplant.58.032806.103835
Chen, Z. J., and Pikaard, C. S. (1997). Transcriptional analysis of nucleolar dominance in polyploid plants: biased expression/silencing of progenitor rRNA genes is developmentally regulated in Brassica. Proc. Natl. Acad. Sci. U.S.A. 94, 3442–3447. doi: 10.1073/pnas.94.7.3442
Comai, L. (2005). The advantages and disadvantages of being polyploid. Nat. Rev. Genet. 6, 836–846. doi: 10.1038/nrg1711
Crasta, K., Ganem, N. J., Dagher, R., Lantermann, A. B., Ivanova, E. V., Pan, Y., et al. (2012). DNA breaks and chromosome pulverization from errors in mitosis. Nature 482, 53–58. doi: 10.1038/nature10802
Cui, C., Ge, X., Gautam, M., Kang, L., and Li, Z. (2012). Cytoplasmic and genomic effects on meiotic pairing in Brassica hybrids and allotetraploids from pair crosses of three cultivated diploids. Genetics 191, 725–738. doi: 10.1534/genetics.112.140780
Cui, C., Ge, X., Zhou, Y., Li, M., and Li, Z. (2013). Cytoplasmic and genomic effects on non-meiosis-driven genetic changes in Brassica hybrids and allotetraploids from pairwise crosses of three cultivated diploids. PLoS ONE 8:e65078. doi: 10.1371/journal.pone.0065078
Ding, L., Zhao, Z. G., Ge, X. H., and Li, Z. Y. (2013). Intergeneric addition and substitution of Brassica napus with different chromosomes from Orychophragmus violaceus: phenotype and cytology. Sci. Hortic. 164, 303–309. doi: 10.1016/j.scienta.2013.09.043
Doyle, J. J., Flagel, L. E., Paterson, A. H., Rapp, R. A., Soltis, D. E., Soltis, P. S., et al. (2008). Evolutionary genetics of genome merger and doubling in plants. Annu. Rev. Genet. 42, 443–461. doi: 10.1146/annurev.genet.42.110807.091524
Ellis, T. H., Poyser, S. J., Knox, M. R., Vershinin, A. V., and Ambrose, M. J. (1998). Polymorphism of insertion sites of Ty1-copia class retrotransposons and its use for linkage and diversity analysis in pea. Mol. Gen. Genet. 260, 9–19. doi: 10.1007/PL00008630
Gaeta, R. T., Pires, J. C., Iniguez-Luy, F., Leon, E., and Osborn, T. C. (2007). Genomic changes in resynthesized Brassica napus and their effect on gene expression and phenotype. Plant Cell 19, 3403–3417. doi: 10.1105/tpc.107.054346
Gaeta, R. T., Yoo, S. Y., Pires, J. C., Doerge, R. W., Chen, Z. J., and Osborn, T. C. (2009). Analysis of gene expression in resynthesized Brassica napus Allopolyploids using Arabidopsis 70mer oligo microarrays. PLoS ONE 4:e4760. doi: 10.1371/journal.pone.0004760
Ge, X. H., Ding, L., and Li, Z. Y. (2009). Different genome-specific chromosome stabilities in synthetic Brassica allohexaploids revealed by wide crosses with Orychophragmus. Ann. Bot. 104, 19–31. doi: 10.1093/aob/mcp099
Ge, X. H., Ding, L., and Li, Z. Y. (2013). Nucleolar dominance and different genome behaviors in hybrids and allopolyploids. Plant Cell Rep. 32, 1661–1673. doi: 10.1007/s00299-013-1475-5
Geleta, M., Heneen, W. K., Stoute, A. I., Muttucumaru, N., Scott, R. J., King, G. J., et al. (2012). Assigning Brassica microsatellite markers to the nine C-genome chromosomes using Brassica rapa var. trilocularis-B. oleracea var. alboglabra monosomic alien addition lines. Theor. Appl. Genet. 125, 455–466. doi: 10.1007/s00122-012-1845-3
Hegarty, M. J., Batstone, T., Barker, G. L., Edwards, K. J., Abbott, R. J., and Hiscock, S. J. (2011). Nonadditive changes to cytosine methylation as a consequence of hybridization and genome duplication in Senecio (Asteraceae). Mol. Ecol. 20, 105–113. doi: 10.1111/j.1365-294X.2010.04926
Janssen, A., van der Burg, M., Szuhai, K., Kops, G. J., and Medema, R. H. (2011). Chromosome segregation errors as a cause of DNA damage and structural chromosome aberrations. Science 333, 1895–1898. doi: 10.1126/science.1210214
Josefsson, C., Dilkes, B., and Comai, L. (2006). Parent-dependent loss of gene silencing during interspecies hybridization. Curr. Biol. 16, 1322–1328. doi: 10.1016/j.cub.2006.05.045
Kang, L., Du, X., Zhou, Y. Y., Zhu, B., Ge, X. H., and Li, Z. Y. (2014). Development of a complete set of monosomic alien addition lines between Brassica napus and Isatis indigotica (Chinese woad). Plant Cell Rep. 33, 1355–1364. doi: 10.1007/s00299-014-1621-8
Kidwell, K. K., and Osborn, T. C. (1992). “Simple plant DNA isolation procedures,” in Plant Genomes: Methods for Genetic and Physical Mapping, eds J. S. Beckmann and T. C. Osborn (Dordrecht: Kluwer Academic Publishers), 1–13.
Ksiazczyk, T., Kovarik, A., Eber, F., Huteau, V., Khaitova, L., Tesarikova, Z., et al. (2011). Immediate unidirectional epigenetic reprogramming of NORs occurs independently of rDNA rearrangements in synthetic and natural forms of a polyploid species Brassica napus. Chromosoma 120, 557–571. doi: 10.1007/s00412-011-0331-z
Letourneau, A., Santoni, F. A., Bonilla, X., Sailani, M. R., Gonzalez, D., Kind, J., et al. (2014). Domains of genome-wide gene expression dysregulation in Down’s syndrome. Nature 508, 345–350. doi: 10.1038/nature13200
Li, A., Liu, D., Wu, J., Zhao, X., Hao, M., Geng, S., et al. (2014). mRNA and small RNA transcriptomes reveal insights into dynamic homoeolog regulation of allopolyploid heterosis in nascent hexaploid wheat. Plant Cell 26, 1878–1900. doi: 10.1105/tpc.114.124388
Li, Z. Y., and Ge, X. H. (2007). Unique chromosome behavior and genetic control in Brassica ×Orychophragmus wide hybrids: a review. Plant Cell Rep. 26, 701–710. doi: 10.1007/s00299-006-0290-7
Li, Z. Y., Liu, H. L., and Luo, P. (1995). Production and cytogenetics of intergeneric hybrids between Brassica napus and Orychophragmus violaceus. Theor. Appl. Genet. 91, 131–136. doi: 10.1007/bf00220869
Lukens, L. N., Pires, J. C., Leon, E., Vogelzang, R., Oslach, L., and Osborn, T. (2006). Patterns of sequence loss and cytosine methylation within a population of newly resynthesized Brassica napus allopolyploids. Plant Physiol. 140, 336–348. doi: 10.1104/pp.105.066308
Madlung, A., Tyagi, A. P., Watson, B., Jiang, H., Kagochi, T., Doerge, R. W., et al. (2005). Genomic changes in synthetic Arabidopsis polyploids. Plant J. 41, 221–230. doi: 10.1111/j.1365-313X.2004.02297
Makarevitch, I., and Harris, C. (2010). Aneuploidy causes tissue-specific qualitative changes in global gene expression patterns in maize. Plant Physiol. 152, 927–938. doi: 10.1104/pp.109.150466
McClintock, B. (1984). The significance of responses of the genome to challenge. Science 226, 792–801. doi: 10.1126/science.15739260
Moore, R. C., and Purugganan, M. D. (2005). The evolutionary dynamics of plant duplicate genes. Curr. Opin. Plant Biol. 8, 122–128. doi: 10.1016/j.pbi.2004.12.001
Nakazaki, T., Okumoto, Y., Horibata, A., Yamahira, S., Teraishi, M., Nishida, H., et al. (2003). Mobilization of a transposon in the rice genome. Nature 421, 170–172. doi: 10.1038/nature01219
Parisod, C., Alix, K., Just, J., Petit, M., Sarilar, V., Mihiri, C., et al. (2010a). Impact of transposable elements on the organization and function of allopolyploid genomes. New Phytol. 186, 37–45. doi: 10.1111/j.1469-8137.2009.03096
Parisod, C., Holderegger, R., and Brochmann, C. (2010b). Evolutionary consequences of autopolyploidy. New Phytol. 186, 5–17. doi: 10.1111/j.1469-8137.2009.03142
Petit, M., Guidat, C., Daniel, J., Denis, E., Montoriol, E., Bui, Q. T., et al. (2010). Mobilization of retrotransposons in synthetic allotetraploid tobacco. New Phytol. 186, 135–147. doi: 10.1111/j.1469-8137.2009.03140
Pontes, O., Neves, N., Silva, M., Lewis, M. S., Madlung, A., Comai, L., et al. (2004). Chromosomal locus rearrangements are a rapid response to formation of the allotetraploid Arabidopsis suecica genome. Proc. Natl. Acad. Sci. U.S.A. 101, 18240–18245. doi: 10.1073/pnas.0407258102
Pouilly, N., Delourme, R., Alix, K., and Jenczewski, E. (2008). Repetitive sequence-derived markers tag centromeres and telomeres and provide insights into chromosome evolution in Brassica napus. Chromosome Res. 16, 683–700. doi: 10.1007/s10577-008-1219-5
Prakash, S., Bhat, S. R., Quiros, C. F., Kirti, P. B., and Chopra, V. L. (2009). Brassica and its close allies: cytogenetics and evolution. Plant Breed. Rev. 31, 121–187.
Ramsey, J., and Schemske, D. W. (1998). Pathways, mechanisms, and rates of polyploid formation in flowering plants. Annu. Rev. Ecol. Syst. 29, 467–501. doi: 10.2307/221716
Ramsey, J., and Schemske, D. W. (2002). Neopolyploidy in flowering plants. Annu. Rev. Ecol. Syst. 33, 589–639. doi: 10.2307/3069274
Remus, R., Kammer, C., Heller, H., Schmitz, B., Schell, G., and Doerfler, W. (1999). Insertion of foreign DNA into an established mammalian genome can alter the methylation of cellular DNA sequences. J. Virol. 73, 1010–1022.
Sarilar, V., Marmagne, A., Brabant, P., Joets, J., and Alix, K. (2011). BraSto, a Stowaway MITE from Brassica: recently active copies preferentially accumulate in the gene space. Plant Mol. Biol. 77, 59–75. doi: 10.1007/s11103-011-9794-9
Shaked, H., Kashkush, K., Ozkan, H., Feldman, M., and Levy, A. A. (2001). Sequence elimination and cytosine methylation are rapid and reproducible responses of the genome to wide hybridization and allopolyploidy in wheat. Plant Cell 13, 1749–1759. doi: 10.2307/3871316
Shapiro, J. A. (2010). Mobile DNA and evolution in the 21st century. Mob. DNA 1, 4. doi: 10.1186/1759-8753-1-4
Siegel, J. J., and Amon, A. (2012). New insights into the troubles of aneuploidy. Annu. Rev. Cell Dev. Biol. 28, 189–214. doi: 10.1146/annurev-cellbio-101011-155807
Song, K., Lu, P., Tang, K., and Osborn, T. C. (1995). Rapid genome change in synthetic polyploids of Brassica and its implications for polyploid evolution. Proc. Natl. Acad. Sci. U.S.A. 92, 7719–7723. doi: 10.1073/pnas.92.17.7719
Vos, P., Hogers, R., Bleeker, M., Reijans, M., van de Lee, T., Hornes, M., et al. (1995). AFLP: a new technique for DNA fingerprinting. Nucleic Acids Res. 23, 4407–4414. doi: 10.1093/nar/23.21.4407
Wang, X., Shi, X., Hao, B., Ge, S., and Luo, J. (2005). Duplication and DNA segmental loss in the rice genome: implications for diploidization. New Phytol. 165, 937–946. doi: 10.1111/j.1469-8137.2004.01293
Waugh, R., McLean, K., Flavell, A. J., Pearce, S. R., Kumar, A., Thomas, B. B., et al. (1997). Genetic distribution of Bare-1-like retrotransposable elements in the barley genome revealed by sequence-specific amplification polymorphisms (S-SAP). Mol. Gen. Genet. 253, 687–694. doi: 10.1007/s004380050372
Xiong, L., Xu, C., Maroof, M. S., and Zhang, Q. (1999). Patterns of cytosine methylation in an elite rice hybrid and its parental lines, detected by a methylation-sensitive amplification polymorphism technique. Mol. Gen. Genet. 261, 439–446. doi: 10.1007/s004380050986
Xiong, Z. Y., Gaeta, R. T., and Pires, J. C. (2011). Homoeologous shuffling and chromosome compensation maintain genome balance in resynthesized allopolyploid Brassica napus. Proc. Natl. Acad. Sci. U.S.A. 108, 7908–7913. doi: 10.1073/pnas.1014138108
Xu, M., Li, X., and Korban, S. (2000). AFLP-Based detection of DNA methylation. Plant Mol. Biol. Rep. 18, 361–368. doi: 10.1007/BF02825064
Xu, Y. H., Zhong, L., Wu, X. M., Fang, X. P., and Wang, J. B. (2009). Rapid alterations of gene expression and cytosine methylation in newly synthesized Brassica napus allopolyploids. Planta 229, 471–483. doi: 10.1007/s00425-008-0844-8
Zhang, X., Ge, X., Shao, Y., Sun, G., and Li, Z. (2013). Genomic change, retrotransposon mobilization and extensive cytosine methylation alteration in Brassica napus introgressions from two intertribal hybridizations. PLoS ONE 8:e56346. doi: 10.1371/journal.pone.0056346
Zhao, Z. G., Hu, T. T., Ge, X. H., Du, X. Z., Ding, L., and Li, Z. Y. (2008). Production and characterization of intergeneric somatic hybrids between Brassica napus and Orychophragmus violaceus and their backcrossing progenies. Plant Cell Rep. 27, 1611–1621. doi: 10.1007/s00299-008-0582-1
Zhou, R., Moshgabadi, N., and Adams, K. L. (2011). Extensive changes to alternative splicing patterns following allopolyploidy in natural and resynthesized polyploids. Proc. Natl. Acad. Sci. U.S.A. 108, 16122–16127. doi: 10.1073/pnas.1109551108
Zhu, B., Shao, Y., Pan, Q., Ge, X., and Li, Z. (2015). Genome-wide gene expression perturbation induced by loss of C2 chromosome in allotetraploid Brassica napus L. Front. Plant Sci. 6:763. doi: 10.3389/fpls.2015.00763
Zou, J., Fu, D. H., Gong, H. H., Qian, W., Pires, J. C., Li, R., et al. (2011). De novo genetic variation associated with retrotransposon activation, genomic rearrangements and trait variation in a recombinant inbred line population of Brassica napus derived from interspecific hybridization with Brassica rapa. Plant J. 68, 212–224. doi: 10.1111/j.1365-313X.2011.04679
Keywords: allopolyploidization, monosomic additions, transposons, cytosine methylation, Brassica napus
Citation: Gautam M, Dang Y, Ge X, Shao Y and Li Z (2016) Genetic and Epigenetic Changes in Oilseed Rape (Brassica napus L.) Extracted from Intergeneric Allopolyploid and Additions with Orychophragmus. Front. Plant Sci. 7:438. doi: 10.3389/fpls.2016.00438
Received: 16 October 2015; Accepted: 21 March 2016;
Published: 12 April 2016.
Edited by:
Soren K. Rasmussen, University of Copenhagen, DenmarkReviewed by:
Zhongyun Piao, Shenyang Agricultural University, ChinaTomasz Ksikażczyk, Institute of Plant Genetics of the Polish Academy of Sciences, Poland
Copyright © 2016 Gautam, Dang, Ge, Shao and Li. This is an open-access article distributed under the terms of the Creative Commons Attribution License (CC BY). The use, distribution or reproduction in other forums is permitted, provided the original author(s) or licensor are credited and that the original publication in this journal is cited, in accordance with accepted academic practice. No use, distribution or reproduction is permitted which does not comply with these terms.
*Correspondence: Yujiao Shao, c3lqc3lqNTIwQDEyNi5jb20=; Zaiyun Li, bGl6YWl5dW5AbWFpbC5oemF1LmVkdS5jbg==
†These authors have contributed equally to this work.