- Department of Ornamental Horticulture, College of Horticulture, Gansu Agricultural University, Lanzhou, China
Hydrogen peroxide (H2O2), as a reactive oxygen species, is widely generated in many biological systems. It has been considered as an important signaling molecule that mediates various physiological and biochemical processes in plants. Normal metabolism in plant cells results in H2O2 generation, from a variety of sources. Also, it is now clear that nitric oxide (NO) and calcium (Ca2+) function as signaling molecules in plants. Both H2O2 and NO are involved in plant development and abiotic responses. A wide range of evidences suggest that NO could be generated under similar stress conditions and with similar kinetics as H2O2. The interplay between H2O2 and NO has important functional implications to modulate transduction processes in plants. Moreover, close interaction also exists between H2O2 and Ca2+ in response to development and abiotic stresses in plants. Cellular responses to H2O2 and Ca2+ signaling systems are complex. There is quite a bit of interaction between H2O2 and Ca2+ signaling in responses to several stimuli. This review aims to introduce these evidences in our understanding of the crosstalk among H2O2, NO, and Ca2+ signaling which regulates plant growth and development, and other cellular and physiological responses to abiotic stresses.
Introduction
Hydrogen peroxide (H2O2), a form of reactive oxygen species, is regarded as a common cellular metabolite. H2O2 is continually synthesized through various sources including enzyme and non-enzyme pathways in plants. To date, it has become accepted that H2O2 plays important roles in plant developmental and physiological processes including seed germination (Barba-Espín et al., 2011), programmed cell death (PCD; Cheng et al., 2015; Vavilala et al., 2015), senescence (Liao et al., 2012b), flowering (Liu et al., 2013), root system development (Liao et al., 2009; Ma et al., 2014; Hernández-Barrera et al., 2015), stomatal aperture regulation (Ge et al., 2015) and many others. It is now clear that H2O2 functions as a signaling molecule which may respond to various stimuli in plant cells. These results suggest that H2O2 may be involved in cellular signaling transduction pathways and gene expression modulations in plants.
Nitric oxide (NO), as a small signaling molecule, appears to be involved in plant developmental and physiological processes such as seed germination (Wang et al., 2015), ripening and senescence (Shi Y. et al., 2015) as well as stomatal closure (Shi K. et al., 2015) and pollen tube growth (Wang et al., 2009). Meanwhile, NO signaling may have a vital role in the disease resistance (Kovacs et al., 2015) and response to abiotic stresses such as cold (Fan et al., 2015), salt (Liu W. et al., 2015) and drought (Shan et al., 2015). Calcium ion (Ca2+) signaling is also a core regulator of plant physiological process and stress adaption such as cell polarity regulation (Zhou et al., 2014), leaf de-etiolation (Huang et al., 2012), stomatal closure (Zou et al., 2015). Additionally, Ca2+ signaling is also involved in various responses to abiotic stimuli, including light (Hu et al., 2015) and heavy metal (Li et al., 2016).
A large amount of research show that H2O2, NO and Ca2+ as signaling are involved in plant growth and development as well as response to abiotic stresses. In this review, we focus on H2O2 signaling activities and its cross-talk with Ca2+ and NO in plants.
H2O2 Homeostasis
H2O2 Generation
H2O2 is a byproduct of aerobic metabolism in plants (Mittler, 2002). Figure 1 shows that H2O2 in plants can be synthesized either enzymatically or non-enzymatically. There are numerous routes of H2O2 production in plant cells, such as photorespiration, electron transport chains (ETC), and redox reaction.
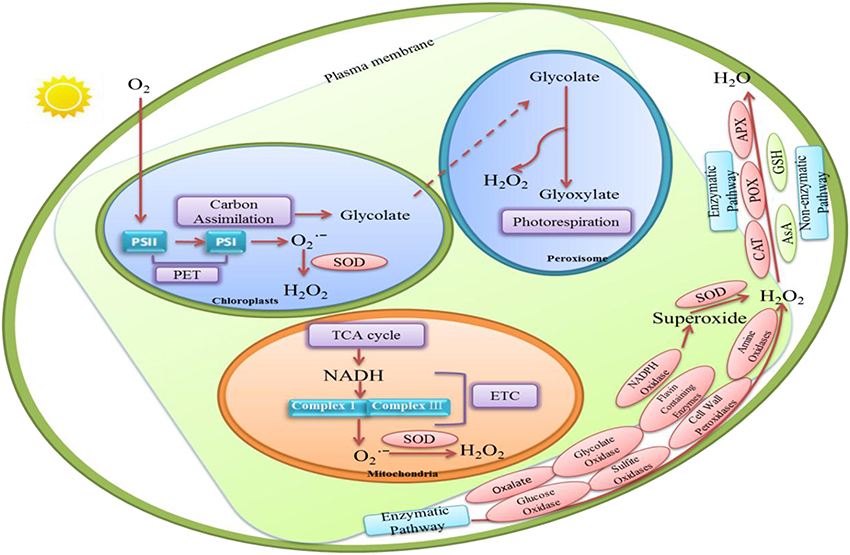
Figure 1. The various routes of hydrogen perioxide (H2O2) production and H2O2 removal in plant cells. Enzymatic production of H2O2 in plants requires several enzymes including cell wall peroxidases (Francoz et al., 2015), oxalate (Hu et al., 2003), amine oxidases and flavin-containing enzymes (Cona et al., 2006), glucose oxidases, glycolate oxidases (Chang and Tang, 2014), and sulfite oxidases (Brychkova et al., 2012). In these enzymes, some of them may convert O to H2O2 and O2. And others may oxidize their each substrates to generate H2O2in biocatalysis processes. Several non-enzymatic reactions are also known to produce H2O2. In peroxisome, H2O2 synthesis is associated with glycolate oxidation during photosynthetic carbon oxidation cycle (Foyer and Noctor, 2003). In chloroplasts, H2O2 production can be produced by the reduction of O by photosynthetic electron transport (PET) chain. H2O2 in chloroplast also may be detected at the manganese-containing, oxygen evolving complex which is the donor site of photosystem II. Moreover, H2O2 could be generated in mitochondria through aerobic respiration because O is produced from complexes I and III in the electron transport chain. H2O2-scavenging enzymes include catalase (CAT; Willekens et al., 1997), peroxidase (POX; Fan and Huang, 2012), ascorbate peroxidase (APX) and glutathione reductase (GR; Jahan and Anis, 2014). In non-enzymatic pathway, Ascorbate (AsA) and glutathione (GSH) are responsible for decreasing H2O2 level (Kapoor et al., 2015).
There is evidence for H2O2 production in plants through several enzymes includingcell wall peroxidases (Francoz et al., 2015), oxalate (Hu et al., 2003), amine oxidases and flavin-containing enzymes (Cona et al., 2006; Figure 1). Moreover, nicotinamide adenine dinucleotide phosphate (NADPH) oxidases may also increase H2O2 level through generating superoxide which could be converted to H2O2 by superoxide dismutases (SOD; Grivennikova and Vinogradov, 2013; Brewer et al., 2015). Remans et al. (2010) observed that ROS accumulation, especially H2O2 formation, is mostly related with the stimulation of NADPH oxidase in plants under heavy metal stresses. Moreover, H2O2 produced by NADPH oxidases may significantly increase proline accumulation in Arabidopsis thaliana under salt or mannitol stress (Ben Rejeb et al., 2015). Additionally, some other oxidases such as glucose oxidases, glycolate oxidases (Chang and Tang, 2014), and sulfite oxidases (Brychkova et al., 2012) may oxidize their own substrates to produce H2O2 (Figure 1).
Several non-enzymatic reactions are also known to produce H2O2. For example, many reactions involved in photosynthesis and respiration are responsible for H2O2 production. It is generated continually via electron transport reactions both in mitochondria and chloroplasts (Figure 1).
Peroxisomes
Peroxisome is considered to be the site of photorespiration in plant cell, which needs light-dependent uptake of O2 and releases CO2 accompanying with the generation of H2O2. It is suggested that H2O2 synthesis is associated with the oxidation of glycolate during the photosynthetic carbon oxidation cycle (Foyer and Noctor, 2003; Figure 1).
Chloroplasts
Chloroplast is the source of photosynthesis in plants. Chloroplasts are the crucial sites for H2O2 production during photosynthesis. H2O2 generation is associated with oxygen reduction in chloroplast (Figure 1). Mehler (1951) discovered that reduction of O2 lead to the formation of H2O2 in the presence of light in chloroplast. Moreover, H2O2 production can also be produced by the reduction of O by photosynthetic electron transport (PET) chain components such as Fe–S centers, reduced thioredoxin (TRX), ferredoxin and reduced plastoquinone in the chloroplast (Dat et al., 2000). In addition, non-enzymatic production of H2O2 in chloroplast may be detected at the manganese-containing, oxygen evolving complex which is the donor site of photosystem II (Figure 1). But this process, in most cases, may probably be ignored under physiological conditions.
Mitochondria
One important source of endogenously produced H2O2 in plant cell is mitochondria (Dickinson and Chang, 2011). H2O2 is generated in mitochondria during aerobic respiration when O is produced from complexes I and III in the electron transport chain, which is then rapidly converted to H2O2 by the enzyme superoxide dismutase (Figure 1).
H2O2 Removal
The antioxidant systems that regulate H2O2 levels consist of both non-enzymatic and enzymatic H2O2 scavengers (Figure 1). H2O2-scavenging enzymes include catalase (CAT; Willekens et al., 1997), peroxidase (POX; Fan and Huang, 2012), ascorbate peroxidase (APX) and glutathione reductase (GR; Jahan and Anis, 2014). Some studies revealed that APX was found in the cytosol (Begara-Morales et al., 2013), chloroplasts (Asada, 2006), and mitochondria (Navrot et al., 2007). Meanwhile, CAT can decompose H2O2 in peroxisome (Nyathi and Baker, 2006). It is quite clear that these enzymes exist in different organelles and they might decrease H2O2 content efficiently and maintain the stability of membranes.
Ascorbate (AsA) and glutathione (GSH), as non-enzymatic compounds, are constantly participated in regulating ROS level (Kapoor et al., 2015). AsA, a key antioxidant for elimination of H2O2, can react with H2O2 directly. GSH is a crucial antioxidant which may be associated with regenerating AsA, and rapidly oxidizes excess H2O2. Therefore, GSH is also involved in regulating H2O2 level and redox balance in plant cells (Krifka et al., 2012). In fact, H2O2 homeostasis seems to result in some biological effects on plant cells which may be as a signaling sign in signaling transduction pathway.
Responses to H2O2
Growth and Development
Table 1 shows that H2O2 mediates various developmental and physiological processes in plants. These findings indicate that H2O2 may affect different parts of plants by increasing endogenous H2O2 level or by regulating relative gene expression. Also, the change of H2O2 level may impact metabolic and antioxidant enzyme activity in favor of plant growth and development (Barba-Espín et al., 2011; Liu et al., 2013). However, the mechanisms that allow different H2O2 function in plants still require examination.
Stress Condition
Recent studies have demonstrated that H2O2 is a key signaling molecule in the signaling pathway, which associated with abiotic stress response. A number of discussions showed that H2O2 could respond to abiotic stresses such as drought (Hameed and Iqbal, 2014; Ashraf et al., 2015), salinity (Sathiyaraj et al., 2014; Mohamed et al., 2015), cold (Orabi et al., 2015), high temperatures (Wang Y. et al., 2014; Wu et al., 2015), UV radiation (He et al., 2005), ozone (Oksanen et al., 2004), and heavy metal (Wen et al., 2013; Table 2). It is clear from these studies that H2O2 could enhance abiotic stress resistance through protecting organelle structure under abiotic stress conditions. For instance, H2O2 may protect chloroplast ultrastructure to preserve photosynthesis under abiotic stress. Similarly, to improve plant abiotic stress tolerance, H2O2 may modulate the expression of resistance genes and antioxidant enzyme activities during abiotic stress response.
H2O2 as a Signaling Molecule in Plant
Among ROS, H2O2 has comparatively long life span and small size, which permit it to traverse through cellular membranes to different cellular compartments. García-Mata and Lamattina (2013) found that H2O2 may move between cells through aquaporin channels for signaling transduction. Increasing evidences point out that H2O2 signaling may regulate various plant physiological processes. For example, H2O2 as signaling molecule may participate in nitrosative stress-triggered cell death in kimchi cabbage (Brassica rapa var. glabra Regel) seedlings (Kim et al., 2015). Also, Li et al. (2015) suggested that H2O2 is involved in signaling crosstalk between NO and hydrogen sulfide (H2S) to induce thermotolerance in maize seedlings. Moreover, the interaction among H2O2, NO and Ca2+ could relieve copper stress in Ulva compressa (González et al., 2012). H2O2 signaling was also demonstrated to play a salient role in brassinosteroid-regulated stomatal movement (Shi C. et al., 2015). As stated above, H2O2 as an important signaling molecule may play a significant role at every stage of plant life and under various abiotic stress conditions. H2O2 signaling appears to crosstalk with many different signaling molecules such as hormones (Shi C. et al., 2015), protein kinase (González et al., 2012) and many other small signaling molecules (Li et al., 2015). H2O2 and these signaling molecules may influence each other through various positive and negative feedback loops. Thus, they co-regulate cell division and differentiation, antioxidant system as well as gene expression involved in plant development and defense.
Crosstalk Between H2O2 and No
NO is a diatomic free radical gas. Previous studies suggested that NO could take part in a wide range of physiological processes such as vasorelaxation, nervous system, defense against pathogens in animals (Mayer and Hemmens, 1998). In mammals, NO is synthesized via three different isoforms of NO synthase (NOS) including inducible NOS (iNOS; Nathan and Hibbs, 1991), endothelial NOS (eNOS) and neuronal NOS (nNOS; Förstermann et al., 1994). In plants, NO could be synthesized through enzymatic and non-enzymatic pathways (Figure 2). The enzymatic pathway includes nitrate reductase (NR; Rockel et al., 2002), nitric oxide-like (NOS-like) synthase (Guo et al., 2003), Nitrite-NO reductase (Ni-NOR; Stöhr et al., 2001) and xanthine oxidase (XOR; Corpas et al., 2004) pathways. The non-enzymatic generation of NO includes nitrification or de-nitrification processes (Skiba et al., 1993, Figure 2).
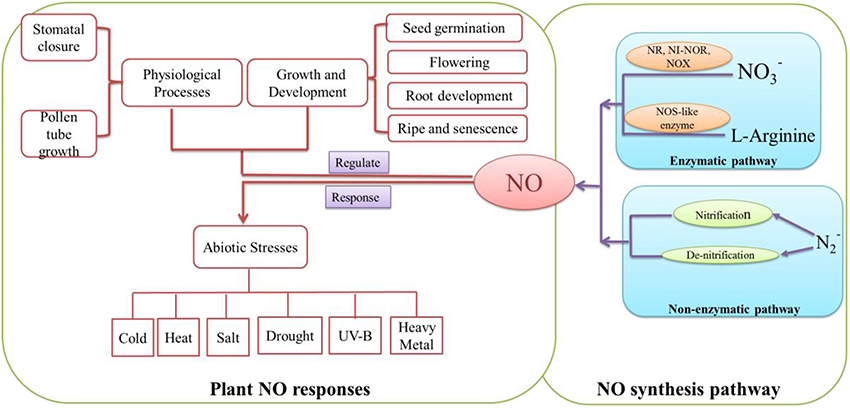
Figure 2. Summary of the main NO systhetic pathways and NO functions in plant growth, development and defense processes. NO may be synthesized by enzymatically and non-enzymatically pathways. In enzymatic pathway, nitrate reductase (NR; Rockel et al., 2002), Nitrite-NO reductase (Ni-NOR; Stöhr et al., 2001) and xanthine oxidase (XOR; Corpas et al., 2004) could convert NO and NO to NO. Meanwhile, because of NOS-like enzyme (Guo et al., 2003), L-Arginine may be catalyzed to NO. In non-enzymatic pathway, N could be transformed to NO through nitrification and denitrification (Skiba et al., 1993). NO plays an important signaling molecule in plant. It could regulate developmental and physiological processes such as seed germination (Wang et al., 2015), root development (Liao et al., 2011) and stomatal closure (Shi C. et al., 2015). Also, it may be involved in response to abiotic stresses such as cold (Fan et al., 2015), salt (Liu W. et al., 2015) and drought (Shan et al., 2015).
A plethora of evidences suggest that NO, as a versatile signaling molecule, is involved in regulating every aspect of plant growth and developmental processes such as seed germination (Fan et al., 2013; Wang et al., 2015), flowering (Liu W. W. et al., 2015), root growth and development (Liao et al., 2011; Wu et al., 2014; Xiang et al., 2015), ripening and senescence (Liao et al., 2013; Shi Y. et al., 2015). Meanwhile, as a physiological regulator, NO signaling is involved in mediating stomatal closure (Noelia et al., 2015; Shi K. et al., 2015; Chen et al., 2016), pollen tube growth (Wang et al., 2009). Also, NO plays an essential role in plant disease resistance (Rasul et al., 2012; Kovacs et al., 2015) and responses to various abiotic stresses such as cold (Fan et al., 2015), heat (Yu et al., 2015), salt (Liu W. et al., 2015), drought (Shan et al., 2015), UV-B (Esringu et al., 2015) and heavy metal (Alemayehu et al., 2015; Chen et al., 2015; Kaur et al., 2015). These studies have paved the way to understand the signaling roles of NO which may affect cell metabolism, cellular redox balance and gene expression in plants. The relative target receptor may receive signaling activated by various stimuli. As a result, NO may activate regulatory mechanism to promote developmental and physiological processes and regulate abiotic stress response in plants.
Interaction in Growth and Development
To date, the interaction between H2O2 and NO has been demonstrated clearly in plants. The signaling crosstalk between H2O2 and NO has been considered to be an essential factor to influence plant developmental and physiological processes such as leaf cell death (Lin et al., 2012), delay senescence (Iakimova and Woltering, 2015), root growth and development (Liao et al., 2010, 2011), stomatal closure (Huang et al., 2015; Shi K. et al., 2015), and pollen tube growth (Serrano et al., 2012). Table 3 shows the interaction of H2O2 and NO at different levels in a great number of developmental and physiological processes in plants. On the one side, H2O2 may act as a cofactor to promote endogenous NO synthesis. For example, Lin et al. (2012) implied that H2O2 may stimulate NO production through increasing NR activity in leaves of noe1 plants under high light. Shi C. et al. (2015) reported that Gα-activated H2O2 production may induce NO synthesis. The research found that NO could modulate stomatal closure in H2O2 mutants AtrbohF and AtrbohD AtrbohF and in the wild type treated with H2O2 scavenger and inhibitor. However, H2O2 did not close or reduce the stomatal closure in mutants Nia1-2 and Nia2-5 Nia1-2, and in the wild type treated c-PTIO or tungstate (Shi C. et al., 2015). These results clearly show that H2O2 might induce NO synthesis in stomatal closure. On the other side, NO may induce H2O2 generation in plants. Liao et al. (2011) reported cPTIO or L-NAME could inhibit the endogenous H2O2 generation implying that NO was required for the production of H2O2 during adventitious rooting. Meanwhile, NO could mediate antioxidant enzyme activities to influence the H2O2 level (Zhang et al., 2007). Thus, the interaction of H2O2 and NO may trigger a serious of physiological and biological response in plant cells.
Interaction during Abiotic Stress
Recently, the roles of H2O2and NO signaling and their crosstalk in mediating plant response to abiotic stresses have been largely established (Table 4).
Drought
Drought stress is a major environmental factor that affects plant growth and development. As reported by Liao et al. (2012a), both H2O2 and NO could protect mesophyll cells ultrastructure and improve the photosynthetic level of leaves under drought stress during adventitious rooting in marigold explants. Similarly, the interplay between H2O2 and NO signaling may increase the activity of myo-inositol phosphate synthase to alleviate drought stress (Tan et al., 2013). Additionally, Lu et al. (2009) suggested that endogenous NO and H2O2 may be involved in ABA-induced drought tolerance of bermudagrass by increasing antioxidant enzyme activities. NO may be considered to be upstream or downstream signaling molecule of H2O2 (Lu et al., 2009; Liao et al., 2012a). Thus, the interaction between H2O2 and NO may alleviate drought stress through up-regulating antioxidant defense system to protect cell membrane and maintain ion homeostasis in plants.
Salt
The interaction between H2O2 and NO plays an important role in plant tolerance to salt stress (Zhang et al., 2007; Tan et al., 2013). Tanou et al. (2009) suggested that H2O2 and NO pre-treatments could alleviate salinity-induced protein carbonylation in citrus. The authors suggested an interaction between H2O2 and NO during salt stress response. Furthermore, H2O2- and NO-responsive proteins have been identified which may further reveal a protein interaction network between H2O2 and NO signaling under salt stress (Tanou et al., 2010).
UV-B
UV-B, a key environmental signal, initiates diverse responses in plants (Jansen and Bornman, 2012). UV-B radiation can also influence plant growth, development, and productivity. It has been shown that the crosstalk between H2O2 and NO could be involved in the response to UV-B stress. There was an interrelationship among Gα protein, H2O2, and NO during UV-B-induced stomatal closure in Arabidopsis leaves (He et al., 2013). This study found that there was a significant increase in H2O2 or NO levels which associated with stomatal closure in the wild type by UV-B stress. However, these effects were abolished by double mutants of AtrbohD and AtrbohF or Nia1 mutants. These results strongly suggested that the crosstalk between H2O2 and NO signaling might play an essential role during UV-B-induced stomatal closure in guard cells. Recently, Tossi et al. (2014) also showed a mechanism involving both H2O2 and NO generation in response to UV-B exposure. Therefore, the crosstalk between H2O2 and NO can regulate stomatal movement to reduce UV-B stress damage to plant cells.
Cold
Cold stress adversely influences plant growth and development. Guo et al. (2014) reported that the interaction of H2O2 and NO may affect cold-induced S-adenosylmethionine synthetase and increase cold tolerance through up-regulating polyamine oxidation in Medicago sativa subsp. falcate. Moreover, signaling interplay of H2O2 and NO was essential for cold-induced gene expression of falcata myo-inositol phosphate synthase (MfMIPS), which improved tolerance to cold stress (Tan et al., 2013). Thus, the interaction between H2O2 and NO may initiate different mechanisms to response to cold stresses.
Heat
Recently, many studies have been conducted to investigate the relationship between H2O2 and NO under heat stress. Li et al. (2015) reported that a signaling crosstalk between H2O2 and NO may be involved in inducing thermotolerance in maize seedlings. Moreover, H2O2 may be upstream signaling of NO in the heat shock pathway in Arabidopsis seedlings (Wang L. et al., 2014). In addition, treatment with low level of H2O2 or NO could increase seedling viability under heat resistance (Karpets et al., 2015). These studies support the existence of crosstalk between H2O2 and NO in heat responses in plants.
Heavy Metal Stress
Alberto et al. (2012) suggested that the signaling interaction between H2O2 and NO was involved in alleviating copper stress of Ulva compressa through mediating antioxidant enzyme activities and activating relative gene expression. Besides, the interplay of NO and H2O2 in wheat seedlings participated in regulating root growth under zinc stress and alleviated zinc stress through increasing antioxidant system, decreasing lipid peroxidation as well as up-regulating resistance gene expression (Duan et al., 2015). Obviously, the crosstalk of H2O2 and NO has been found under heavy metal stress condition, which may trigger a variety of antioxidant responses in plants.
As stated above, the physiological effect of H2O2 and NO is similar and synergetic. In different cases, these forms of interaction are various. However, the form of H2O2 and NO crosstalk depend on plant species and environmental stresses. H2O2 and NO could modulate each other through regulating antioxidant enzymes activities and relative gene expression in plants. Meanwhile, H2O2 and NO may synergistically regulate many common target genes which were related to signaling transduction, defense reaction, plant hormone interactions, protein transport and metabolism. Therefore, it has a significant meaning to elaborate the mechanism of the interaction between H2O2 and NO in plant developmental processes and response to abiotic stresses.
Crosstalk Between H2O2 and Ca2+
Ca2+ is a widespread signaling molecule in plants. When plants receive stimuli, the change of intracellular Ca2+ concentration may transfer signaling to regulate a series of cellular processes in plants (Kong et al., 2015; Tang et al., 2015). There are various types of Ca2+ receptors and channels in plants such as Ca2+-ATPases (Pászty et al., 2015), Ca2+-binding sensor protein (Wagner et al., 2015), inositol-1,4,5-trisphosphate (IP3; Serrano et al., 2015) and cyclic ADP-ribose (cADPR, Gerasimenko et al., 2015). It is well known that Ca2+ is involved in plant growth and development such as seed germination (Kong et al., 2015), pollen tube growth (Zhou et al., 2014), leaf de-etiolation (Huang et al., 2012), root growth and development (Liao et al., 2012a; Han et al., 2015) and other physiological processes including cell polarity regulation (Zhou et al., 2014; Himschoot et al., 2015), stomatal closure (Zou et al., 2015) and immune response (Seybold et al., 2014). Furthermore, variations in cytosolic free Ca2+ concentration have been demonstrated to response to a wide range of environmental stresses such as heat shock (Urao et al., 1994), drought (Zou et al., 2015), light (Hu et al., 2015), salt (Tepe and Aydemir, 2015), and heavy metal (Li et al., 2016). Because of Ca2+ has various receptors and channels in plants, it may receive different upstream signaling molecules quickly and then respond to abiotic stress.
Interaction in Growth and Development
Crosstalk between H2O2 and Ca2+ occurs in plant cells (Table 5). For example, exogenous H2O2 caused transiently dose-dependent increase in Ca2+ influx in Arabidopsis thaliana root epidermis (Demidchik et al., 2007). Two Ca2+ channels could be regulated by H2O2 level in root elongation zone. Han et al. (2015) demonstrated that H2O2 signaling could induce root elongation by mediating Ca2+ influx in the plasma membrane of root cells in Arabidopsis seedlings. Richards et al. (2014) also suggested that Annexin 1, a Ca2+ transport protein, may regulate H2O2-induced Ca2+ signature in Arabidopsis thaliana roots to promote root growth and development. Additionally, Ca2+ signaling was involved in H2O2-induced adventitious rooting in marigold because removal of Ca2+ could inhibit H2O2-induced adventitious root development (Liao et al., 2012a). Interestingly, Wu et al. (2010)'s findings strongly suggested that spermidine oxidase (Spd)-derived H2O2 signaling may mediate Ca2+ influx. Spd was probably related to downstream induction of H2O2 signaling and then H2O2 activated Ca2+-permeable channels during pollen tube growth (Wu et al., 2010). Cross talk between Ca2+–Calmodulin (CaM) and H2O2 also played a significant role in antioxidant defense in ABA signaling in maize leaves (Hu et al., 2007; Table 5). Thus, the signaling crosstalk between H2O2 and Ca2+ may affect every stage of plant development by modulating cell elongation and division, antioxidant enzyme activity and gene expression. H2O2 may activate Ca2+ receptors and target proteins to increase [Ca2+]cyt level and Ca2+ may induce endogenous H2O2 generation during plant growth and development.
Interaction in Abiotic Stress
Clearly, correlations also exist between H2O2 and Ca2+ in response to abiotic stresses in plants (Table 6). Shoresh et al. (2011) investigated that supplemental Ca2+ had a significant effect on H2O2 metabolism and regulating leaves and roots growth in maize under salt stress. The authors indicated that extracellular Ca2+ may modulate endogenous H2O2 levels through activating polyamine oxidase activity. Also, salt stress may induce H2O2 accumulation in Ca2+-dependent salt resistance pathway in Arabidopsis thaliana roots (Li et al., 2011). Moreover, Lu et al. (2013) suggested that exogenous H2O2 and Ca2+ may mediate root ion fluxes in mangrove species under NaCl stress. Obviously, H2O2 may interact with Ca2+ under salt stress in plants through mediating root ion balance, increasing antioxidant enzymatic activity and up-regulating the expression of related genes. Moreover, H2O2 and Ca2+ signaling were also involved in ABA responses to drought stress in Arabidopsis thaliana through Ca2+-dependent protein kinase8 (CPK8) which could regulate catalase3 (CAT3) activity mediating stomatal movement (Zou et al., 2015). In addition, Qiao et al. (2015) reported that a Ca2+-binding protein (rice annexin OsANN1) could enhance heat stress tolerance by modulating H2O2 production. Over production of H2O2 induced by heat stress increased OsANN1 expression and up-regulated the level of SOD and CAT expression, which constructed a signaling mechanism for stress defense in plants (Qiao et al., 2015). Until now, the signaling crosstalk between H2O2 and Ca2+ may regulate various responses to abiotic stresses in plants. It may be connected with the regulation of antioxidant system. Thus, the interaction between H2O2 and Ca2+ may increase antioxidant enzyme activities such as APX, SOD, and GR. These antioxidant enzymes may alleviate stress damages in plants. In addition, the crosstalk between H2O2 and Ca2+ could regulate gene expression level and induce protein interactions.
It appears that the interrelationship between H2O2 and Ca2+ may be involved in various aspects of plant growth and development processes and abiotic stress responses. In fact, the change of Ca2+ concentration is closely related to H2O2 burst in plant cells. The combination of H2O2 and Ca2+ may play crucial roles in plants. Different plants even different parts of the same plant may have different modulation mechanisms. Thus, relationship between H2O2 and Ca2+ signaling in plants is very complex. The interplay of H2O2, Ca2+ and its mechanism need to be illustrated clearly in the future.
Crosstalk Among H2O2, No and Ca2+
It has been suggested that there is a connection among H2O2, NO, and Ca2+ in plants. H2O2, NO, and Ca2+ may act as essential signaling molecules which may form a complex signaling network to regulate different developmental and physiological processes in plants (Figure 3). For instance, during adventitious rooting of mung bean, Ca2+ signaling played a pivotal role and functioned as a downstream molecule of H2O2 and NO signal pathway (Li and Xue, 2010; Figure 3). Similarly, there is a possible relationship among H2O2,NO and Ca2+/CaM during adventitious rooting in marigold explants (Liao et al., 2012a). The authors found that exogenous NO and H2O2 promoted adventitious root development in marigold explants through increasing endogenous Ca2+ and CaM levels. Moreover, H2O2, NO and Ca2+ were also involved in oligochitosan-induced programmed cell death in tobacco suspension cells (Zhang et al., 2012). Pharmacological experiments revealed that Ca2+ signaling induced NO accumulation through inducing H2O2 generation during stomatal closure in Arabidopsis guard cells (Li et al., 2009). Furthermore, Wang et al. (2011) suggested a functional correlationship among H2O2, calcium-sensing receptor (CAS) and NO in Ca2+-dependent guard cell signaling. It was shown that CAS may transduce Ca2+ signaling through activating its downstream target NO and H2O2 signaling pathway (Wang et al., 2011). Therefore, it is thus clear that the interplay of H2O2, NO, and Ca2+ may have an significant effect on plant growth and physiological processes through promoting cell proliferation, controlling cell metabolism, meanwhile, regulating modes of cell death. Moreover, Vandelle et al. (2006) has reported that NO and H2O2 synthesis could also act upstream to increase cytosolic Ca2+ concentration during hypersensitive response (HR) through activating plasma membrane- and intracellular membrane-associated Ca2+ channels. Besides, the interaction among H2O2, NO, and Ca2+ signaling may regulate ABA-induced antioxidant defense in maize (Ma et al., 2012). Obviously, the mutual effect among H2O2, NO and Ca2+ may increase antioxidant system and induce disease defense in plants.
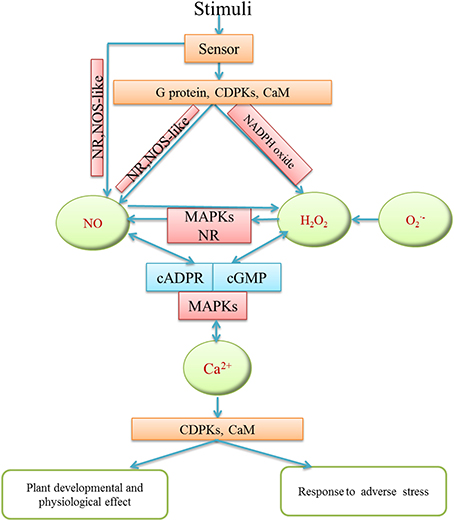
Figure 3. Schematic model of the interaction among H2O2, NO, Ca2+ in different plant physiological and defense processes. H2O2, NO and Ca2+ may receive various stimuli through signaling sensors. They might interact via cross-regulation and transduce signaling to downstream molecules by activating phosphokinase like MAPKs, or relative enzyme activity in order to regulate plant development and growth and abiotic stress responses.
Furthermore, the interplay among H2O2, NO, and Ca2+ also have an effect on abiotic stress response in plants. For example, Lang et al. (2014) reported that NO likely interacted with Ca2+ and H2O2 in Aegiceras corniculatum to up-regulate Na+/H+ antiport system of plasma membrane under salt stress. There were species-specific interactions between H2O2, Ca2+, NO, and ATP in salt-induced reduction of K+ efflux (Lang et al., 2014). Moreover, there was a crosstalk among H2O2, NO, and Ca2+ when Ulva compressa exposed to copper excess and the interaction had a significant effect on transcriptional activation of target genes (Alberto et al., 2012). The H2O2-induced NO generation could be inhibited by Ca2+ channel blockers, implicating that Ca2+ may mediate the effect of H2O2 on NO production. Furthermore, Ca2+ release through different type of Ca2+ channels was also shown to be activated by NO and H2O2 (Alberto et al., 2012; Figure 3). The interrelationship between H2O2, NO and Ca2+ may provide additional layers of responses to abiotic stresses through controlling ion transport, increasing antioxidant enzyme activities and affecting expression of resistance genes, indicating a feedback mechanism between H2O2, NO and Ca2+ under abiotic stresses. In a word, the combination of these findings strongly supports the view that there has an interaction among H2O2, NO, and Ca2+ signaling pathway in plant growth, development and abiotic stress responses. During signaling transduction, Ca2+ signaling could be activated by H2O2 and NO; it could also regulate H2O2 and NO signaling. Ca2+ may act as a point of signaling convergence between H2O2 and NO signaling pathways in plants. However, the network of H2O2, NO, and Ca2+ seems to be intricate and multidimensional. Therefore, considerably more work will need to be done to determine the interaction among H2O2, NO and Ca2+ signaling in plants.
Conclusion
H2O2 was once considered as a poisonous molecule in plants. Based on current studies, H2O2 may be a vital signaling molecule which controls plant growth and development. Interestingly, NO and Ca2+ which also act as the key component of signaling transduction in plants seem to be as upstream or downstream signaling molecules of H2O2. Meanwhile, H2O2 modulates NO and Ca2+ signaling pathways. There is a complex interactive network among H2O2, NO, and Ca2+ in plants. Moreover, the interplay among them has functional implications for regulating developmental and physiological processes which may increase the possibility of signal reception and transduction in plants. Future work will need to focus on the molecular mechanism of the interplay among H2O2, NO, and Ca2+ during signaling transduction in plants.
Author Contributions
LN wrote the paper. WL provided the idea and revised the paper.
Conflict of Interest Statement
The authors declare that the research was conducted in the absence of any commercial or financial relationships that could be construed as a potential conflict of interest.
Acknowledgments
This research was supported by the National Natural Science Foundation of China (Nos. 31160398 and 31560563), the Post Doctoral Foundation of China (Nos. 20100470887 and 2012T50828), the Key Project of Chinese Ministry of Education (No. 211182), the Research Fund for the Doctoral Program of Higher Education (No. 20116202120005), the Natural Science Foundation of Gansu References Province, China (Nos. 1308RJZA179 and 1308RJZA262), and the Fundamental Research Funds for Universities in Gansu, P. R. China.
References
Alberto, G., Cabrera, M. D., Josefa, H., Contreras, R. A., Bernardo, M., and Alejandra, M. (2012). Cross talk among calcium, hydrogen peroxide, and nitric oxide and activation of gene expression involving calmodulins and calcium-dependent protein kinases in ulva compressa exposed to copper excess. Plant Physiol. 158, 1451–1462. doi: 10.1104/pp.111.191759
Alemayehu, A., Zelinová, V., Boèová, B., Huttová, J., Mistrík, I., and Tamás, L. (2015). Enhanced nitric oxide generation in root transition zone during the early stage of cadmium stress is required for maintaining root growth in barley. Plant Soil 390, 213–222. doi: 10.1007/s11104-015-2397-5
Asada, K. (2006). Production and scavenging of reactive oxygen species in chloroplasts and their functions. Plant Physiol. 141, 391–396. doi: 10.1104/pp.106.082040
Ashraf, M. A., Rasheed, R., Hussain, I., Iqbal, M., Haider, M. Z., Parveen, S., et al. (2015). Hydrogen peroxide modulates antioxidant system and nutrient relation in maize (Zea mays L.) under water-deficit conditions. Arch. Agron. Soil Sci. 61, 507–523. doi: 10.1080/03650340.2014.938644
Barba-Espín, G., Diaz-Vivancos, P., Job, D., Belghazi, M., Job, C., and Hernández, J. A. (2011). Understanding the role of H2O2 during pea seed germination: a combined proteomic and hormone profiling approach. Plant Cell Environ. 34, 1907–1919. doi: 10.1111/j.1365-3040.2011.02386.x
Begara-Morales, J. C., Sánchez-Calvo, B., Chaki, M., Valderrama, R., Mata-Pérez, C., López-Jaramillo, J., et al. (2013). Dual regulation of cytosolic ascorbate peroxidase (APX) by tyrosine nitration and S-nitrosylation. J. Exp. Bot. 65, 527–538. doi: 10.1093/jxb/ert396
Ben Rejeb, K., Vos, L. D., Le Disquet, I., Leprince, A. S., Bordenave, M., Maldiney, R., et al. (2015). Hydrogen peroxide produced by NADPH oxidases increases proline accumulation during salt or mannitol stress in Arabidopsis thaliana. New Phytol. 208, 1138–1148. doi: 10.1111/nph.13550
Brewer, T. F., Garcia, F. J., Onak, C. S., Carroll, K. S., and Chang, C. J. (2015). Chemical approaches to discovery and study of sources and targets of hydrogen peroxide redox signaling through NAPDH oxidase proteins. Annu. Rev. Biochem. 84, 765–790. doi: 10.1146/annurev-biochem-060614-034018
Brychkova, G., Yarmolinsky, D., Fluhr, R., and Sagi, M. (2012). The determination of sulfite levels and its oxidation in plant leaves. Plant Sci. 190, 123–130. doi: 10.1016/j.plantsci.2012.04.004
Chang, Q., and Tang, H. (2014). Optical determination of glucose and hydrogen peroxide using a nanocomposite prepared from glucose oxidase and magnetite nanoparticles immobilized on graphene oxide. Microchim. Acta 181, 527–534. doi: 10.1007/s00604-013-1145-x
Chen, J., Xiang, L., Chao, W., Yin, S. S., Li, X. L., Hu, W. J., et al. (2015). Nitric oxide ameliorates zinc oxide nanoparticles-induced phytotoxicity in rice seedlings. J. Hazard. Mater. 297, 173–182. doi: 10.1016/j.jhazmat.2015.04.077
Chen, Z. H., Wang, Y. Z., Wang, J. W., Babla, M., Zhao, C., García-Mata, C., et al. (2016). Nitrate reductase mutation alters potassium nutrition as well as nitric oxide-mediated control of guard cell ion channels in Arabidopsis. New Phytol. 209, 1456–1469. doi: 10.1111/nph.13714
Cheng, X. X., Yu, M., Zhang, N., Zhou, Z. Q., Xu, Q. T., Mei, F. Z., et al. (2015). Reactive oxygen species regulate programmed cell death progress of endosperm in winter wheat (Triticum aestivum L.) under waterlogging. Protoplasma. doi: 10.1007/s00709-015-0811-8. [Epub ahead of print].
Cona, A., Rea, G., Botta, M., Corelli, F., Federico, R., and Angelini, R. (2006). Flavin-containing polyamine oxidase is a hydrogen peroxide source in the oxidative response to the protein phosphatase inhibitor cantharidin in Zea mays L. J. Exp. Bot. 57, 2277–2289. doi: 10.1093/jxb/erj195
Corpas, F. J., Barroso, J. B., and Del Río, L. A. (2004). Enzymatic sources of nitric oxide in plant cells—beyond one protein–one function. New Phytol. 162, 246–248. doi: 10.1111/j.1469-8137.2004.01058.x
Dat, J., Vandenabeele, S., Vranová, E., Van Montagu, M., Inzé, D., and Van Breusegem, F. (2000). Dual action of the active oxygen species during plant stress responses. Cell Mol. Life Sci. 57, 779–795. doi: 10.1007/s000180050041
Demidchik, V., Shabala, S. N., and Davies, J. M. (2007). Spatial variation in H2O2 response of Arabidopsis thaliana root epidermal Ca2+ flux and plasma membrane Ca2+ channels. Plant J. 49, 377–386. doi: 10.1111/j.1365-313X.2006.02971.x
Dickinson, B. C., and Chang, C. J. (2011). Chemistry and biology of reactive oxygen species in signaling or stress responses. Nat. Chem. Biol. 7, 504–511. doi: 10.1038/nchembio.607
Duan, X., Li, X., Ding, F., Zhao, J., Guo, A., Zhang, L., et al. (2015). Interaction of nitric oxide and reactive oxygen species and associated regulation of root growth in wheat seedlings under zinc stress. Ecotox. Environ. Safe 113, 95–102. doi: 10.1016/j.ecoenv.2014.11.030
Esringu, A., Aksakal, O., Tabay, D., and Kara, A. A. (2015). Effects of sodium nitroprusside (SNP) pretreatment on UV-B stress tolerance in lettuce (Lactuca sativa L.) seedlings. Environ. Sci. Pollut. Res. 23, 589–597. doi: 10.1007/s11356-015-5301-1
Fan, H. F., Du, C. X., Ding, L., and Xu, Y. L. (2013). Effects of nitric oxide on the germination of cucumber seeds and antioxidant enzymes under salinity stress. Acta Physiol. Plant 35, 2707–2719. doi: 10.1007/s11738-013-1303-0
Fan, J., Chen, K., Amombo, E., Hu, Z., Chen, L., and Fu, J. (2015). Physiological and molecular mechanism of nitric oxide (NO) involved in bermudagrass response to cold stress. PLoS ONE 10:e0132991. doi: 10.1371/journal.pone.0132991
Fan, Y., and Huang, Y. (2012). The effective peroxidase-like activity of chitosan-functionalized CoFe2O4 nanoparticles for chemiluminescence sensing of hydrogen peroxide and glucose. Analyst 137, 1225–1231. doi: 10.1039/c2an16105b
Förstermann, U., Closs, E. I., Pollock, J. S., Nakane, M., Schwarz, P., Gath, I., et al. (1994). Nitric oxide synthase isozymes. Characterization, purification, molecular cloning, and functions. Hypertension 23, 1121–1131. doi: 10.1161/01.HYP.23.6.1121
Foyer, C. H., and Noctor, G. (2003). Redox sensing and signalling associated with reactive oxygen in chloroplasts, peroxisomes and mitochondria. Physiol. Plant 119, 355–364. doi: 10.1034/j.1399-3054.2003.00223.x
Francoz, E., Ranocha, P., Nguyen-Kim, H., Jamet, E., Burlat, V., and Dunand, C. (2015). Roles of cell wall peroxidases in plant development. Phytochemistry 112, 15–21. doi: 10.1016/j.phytochem.2014.07.020
García-Mata, C., and Lamattina, L. (2013). Gasotransmitters are emerging as new guard cell signaling molecules and regulators of leaf gas exchange. Plant Sci. 201, 66–73. doi: 10.1016/j.plantsci.2012.11.007
Ge, X. M., Cai, H. L., Lei, X., Zou, X., Yue, M., and He, J. M. (2015). Heterotrimeric G protein mediates ethylene-induced stomatal closure via hydrogen peroxide synthesis in Arabidopsis. Plant J. 82, 138–150. doi: 10.1111/tpj.12799
Gerasimenko, J. V., Charlesworth, R. M., Sherwood, M. W., Ferdek, P. E., Mikoshiba, K., Parrington, J., et al. (2015). Both RyRs and TPCs are required for NAADP-induced intracellular Ca2+ release. Cell Calcium 58, 237–245. doi: 10.1016/j.ceca.2015.05.005
González, A., de los Ángeles Cabrera, M., Henríquez, M. J., Contreras, R. A., Morales, B., and Moenne, A. (2012). Cross talk among calcium, hydrogen peroxide, and nitric oxide and activation of gene expression involving calmodulins and calcium-dependent protein kinases in Ulva compressa exposed to copper excess. Plant Physiol. 158, 1451–1462. doi: 10.1104/pp.111.191759
Grivennikova, V. G., and Vinogradov, A. D. (2013). Partitioning of superoxide and hydrogen peroxide production by mitochondrial respiratory complex I. BBA-MOL. Cell. Res. 1827, 446–454. doi: 10.1016/j.bbabio.2013.01.002
Guo, F., Okamoto, M., and Crawford, N. M. (2003). Identification of a plant nitric oxide synthase gene involved in hormonal signaling. Science 302, 100–103. doi: 10.1126/science.1086770
Guo, Z., Tan, J., Zhuo, C., Wang, C., Xiang, B., and Wang, Z. (2014). Abscisic acid, H2O2 and nitric oxide interactions mediated cold-induced S-adenosylmethionine synthetase in Medicago sativa subsp. falcata that confers cold tolerance through up-regulating polyamine oxidation. Plant Biotechnol. J. 12, 601–612. doi: 10.1111/pbi.12166
Hameed, A., and Iqbal, N. (2014). Chemo-priming with mannose, mannitol and H2O2 mitigate drought stress in wheat. Cereal Res. Commun. 42, 450–462. doi: 10.1556/CRC.2013.0066
Han, S., Fang, L., Ren, X., Wang, W., and Jiang, J. (2015). MPK6 controls H2O2-induced root elongation by mediating Ca2+ influx across the plasma membrane of root cells in Arabidopsis seedlings. New Phytol. 205, 695–706. doi: 10.1111/nph.12990
He, J. M., Ma, X. G., Zhang, Y., Sun, T. F., Xu, F. F., Chen, Y. P., et al. (2013). Role and interrelationship of Gα protein, hydrogen peroxide, and nitric oxide in ultraviolet B-induced stomatal closure in Arabidopsis leaves. Plant Physiol. 161, 1570–1583. doi: 10.1104/pp.112.211623
He, J. M., Xu, H., She, X. P., Song, X. G., and Zhao, W. M. (2005). The role and the interrelationship of hydrogen peroxide and nitric oxide in the UV-B-induced stomatal closure in broad bean. Funct. Plant Biol. 32, 237–247. doi: 10.1071/FP04185
Hernández-Barrera, A., Velarde-Buendía, A., Zepeda, I., Sanchez, F., Quinto, C., Sánchez-Lopez, R., et al. (2015). Hyper, a hydrogen peroxide sensor, indicates the sensitivity of the Arabidopsis root elongation zone to aluminum treatment. Sensors 15, 855–867. doi: 10.3390/s150100855
Himschoot, E., Beeckman, T., Friml, J., and Vanneste, S. (2015). Calcium is an organizer of cell polarity in plants. Biochim. Biophys. Acta 1853, 2168–2172. doi: 10.1016/j.bbamcr.2015.02.017
Hu, X., Bidney, D. L., Yalpani, N., Duvick, J. P., Crasta, O., Folkerts, O., et al. (2003). Overexpression of a gene encoding hydrogen peroxide-generating oxalate oxidase evokes defense responses in sunflower. Plant Physiol. 133, 170–181. doi: 10.1104/pp.103.024026
Hu, X., Jiang, M., Zhang, J., Zhang, A., Lin, F., and Tan, M. (2007). Calcium-calmodulin is required for abscisic acid-induced antioxidant defense and functions both upstream and downstream of H2O2 production in leaves of maize (Zea mays) plants. New Phytol. 173, 27–38. doi: 10.1111/j.1469-8137.2006.01888.x
Hu, Z., Li, T., Zheng, J., Yang, K., He, X., and Leng, P. (2015). Ca2+ signal contributing to the synthesis and emission of monoterpenes regulated by light intensity in Lilium ‘siberia’. Plant Physiol. Biochem. 91, 1–9. doi: 10.1016/j.plaphy.2015.03.005
Huang, A. X., Wang, Y. S., She, X. P., Mu, J., and Zhao, J. L. (2015). Copper amine oxidase-catalysed hydrogen peroxide involves production of nitric oxide in darkness-induced stomatal closure in broad bean. Funct. Plant Biol. 42, 1057–1067. doi: 10.1071/FP15172
Huang, S. S., Chen, J., Dong, X. J., Patton, J., Pei, Z. M., and Zheng, H. L. (2012). Calcium and calcium receptor CAS promote Arabidopsis thaliana de-etiolation. Physiol. Plant. 144, 73–82. doi: 10.1111/j.1399-3054.2011.01523.x
Iakimova, E. T., and Woltering, E. J. (2015). Nitric oxide prevents wound-induced browning and delays senescence through inhibition of hydrogen peroxide accumulation in fresh-cut lettuce. Innov. Food Sci. Emerg. Technol. 30, 157–169. doi: 10.1016/j.ifset.2015.06.001
Jahan, A. A., and Anis, M. (2014). Changes in antioxidative enzymatic responses during acclimatization of in vitro raised plantlets of Cardiospermum halicacabum L. against oxidative stress. J. Plant Physiol. Pathol. 4, 2. doi: 10.4172/2329-955x.1000137
Jansen, M. A. K., and Bornman, J. F. (2012). UV-B radiation: from generic stressor to specific regulator. Physiol. Plant. 145, 501–504. doi: 10.1111/j.1399-3054.2012.01656.x
Kapoor, D., Sharma, R., Handa, N., Kaur, H., Rattan, A., Yadav, P., et al. (2015). Redox Homeostasis in Plants under Abiotic Stress: role of electron carriers, energy metabolism mediators and proteinaceous thiols. Front. Environ. Sci. 3:13. doi: 10.3389/fenvs.2015.00013
Karpets, Y. V., Kolupaev, Y. E., and Vayner, A. A. (2015). Functional interaction between nitric oxide and hydrogen peroxide during formation of wheat seedling induced heat resistance. Russ. J. Plant Physiol. 62, 65–70. doi: 10.1134/S1021443714060090
Kaur, G., Singh, H. P., Batish, D. R., Mahajan, P., Kohli, R. K., and Rishi, V. (2015). Exogenous nitric oxide (NO) interferes with lead (Pb)-induced toxicity by detoxifying reactive oxygen species in hydroponically grown wheat (triticum aestivum) roots. PLoS ONE 10:e0138713. doi: 10.1371/journal.pone.0138713
Kim, Y. J., Lee, Y. H., Lee, H. J., Jung, H., and Hong, J. K. (2015). H2O2 production and gene expression of antioxidant enzymes in kimchi cabbage (Brassica rapa var. glabra Regel) seedlings regulated by plant development and nitrosative stress-triggered cell death. Plant Biotechnol. Rep. 9, 67–78. doi: 10.1007/s11816-015-0343-x
Kong, D., Ju, C., Parihar, A., Kim, S., Cho, D., and Kwak, J. M. (2015). Arabidopsis glutamate receptor homolog3.5 modulates cytosolic Ca2+ level to counteract effect of abscisic acid in seed germination. Plant Physiol. 167, 1630–1642. doi: 10.1104/pp.114.251298
Kovacs, I., Durner, J., and Lindermayr, C. (2015). Crosstalk between nitric oxide and glutathione is required for NONEXPRESSOR OF PATHOGENESIS-RELATED GENES 1 (NPR1)-dependent defense signaling in Arabidopsis thaliana. New Phytol. 208, 860–872. doi: 10.1111/nph.13502
Krifka, S., Hiller, K. A., Spagnuolo, G., Jewett, A., Schmalz, G., and Schweikl, H. (2012). The influence of glutathione on redox regulation by antioxidant proteins and apoptosis in macrophages exposed to 2-hydroxyethyl methacrylate (HEMA). Biomaterials 33, 5177–5186. doi: 10.1016/j.biomaterials.2012.04.013
Lang, T., Sun, H. M., Li, N. Y., Lu, Y. J., Shen, Z. D., Jing, X. S., et al. (2014). Multiple signaling networks of extracellular ATP, hydrogen peroxide, calcium, and nitric oxide in the mediation of root ion fluxes in secretor and non-secretor mangroves under salt stress. Aquat. Bot. 119, 33–43. doi: 10.1016/j.aquabot.2014.06.009
Li, J., Wang, X., Zhang, Y., Jia, H., and Bi, Y. (2011). cGMP regulates hydrogen peroxide accumulation in calcium-dependent salt resistance pathway in Arabidopsis thaliana roots. Planta 234, 709–722. doi: 10.1007/s00425-011-1439-3
Li, J. H., Liu, Y. Q., Lü, P., Lin, H. F., Bai, Y., Wang, X. C., et al. (2009). A signaling pathway linking nitric oxide production to heterotrimeric G protein and hydrogen peroxide regulates extracellular calmodulin induction of stomatal closure in Arabidopsis. Plant Physiol. 150, 114–124. doi: 10.1104/pp.109.137067
Li, P., Zhao, C., Zhang, Y., Wang, X., Wang, X., Wang, J., et al. (2016). Calcium alleviates cadmium-induced inhibition on root growth by maintaining auxin homeostasis in Arabidopsis seedlings. Protoplasma 253, 185–200. doi: 10.1007/s00709-015-0810-9
Li, S. W., and Xue, L. G. (2010). The interaction between H2O2 and NO, Ca2+, cGMP, and MAPKs during adventitious rooting in mung bean seedlings. In Vitro Cell Dev. Biol. 46, 142–148. doi: 10.1007/s11627-009-9275-x
Li, Z. G., Luo, L. J., and Sun, Y. F. (2015). Signal crosstalk between nitric oxide and hydrogen sulfide may be involved in hydrogen peroxide –induced thermotolerance in maize seedlings. Russ. J. Plant Physiol. 62, 507–514. doi: 10.1134/S1021443715030127
Liao, W. B., Huang, G. B., Yu, J. H., Zhang, M. L., and Shi, X. L. (2011). Nitric oxide and hydrogen peroxide are involved in indole-3-butyric acid-induced adventitious root development in marigold. J. Hortic. Sci. Biotech. 86, 159–165. doi: 10.1080/14620316.2011.11512742
Liao, W. B., Xiao, H. L., and Zhang, M. L. (2009). Role and relationship of nitric oxide and hydrogen peroxide in adventitious root development of marigold. Acta Physiol. Plant. 31, 1279–1289. doi: 10.1007/s11738-009-0367-3
Liao, W. B., Xiao, H. L., and Zhang, M. L. (2010). Effect of nitric oxide and hydrogen peroxide on adventitious root development from cuttings of ground-cover chrysanthemum and associated biochemical changes. J. Plant Growth Regul. 29, 338–348. doi: 10.1007/s00344-010-9140-5
Liao, W. B., Zhang, M. L., and Yu, J. H. (2013). Role of nitric oxide in delaying senescence of cut rose flowers and its interaction with ethylene. Sci. Horticult. 155, 30–38. doi: 10.1016/j.scienta.2013.03.005
Liao, W. B., Zhang, M. L., Huang, G. B., and Yu, J. H. (2012a). Ca2+ and CaM are involved in NO-and H2O2-induced adventitious root development in marigold. J. Plant Growth Regul. 31, 253–264. doi: 10.1007/s00344-011-9235-7
Liao, W. B., Zhang, M. L., Huang, G. B., and Yu, J. H. (2012b). Hydrogen peroxide in the vase solution increases vase life and keeping quality of cut Oriental × Trumpet hybrid lily ‘Manissa’. Sci. Horticult. 139, 32–38. doi: 10.1016/j.scienta.2012.02.040
Lin, A. H., Wang, Y. Q., Tang, J. Y., Xue, P., Li, C. L., Liu, L. C., et al. (2012). Nitric oxide and protein S-Nitrosylation are integral to hydrogen peroxide-induced leaf cell death in Rice. Plant Physiol. 158, 451–464. doi: 10.1104/pp.111.184531
Liu, J., Macarisin, D., Wisniewski, M., Sui, Y., By, S., Norelli, J., et al. (2013). Production of hydrogen peroxide and expression of ros-generating genes in peach flower petals in response to host and non-host fungal pathogens. Plant Pathol. 62, 820–828. doi: 10.1111/j.1365-3059.2012.02683.x
Liu, W., Li, R. J., Han, T. T., Cai, W., Fu, Z. W., and Lu, Y. T. (2015). Salt stress reduces root meristem size by nitric oxide-mediated modulation of auxin accumulation and signaling in Arabidopsis. Plant Physiol. 168, 343–356. doi: 10.1104/pp.15.00030
Liu, W. W., Chen, H. B., Lu, X. Y., Rahman, M. J., Zhong, S., and Zhou, B. Y. (2015). Identification of nitric oxide responsive genes in the floral buds of Litchi chinensis. Biol. Plant. 59, 115–122. doi: 10.1007/s10535-014-0466-x
Lu, S., Su, W., Li, H., and Guo, Z. (2009). Abscisic acid improves drought tolerance of triploid bermudagrass and involves H2O2-and NO-induced antioxidant enzyme activities. Plant Physiol. Biochem. 47, 132–138. doi: 10.1016/j.plaphy.2008.10.006
Lu, Y., Li, N., Sun, J., Hou, P., Jing, X., Zhu, H., et al. (2013). Exogenous hydrogen peroxide, nitric oxide and calcium mediate root ion fluxes in two non-secretor mangrove species subjected to NaCl stress. Tree Physiol. 33, 81–95. doi: 10.1093/treephys/tps119
Ma, F., Wang, L. J., Li, J. L., Samma, M. K., Xie, Y. J., Wang, R., et al. (2014). Interaction between HY1 and H2O2 in auxin-induced lateral root formation in Arabidopsis. Plant Mol. Biol. 85, 49–61. doi: 10.1007/s11103-013-0168-3
Ma, F. F., Lu, R., Liu, H. Y., Shi, B., Zhang, J. H., Tan, M. P., et al. (2012). Nitric oxide-activated calcium/calmodulin-dependent protein kinase regulates the abscisic acid-induced antioxidant defence in maize. J. Exp. Bot. 63, 4835–4847. doi: 10.1093/jxb/ers161
Mayer, B., and Hemmens, B. (1998). Biosynthesis and action of nitric oxide in mammalian cells. Trends Biochem. Sci. 22, 477–481. doi: 10.1016/S0968-0004(97)01147-X
Mehler, A. H. (1951). Studies on reactions of illuminated chloroplasts. II. Stimulation and inhibition of the reaction with molecular oxygen. Arch. Biochem. Biophys.33, 339–351. doi: 10.1016/0003-9861(51)90082-3
Mittler, R. (2002). Oxidative stress, antioxidants and stress tolerance. Trends Plant Sci. 7, 405–410. doi: 10.1016/S1360-1385(02)02312-9
Mohamed, H. E., Hemeida, A. E., and Mohamed, A. G. (2015). Role of hydrogen peroxide pretreatment on developing antioxidant capacity in the leaves of tomato plant (Lycopersicon esculentum) grown under saline stress. Int. J. Adv. Res. 3, 878–879.
Nathan, C. F., and Hibbs, J. B. (1991). Role of nitric oxide synthesis in macrophage antimicrobial activity. Curr. Opin. Immunol. 3, 65–70. doi: 10.1016/0952-7915(91)90079-G
Navrot, N., Rouhier, N., Gelhaye, E., and Jacquot, J. P. (2007). Reactive oxygen species generation and antioxidant systems in plant mitochondria. Physiol. Plant. 129, 185–195. doi: 10.1111/j.1399-3054.2006.00777.x
Noelia, F., Mayta, M. L., Lodeyro, A. F., Denise, S., Natalia, C., Carlos, G., et al. (2015). Expression of the tetrahydrofolate-dependent nitric oxide synthase from the green alga ostreococcus tauri increases tolerance to abiotic stresses and influences stomatal development in Arabidopsis. Plant J. 82, 806–821. doi: 10.1111/tpj.12852
Nyathi, Y., and Baker, A. (2006). Plant peroxisomes as a source of signaling molecules. Biochim. Biophys. Acta 1763, 1478–1495. doi: 10.1016/j.bbamcr.2006.08.031
Oksanen, E., Häikiö, E., Sober, J., and Karnosky, D. F. (2004). Ozone-induced H2O2 accumulation in field-grown aspen and birch is linked to foliar ultrastructure and peroxisomal activity. New Phytol. 161, 791–799. doi: 10.1111/j.1469-8137.2003.00981.x
Orabi, S. A., Dawood, M. G., and Salman, S. R. (2015). Comparative study between the physiological role of hydrogen peroxide and salicylic acid in alleviating the harmful effect of low temperature on tomato plants grown under sand-ponic culture. Sci. Agric. 9, 49–59. doi: 10.15192/PSCP.SA.2015.1.9.4959
Pászty, K., Caride, A. J., Bajzer, Ž., Offord, C. P., Padányi, R., Hegedûs, L., et al. (2015). Plasma membrane Ca2+-ATPases can shape the pattern of Ca2+ transients induced by store-operated Ca2+ entry. Sci. Signal. 8, 1–32. doi: 10.1126/scisignal.2005672
Qiao, B., Zhang, Q., Liu, D., Wang, H., Yin, J., Wang, R., et al. (2015). A calcium-binding protein, rice annexin OsANN1, enhances heat stress tolerance by modulating the production of H2O2. J. Exp. Bot. 66, 5853–5866. doi: 10.1093/jxb/erv294
Rasul, S., Dubreuil-Maurizi, C., Lamotte, O., Koen, E., Poinssot, B., Alcaraz, G., et al. (2012). Nitric oxide production mediates oligogalacturonide-triggered immunity and resistance to botrytis cinerea in Arabidopsis thaliana. Plant Cell Environ. 35, 1483–1499. doi: 10.1111/j.1365-3040.2012.02505.x
Remans, T., Opdenakker, K., Smeets, K., Mathijsen, D., Vangronsveld, J., and Cuypers, A. (2010). Metal-specific and NADPH oxidase dependent changes in lipoxygenase and NADPH oxidase gene expression in Arabidopsis thaliana exposed to cadmium or excess copper. Funct. Plant Biol. 37, 532–544. doi: 10.1071/FP09194
Richards, S. L., Laohavisit, A., Mortimer, J. C., Shabala, L., Swarbreck, S. M., Shabala, S., et al. (2014). Annexin 1 regulates the H2O2-induced calcium signature in Arabidopsis thaliana roots. Plant J. 77, 136–145. doi: 10.1111/tpj.12372
Rockel, P., Strube, F., Rockel, A., Wildt, J., and Kaiser, W. M. (2002). Regulation of nitric oxide (NO) production by plant nitrate reductase in vivo and in vitro. J. Exp. Bot. 53, 103–110. doi: 10.1093/jexbot/53.366.103
Sathiyaraj, G., Srinivasan, S., Kim, Y. J., Lee, O. R., Parvin, S., Balusamy, S. R. D., et al. (2014). Acclimation of hydrogen peroxide enhances salt tolerance by activating defense-related proteins in Panax ginseng C.A. Meyer. Mol. Biol. Rep. 41, 3761–3771. doi: 10.1007/s11033-014-3241-3
Serrano, I., Romero-Puertas, M. C., Rodríguez-Serrano, M., Sandalio, L. M., and Olmedilla, A. (2012). Peroxynitrite mediates programmed cell death both in papillar cells and in self-incompatible pollen in the olive (Olea europaea L.). J. Exp. Bot. 63, 1479–1493. doi: 10.1093/jxb/err392
Serrano, M. L., Luque, M. E., and Sánchez, S. S. (2015). Xepac protein and IP3/Ca2+ pathway implication during Xenopus laevis vitellogenesis. Zygote 23, 99–110. doi: 10.1017/S0967199413000324
Seybold, H., Trempel, F., Ranf, S., Scheel, D., Romeis, T., and Lee, J. (2014). Ca2+ signalling in plant immune response: from pattern recognition receptors to Ca2+ decoding mechanisms. New Phytol. 204, 782–790. doi: 10.1111/nph.13031
Shan, C., Yan, Z., and Liu, M. (2015). Nitric oxide participates in the regulation of the ascorbate-glutathione cycle by exogenous jasmonic acid in the leaves of wheat seedlings under drought stress. Protoplasma 252, 1397–1405. doi: 10.1007/s00709-015-0756-y
Shi, C., Qi, C., Ren, H. Y., Huang, A. X., Hei, S. M., and She, X. P. (2015). Ethylene mediates brassinosteroid-induced stomatal closure via Gα protein-activated hydrogen peroxide and nitric oxide production in Arabidopsis. Plant J. 82, 280–301. doi: 10.1111/tpj.12815
Shi, K., Li, X., Zhang, H., Zhang, G., Liu, Y., Zhou, Y., et al. (2015). Guard cell hydrogen peroxide and nitric oxide mediate elevated CO2-induced stomatal movement in tomato. New Phytol. 208, 342–353. doi: 10.1111/nph.13621
Shi, Y., Liu, J., Xin, N., Gu, R., Qin Zhu, L., Zhang, C., et al. (2015). Signals induced by exogenous nitric oxide and their role in controlling brown rot disease caused by monilinia fructicola in postharvest peach fruit. J. Gen. Plant Pathol. 81, 68–76. doi: 10.1007/s10327-014-0562-y
Shoresh, M., Spivak, M., and Bernstein, N. (2011). Involvement of calcium-mediated effects on ROS metabolism in the regulation of growth improvement under salinity. Free Radic. Biol. Med. 51, 1221–1234. doi: 10.1016/j.freeradbiomed.2011.03.036
Skiba, U., Smith, K. A., and Fowler, D. (1993). Nitrification and denitrification as sources of nitric oxide and nitrous oxide in a sandy loam soil. Soil Biol. Biochem. 25, 1527–1536. doi: 10.1016/0038-0717(93)90007-X
Stöhr, C., Strube, F., Marx, G., Ullrich, W. R., and Rockel, P. (2001). A plasma membrane-bound enzyme of tobacco roots catalyses the formation of nitric oxide from nitrite. Planta 212, 835–841. doi: 10.1007/s004250000447
Tan, J., Wang, C., Xiang, B., Han, R., and Guo, Z. (2013). Hydrogen peroxide and nitric oxide mediated cold- and dehydration-induced myo-inositol phosphate synthase that confers multiple resistances to abiotic stresses. Plant Cell Environ. 36, 288–299. doi: 10.1111/j.1365-3040.2012.02573.x
Tang, R. J., Zhao, F. G., Garcia, V. J., Kleist, T. J., Yang, L., Zhang, H. X., et al. (2015). Tonoplast CBL–CIPK calcium signaling network regulates magnesium homeostasis in Arabidopsis. Proc. Natl. Acad. Sci. U.S.A. 112, 3134–3139. doi: 10.1073/pnas.1420944112
Tanou, G., Job, C., Belghazi, M., Molassiotis, A., Diamantidis, G., and Job, D. (2010). Proteomic signatures uncover hydrogen peroxide and nitric oxide cross-talk signaling network in citrus plants. J. Proteome Res. 9, 5994–6006. doi: 10.1021/pr100782h
Tanou, G., Job, C., Rajjou, L., Arc, E., Belghazi, M., Diamantidis, G., et al. (2009). Proteomics reveals the overlapping roles of hydrogen peroxide and nitric oxide in the acclimation of citrus plants to salinity. Plant J. 60, 795–804. doi: 10.1111/j.1365-313X.2009.04000.x
Tepe, H. D., and Aydemir, T. (2015). Protective effects of Ca2+ against NaCl induced salt stress in two lentil (Lens culinaris) cultivars. Afr. J. Agr. Res. 10, 2389–2398. doi: 10.5897/AJAR2014.9479
Tossi, V., Lamattina, L., Jenkins, G. I., and Cassia, R. O. (2014). Ultraviolet-B-induced stomatal closure in Arabidopsis is regulated by the UV RESISTANCE LOCUS8 photoreceptor in a nitric oxide-dependent mechanism. Plant Physiol. 164, 2220–2230. doi: 10.1104/pp.113.231753
Urao, T., Katagiri, T., Mizoguchi, T., Yamaguchi-Shinozaki, K., Hayashida, N., and Shinozaki, K. (1994). Two genes that encode Ca2+-dependent protein kinases are induced by drought and high-salt stresses in Arabidopsis thaliana. Mol. Gen. Genet. 244, 331–340. doi: 10.1007/BF00286684
Vandelle, E., Poinssot, B., Wendehenne, D., Bentejac, M., and Alain, P. (2006). Integrated signaling network involving calcium, nitric oxide, and active oxygen species but not mitogen-activated protein kinases in BcPG1-elicited grapevine defenses. Mol. Plant Microbe Interact. 19, 429–440. doi: 10.1094/MPMI-19-0429
Vavilala, S. L., Gawde, K. K., Sinha, M., and D'Souza, J. S. (2015). Programmed cell death is induced by hydrogen peroxide but not by excessive ionic stress of sodium chloride in the unicellular green alga Chlamydomonas reinhardtii. Eur. J. Phycol. 50, 422–438. doi: 10.1080/09670262.2015.1070437
Wagner, S., Behera, S., De Bortoli, S., Logan, D. C., Fuchs, P., Carraretto, L., et al. (2015). The EF-Hand Ca2+ binding protein MICU choreographs mitochondrial Ca2+ dynamics in Arabidopsis. Plant Cell 27, 3190–3212. doi: 10.1105/tpc.15.00509
Wang, L., Guo, Y., Jia, L., Chu, H., Zhou, S., Chen, K., et al. (2014). Hydrogen peroxide acts upstream of nitric oxide in the heat shock pathway in Arabidopsis seedlings. Plant Physiol. 164, 2184–2196. doi: 10.1104/pp.113.229369
Wang, P., Zhu, J. K., and Lang, Z. (2015). Nitric oxide suppresses the inhibitory effect of abscisic acid on seed germination by s-nitrosylation of snrk2 proteins. Plant Signal. Behav. 10:e1031939. doi: 10.1080/15592324.2015.1031939
Wang, W. H., Yi, X. Q., Han, A. D., Liu, T. W., Chen, J., Wu, F. H., et al. (2011). Calcium-sensing receptor regulates stomatal closure through hydrogen peroxide and nitric oxide in response to extracellular calcium in Arabidopsis. J. Exp. Bot. 63, 177–190. doi: 10.1093/jxb/err259
Wang, Y., Chen, T., Zhang, C., Hao, H., Liu, P., Zheng, M., et al. (2009). Nitric oxide modulates the influx of extracellular Ca2+ and actin filament organization during cell wall construction in pinus bungeana pollen tubes. New Phytol. 182, 851–862. doi: 10.1111/j.1469-8137.2009.02820.x
Wang, Y., Zhang, J., Li, J. L., and Ma, X. R. (2014). Exogenous hydrogen peroxide enhanced the thermotolerance of Festuca arundinacea and Lolium perenne by increasing the antioxidative capacity. Acta Physiol. Plant 36, 2915–2924. doi: 10.1007/s11738-014-1661-2
Wen, J. F., Gong, M., Liu, Y., Hu, J. L., and Deng, M. H. (2013). Effect of hydrogen peroxide on growth and activity of some enzymes involved in proline metabolism of sweet corn seedlings under copper stress. Sci. Horticult. 164, 366–371. doi: 10.1016/j.scienta.2013.09.031
Willekens, H., Chamnongpol, S., Davey, M., Schraudner, M., Langebartels, C., Van Montagu, M., et al. (1997). Catalase is a sink for H2O2 and is indispensable for stress defence in C3 plants. EMBO. J. 16, 4806–4816. doi: 10.1093/emboj/16.16.4806
Wu, A. P., Gong, L., Chen, X., and Wang, J. X. (2014). Interactions between nitric oxide, gibberellic acid, and phosphorus regulate primary root growth in Arabidopsis. Biol. Plant. 58, 335–340. doi: 10.1007/s10535-014-0408-7
Wu, D., Chu, H. Y., Jia, L. X., Chen, K. M., and Zhao, L. Q. (2015). A feedback inhibition between nitric oxide and hydrogen peroxide in the heat shock pathway in arabidopsis seedlings. Plant Growth Regul. 75, 503–509. doi: 10.1007/s10725-014-0014-x
Wu, J., Shang, Z., Wu, J., Jiang, X., Moschou, P. N., Sun, W., et al. (2010). Spermidine oxidase-derived H2O2 regulates pollen plasma membrane hyperpolarization-activated Ca2+-permeable channels and pollen tube growth. Plant J. 63, 1042–1053. doi: 10.1111/j.1365-313X.2010.04301.x
Xiang, Z., Jin, W., Jing, Y., Wang, X. L., Zhao, Q. P., Kong, P. T., et al. (2015). Nitric oxide-associated protein1 (atnoa1) is essential for salicylic acid-induced root waving in Arabidopsis thaliana. New Phytol. 207, 211–224. doi: 10.1111/nph.13327
Yu, Y., Yang, Z., Guo, K., Li, Z., Zhou, H., Wei, Y., et al. (2015). Oxidative damage induced by heat stress could be relieved by nitric oxide in trichoderma harzianum LTR-2. Curr. Microbiol. 70, 618–622. doi: 10.1007/s00284-014-0764-8
Zhang, H., Wang, W., Yin, H., Zhao, X., and Du, Y. (2012). Oligochitosan induces programmed cell death in tobacco suspension cells. Carbohyd. Polym. 87, 2270–2278. doi: 10.1016/j.carbpol.2011.10.059
Zhang, F., Wang, Y., Yang, Y., Wu, H., Wang, D., and Liu, J. (2007). Involvement of hydrogen peroxide and nitric oxide in salt resistance in the calluses from Populus euphratica. Plant Cell Environ. 30, 775–785. doi: 10.1111/j.1365-3040.2007.01667.x
Zhou, L., Lan, W., Jiang, Y., Fang, W., and Luan, S. (2014). A calcium-dependent protein kinase interacts with and activates a calcium channel to regulate pollen tube growth. Mol. Plant 7, 369–376. doi: 10.1093/mp/sst125
Zou, J. J., Li, X. D., Ratnasekera, D., Wang, C., Liu, W. X., Song, L. F., et al. (2015). Arabidopsis Calcium-Dependent Protein Kinse8 and Catalase3 function in Abscisic Acid-mediated signaling and H2O2 homeostasis in stomatal guard cells under drought stress. Plant Cell 27, 1445–1460. doi: 10.1105/tpc.15.00144
Keywords: hydrogen peroxide (H2O2), nitric oxide (NO), calcium (Ca2+), signal molecule, crosstalk
Citation: Niu L and Liao W (2016) Hydrogen Peroxide Signaling in Plant Development and Abiotic Responses: Crosstalk with Nitric Oxide and Calcium. Front. Plant Sci. 7:230. doi: 10.3389/fpls.2016.00230
Received: 14 November 2015; Accepted: 11 February 2016;
Published: 04 March 2016.
Edited by:
Cristina Ortega-Villasante, Universidad Autónoma de Madrid, SpainReviewed by:
Eva-Mari Aro, University of Turku, FinlandClay Carter, University of Minnesota Twin Cities, USA
Copyright © 2016 Niu and Liao. This is an open-access article distributed under the terms of the Creative Commons Attribution License (CC BY). The use, distribution or reproduction in other forums is permitted, provided the original author(s) or licensor are credited and that the original publication in this journal is cited, in accordance with accepted academic practice. No use, distribution or reproduction is permitted which does not comply with these terms.
*Correspondence: Weibiao Liao, bGlhb3diQGdzYXUuZWR1LmNu