- 1Jiangsu Collaborative Innovation Center of Regional Modern Agriculture and Environment Protection, Jiangsu Key Laboratory for Eco-Agriculture Biotechnology around Hongze Lake, Huaiyin Normal University, Huaian, China
- 2Crop Protection and Management Research Unit, Agricultural Research Service – United States Department of Agriculture, Tifton, GA, USA
- 3Department of Plant Pathology, The University of Georgia, Tifton, GA, USA
- 4Crop Genetics and Breeding Research Unit, Agricultural Research Service – United States Department of Agriculture, Tifton, GA, USA
- 5Department of Plant Production, College of Food and Agricultural Sciences, King Saud University, Riyadh, Saudi Arabia
- 6Key Laboratory of Biodiversity and Biogeography, Kunming Institute of Botany, Institute of Tibet Plateau Research at Kunming, Chinese Academy of Sciences, Kunming, China
To clarify the roles of carbon monoxide (CO), nitric oxide (NO), and auxin in the plant response to iron deficiency (–Fe), and to establish how the signaling molecules interact to enhance Fe acquisition, we conducted physiological, genetic, and molecular analyses that compared the responses of various Arabidopsis mutants, including hy1 (CO deficient), noa1 (NO deficient), nia1/nia2 (NO deficient), yuc1 (auxin over-accumulation), and cue1 (NO over-accumulation) to –Fe stress. We also generated a HY1 over-expression line (named HY1-OX) in which CO is over-produced compared to wild-type. We found that the suppression of CO and NO generation using various inhibitors enhanced the sensitivity of wild-type plants to Fe depletion. Similarly, the hy1, noa1, and nia1/nia2 mutants were more sensitive to Fe deficiency. By contrast, the yuc1, cue1, and HY1-OX lines were less sensitive to Fe depletion. The hy1 mutant with low CO content exhibited no induced expression of the Fe uptake-related genes FIT1 and FRO2 as compared to wild-type plants. On the other hand, the treatments of exogenous CO and NO enhanced Fe uptake. Likewise, cue1 and HY1-OX lines with increased endogenous content of NO and CO, respectively, also exhibited enhanced Fe uptake and increased expression of bHLH transcriptional factor FIT1as compared to wild-type plants. Furthermore, we found that CO affected auxin accumulation and transport in the root tip by altering the PIN1 and PIN2 proteins distribution that control lateral root structure under –Fe stress. Our results demonstrated the integration of CO, NO, and auxin signaling to cope with Fe deficiency in Arabidopsis.
Introduction
Iron deficiency (–Fe) severely limits the yield of crops growing in calcareous soils (Curie and Briat, 2003). Although Fe is abundant in the soil, Fe availability is limited due to its poor solubility in aerobic and neutral pH environments (Briat et al., 1995). Plants have evolved two distinct strategies of Fe acquisition to cope with –Fe (Imsande, 1998). Non-graminaceous plants acidify the extracellular medium surrounding the roots and improve the ferric-reducing capability of the roots to enhance ferrous Fe uptake (Strategy I), whereas graminaceous plants secrete phytosiderophores to enhance Fe uptake from the soil (Strategy II) (Marschner, 2011). In Arabidopsis (Arabidopsis thaliana), ferric Fe reduction is mainly achieved through the activity of Fe reductase, FRO2 (Ferric Reductase Oxidase 2), on the surface of roots. Ferrous Fe is then absorbed through the root epidermis by the metal transporter IRT1 (Iron-Regulated Transporter 1) (Vert et al., 2002). Fe-Deficiency Induced Transcription Factor 1 (FIT1) forms heterodimers with basic Helix-Loop-Helix (bHLH) 38 and bHLH39 to regulate Fe acquisition (Yuan et al., 2008). Furthermore, two other bHLH proteins, bHLH 100 and bHLH 101, are highly induced by –Fe, which indicates their involvement in Fe acquisition (Wang et al., 2007; Sivitz et al., 2012). The intercellular distribution of Fe throughout the plant also affects Fe availability. Ferric Reductase Defective 3 (FRD3), an efflux transporter of ion chelator citrate, facilitates root-to-shoot circulation of iron in the xylem sap (Green and Rogers, 2004). Nicotianamine (NA) is a low molecular mass metal chelator with a high binding affinity for a range of transition elements including Fe. The Fe transporter Yellow Stripe-like 1 (YSL1) moves Fe (II)-NA from the xylem to the phloem (Le Jean et al., 2005). Various signaling molecules and plant hormones including nitric oxide (NO), auxin and ethylene modulate the response to –Fe by plants (Graziano and Lamattina, 2007; Chen et al., 2010; Lingam et al., 2011; Meiser et al., 2011). In addition, Fe uptake is regulated at the post-transcriptional level. For instance, FIT and IRT undergo ubiquitin-dependent protein degradation in relation to Fe availability (Barberon et al., 2011; Meiser et al., 2011). However, many of the mechanisms underlying Fe acquisition during plant exposure to –Fe remain to be identified and characterized.
Carbon monoxide (CO), an important reactive trace gas in the troposphere, has recently been shown to be generated in animals through the activity of heme oxygenase (HY; EC1.14.99.3), and is an essential regulator (Otterbein et al., 2000). Recent research in plants suggests that CO plays a role in root formation, and in the response to saline and heavy metal stress (Guo et al., 2008; Han et al., 2008; Xuan et al., 2008; Xie et al., 2011). Likewise, NO is a free radical gas involved in the regulation of multiple physiological functions in animals and plants (Wendehenne et al., 2004; Yang et al., 2013, 2015). In plants, NO is generated through the activities of nitrate reductase (NR) and NO synthase (NOS)-like enzymes, and possibly also non-enzymatic processes (Besson-Bard et al., 2008; Scheler et al., 2013). Under chilling stress, NO normally interacts with CO to regulate seed germination (Bai et al., 2012). In Arabidopsis, long-hypocotyl protein 1 (HY1), a heme oxygenase, has been shown to participate in the biosynthesis of the phytochrome chromophore (Davis et al., 1999). Recently the role of HY1 in CO synthesis has drawn much attention (Han et al., 2008; Xuan et al., 2008; Xie et al., 2011). A previous study suggests that HY1-dependent CO generation induces the subsequent accumulation of NO, thereby improving the tolerance of Arabidopsis to –Fe (Li et al., 2013). Auxin also induces NO accumulation which increases the activity of ferric chelate reductase (FCR) to promote Fe uptake in Arabidopsis subjected to –Fe (Chen et al., 2010). It has been suggested that there are possible interplays among CO, NO, and auxin signaling upon plant exposure to –Fe. However, the patterns of these signaling interactions in response to iron depletion remains to be characterized further.
Materials and Methods
Plant Growth and Treatments
The Columbia ecotype (Col-0) of Arabidopsis served as wild-type and all mutants in the Col-0 background were obtained from the Arabidopsis stock center (Scholl et al., 2000), including the HY1 null mutant hy1, the auxin-insensitive mutants axr1-3 (Lincoln et al., 1990), the auxin-transport mutant aux1-7 (Pickett et al., 1990), the auxin-overproducing mutant yuc1 (Zhao et al., 2001), the NO overproducing mutant cue1 (He et al., 2004), the NOA-deficient mutant noa1 (Guo and Crawford, 2005), the NR-null-deficient double mutant nr (nia1/nia2), the auxin-related reporter lines DR5:GFP (Ottenschläger et al., 2003), AUX1-YFP (Giehl et al., 2012), PIN1-GFP (Ottenschläger et al., 2003), and PIN2-GFP (Ottenschläger et al., 2003). The double mutants, yuc1/hy1 and hy1/cue1, were generated by crossing. The resulting homozygous lines were identified and isolated using the PCR primers listed in Supplementary Table S1.
Seeds were surface sterilized, kept in dark at 4°C for three days, and then germinated on 0.9% (w/v) agar plates supplemented with 1.0% (w/v) sucrose and a nutrient solution, consisting of 300 μM Ca(NO3)2, 50 μM MgSO4, 30 μM NaH2PO4, 50 μM K2SO4, 3 μM H3BO3, 0.4 μM ZnSO4, 0.2 μM CuSO4, 0.5 μM MnCl2, 1 μM (NH4)6(Mo7)O24, and 20 μM Fe-EDTA at pH 6.5 (KOH). One-week old seedlings were transferred into vermiculite supplemented with the same nutrient solution as described above. After another week, the seedlings were transplanted from vermiculite into the compartments of 1 L hydroponic holders filled with the nutrient solution described above with aeration. The nutrient solution was refreshed every other day. The seedlings were then grown at 21°C with a relative humidity of 70% and a daily light-dark cycle of 10 and 14 h. The daytime light intensity was 200 to 250 μmol photons m-2s-1. One week later, plants were transferred into the media containing either 20 μM (+Fe) or 0 μM (–Fe) FeNaEDTA for another week. The media was refreshed daily.
Generation of Transgenic HY1-6HA-Overexpressing Plants
The cDNA of the HY1 gene was synthesized through reverse transcription and served as the template to PCR amplify HY1 fragments using the primers listed in Supplementary Table S1. The resulting HYI fragment was cloned into a pMD18 T-vector. The HY1 insert was then excised using EcoRI and BamHI, and cloned into the EcoRI and BamHI sites of the pOE-6HA vector to obtain the expression vector pOE-HY1-6HA, in which the coding region of HY1 was fused with 5′ end of a 6XHA (hemagglutinin) reporter tag. pOE-HY1-6HA was then transformed into Col-0 plants using Agrobacterium tumefaciens strain GV3101. Transgenic seeds were screened based on Basta resistance and the homozygous transgenic lines, named HY1-OX, were further verified by PCR and immunoblot analysis using anti-HA antibodies.
Chemical/Inhibitor Treatments
The final concentrations of chemicals added to the culture solutions were as follows: 100 nM NAA, 10 μM NPA, 30 μM GSNO, 30 μM SNAP, 100 nM CORM2, 1 mM L-NAME, 10 mM tungstate, 300 μM cPTIO, and 200 μM ZnPPIX. Prior to inhibitor treatment, all lines were initially grown in +Fe media for 2 weeks. Afterward, all lines were transferred to –Fe media or –Fe media supplemented with various inhibitors and cultured for 1 week. Lines transferred to new +Fe media served as controls. After the indicated periods of treatments, the seedlings were collected for further analysis.
CO Quantification and Assay of HY1 Enzyme Activity
Carbon monoxide in plant tissues was quantified using a previously described method with minor modifications (Bai et al., 2012). Briefly using liquid nitrogen, 0.5 g of treated plants were ground into a fine powder and transferred into a 4 ml bottle. Samples were stored under vacuum conditions in an ultralow chiller prior to further processing. To avoid foaming, 20 μl 1-octanol and 1 ml distilled water were sequentially added to each bottle. Each bottle was then immediately capped and shaken vigorously for approximately 30 s. One milliliter of sulfuric acid was injected into each bottle through the rubber cap using a syringe with a needle. Bottles were shaken briefly, and then placed into a 70°C water bath for 3 h with occasional shakings. After each bottle was cooled down to room temperature, 1 ml of air from the headspace was taken to quantify the CO concentration using a GC/MS system. The HY1 enzyme activity was determined as previously described (Bai et al., 2012).
NO In situ Measurement
Nitric oxide content in root was quantified using DAF-FMDA under epifluorescence microscopy (Guo et al., 2003; Chen et al., 2010). Five millimeter of root tip segments were soaked in 20 mM HEPES/NaOH buffer (pH 7.4) supplemented with 5 mM DAF-FMDA for 20 min. After washing three times with 20 mM HEPES/NaOH buffer, the root tips were analyzed microscopically (Nikon Eclipse 80i, Nikon, EX 460-500, DM 505, BA 510-560). The intensities of the green fluorescence from the root tips were quantified by measuring the average pixel intensity with Photoshop software (Adobe Systems) (Guo et al., 2003). Data are presented as the mean percentages of fluorescence intensity relative to that of the wild-type plants grown under the same conditions.
Measurement of Fe Content
Seedlings were washed for 5 min in a solution containing 5 mM CaSO4 and 10 mM EDTA, and rinsed briefly in de-ionized water prior to further processing. Roots and shoots were cut into smaller pieces, and dehydrated at 70°C. A hundred milligrams of the dried tissues were digested completely in 70% HNO3 at 120°C. The Fe content was determined using an Inductively Coupled Plasma-Optical Emission Spectrometer (ICP–OES, Perkin Elmer Optima 2100DV).
Immunoblot Analysis
Total proteins of roots were prepared by grinding on ice with an extraction buffer consisting of 50 mM Tris, 5% glycerol, 4% SDS, 1% polyvinylpolypyrrolidone, and 1 mM phenylmethylsulfonyl fluoride (pH 8.0), followed by 14,000 × g centrifugation at 4°C for 15 min. Fifteen milligrams of total protein were separated by electrophoresis on a 12% SDS–polyacrylamide gel and blotted onto polyvinylidene difluoride membranes, which were then probed with the appropriate primary anti-HA antibody (1:1000) and horseradish peroxidase-conjugated goat anti-mouse secondary antibody (1:3000, Promega). Protein levels were visualized using an ECL Kit (GE healthcare, USA).
Assay of Auxin Polar Basipetal Transport
Auxin transport was assayed using [3H] IAA as described previously (Guo et al., 2003). Briefly, the primary roots of 12-days-old seedlings were removed from intact plants. Then the seedlings, without primary roots, were placed onto petri dishes with 0.8% agar supplemented with half-strength Murashige and Skoog medium and additional chemicals for various treatments. For the assay of auxin polar basipetal transport, ten 1 cm hypocotyl segments excised below the cotyledons were carefully transferred into a microcentrifuge tube containing 0.1 mL of [3H]IAA (American Radiolabeled Chemicals) in an orientation of apical end down. After incubation for various periods, 0.2 cm segments from the apical end were excised and used to measure radioactivity in a liquid scintillation counter. The non-polar transport of auxin in the segments was determined by adding NPA (Chem Service) to the medium or by reversing the orientation of the stem segments in the medium.
Determination of FCR Activity in Roots
Ferric Chelate Reductase activity was determined according to a method reported previously (Chen et al., 2010). Briefly, 0.1 g of the whole roots excised from seedlings were transferred into a test tube filled with 5 mL of assay solution consisting of 0.5 mM CaSO4, 0.1 mM 4-morpho-lineethanesulfonic acid, 0.1 mM bathophenanthrolinedisulfonic acid disodium salt hydrate (BPDS), and 100 mM Fe-EDTA (pH 5.5 using NaOH). The tubes were kept in the dark at room temperature for 1 h with brief swirling by hand every 10 min. The absorbance of samples at 535 nm was measured using a spectrophotometer, and the concentration of Fe(II)[BPDS]3 was calculated using an extinction coefficient of 22.14 mM-2⋅cm-2. Data were expressed as the mean percentages of FCR activity of the wild-type control grown on +Fe medium. To localize the distribution of FCR activities in roots, roots excised from the seedlings were embedded in 0.75% agarose medium containing 0.5 mM CaSO4, 0.5 mM FeNaEDTA, and 0.5 mM ferrozine. Roots were then incubated at room temperature for 20 min. Color patterns of 5 mm root tips were imaged by light microscopy (Nikon Eclipse 80i, Nikon).
RNA Extraction and qRT-PCR
Total RNA was extracted from Arabidopsis seedlings using Trizol reagent (Invitrogen, USA). qRT-PCR was performed as described previously (Chen et al., 2010). Briefly, 1.5 μg of DNA-free RNA served as the template to synthesize first-strand cDNA in a 20 μL reaction using a Thermo Scientific RevertAid First Strand cDNA Synthesis Kit (Thermo Scientific, USA) and oligo (dT)18 primer. qRT-PCR was performed using 2x SYBR Green I Master Mix on a Roche Light Cycler 480 real-time PCR machine, according to the manufacturer’s instructions. At least three biological replicates were analyzed. ACTIN2 served as an internal control of gene expression. Gene-specific primers that were used are listed in Supplementary Table S1.
GUS Staining
The HY1 promoter was cloned into the pSUNG vector using the primers listed in Supplementary Table S1. The resulting pHY1:GUS vector was transformed into Col-0 using the floral dip method (Clough and Bent, 1998). Basta-resistant T2 seedlings was used in the GUS staining assay as described previously (Bai et al., 2012).
Histochemical Analysis
The activities of FCR and chlorophyll content were measured as described previously (Long et al., 2010). To localize Fe3+, 2-week-old seedlings were vacuum infiltrated with Perl’s stain solution containing 2% HCI and 2% K-ferrocyanide for 30 min. Seedlings were then incubated for another 30 min in Perl’s staining solution. After washing three times with distilled water, seedlings were imaged using a Leica DM500B microscope.
Other Methods
Root growth rates were measured by marking the position of the root tips daily. Two-week-old seedlings were scanned and ImageJ software was used to measure root length. Lateral roots and lateral root primordia were counted under light microscopy.
Rhizosphere acidification was performed as described previously (Long et al., 2010). Briefly, seeds were germinated in +Fe medium and then were transferred into –Fe medium and grown for 1 week. The seedlings were then transferred onto a 1% agar plate (pH 6.5 using NaOH) containing 0.006% Bromocresol Purple and 0.2 mM CaSO4 and cultured for 24 h.
Results
Fe Depletion Induces Heme Oxygenase Activity and CO Production
To understand the regulatory role of CO in Arabidopsis plants exposed to –Fe, we first obtained HY1-null lines hy1-100 (hereafter hy1), which harbors a single base pair mutation in the second exon of the HY1 gene encoding heme oxygenase. The hy1 mutant had yellowish leaves and longer hypocotyls as compared to wild-type Columbia after 5 days of continuous white light (Davis et al., 1999). We also generated several independent transgenic lines in which HY1-6HA was constitutively expressed under the control of CaMV 35S promoter. Among them, HY1-6HA expression was up-regulated most strongly in the line 3 and was named HY1-OX (Supplementary Figure S1). Thus, this line was used in the following experiments. First, we measured the heme oxygenase activity and CO release after Fe depletion for wild-type, hy1, and HY1-OX plants. As shown in Figure 1A, –Fe gradually induced heme oxygenase activity and CO production in the wild-type as well as increased HY1 transcript abundance (Supplementary Figure S2). Compared to the wild-type, the hy1 mutant, impaired in heme oxygenase activity, produced less CO. We also generated a transgenic line, pHY1:GUS, in which the promoter region of HY1 drives the expression of the beta-glucuronidase (GUS) reporter gene. Consistent with the findings described above, the pHY1:GUS reporter line showed enhanced GUS staining under Fe depletion compared to wild-type (Figure 1B), indicating HY1 expression and enzyme activity is inducible by Fe deficiency.
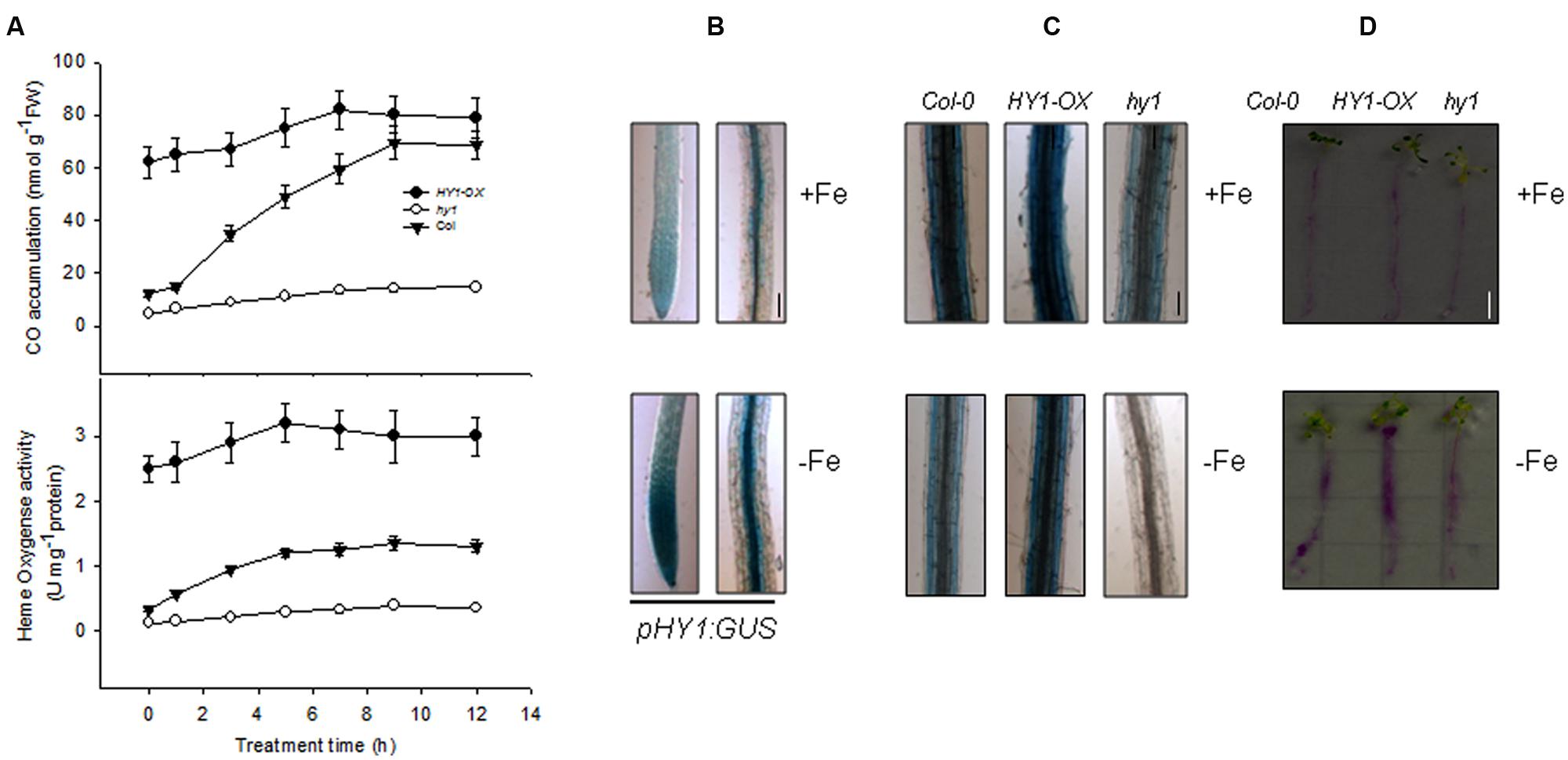
FIGURE 1. Induction of heme oxygenase activity and CO generation by –Fe. (A) –Fe induced heme oxygenase activity and CO generation. Two-week-old wild-type Col-0, hy1 seedlings and HY1-OX lines were transferred to –Fe hydroponic medium, and heme oxygenase activity and CO generation were measured at the indicated time points. Data are the means ± SD (n = 6). (B) –Fe induced the transcription of HY1. Two-week-old wild-type Col-0 or transgenic pHY1:GUS seedlings were shifted to –Fe hydroponic medium, and the seedlings were subjected to GUS staining after 3 days of –Fe stress. Bar = 0.1 cm. (C,D) Perl staining analysis of Col-0, hy1, and 2-week-old HY1-OX plants subjected to –Fe stress. Two-week-old Col-0, hy1, and HY1-OX seedlings were shifted to –Fe liquid media for 1 week and the roots were analyzed using a ferrozine assay©, or to –Fe solid media containing Bromocresol Purple (D) for 1 day. Red indicates acidification. Bar = 0.5 cm.
To determine the role of CO in regulating Fe uptake, we measured Fe content using two distinct techniques. Perl staining revealed that the wild-type and HY1-OX line contained more ferric Fe in their roots compared to the hy1 line defective in the activity of heme oxygenase under Fe depletion (Figure 1C). The abundance of ferric Fe was also reduced in the wild-type and HY1-OX under –Fe, but ferric Fe was at such a low amount in the hy1 line that it was not detectable. Similarly, the acidification capability of wild-type and HY1-OX was markedly higher than the hy1 line under Fe depletion (Figure 1D). This suggests that HY1-dependent CO production plays a pivotal role in Fe uptake under Fe deficiency.
Carbon Monoxide Enhances the Activity of FCR and Increases Fe Uptake in Arabidopsis Exposed to Fe Deficiency
We recorded the visible phenotypes of wild-type, hy1, and HY1-OX under Fe depletion. The wild-type and HY1-OX line grew well under regular conditions, but hy1 was chlorotic (Figure 2A). Upon exposure to –Fe, the chlorophyll content and Fe content were both reduced in all lines as compared to +Fe (Figures 2B,C). However, the reductions were less in wild-type and HY1-OX plants as compared to hy1 (Figures 2B,C). FCR activity was enhanced upon exposure to –Fe stress in the wild-type and HY1-OX plants but not in the hy1 mutant. To determine whether CO is involved in the response to –Fe, we added the exogenous CO donor CORM2 to Fe-depleted medium. As shown in Figure 2B, the addition of CORM2 improved chlorophyll content and FCR activity in the wild-type and hy1 mutant. Furthermore, the addition of exogenous CO under –Fe conditions alone significantly improved Fe content in the wild-type and hy1 lines as compared to –Fe treatment (Figure 2C).
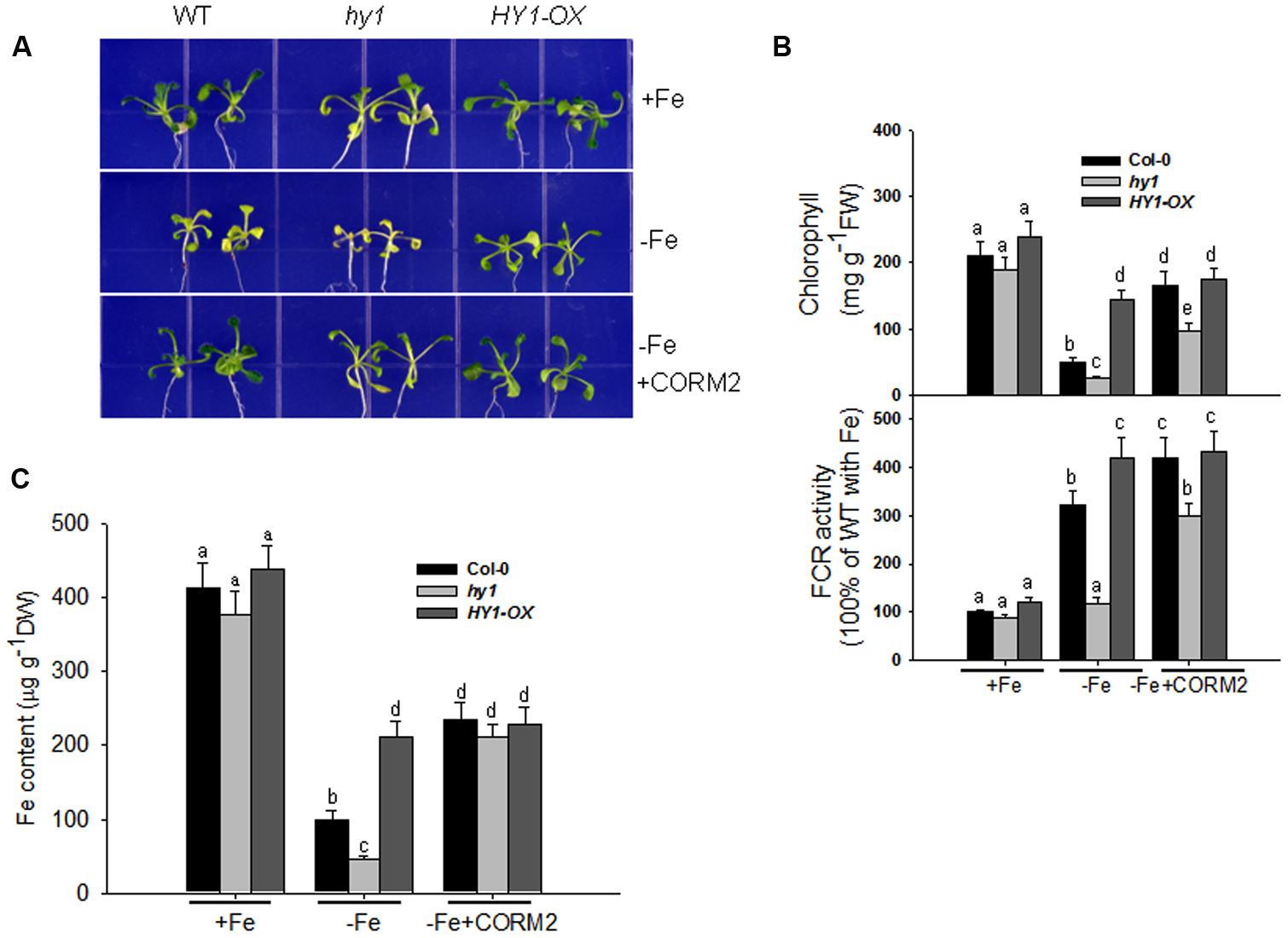
FIGURE 2. The differential responses of the hy1 mutant and HY1-OX line to Fe deficiency. (A) The phenotype of wild-type Col-0, hy1, and HY1-OX lines in response to –Fe stress. Upper: +Fe media; Middle panel: –Fe media; Bottom: –Fe media plus the CO donor, CORM2. Col-0, hy1, and HY1-OX seeds were germinated for 7 days and then grown on vermiculite with fresh complete nutrient solution for another 7 days. The seeds were transferred to –Fe hydroponic media or –Fe hydroponic media plus 100 nM CORM2 for 1 week and then photographed. Seedlings growing in the +Fe hydroponic media were used as the positive control. (B,C) Quantification of chlorophyll content (B), FCR activity (B), and Fe content (C) of plants growing in +Fe or –Fe hydroponic media. Two-week-old seedlings of the Col-0, hy1, and HY1-OX lines were grown in +Fe hydroponic media, were shifted to –Fe hydroponic media for 1 week, and the chlorophyll content and FCR activity were measured. Seedlings left in +Fe hydroponic media were used as the control. Data are the means ± SD (n = 12), and bars labeled with different letters are significantly different at p < 0.05 (Tukey’s test).
Auxin Induces HY1 Expression
Because auxin signaling is involved in the Arabidopsis response to –Fe (Chen et al., 2010), we deduced that auxin and CO signaling processes may interact somehow in response to –Fe. To this end, we examined the effect of auxin on HY1 expression. As shown in Figure 3A, the treatment of low concentration of NAA enhanced GUS staining in transgenic pHY1:GUS lines. Consistent with this observation, NAA treatment increased the abundance of HY1 transcripts (Figure 3B). We also measured the impact of auxin on CO production. As shown in Supplementary Figure S3, NAA treatment induced CO generation and activity of heme oxygenase. In the HY1-OX line, NAA treatment enhanced HY1 protein accumulation, and the effect was reduced if HY1-OX was treated with ZnPPIX, a specific inhibitor of HY1 (Figure 3C). Interestingly, two protein fragments were identified in the samples at all timepoints, suggesting that possible post-translational modification of HY1 occurs. These findings indicate the possible post-translational modification of HY1 after auxin treatment.
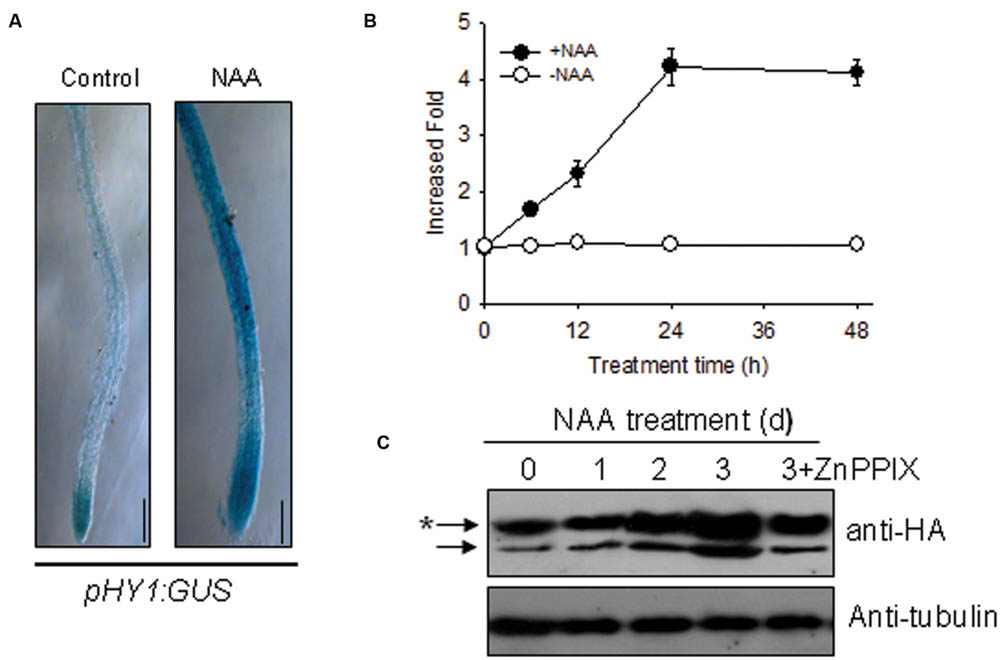
FIGURE 3. Auxin induced HY1 activity and HY1-dependent CO generation. (A) Auxin treatment induced GUS expression in the pHY1: GUS transgenic line. Two-week-old pHY1: GUS seedlings were treated with 100 nM NAA for 1 day, and HY1 expression was monitored by GUS staining. Seedlings not exposed to NAA treatment were used as a control. Bar = 0.2 cm. (B) Time-course qRT-PCR analysis of HY1 transcripts abundance after treatment with 100 nM NAA. (C) Auxin induced HY1-HA protein accumulation. Transgenic HY1-OX seedlings (termed HY1-OX) were treated with 100 nM NAA at the indicated time points, and HY1-HA accumulation was analyzed by immunoblot using anti-HA antibody. Blotting with an anti-tubulin antibody was used as a loading control. 3d+ZnPPIX: HY1-OX lines treated with 100 nM NAA plus 200 μM ZnPPIX for 3 days, before GFP-HY1 accumulation was measured. The asterisk indicates putative post-translational modification bands.
CO Modulates Auxin, the Expression of the Auxin Transport Components, and Root Hair Development Under Fe Deficiency
To gain insight into the role of CO in auxin signaling, we investigated the distribution of auxin in hy1 and HY1-OX lines in response to the change of CO levels. We used an Arabidopsis DR5:GFP line in which DR5, a synthetic auxin-inducible promoter, drives the expression of the GFP reporter gene (Ulmasov et al., 1997). The line is widely used to detect the accumulation and distribution of auxin (Ottenschläger et al., 2003; Müller and Sheen, 2008). We transferred the DR5:GFP cassette into hy1 and HY1-OX lines through genetic crossing. The analysis of confocal microscopy revealed that the GFP signal was stronger in the wild-type and HY1-OX lines than in the hy1 mutant grown on +Fe media, and that the difference was enhanced further under Fe depletion (Figure 4A). Moreover, the CO donor CORM-2 treatment restored the auxin level in the root tip of hy1 to that of the wild-type (Figure 4A). This may suggest that HY1-dependent CO production is positively correlated with the abundance of auxin in root tips.
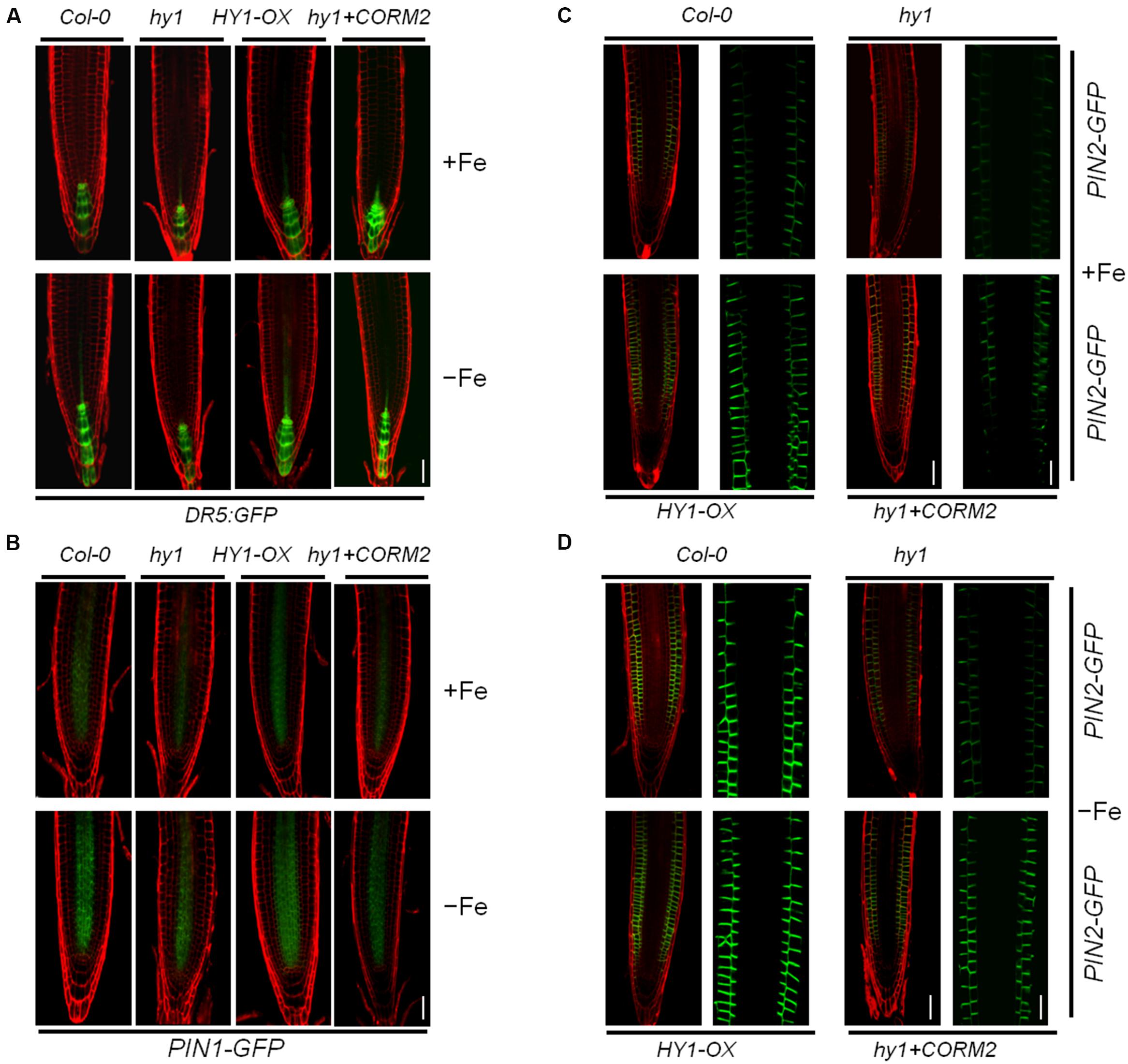
FIGURE 4. Iron deficiency stress altered the expression patterns of Dr5: GFP, PIN1-GFP, and PIN2-GFP in the root tip. (A,B) Confocal images of the DR5:GFP reporter (A) and PIN1-GFP (B) in Col-0, hy1, and HY1-OX lines treated with +Fe, –Fe, or –Fe +100 nM CORM2 for 1 day. (C,D) Confocal images of the PIN2-GFP reporter in Col-0, hy1, and HY1-OX lines treated with +Fe, –Fe, or –Fe +100 nM CORM2 for 3 days. Left: fluorescence images of the root tip; Right: enlarged window.
The flux of auxin from the shoot to the root is largely determined by the polar localization of auxin transporters, including the auxin influx carrier AUX1 and efflux carriers PIN1 and PIN2, on the plasma membrane (Blilou et al., 2005). To investigate the polar localization of AUX1 and PIN1/2 in wild-type, hy1, and HY1-OX, AUX1-YFP, PIN1-GFP, and PIN2-GFP constructs were introgressed into these lines by crossings. We found that Fe depletion for one day dramatically reduced the abundance of PIN2-GFP, and to a lesser extent, of PIN1-GFP and AUX1-YFP, in hy1 mutant compared with wild-type (Figures 4B,C; Supplementary Figure S4).
Iron deficiency also increased the root hair density in the wild-type and HY1-OX lines (Supplementary Figure S5A,B). The hy1 mutant impaired in CO generation did not have an increase in root hair density induced by –Fe yet the addition of exogenous CO increased root hair formation (Supplementary Figure S5A,B). A similar observation has been reported previously (Graziano and Lamattina, 2007). The CO signal was also involved in lateral root development (Supplementary Figure S5C). The HY1-OX line developed more lateral roots under +Fe or –Fe conditions, and the hy1 mutant had fewer lateral roots under –Fe conditions compared to the wild-type. –Fe treatment caused an increase in lateral root number in wild-type and HY1-OX lines, but not in hy1, which had fewer lateral roots even under +Fe conditions compared to wild-type (Supplementary Figure S5C). Addition of CORM2 under –Fe caused an increase in the root length of hy1 (Supplementary Figure S5C).
CO Increases FCR Activity, Fe Content, and Polar Auxin Transport but Requires Auxin Transporters
To further characterize the modulation of auxin in response to –Fe, we crossed the auxin over-accumulation mutant yuc1 with hy1 to obtain the double mutant yuc1/hy1 (Chen et al., 2010). Compared with hy1, the yuc1 mutant was less chlorotic under –Fe, indicating an increased tolerance to –Fe (Figure 5A). Compared to wild-type, yuc1 had increased iron content, FCR activity, and basipetal auxin transport after –Fe treatment for 1 week (Figure 5B). The addition of CORM-2 improved the growth of both the hy1 and yuc1/hy1 (Figure 5A). HY1-OX and yuc1 had higher root Fe content and higher FCR activity after exposure to –Fe stress compared to wild-type (Figures 5B,C). Like hy1, yuc1/hy1 double mutant had lower Fe content and FCR activity as compared to the wild-type plants (Figures 5B,C), implying that HY1 activity is essential/required for Fe acquisition during –Fe.
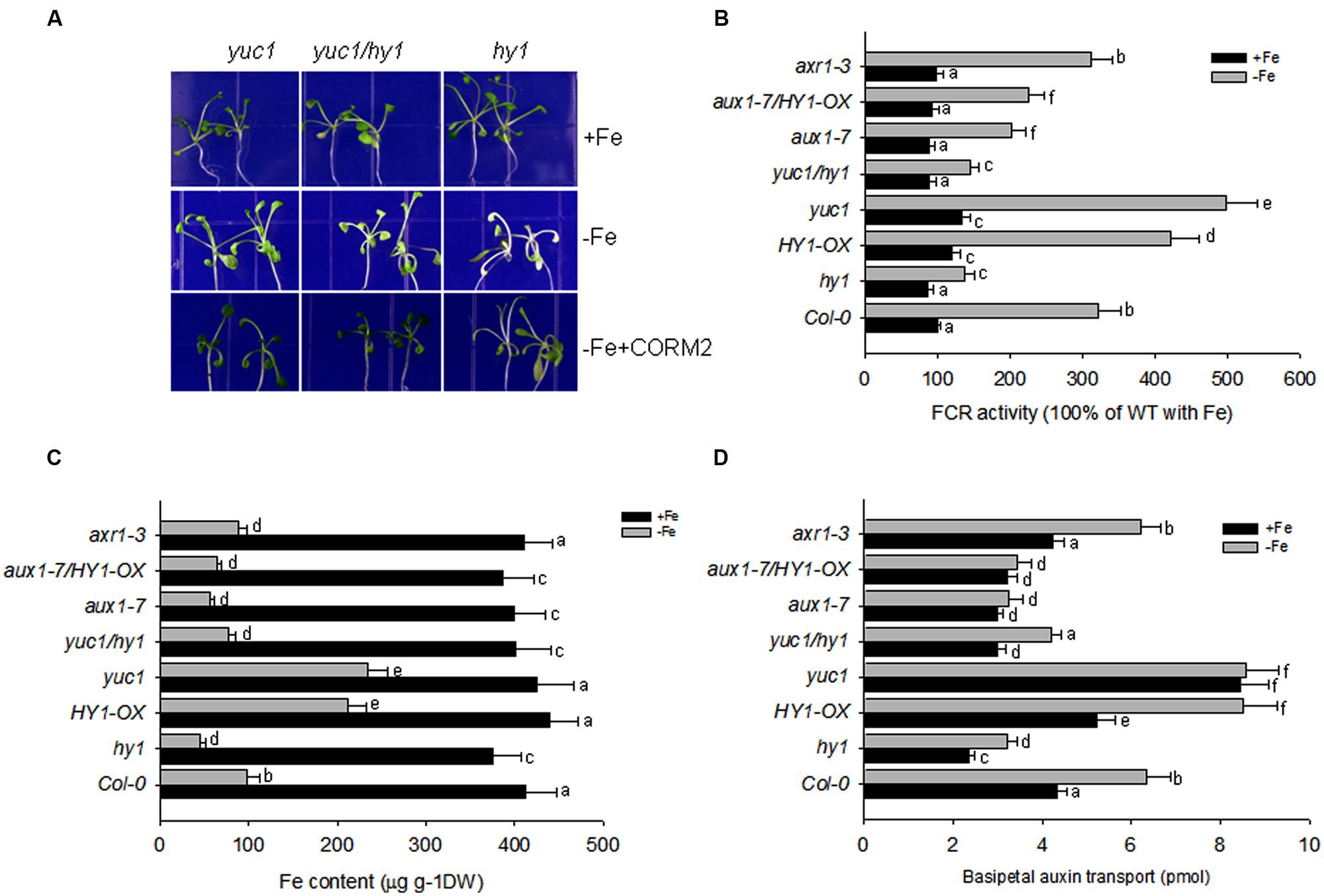
FIGURE 5. Auxin and CO modulate the response to –Fe in Arabidopsis. (A) The phenotypes of yuc1, yuc1/hy1, and hy1 seedlings grown on +Fe, –Fe, or –Fe+CORM2 media for 1 week. These lines were all first grown on +Fe media for 2 weeks. Lines kept on +Fe media were used as the positive control. (B–D) The effect of –Fe stress on FCR activity (B), Fe content (C), and basipetal auxin transport (D) in the wild-type Col-0, hy1, and HY1-OX lines. All lines were first grown on +Fe media for 2 weeks and then shifted to –Fe media (–Fe) or kept on +Fe media (+Fe) for 1 week before measurements were taken. Data are the means ± SD (n = 10), and bars with different letters are significantly different at p < 0.05 (Tukey’s test).
Because CO serves as a signal molecule to modulate the distributions of the polar auxin transporters, PIN1, PIN2, and AUX1, we also investigated the impact of CO treatment on basipetal auxin transport in relation to Fe depletion. Figure 5D shows the net amount of indole acetic acid (IAA) undergoing basipetal transport in a 1-cm apical segment after various treatments. With and without iron, the auxin over-producing mutant yuc1, and CO overproducing mutant HY1-OX had the greatest capacity for basipetal auxin transport. In contrast, under –Fe, hy1, aux1-7, aux1-7/HY1-OX lines had the lowest transport of auxin among all lines. Compared to yuc1, the high transport activity of auxin was impaired in yuc1/hy1, indicating that the presence of CO signaling is required for active transport of auxin.
To determine whether auxin contributes to the –Fe response through changes in auxin transport or auxin signal transduction, we examined the responses of the auxin transport mutant, aux1-7, and the auxin signaling mutant, axr1-3, under Fe depletion. We found that aux1-7 was more sensitive to –Fe, exhibiting lower FCR activity and basipetal auxin transport than axr1-3. axr1-3 did not differ from wild-type plants during –Fe for FCR activity and basipetal auxin transport (Figures 5B–D). Furthermore, HY1-OX/aux1-7 was still sensitive to Fe depletion, indicating over-expression of HY1 cannot compensate the defects of auxin transport to improve Fe acquisition in the aux1-7 mutant background (Figures 5B–D).
Double mutant analysis identified that HY1 is epistatic to YUC1 and AUX1-7 is epistatic to HY1-OX for FCR activity, Fe content, and basipetal auxin transport with or without Fe (Figures 5B–D). This suggests, under –Fe, in order to have CO signaling to cause downstream effects (increase in FCR activity, iron content, etc.) the auxin 1–7 transporter is required.
Pharmacological experiments also revealed the importance of auxin and CO in the response to –Fe in Arabidopsis. As shown in Supplementary Figures S6–S8, as compared to untreated plants, the permeable auxin analog NAA significantly improved FCR activity, Fe content, and basipetal auxin transport under –Fe. The addition of CORM2, during –Fe, also increased FCR activity, iron content, and basipetal auxin transport in the wild-type plants but this effect required auxin transporters. The auxin transport inhibitor NPA alone markedly suppressed FCR activity, basipetal auxin transport, and reduced Fe content under –Fe. ZnPPIX treatment, a HY1 inhibitor, also reduced FCR activity, iron content, and basipetal auxin transport as compared to untreated plants during Fe depletion.
Under Fe Deficiency, Auxin Effects on NO generation are HY1 Dependent and Auxin Transporters are needed for NO Generation
Arabidopsis noa1 and nia1/nia2 (termed nr) mutants are unable to accumulate sufficient amounts of NO (Guo et al., 2003; Wang et al., 2010), whereas cue1 produces excess amounts of NO (He et al., 2004). NO was reported to regulate the plant’s response to –Fe (Chen et al., 2010). To unravel the role of cross-talk between CO and NO signaling under –Fe stress in Arabidopsis, we examined NO level of the lines subjected to various treatments using the NO dye, DAF-FMDA. With iron, the yuc1, cue1, HY1-OX, and yuc1/hy1, hy1/cue1 lines had higher levels of NO as compared to wild-type (Figures 6A,B). The NO levels in yuc1/hy1 was weaker than in yuc1, suggesting that the effects of auxin on the generation of NO are HY1 dependent. hy1/cue1 and cue1 both had higher NO levels than wild-type plants (Figures 6A,B). –Fe induced an increase in NO generation, among which there was greatest generation in the yuc1 (auxin over-accumulating) and cue1 (NO over-accumulating) lines. By contrast, NO generation was very low in hy1 or yuc1/hy1 under –Fe conditions. In the yuc1/noa1 and yuc1/nr mutants, the NO generation induced by –Fe was lower than in yuc1.
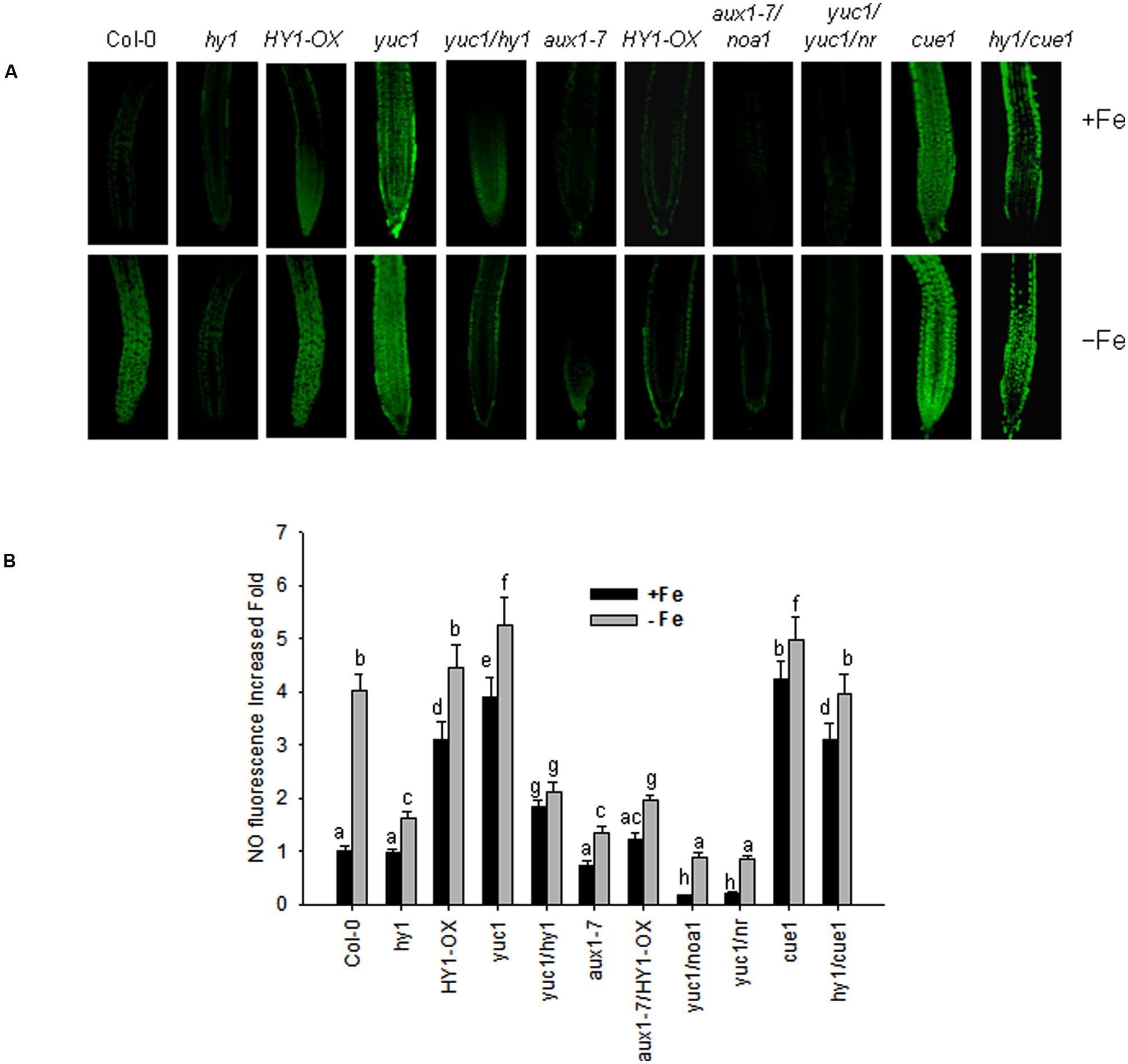
FIGURE 6. Iron deficiency stress induced NO generation. Wild-type Col-0, hy1, HY1-OX, yuc1, yuc1/hy1, aux1-7, aux1-7/HY1-OX, yuc1/noa1, yuc1/nr, cue1, and hy1/cue1 lines were grown on +Fe media and then shifted or not to –Fe media, for 1 day. Green fluorescence indicates NO (A). The corresponding relative fluorescence intensities (B) were recorded. Data are the means ± SD (n = 15), and bars with different letters are significantly different at p < 0.05 (Tukey’s test). BAR = 0.1 cm.
Accordingly, FCR activity and Fe content were higher in HY1-OX and cue1, and lower in hy1, noa1, and nr as compared to wild-type plants grown without iron. Furthermore, under –Fe, hy1/cue1 had higher FCR activity and Fe content than hy1. However under –Fe, HY1-OX/nr had lower FCR activity, Fe content, and basipetal auxin transport than HY1-OX (Figures 7B–D). Under –Fe, basipetal auxin transport was lower in hy1 and hy1/cue1 lines and higher in HY1-OX as compared to wild-type (Figure 7C). Under –Fe, basipetal auxin transport was significantly less in noa1, nr, hy1, and hy1/cue1 as compared to wild-type (Figure 7C).
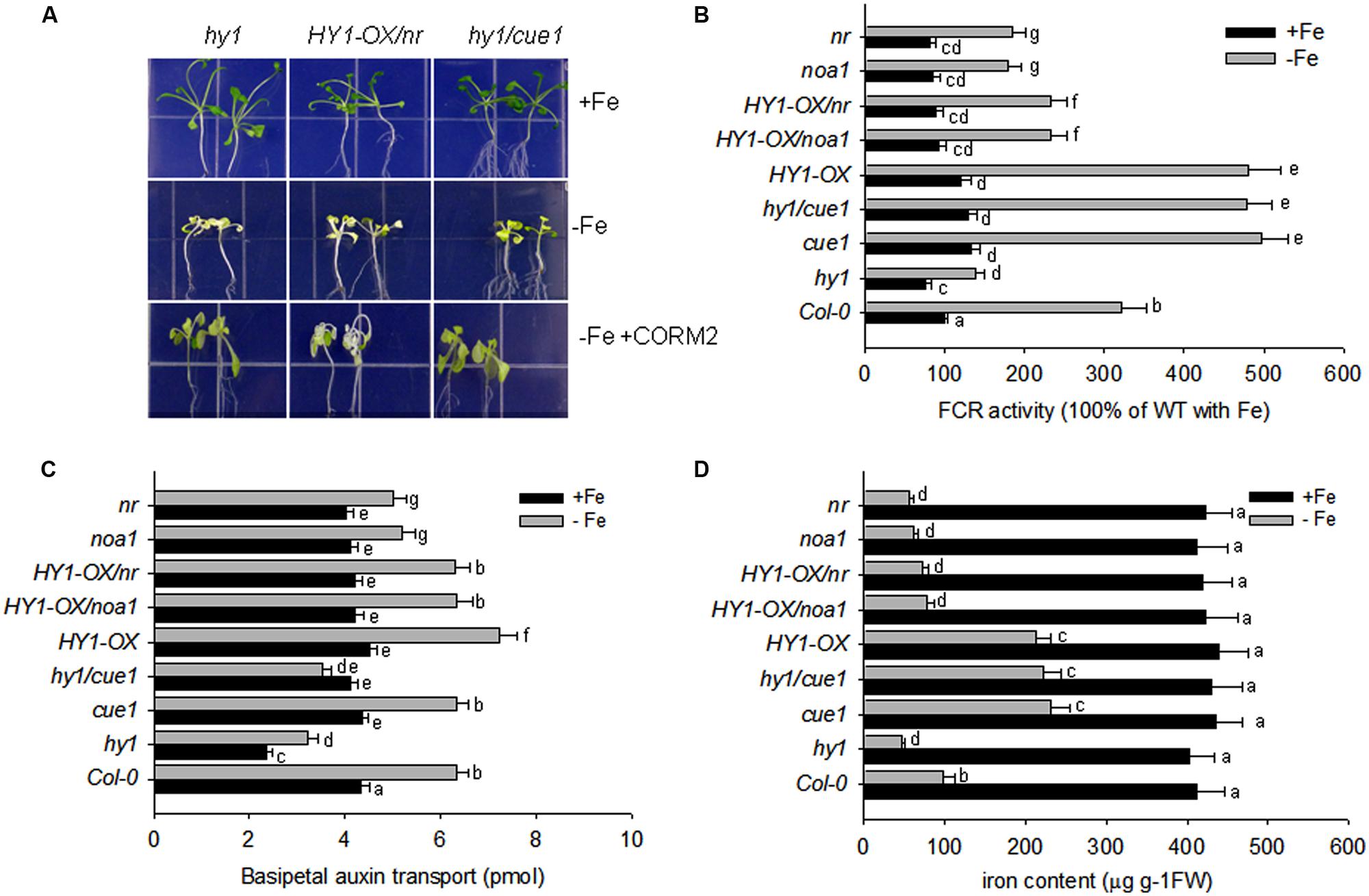
FIGURE 7. Auxin and CO modulate the response to –Fe stress in Arabidopsis. (A) The phenotypes of hy1, HY1-OX/nr, and hy1/cue1 mutants grown on +Fe, –Fe, or –Fe+CORM2 media for 1 week. All lines were first grown on +Fe media for 2 weeks and then subjected to the specified treatment for 1 week. Lines kept on +Fe media were used as the positive control. (B–D) FCR activity, Fe content, and root basipetal auxin transport in the wild-type Col-0, hy1, and HY1-OX lines upon exposure to –Fe stress. The wild-type Col-0, hy1, and HY1-OX lines were grown on +Fe media for 2 weeks and then shifted to –Fe media (–Fe), or +Fe media (+Fe) for 1 week before measurements were taken. The FCR activity (B), Fe content (C), and basipetal auxin transport (D) were measured after 1 week of –Fe stress. Data are the means ± SD (n = 12), and bars with different letters are significantly different at p < 0.05 (Tukey’s test).
Our other experiments also confirmed a role for NO signaling in the response to –Fe in Arabidopsis. The treatment with the NO scavenger, cPTIO, greatly suppressed FCR activity induced by Fe depletion, as well as NO generation (Supplementary Figures S6 and S9). Treatments with the NR inhibitor tungstate, or the NO synthase enzyme inhibitor L-NAME, also arrested the increase in FCR activity under –Fe conditions (Supplementary Figure S6). By contrast, the treatment with the NO donor (SNAP), increased FCR activity and Fe content under –Fe conditions as compared to untreated plants (Supplementary Figures S6 and S8), However, treatment with the HY1 enzyme inhibitor ZnPPIX, or the auxin transport inhibitor, NPA, reduced NO production, FCR activity, and Fe content as compared to untreated plants (Supplementary Figures S6, S8, and S9). Moreover under –Fe conditions, for the cue1 mutant, in which NO is constitutively produced, FCR activity and Fe content is increased as compared to wild-type plants (Figures 7B,D). Also, the treatments with SNAP, the NO donor, did not have significant effect on the basipetal auxin transport under –Fe status (Supplementary Figure S7).
Auxin, CO, and NO Cooperatively Modulate the –Fe Response
The bHLH transcriptional factor FIT1 up-regulates the expression of ferric reduction oxidase 2 (FRO2) in Fe-deficient Arabidopsis. We found that Fe depletion caused elevated transcription of FIT1 and FRO2 in wild-type plants, and this effect was greater in the yuc1 line, but less in hy1, yuc1/hy1, noa1, nr, HY1-OX/noa1, and HY1-OX/nr (Figure 8). Consistent with the observations, as compared to untreated plants, the treatment with either CORM2 (CO donor) or NAA (auxin) enhanced the expression of FIT1 and FRO2, whereas NPA (auxin inhibitor), ZnPPIX (HY1 inhibitor), cPTIO (NO scavenger), L-NAME (NO synthase inhibitor), and tungstate (NR inhibitor) treatments suppressed the transcriptions of FIT1 and FRO2 in wild-type plants subjected to –Fe depletion (Supplementary Figure S10).
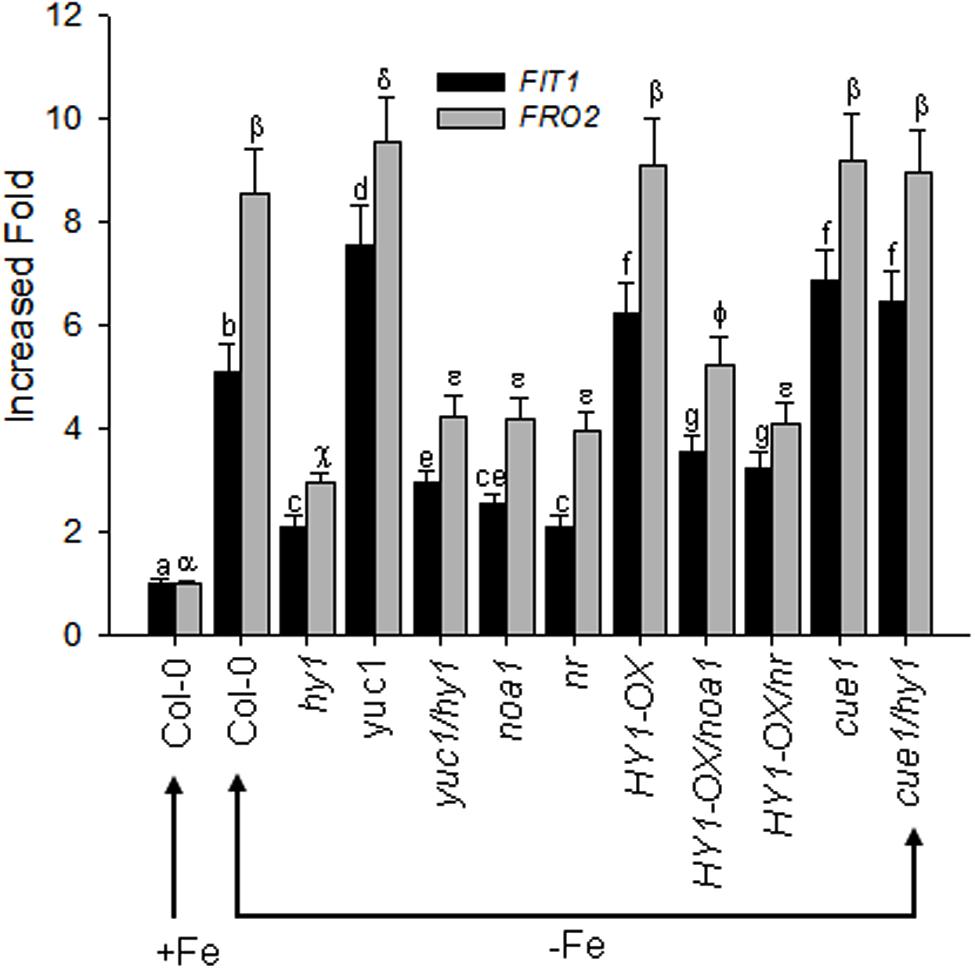
FIGURE 8. Differential FIT1 and FRO2 expression in CO–, NO–, and auxin-related mutants in response to –Fe stress. Two-week-old wild-type Arabidopsis Col-0 seedlings, CO-related lines (hy1 and HY1-OX), auxin-related mutant (yuc1) and NO-related mutants (cue1, noa1, nr), and double mutants (yuc1/hy1, HY1-OX/nr, HY1-OX/noa1, cue1/hy1) were transferred to –Fe media. The FIT1 and FRO2 gene expression in the roots were analyzed by RT-PCR after 24 h of treatment. Tubulin gene expression was used as a control. Data are the means ± SD (n = 9), and bars with different letters are significantly different at p < 0.05 (Tukey’s test).
Discussion
CO Modulates the –Fe Response in Arabidopsis
Numerous studies suggest that CO is a gas messenger that regulates the plant’s response to multiple abiotic stresses (Han et al., 2008; Xuan et al., 2008; Bai et al., 2012; Li et al., 2013). In the present study, we found that CO enhances the tolerance to –Fe in Arabidopsis. Heme oxygenase encoded by HY1 is mainly responsible for CO generation in response to various environmental stimuli in Arabidopsis (Li et al., 2013). Our experiments showed that inhibiting heme oxygenase activity by using specific inhibitor ZnPPIX reduced the activity of FCR and decreased Fe content as compared to untreated plants (Supplementary Figures S6 and S8), suggesting that CO generation modulates the response to –Fe in Arabidopsis. –Fe induces heme oxygenase activity and CO generation in wild-type, but not in hy1 null mutant (Figure 1A). This suggests that HY1 is needed for increased heme oxygenase activity and CO accumulation for plants. By contrast, HY1-OX transgenic line showed higher heme oxygenase activity and CO accumulation than wild-type plants (Figure 1A). The hy1 null mutant was more sensitive to –Fe than the wild-type, exhibiting lower levels of chlorophyll content, FCR activity, and endogenous Fe content. In contrast, the transgenic line over-expressing HY1 was more tolerant to –Fe than wild-type plants (Figure 2). The treatment with artificial CO donor, CORM2, increased chlorophyll content, FCR activity, and Fe content in wild-type and hy1 lines subjected to –Fe, and treatment with CORM2 up-regulated FIT1/FRO2 expression in wild-type plants (Figure 2; Supplementary Figure S10). In agreement with previous research (Li et al., 2013), our results indicate that CO improves the ability of Arabidopsis to cope with –Fe. In Arabidopsis, heme oxygenase encoded by HY1 degrades porphyrin into biliverdin, CO and ionic Fe (Schmitt, 1997). Therefore, induced heme oxygenase activity improves the supply of ionic Fe during short term –Fe. However, this pathway is not sustainable due to the limited amounts of porphyrin in Arabidopsis. In fact, elevated CO level through HY1 activity initiates a downstream cascade of events that enhance Fe uptake by increasing the expression of FIT1 (Figure 8). Our finding that the addition of the CO donor CORM2 increases chlorophyll, FCR activity, and Fe content in the hy1 mutant under Fe depletion (Figure 2) also supports this observation.
CO Promotes Auxin Polar Transport that Improves the Tolerance to –Fe in Arabidopsis
Auxin regulates the accumulation of NO that enhances Fe uptake in Arabidopsis (Chen et al., 2010). Furthermore, CO and NO interact in Arabidopsis to modulate the response to –Fe (Li et al., 2013). We thus reasoned that cross-talk exists between CO and auxin under –Fe. Indeed, we found that auxin induced HY1 transcription and promoted CO generation (Figures 3A,B; Supplementary Figure S3) as did –Fe (Figure 1). Interestingly, immunoblot analysis revealed two bands of HY1-6HA after auxin or CO biosynthesis inhibitor ZnPPIX treatment in HY1-OX seedlings (Figure 3C), which suggests that HY1 undergoes post-transcriptional modification such as protein phosphorylation when subjected to auxin treatment. FIT1 and IRT1, which are involved in the –Fe response, were previously shown to undergo post-transcriptional modification (Barberon et al., 2011; Meiser et al., 2011). Furthermore, the transgenic lines with altered CO accumulation (i.e., hy1 or HY1-OX lines) exhibited changes in auxin accumulation in the root tip under Fe depletion, as shown by an increase of DR5:GFP fluorescence intensity in HY1-OX lines and a decrease in auxin in hy1 as compared with wild-type and addition of exogenous CO to hy1 also enhanced auxin-dependent fluorescence (Figure 4). These observations suggest that CO increases auxin accumulation in the root tip. PIN1 and PIN2 are both important shoot-to-root auxin transporters (Blilou et al., 2005). A previous study showed that localized treatment with Fe ions altered the distribution of auxin in Arabidopsis seedlings (Giehl et al., 2012). We found that more PIN2 accumulated in the HY1-OX lines which produced a higher level of CO than wild-type, and addition of exogenous CO increased PIN2 accumulation in the hy1 mutant impaired in CO production under –Fe (Figures 4B–D). This finding correlates with the pattern of auxin distribution in the root tip under –Fe (Figure 4A), and suggests that CO promotes auxin redistribution under –Fe. In agreement with the conclusion that CO affects auxin distribution in the root tips by increasing the accumulation of PIN2, we also found that –Fe stress caused an increased number of lateral roots and root hairs in wild-type plants. In contast, the hy1 line impaired in CO generation did not (Supplementary Figure S5). HY1-OX, which accumulates a high level of of CO, also exhibited increased lateral root and root hair densities as compared to wild-type plants grown with or without Fe (Supplementary Figure S5). Given that plants adjust their lateral root architecture and root hair density to adapt to –Fe stress (Ling et al., 2002; Graziano and Lamattina, 2007), and that CO is an essential signal in root development (Guo et al., 2008), we propose that CO regulates lateral root architecture and root hair density under –Fe stress.
We also evaluated basipetal auxin transport in wild-type, hy1, and HY1-OX lines, as well as in the auxin over-accumulating line yuc1 and the auxin transport defective mutant aux1-7 (Figure 5D). Basipetal auxin transport was increased in HY1-OX and yuc1 lines and decreased in hy1 plants as compared to wild-type plants (Figure 5D). As compared to untreated plants, addition of exogenous CO also enhanced basipetal auxin transport (Supplementary Figure S7), suggesting that CO generation promotes basipetal auxin transport by affecting the accumulation of PIN1 and PIN2 (Figure 4). NPA treatment that impairs auxin transport also caused a dramatic reduction in Fe content and down-regulation of FIT1 and FRO2 in Arabidopsis subjected to –Fe stress (Supplementary Figures S8 and S10). This suggests that auxin transport is critical in the plants response to –Fe. Suppressing CO generation using the HY1 inhibitor ZnPPIX impaired basipetal auxin transport (Supplementary Figure S7) and reduced Fe content under –Fe stress (Supplementary Figure S8). Conversely, treatment with the CO donor CORM2 increased basipetal auxin transport and Fe uptake as compared to untreated plants. These results confirm that CO promotes basipetal auxin transport that favors the adaptation of Arabidopsis under –Fe stress.
CO Interacts with Auxin and NO Signals to Regulate the Fe Deficiency Response in Arabidopsis
Nitric oxide, another gas messenger, plays multiple regulatory roles in processes such as systemic acquired resistance and the hypersensitive response (Guo et al., 2003; He et al., 2004; Wendehenne et al., 2004; Scheler et al., 2013). Recently, NO has been shown to increase FCR activity in roots and enhances the tolerance to –Fe stress in various plants (Graziano and Lamattina, 2007; Chen et al., 2010). Furthermore, NO acts downstream of auxin signaling (Chen et al., 2010). Our results confirm that the NO signaling is involved in the tolerance of Arabidopsis to –Fe stress. noa1 and nr, which are unable to generate NO, show lower levels of Fe content, FCR activity, and basipetal auxin transport under –Fe stress as compared to wild-type plants, while cue1, which accumulates high levels of endogenous NO, is tolerant to –Fe stress (Figure 7). Similar to the previous findings (Chen et al., 2010), we found that suppressing NO generation using cPTIO, L-NAME, and tungstate caused down-regulation of FIT1 and FRO2 (Supplementary Figure S10) and a marked reduction of Fe content (Supplementary Figure S8). A previous study suggests that the redistribution of auxin triggers NO synthesis and release that initiates the expression of –Fe response genes to enhance Fe uptake (Chen et al., 2010). In agreement with this hypothesis, we found that suppressing auxin transport through NPA treatment or loss of AUX (i.e., aux1-7) decreased NO release under –Fe stress (Figure 6, Supplementary Figure S9), while the auxin over-accumulation mutant yuc1 produced high levels of NO (Figure 6). These findings demonstrate the interplay between auxin and NO under –Fe stress again. In the present study, we also unraveled that by manipulating the CO signal, including treatment with ZnPPIX, a specific inhibitor of HY1, knockout of HY1 (i.e., hy1), treatment with the CO donor, CORM2, and overexpressing HY1 (i.e., HY1-OX), polar auxin transport was affected (Figure 5; Supplementary Figure S7), as evidenced by corresponding PIN1/2 protein accumulation pattern, DR5:GFP fluorescence intensity (Figure 4), lateral root number and the root hair density (Supplementary Figure S5). Ultimately, the NO levels were altered in a corresponding manner (Figure 6). Changes in NO levels affected expression of FIT1/FRO2 which directly determines the capacity of Fe acquisition (Figure 8). These results suggest that CO effects auxin and NO signals in response to –Fe stress in Arabidopsis.
In agreement with our findings, recent studies have suggested that both CO and NO are involved in the plant response to various environmental stresses, including –Fe (Hartsfield, 2002; Guo et al., 2008; Bai et al., 2012; Li et al., 2013). However, the integrative signaling pathway for CO and NO remains largely unknown. Xie et al. (2011) reported that the NOA1/NR-dependent NO signal and HY1-dependent CO signal function in both compensatory and synergistic modes to modulate plant tolerance to salt stress (Xie et al., 2008, 2011). Like noa1 and nr, we found that hy1 showed increased sensitivity to –Fe stress. However, increasing NO content rescued the sensitivity of hy1 to –Fe (hy1cue1 mutant), in agreement with a previous report that NO directly triggers FCR activity and improves Fe uptake (Graziano and Lamattina, 2007; Chen et al., 2010). Furthermore, increasing CO generation did not increase FCR activity or Fe acquisition in noa1 or nr mutants impaired in NO production in our study or a previous study (Bai et al., 2012), suggesting that NO signaling is downstream to CO signaling for FCR activity and Fe content. A recent study has showed that a combination of –Fe and cadmium stress induced NO generation (Han et al., 2008). However, in contrast to our findings, this study found that NO accumulated in hy1 roots exposed to –Fe stress. Another study found that transgenic HY1-OX lines generate more NO than the wild-type after –Fe treatment, while a knock-down of HY1 expression reduces NO accumulation (Li et al., 2013), which is in agreement with our findings. The discrepancies of NO generation in response to –Fe treatment between these studies may be due to differences in experimental conditions. In the first study, seedlings were grown on solid vertical medium for 5 days and then transferred by hand to +Fe medium for a 3-day treatment. However, young seedlings can easily be wounded when moved by hand, and this may have caused NO to accumulate. As suggested in the second study (Li et al., 2013), we used a liquid-culture system. This system is widely used in Fe stress studies, as it does not damage young seedlings.
In summary, our findings demonstrate that –Fe induces heme oxygenase enzyme-dependent CO generation, which promotes basipetal polar auxin transport in root tip by altering the asymmetric accumulation of PIN1 and PIN2. The elevated level of auxin in the root tip promotes Fe acquisition by up-regulating the expression of Fe-uptake genes FIT1 and FRO2. Our study unravels the interactions among CO, auxin, and NO signals to cope with –Fe in Arabidopsis.
Author Contributions
LY, YG, and JJ performed the experiments, data analysis, and drafted the manuscript. HW, KH-S, EA, and YL assisted in manuscript preparation and revision. XH and YG conceived the project and finalized the manuscript.
Conflict of Interest Statement
The authors declare that the research was conducted in the absence of any commercial or financial relationships that could be construed as a potential conflict of interest.
Acknowledgments
This work was supported by National High-tech R&D Program of China (2013AA102705), National Science Foundation grant of China (30900871, 30971452, 31170256, 31400169), Natural Science Foundation of Jiangsu Province (BK2011409, BK20140454), Jiangsu Collaborative Innovation Center of Regional Modern Agriculture and Environment Protection (HSXT305), Major Science and Technology Program (110201101003-TS-03, TS-02-20110014, 2011YN02, and 2011YN03), Jiangsu Government Scholarship for Overseas Studies and Qinglan Project of Jiangsu Province. The authors would like to extend their sincere appreciation to the Deanship of Scientific Research at King Saud University for its funding this Research group NO (RG- 1435-014). Mention of trade names or commercial products in this publication is solely for the purpose of providing specific information and does not imply recommendation or endorsement by the USDA. The USDA is an equal opportunity provider and employer.
Supplementary Material
The Supplementary Material for this article can be found online at: http://journal.frontiersin.org/article/10.3389/fpls.2016.00112
FIGURE S1 | The HY1-HA expression level in different individual transgenic lines that overexpress HY1-HA. The proteins from different individual lines that over-express HY1-HA were extracted for determining the HY1-HA protein content by immunoblot analysis using anti-HA antibodies. The total protein was stained with Coomassie blue (ribulose-1,5-bisphosphate carboxylase/oxygenase [Rubisco]) to confirm equal loading.
FIGURE S2 | Time-course analyses of HY1 transcripts under –Fe or +Fe. Two-week-old wild-type Col-0 lines under –Fe or +Fe medium were used to measure HY1 transcripts at the indicated times. Data are the means ± SD (n = 3).
FIGURE S3 | Auxin induced heme oxygenase activity and CO generation. Two-week-old wild-type Col-0 were treated with 100 nM NAA for indicated time, and the heme oxygenase activity and CO generation were measured. Data are the means ± SD (n = 3).
FIGURE S4 | Expression patterns of AUX1-YFP in the root tip of wild-type Col-0, hy1, HY1-OX lines, and hy1 line treated with CORM2. The seedlings were treated with 100 nM CORM2 after 12 h of –Fe stress, and YFP fluorescence were recorded.
FIGURE S5 |Carbon monoxide (CO) modulates root hair and lateral root development. (A,B) Root hair phenotype of Col-0, hy1, and HY1-OX lines grown in +Fe, –Fe, and –Fe+CORM2 media for 1 week. (C) Col-0, hy1, and HY1-OX lines grown on +Fe, –Fe, or –Fe+CORM2 media for 1 week.
FIGURE S6 | The effects of different inhibitors on FCR activity in the wild-type Col-0 lines with or without –Fe treatment. Two-week-old wild-type-lines were treated with 100 nM NAA, 10 μM NPA, 200 μM ZnPPIX, 300 μM cPTIO, 1 mM L-NAME, 10 mM tungstate, 100 nM CORM2, 100 nM CORM2+10 μM NPA, 30 μM SNAP, 30 μM GSNO, and 30 μM SNAP+10 μM NPA, respectively, for 1 week, and the FCR activity was measured. Data are the means ± SD (n = 12), and bars with different letters are significantly different at p < 0.05 (Tukey’s test).
FIGURE S7 |The effects of different inhibitors on auxin transport in the roots of wild-type Col-0 lines with or without –Fe treatment. Two-week-old wild-type Col-0 lines were treated with 100 nM NAA, 10 μM NPA, 200 μM ZnPPIX, 300 μM cPTIO, 1 mM L-NAME, 10 mM tungstate, 100 nM CORM2, 100 nM CORM2+10 μM NPA, 30 μM SNAP, 30 μM GSNO, and 30 μM SNAP+10 μM NPA, respectively, for 1 week, and basipetal auxin transport was measured. Data are the means ± SD (n = 9), and bars with different letters are significantly different at p < 0.05 (Tukey’s test).
FIGURE S8 | The effects of different inhibitors on Fe content in roots of wild-type Col-0 lines with or without –Fe treatment. Two-week-old wild-type Col-0 lines were treated with 100 nM NAA, 10 μM NPA, 200 μM ZnPPIX, 300 μM cPTIO, 1 mM L-NAME, 10 mM tungstate, 100 nM CORM2, 100 nM CORM2+10 μM NPA, 30 μM SNAP, 30 μM GSNO, and 30 μM SNAP+10 μM NPA, respectively, for 1 week, and the Fe content was measured. Data are the means ± SD (n = 9), and bars with different letters are significantly different at p < 0.05 (Tukey’s test).
FIGURE S9 | The effect of various inhibitors on NO generation after –Fe stress. Two-week-old wild-type Col-0 lines were treated with 100 nM NAA, 10 mM NPA, 300 μM cPTIO, 1 mM L-NAME, 10 mM tungstate, 200 μM ZnPPIX, 100 nM CORM2, 100 nM CORM2+10 μM NPA, and 30 μM SNAP, respectively, for 1 day, and the green fluorescence (A) and the corresponding relative fluorescence intensity (B) in the roots were recorded. Data are the means ± SD (n = 9), and bars with different letters are significantly different at p < 0.05 (Tukey’s test).
FIGURE S10 | The effects of various inhibitors on FIT1 and FRO2 expression in the roots of wild-type Col-0 after –Fe stress. Two-week-old Col-0 seedlings were treated with the indicated inhibitors for 1 day, and FIT1 (left) and FRO2 (right) expression in the root were measured by qRT-PCR analysis. Data are the means ± SD (n = 3), and bars with different letters are significantly different at p < 0.05 (Tukey’s test).
Abbreviations
CORM2, tricarbonyldichlororuthenium (II) dimer; cPTIO, 2-4-carboxyphenyl-4, 4, 5, 5- tetra-methylimidazoline- 1- oxyl- 3- oxide; DAF-FMDA, 4-amino-5-methylamino-2,7-di-fluorofluorescein diacetate; –Fe, iron deficiency; GSNO, S-Nitrosoglutathione; L-NAME, NG-Nitro-L-arginine Methyl Ester; NAA, a-naphthaleneacetic acid; NPA, N-1-naphthylphtha- lamic acid; SNAP, S- nitroso- N- acetylpenicillamine; ZnPPIX, zinc protoporphyrin IX.
References
Bai, X. G., Chen, J. H., Kong, X. X., Todd, C. D., Yang, Y. P., Hu, X. Y., et al. (2012). Carbon monoxide enhances the chilling tolerance of recalcitrant Baccaurea ramiflora seeds via nitric oxide-mediated glutathione homeostasis. Free Radic. Biol. Med. 53, 710–720. doi: 10.1016/j.freeradbiomed.2012.05.042
Barberon, M., Zelazny, E., Robert, S., Conejero, G., Curie, C., Friml, J., et al. (2011). Monoubiquitin-dependent endocytosis of the iron-regulated transporter 1 (IRT1) transporter controls iron uptake in plants. Proc. Natl. Acad. Sci. U.S.A. 108, E450–E458. doi: 10.1073/pnas.1100659108
Besson-Bard, A., Pugin, A., and Wendehenne, D. (2008). New insights into nitric oxide signaling in plants. Annu. Rev. Plant Biol. 59, 21–39. doi: 10.1146/annurev.arplant.59.032607.092830
Blilou, I., Xu, J., Wildwater, M., Willemsen, V., Paponov, I., Friml, J., et al. (2005). The PIN auxin efflux facilitator network controls growth and patterning in Arabidopsis roots. Nature 433, 39–44. doi: 10.1038/nature03184
Briat, J. F., Fobis-Loisy, I., Grignon, N., Lobreaux, S., Pascal, N., Savino, G., et al. (1995). Cellular and molecular aspects of iron metabolism in plants. Biol. Cell 84, 69–81. doi: 10.1016/0248-4900(96)81320-7
Chen, W. W., Yang, J. L., Qin, C., Jin, C. W., Mo, J. H., Ye, T., et al. (2010). Nitric oxide acts downstream of auxin to trigger root ferric-chelate reductase activity in response to iron deficiency in Arabidopsis. Plant Physiol. 154, 810–819. doi: 10.1104/pp.110.161109
Clough, S. J., and Bent, A. F. (1998). Floral dip: a simplified method for Agrobacterium-mediated transformation of Arabidopsis thaliana. Plant J. 16, 735–743. doi: 10.1046/j.1365-313x.1998.00343.x
Curie, C., and Briat, J. F. (2003). Iron transport and signaling in plants. Annu. Rev. Plant Biol. 54, 183–206. doi: 10.1146/annurev.arplant.54.031902.135018
Davis, S. J., Kurepa, J., and Vierstra, R. D. (1999). The Arabidopsis thaliana HY1 locus, required for phytochrome-chromophore biosynthesis, encodes a protein related to heme oxygenases. Proc. Natl. Acad. Sci. U.S.A. 96, 6541–6546. doi: 10.1073/pnas.96.11.6541
Giehl, R. F., Lima, J. E., and Von Wiren, N. (2012). Localized iron supply triggers lateral root elongation in Arabidopsis by altering the AUX1-mediated auxin distribution. Plant Cell 24, 33–49. doi: 10.1105/tpc.111.092973
Graziano, M., and Lamattina, L. (2007). Nitric oxide accumulation is required for molecular and physiological responses to iron deficiency in tomato roots. Plant J. 52, 949–960. doi: 10.1111/j.1365-313X.2007.03283.x
Green, L. S., and Rogers, E. E. (2004). FRD3 controls iron localization in Arabidopsis. Plant Physiol. 136, 2523–2531. doi: 10.1104/pp.104.045633
Guo, F. Q., and Crawford, N. M. (2005). Arabidopsis nitric oxide synthase1 is targeted to mitochondria and protects against oxidative damage and dark-induced senescence. Plant Cell 17, 3436–3450. doi: 10.1105/tpc.105.037770
Guo, F. Q., Okamoto, M., and Crawford, N. M. (2003). Identification of a plant nitric oxide synthase gene involved in hormonal signaling. Science 302, 100–103. doi: 10.1126/science.1086770
Guo, K., Xia, K., and Yang, Z. M. (2008). Regulation of tomato lateral root development by carbon monoxide and involvement in auxin and nitric oxide. J. Exp. Bot. 59, 3443–3452. doi: 10.1093/jxb/ern194
Han, Y., Zhang, J., Chen, X., Gao, Z., Xuan, W., Xu, S., et al. (2008). Carbon monoxide alleviates cadmium-induced oxidative damage by modulating glutathione metabolism in the roots of Medicago sativa. New Phytol. 177, 155–166.
Hartsfield, C. L. (2002). Cross talk between carbon monoxide and nitric oxide. Antioxid. Redox. Signal. 4, 301–307. doi: 10.1089/152308602753666352
He, Y., Tang, R. H., Hao, Y., Stevens, R. D., Cook, C. W., Ahn, S. M., et al. (2004). Nitric oxide represses the Arabidopsis floral transition. Science 305, 1968–1971. doi: 10.1126/science.1098837
Imsande, J. (1998). Iron, sulfur, and chlorophyll deficiencies: a need for an integrative approach in plant physiology. Physiol. Plant. 103, 139–144. doi: 10.1034/j.1399-3054.1998.1030117.x
Le Jean, M., Schikora, A., Mari, S., Briat, J. F., and Curie, C. (2005). A loss-of-function mutation in AtYSL1 reveals its role in iron and nicotianamine seed loading. Plant J. 44, 769–782. doi: 10.1111/j.1365-313X.2005.02569.x
Li, H., Song, J. B., Zhao, W. T., and Yang, Z. M. (2013). AtHO1 is involved in iron homeostasis in an NO-dependent manner. Plant Cell Physiol. 54, 1105–1117. doi: 10.1093/pcp/pct063
Lincoln, C., Britton, J. H., and Estelle, M. (1990). Growth and development of the axr1 mutants of Arabidopsis. Plant Cell 2, 1071–1080. doi: 10.2307/3869260
Ling, H. Q., Bauer, P., Bereczky, Z., Keller, B., and Ganal, M. (2002). The tomato fer gene encoding a bHLH protein controls iron-uptake responses in roots. Proc. Natl. Acad. Sci. U.S.A. 99, 13938–13943. doi: 10.1073/pnas.212448699
Lingam, S., Mohrbacher, J., Brumbarova, T., Potuschak, T., Fink-Straube, C., Blondet, E., et al. (2011). Interaction between the bHLH transcription factor FIT and ETHYLENE INSENSITIVE3/ETHYLENE INSENSITIVE3-LIKE1 reveals molecular linkage between the regulation of iron acquisition and ethylene signaling in Arabidopsis. Plant Cell 23, 1815–1829. doi: 10.1105/tpc.111.084715
Long, T. A., Tsukagoshi, H., Busch, W., Lahner, B., Salt, D. E., and Benfey, P. N. (2010). The bHLH transcription factor POPEYE regulates response to iron deficiency in Arabidopsis roots. Plant Cell 22, 2219–2236. doi: 10.1105/tpc.110.074096
Meiser, J., Lingam, S., and Bauer, P. (2011). Posttranslational regulation of the iron deficiency basic helix-loop-helix transcription factor FIT is affected by iron and nitric oxide. Plant Physiol. 157, 2154–2166. doi: 10.1104/pp.111.183285
Müller, B., and Sheen, J. (2008). Cytokinin and auxin interaction in root stem-cell specification during early embryogenesis. Nature 453, 1094–1097. doi: 10.1038/nature06943
Ottenschläger, I., Wolff, P., Wolverton, C., Bhalerao, R. P., Sandberg, G., Ishikawa, H., et al. (2003). Gravity-regulated differential auxin transport from columella to lateral root cap cells. Proc. Natl. Acad. Sci. U.S.A. 100, 2987–2991. doi: 10.1073/pnas.0437936100
Otterbein, L. E., Bach, F. H., Alam, J., Soares, M., Lu, H., Wysk, M., et al. (2000). Carbon monoxide has anti-inflammatory effects involving the mitogen-activated protein kinase pathway. Nat. Med. 6, 422–428. doi: 10.1038/74680
Pickett, F. B., Wilson, A. K., and Estelle, M. (1990). The aux1 mutation of Arabidopsis confers both auxin and ethylene resistance. Plant Physiol. 94, 1462–1466. doi: 10.1104/pp.94.3.1462
Scheler, C., Durner, J., and Astier, J. (2013). Nitric oxide and reactive oxygen species in plant biotic interactions. Curr. Opin. Plant Biol. 16, 534–539. doi: 10.1016/j.pbi.2013.06.020
Schmitt, M. P. (1997). Utilization of host iron sources by Corynebacterium diphtheriae: identification of a gene whose product is homologous to eukaryotic heme oxygenases and is required for acquisition of iron from heme and hemoglobin. J. Bacteriol. 179, 838–845.
Scholl, R. L., May, S. T., and Ware, D. H. (2000). Seed and molecular resources for Arabidopsis. Plant Physiol. 124, 1477–1480. doi: 10.1104/pp.124.4.1477
Sivitz, A. B., Hermand, V., Curie, C., and Vert, G. (2012). Arabidopsis bHLH100 and bHLH101 control iron homeostasis via a FIT-independent pathway. PLoS ONE 7:e44843. doi: 10.1371/journal.pone.0044843
Ulmasov, T., Murfett, J., Hagen, G., and Guilfoyle, T. J. (1997). Aux/IAA proteins repress expression of reporter genes containing natural and highly active synthetic auxin response elements. Plant Cell 9, 1963–1971. doi: 10.1105/tpc.9.11.1963
Vert, G., Grotz, N., Dedaldechamp, F., Gaymard, F., Guerinot, M. L., Briat, J. F., et al. (2002). IRT1, an Arabidopsis transporter essential for iron uptake from the soil and for plant growth. Plant Cell 14, 1223–1233. doi: 10.1105/tpc.001388
Wang, H. Y., Klatte, M., Jakoby, M., Baumlein, H., Weisshaar, B., and Bauer, P. (2007). Iron deficiency-mediated stress regulation of four subgroup Ib BHLH genes in Arabidopsis thaliana. Planta 226, 897–908. doi: 10.1007/s00425-007-0535-x
Wang, P., Du, Y., Li, Y., Ren, D., and Song, C. P. (2010). Hydrogen peroxide-mediated activation of MAP kinase 6 modulates nitric oxide biosynthesis and signal transduction in Arabidopsis. Plant Cell 22, 2981–2998. doi: 10.1105/tpc.109.072959
Wendehenne, D., Durner, J., and Klessig, D. F. (2004). Nitric oxide: a new player in plant signalling and defence responses. Curr. Opin. Plant Biol. 7, 449–455. doi: 10.1016/j.pbi.2004.04.002
Xie, Y., Ling, T., Han, Y., Liu, K., Zheng, Q., Huang, L., et al. (2008). Carbon monoxide enhances salt tolerance by nitric oxide-mediated maintenance of ion homeostasis and up-regulation of antioxidant defence in wheat seedling roots. Plant. Cell. Environ. 31, 1864–1881. doi: 10.1111/j.1365-3040.2008.01888.x
Xie, Y. J., Xu, S., Han, B., Wu, M. Z., Yuan, X. X., Han, Y., et al. (2011). Evidence of Arabidopsis salt acclimation induced by up-regulation of HY1 and the regulatory role of RbohD-derived reactive oxygen species synthesis. Plant J. 66, 280–292. doi: 10.1111/j.1365-313X.2011.04488.x
Xuan, W., Zhu, F. Y., Xu, S., Huang, B. K., Ling, T. F., Qi, J. Y., et al. (2008). The heme oxygenase/carbon monoxide system is involved in the auxin-induced cucumber adventitious rooting process. Plant Physiol. 148, 881–893. doi: 10.1104/pp.108.125567
Yang, L., Fountain, J. C., Wang, H., Ni, X., Ji, P., Lee, R. D., et al. (2015). Stress sensitivity is associated with differential accumulation of reactive oxygen and nitrogen species in maize genotypes with contrasting levels of drought tolerance. Int. J. Mol. Sci. 16, 24791–24819. doi: 10.3390/ijms161024791
Yang, L., Tian, D., Todd, C. D., Luo, Y., and Hu, X. (2013). Comparative proteome analyses reveal that nitric oxide is an important signal molecule in the response of rice to aluminum toxicity. J. Proteome Res. 12, 1316–1330. doi: 10.1021/pr300971n
Yuan, Y., Wu, H., Wang, N., Li, J., Zhao, W., Du, J., et al. (2008). FIT interacts with AtbHLH38 and AtbHLH39 in regulating iron uptake gene expression for iron homeostasis in Arabidopsis. Cell Res. 18, 385–397. doi: 10.1038/cr.2008.26
Keywords: CO, NO, auxin, iron deficiency, Arabidopsis, basipetal transport
Citation: Yang L, Ji J, Wang H, Harris-Shultz KR, Abd_Allah EF, Luo Y, Guan Y and Hu X (2016) Carbon Monoxide Interacts with Auxin and Nitric Oxide to Cope with Iron Deficiency in Arabidopsis. Front. Plant Sci. 7:112. doi: 10.3389/fpls.2016.00112
Received: 05 November 2015; Accepted: 21 January 2016;
Published: 07 March 2016.
Edited by:
Shabir Hussain Wani, Sher-e-Kashmir University of Agricultural Sciences and Technology, IndiaReviewed by:
Jisen Shi, Nanjing Forestry University, ChinaHongxiao Zhang, Henan University of Science and Technology, China
Copyright © 2016 Yang, Ji, Wang, Harris-Shultz, Abd_Allah, Luo, Guan and Hu. This is an open-access article distributed under the terms of the Creative Commons Attribution License (CC BY). The use, distribution or reproduction in other forums is permitted, provided the original author(s) or licensor are credited and that the original publication in this journal is cited, in accordance with accepted academic practice. No use, distribution or reproduction is permitted which does not comply with these terms.
*Correspondence: Xiangyang Hu, aHV4aWFuZ3lhbmdAbWFpbC5raWIuYWMuY24=; Yanlong Guan, Z3VhbnlhbmxvbmdAbWFpbC5raWIuYWMuY24=
†These authors have contributed equally to this work.