- 1Laboratoire de Reproduction et Développement des Plantes, UMR 5667, Centre National de la Recherche Scientifique – Institut National de la Recherche Agronomique – Université de Lyon, Ecole Normale Supérieure de Lyon, Lyon, France
- 2Institute of Plant Sciences Paris-Saclay, Centre National de la Recherche Scientifique – Institut National de la Recherche Agronomique – Université de Paris Sud, Orsay, France
The majority of angiosperms are syncarpous- their gynoecium is composed of two or more fused carpels. In Arabidopsis thaliana, this fusion is regulated through the balance of expression between CUP SHAPED COTYLEDON (CUC) genes, which are orthologs of the Petunia hybrida transcription factor NO APICAL MERISTEM (NAM), and their post-transcriptional regulator miR164. Accordingly, the expression of a miR164-insensitive form of A. thaliana CUC2 causes a radical breakdown of carpel fusion. Here, we investigate the role of the NAM/miR164 genetic module in carpel closure in monocarpous plants. We show that the disruption of this module in monocarpous flowers of A. thaliana aux1-22 mutants causes a failure of carpel closure, similar to the failure of carpel fusion observed in the wild-type genetic background. This observation suggested that closely related mechanisms may bring about carpel closure and carpel fusion, at least in A. thaliana. We therefore tested whether these mechanisms were conserved in a eurosid species that is monocarpous in its wild-type form. We observed that expression of MtNAM, the NAM ortholog in the monocarpous eurosid Medicago truncatula, decreases during carpel margin fusion, suggesting a role for the NAM/miR164 module in this process. We transformed M. truncatula with a miR164-resistant form of MtNAM and observed, among other phenotypes, incomplete carpel closure in the resulting transformants. These data confirm the underlying mechanistic similarity between carpel closure and carpel fusion which we observed in A. thaliana. Our observations suggest that the role of the NAM/miR164 module in the fusion of carpel margins has been conserved at least since the most recent common ancestor of the eurosid clade, and open the possibility that a similar mechanism may have been responsible for carpel closure at much earlier stages of angiosperm evolution. We combine our results with studies of early diverging angiosperms to speculate on the role of the NAM/miR164 module in the origin and further evolution of the angiosperm carpel.
Introduction
The female whorl, or gynoecium, of the angiosperm flower consists of one or more carpels which enclose the ovules. In apocarpous gynoecia, the carpels remain separate throughout development, while in syncarpous gynoecia, they are fused together, either from their inception (congenital fusion), or from a later developmental stage (post-genital fusion). If only one carpel is produced per flower, the gynoecium is termed monocarpous. Carpels in apocarpous or monocarpous gynoecia may emerge from the floral meristem with their margins already fused together, in which case they are described as ascidiate (bottle-shaped), or may emerge with unfused margins that subsequently fuse by folding, in which case they are described as plicate.
Syncarpy is believed to confer several selective advantages over apocarpy, including a larger landing platform for pollinating insects, a compitum (a common intersection in the route for pollen tube growth), and larger fruits with more sophisticated mechanisms for seed dispersal. Mapping of character states onto angiosperm phylogeny indicates that syncarpy has arisen at least 17 times in the angiosperms, while the evolution of apocarpy from syncarpy is much less frequent (Armbruster et al., 2002).
The model angiosperm Arabidopsis thaliana possesses a syncarpous gynoecium of two congenitally fused carpels. These organs emerge from the center of the floral meristem as a single dome of cells, within which a central slot-like cavity forms as the gynoecium begins to elongate (Smyth et al., 1990). The positions of the carpel margins within the gynoecium wall only become apparent at a later stage, when this structure undergoes differentiation into valve and abaxial replum tissues. Meristematic activity from the abaxial replum then generates the adaxial replum, or septum, which grows inward to divide the ovary into two chambers. Ovule primordia develop from parietal placentae which form along the carpel margins within each chamber of the ovary.
In contrast to A. thaliana, the model angiosperm Medicago truncatula possesses a plicate, monocarpous gynoecium (Benlloch et al., 2003). At an early stage of M. truncatula flower development, the gynoecial primordium becomes crescent-shaped and its margins then fuse together to enclose the single chamber of the ovary. Ovule primordia in M. truncatula form from a parietal placenta that develops along the fused carpel margin.
Carpel fusion in A. thaliana is regulated by a genetic module, generically termed here the NAM/miR164 module, which consists of a subset of NAC-family (NAC for NAM, ATAF and CUC; Aida et al., 1997) transcription factors and their post-transcriptional regulator miR164 (Mallory et al., 2004). In A. thaliana, the NAC genes involved in this module are CUP-SHAPED COTYLEDON1 (CUC1) and CUC2 (Aida et al., 1997), which are orthologs of the single gene NO APICAL MERISTEM (NAM) from Petunia hybrida (Souer et al., 1996). Loss of miR164 function through mutations to all three MIR164 paralogs in A. thaliana (Sieber et al., 2007), or genetic transformation of A. thaliana with a miR164-resistant version of CUC2 (CUC2g-m4; Nikovics et al., 2006), results in a breakdown of carpel fusion. Accordingly, in miR164 triple mutants or CUC2g-m4 transformants, the two carpels of the A. thaliana gynoecium emerge separately and remain unfused and open throughout development.
In addition to their role in carpel development, studies of NAM orthologs in eudicots show these factors to be involved in meristem formation and cotyledon development (Souer et al., 1996; Aida et al., 1997, 1999; Takada et al., 2001; Weir et al., 2004), leaf development (Ishida et al., 2000; Nikovics et al., 2006; Blein et al., 2008), ovule development (Galbiati et al., 2013; Kamiuchi et al., 2014) and phyllotaxy (Peaucelle et al., 2007). These transcription factors are expressed at organ margins and tissue boundaries, and their down-regulation by miR164 consequently facilitates organ outgrowth and/or developmental fusion. The action of the NAM/miR164 module in the A. thaliana leaf margin has been modeled and found to generate, via effects on the auxin efflux carrier PINFORMED1 (PIN1), an alternating series of auxin maxima and minima that, respectively, generate regions of higher and lower marginal growth (Bilsborough et al., 2011).
In this work, we hypothesized that the role of the NAM/miR164 module in syncarpous fusion in A. thaliana might reflect a more general role in the fusion of carpel margins in angiosperms. Consequently, we tested the role of this module in the closure of monocarpous gynoecia produced both in A. thaliana aux1-22 mutants, which are null mutants of the AUX1 auxin influx transporter (Bennett et al., 1996) and in a wild-type genetic background of the distantly related eurosid M. truncatula. From the results of these experiments, we conclude that the NAM/miR164 module has conserved a role in carpel margin fusion, at least since the most recent common ancestor (MRCA) of living eurosids. A detailed comparison of gene expression patterns suggests that fine-tuning of the NAM/miR164 module may regulate species–specific differences in the timing of carpel margin fusion. Accordingly, we discuss the possibility that the activity of the NAM/miR164 module may be conserved in carpel development throughout the angiosperms, while subtle modulations to this mechanism may determine the distinction between congenital and post-genital carpel margin fusion events in specific angiosperm groups. We further speculate on mechanisms acting upstream of the NAM/miR164 module that may have contributed to the origin of the carpel in the first flowering plants.
Materials and Methods
Plant Cultivation
Arabidopsis thaliana plants were grown from seed on peat-based compost in growth chambers at a daytime temperature of ∼21°C and ∼55% relative humidity (RH). Plants were initially grown under 8/16 h day/night cycles generated using fluorescent lighting consisting of equal numbers of “cool daylight” (Osram Lumilux L36W/865) and “warm white” (Osram Lumilux L36W/830) lamps, giving a total photon flux at bench level of 170 μmol.m-2.s-1. To induce flowering, plants were transferred to long days (16/8 h day/night cycles) under otherwise similar conditions.
Medicago truncatula plants were grown from seed on peat-based compost in a greenhouse at a daytime temperature of ∼22.5°C and 40–60% RH under natural daylight, extended to 16 h daylength using sodium lamps, as necessary.
Vector Construction
MtNAM (MTR_2g078700; Cheng et al., 2012) was initially isolated by radioisotopic screening of an M. truncatula bacterial artificial chromosome (BAC) library (Nam et al., 1999). A 1.2-kb fragment containing the miR164-binding site of MtNAM was released from a sub-cloned BAC DNA fragment by cleavage with SstI and re-ligated into the pGEM T-Easy vector. The resulting plasmid was subjected to oligonucleotide-directed site-specific mutagenesis following the method of Kirsch and Joly (1998), using the sense- and antisense-strand oligonucleotides 5′-GAGCACGTGTCCTGTTTtagtACAACATCTACAACATC and 5′-GATGTTGTAGATGTTGTactaAAACAGGACACGTGCTC, respectively. These oligonucleotides generate the same four-base mismatch (shown above in lower case) present in the miR164-binding site of CUC2g-m4 (Nikovics et al., 2006). Mutagenised and wild-type versions of a MtNAM genomic sequence of 9883 bp, from an EcoRI site situated 6437 bp upstream of the MtNAM initiation codon to an NcoI site situated 2151 bp downstream of its termination codon, were then inserted by ligation between unique EcoR1 and Not1 sites situated between the Left and Right T-DNA borders of the pGREEN II-NosHyg plant transformation vector, thereby generating the plasmids MtNAMg-m4 and MtNAMg-wt, respectively.
Plant Transformation
Arabidopsis thaliana aux1-22 mutants (null mutants of AUX1; Bennett et al., 1996) were transformed by the “floral dip” method (Clough and Bent, 1998) using the CUC2g-wt and CUC2g-m4 constructs of Nikovics et al. (2006) in Agrobacterium tumefaciens strain GV3101 harboring the plasmids pMP90 (Koncz and Schell, 1986) and pSOUP (Hellens et al., 2000). Transformants were selected on plant agar containing 50 μg/mL kanamycin.
MtNAMg-wt and MtNAMg-m4 constructs were introduced into A. tumefaciens GV3101, as described above, and used to transform M. truncatula R108 leaf disks by the protocol of Cosson et al. (2015), in which transgenic calli were selected on media containing 30 μg/mL hygromycin.
In Situ Hybridization
Double-stranded cDNAs representing the full-length coding sequences of A. thaliana CUC1 and CUC2 and of M. truncatula MtNAM were generated by reverse-transcriptase PCR, incorporating a T7-RNA-Polymerase promoter sequence in the reverse primer. Digoxgenin-labeled riboprobes were prepared from these templates using T7 RNA-polymerase and these were then purified and used in in situ hybridizations to sections of fixed floral buds embedded in Paraplast Xtra (Leica-Surgipath), as described by Vialette-Guiraud et al. (2011b). Gene expression patterns were observed and photographed under bright field illumination using a Leica Axio Imager M2 inverted microscope fitted with a Leica AxioCam MRc digital camera.
Phenotypic Observations
Flower buds were dissected, observed, and photographed using a Leica MZ12 dissecting microscope fitted with an AxioCam ICc5 digital camera. Carpel anatomy was revealed in transverse sections of fixed flower buds, prepared as for in situ hybridization and stained with 0.05% (w/v) Toluidine Blue-0 in 0.1 M sodium phosphate buffer (pH 6.8). Scanning electron microscopy was performed on unfixed material using a Hirox 3000 bench-top environmental scanning electron microscope (SEM).
Character State Mapping
A partial cladogram of angiosperm phylogeny was produced, based on the current consensus view of angiosperm phylogeny given by the Angiosperm Phylogeny Group III (APG III, http://www.mobot.org/MOBOT/research/APweb/; Bremer et al., 2009). Carpel fusion character states, obtained from the APG III website and from bibliographic searches, were mapped on this cladogram by maximum parsimony using MacClade4 software.
Results
Monocarpy in Medicago truncatula Arose by Reversion from Syncarpy in a Common Ancestor Shared with Arabidopsis thaliana
To elucidate transitions in carpel fusion in the angiosperms, with emphasis on the model eurosids M. truncatula and A. thaliana, we mapped this character state onto a cladogram (Figure 1) representing the consensus view of angiosperm phylogeny (Bremer et al., 2009). This analysis confirms the findings of earlier studies (Armbruster et al., 2002) which indicated that the MRCA of living angiosperms was apocarpous, and that syncarpy arose several times independently, including in Nymphaeaceae, monocots, Papaveraceae and a common ancestor of the rosids and asterids. Within the eurosids, our analysis indicates that monocarpy in Fabales (including M. truncatula), arose secondarily from syncarpy, which was present in a common ancestor shared with Brassicales (including A. thaliana), Celastrales and Malpighiales. By localizing transitions between apocarpy/monocarpy and syncarpy, this analysis provides a phylogenetic framework for the evolutionary interpretation of data on the molecular mechanisms involved in these processes in living angiosperms.
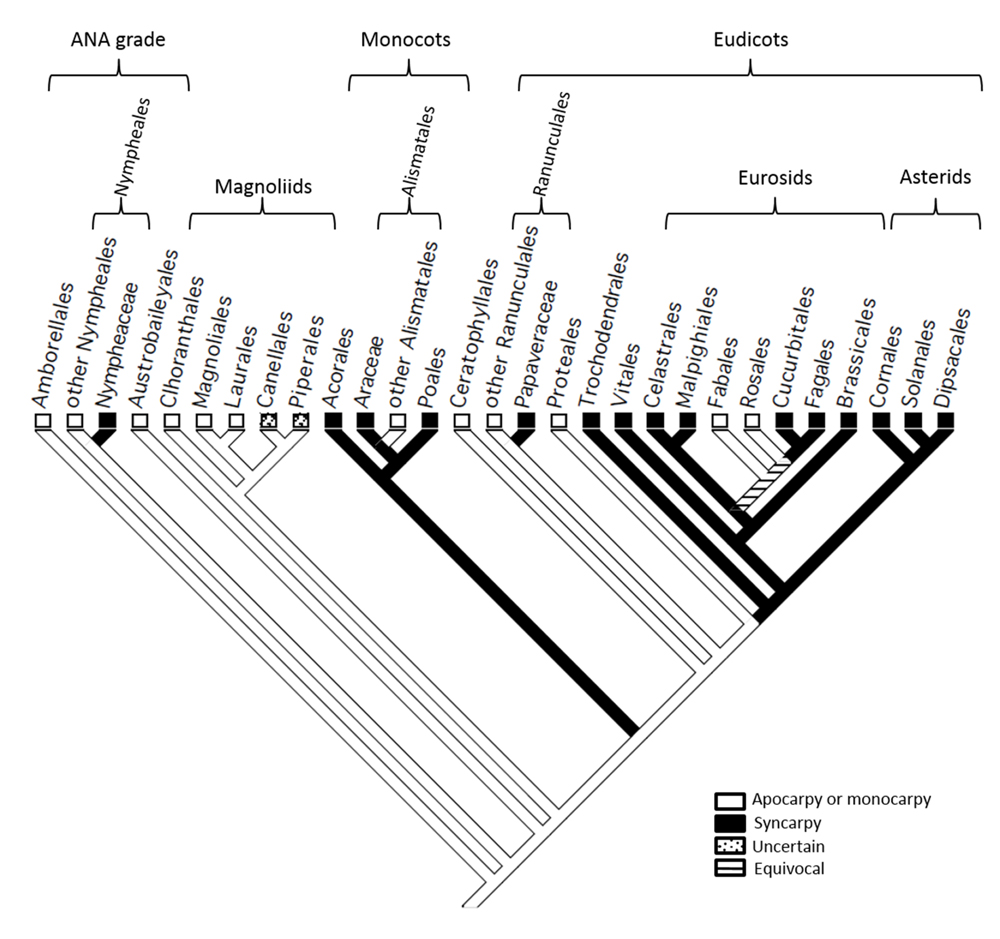
FIGURE 1. A partial cladeogram of the angiosperms showing evolutionary transitions in carpel fusion. Monocarpy in Fabales (including Medicago trucatula) is revealed in this analysis to have reverted from syncarpy present in a common ancestor shared with Brassicales (including Arabidopsis thaliana), Celastrales and Malpighiales.
The NAM/miR164 in Arabidopsis thaliana Plays a Role in Both Syncarpy and the Closure of Single Carpels
As the NAM/miR164 developmental module is necessary for carpel fusion in wild-type, syncarpous A. thaliana (Nikovics et al., 2006; Sieber et al., 2007), we aimed to discover whether this mechanism could also contribute to the closure of single carpels in this species. To do this, we tested whether the introduction of a miR164-resistant version of CUC2 (CUC2g-m4) could cause a breakdown in the closure of the single carpels that are produced in A. thaliana aux1-22 mutants (Bennett et al., 1996), as compared to control plants transformed with a wild-type construct (CUC2g-wt).
Wild-type Col-0 gynoecia are syncarpous (Figure 2A), as are approximately 50% of gynoecia produced in aux1-22 mutants (Figure 2B). The ovary wall in these gynoecia contains two valves, alternating with two abaxial repla. The monocarpous gynoecia, which are also produced in aux1-22 mutants (Figures 2C,D), develop as closed structures whose ovary wall consists of only one valve and one abaxial replum (Figure 2C). These monocarpous gynoecia are not divided by a septum, or adaxial replum. Transformation of aux1-22 mutants with CUC2g-wt produced no apparent change in the morphology of monocarpous gynoecia (Figure 2E). However, transformation of these mutants with CUC2g-m4 produced a high proportion of monocarpous gynoecia that remained open to maturity (Figures 2F–I). In eight of 20 T1 transformants analyzed, all flowers containing two carpels showed carpel fusion defects, while all monocarpous flowers showed a complete or partial lack of carpel closure, remaining open over part or all of the valve margin. In these eight plants, carpel fusion/closure defects resulted in an almost complete loss of female fertility. Thus, disruption of the NAM/miR164 developmental module in monocarpous mutant gynoecia of A. thaliana causes the failure of developmental closure in these structures in a similar manner to the disruption of carpel fusion in syncarpous, wild-type gynoecia.
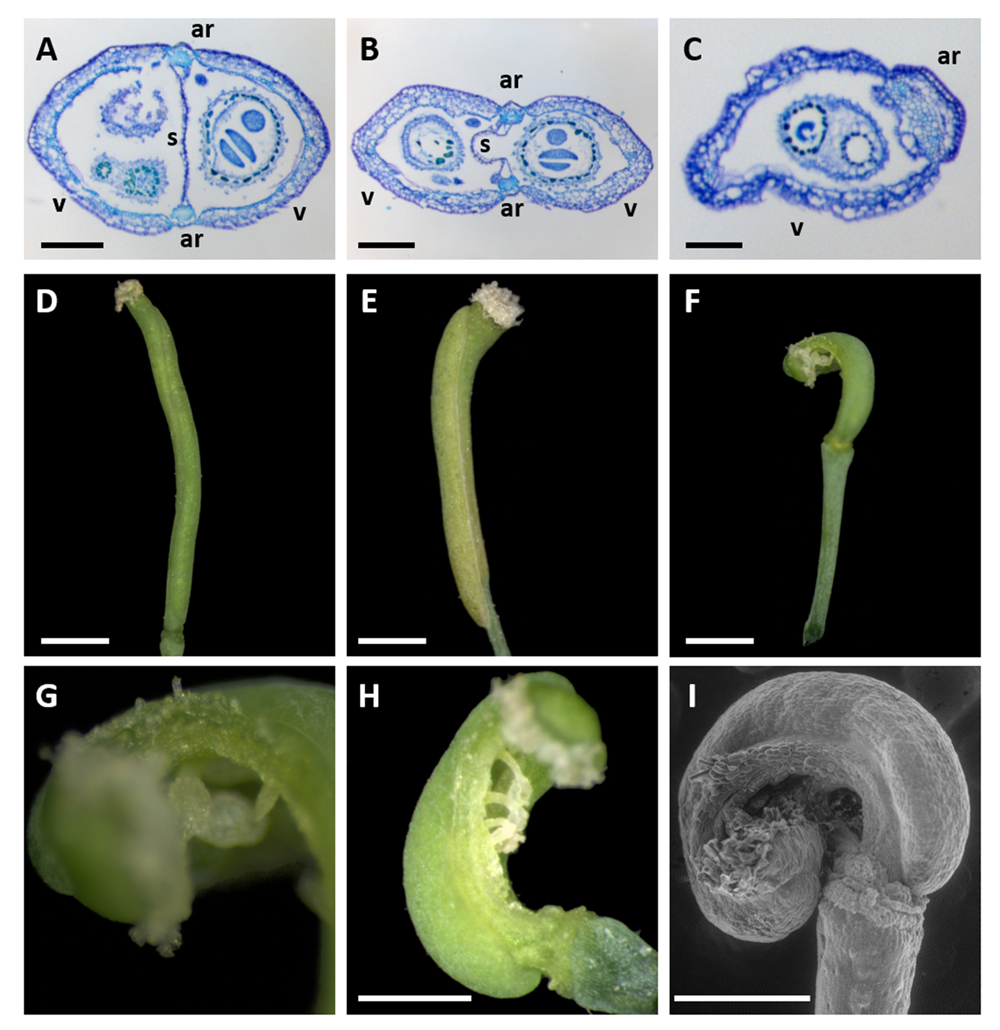
FIGURE 2. Gynoecium morphology of A. thaliana aux1-22 mutants transformed with miR164-resistant (CUC2g-m4) or un-mutated control (CUC2g-wt) constructs. (A–C) Toluidine blue staining of transverse sections of Col-0 wild-type (A) and aux1-22 mutant gynoecia composed of two fused carpels (B), and one closed carpel (C), respectively. (D,E) An untransformed aux1-22 mutant (D) and an aux1-22 CUC2g-wt (control) transformant showing entirely closed monocarpous gynoecia. (F–I) aux1-22 CUC2g-m4 transformants showing a breakdown carpel closure in monocarpous gynoecia. (G is a magnification of the apex of the carpel shown in (F). I is a scanning electron microscope image) ar, abaxial replum; s, septum (or adaxial replum); v, valve. Bars = 200 μm in (A,B), 100 μm in (C), and 1 mm in (D–F,H,I).
Expression of NAM Orthologs is Absent or Reduced During Carpel Margin Fusion in Arabidopsis thaliana and Medicago truncatula
The observation that the NAM/miR164 module regulates developmental closure events in the gynoecium in both syncarpous and monocarpous genotypes of A. thaliana led us to speculate that this molecular mechanism might be widely conserved within the angiosperms. We chose M. truncatula, which produces in its wild-type form a single carpel in each flower, as a candidate model species in which to test this hypothesis. The MRCA between M. truncatula and A. thaliana, which is also the MRCA of the living eurosid clade (comprising Fabidae, or eurosids I and Malvidae, or eurosids II), is estimated to have lived 114–113 million years ago (MYA; Wang et al., 2009). Prior to initiating functional experiments in M. truncatula, we used in situ hybridization to examine the conservation of expression of NAM orthologs in flower tissues between A. thaliana and M. truncatula and thereby ascertain the likelihood that the NAM/miR164 module might function in carpel closure in the latter species.
In situ hybridization in A. thaliana flowers at Stage 7 (Smyth et al., 1990), in which a central slot is beginning to form in the gynoecial cylinder, revealed the expression of CUC2 in the adaxial domain of the gynoecium and in the loculi of the developing anthers (Figure 3A). Recent studies (Galbiati et al., 2013) revealed similar results for CUC1. Thus, no expression of either CUC1 or CUC2 has been detected in regions of the ovary wall destined to become the abaxial repla, or the fusion zones between these tissues and the valves. At Stage 9–10, both CUC1 and CUC2 were expressed in the placentae and at presumptive tissue boundaries within the elongating ovule primordia (Figures 3B,C). At Stage 11, CUC1 was expressed at the base of the expanding ovule integuments (Figure 3D).
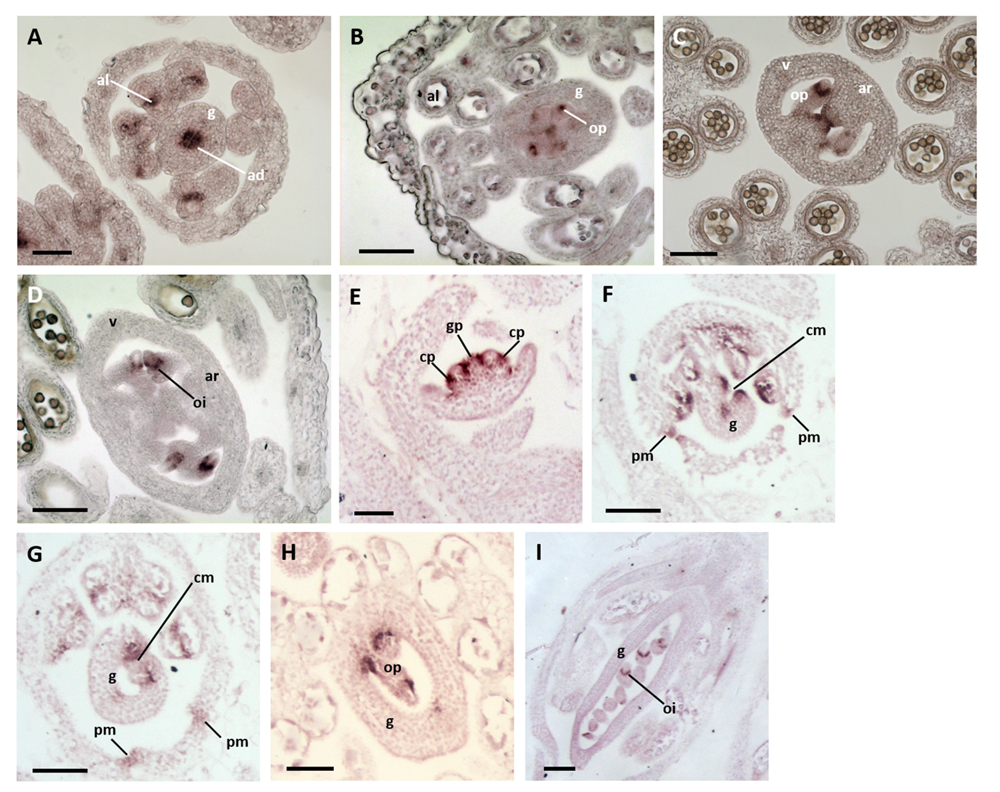
FIGURE 3. Expression of NAM orthologs in A. thaliana and Medicago truncatula flower buds. (A–D) A. thaliana flower buds hybridized to CUC1 and 2 probes. (A) A Stage-7 bud showing CUC2 expression in the abaxial domain of the gynoecium and anther locculi. (B,C) Buds at Stages 9–10 showing CUC1 (B) and CUC2 (C) expression in the placenta and within ovule primordia. (D) A bud at Stage 11 showing CUC1 expression at the base of the expanding ovule integuments. ad, adaxial zone of the gynoecium; al, anther loculus; ar, abaxial replum; g, gynoecium; oi, ovule integuments; op, ovule primordium. Bars = 50 μm. (E–I) M. truncatula flower buds hybridized to an MtNAM probe. (E) A bud at Stages 3–4 showing MtNAM expression between and within organ primordia. (F) A Stage-7 bud showing strong MtNAM expression in the unfused carpel margins. (G) A bud at Stage 8, in which MtNAM expression is reduced in the fused carpel margins. (H,I) Later stages of flower development in which MtNAM expression is absent in the carpel margins, but present first in ovule primordia (H) and then at the base of the expanding integuments (I). cm, carpel margin; cp, common primordium; g, gynoecium; gp, gynoecial primordium; oi, ovule integument; op, ovule primordium; pm, petal margin. Bars = 100 μm.
In situ hybridization in M. truncatula at Stages 3–4 of flower development, following the time course defined by Benlloch et al. (2003), showed MtNAM expression between the gynoecium primordium and the surrounding common primordia that give rise to both stamens and petals (Figure 3E). Signals were also detected within these common primordia (Figure 3E), marking the boundary between the zones destined to produce petals and stamens. At Stage 7, the gynoecium appeared crescent-shaped in transverse section and MtNAM was clearly expressed in the carpel margins, and at the margins of the developing free petals (Figure 3F). By early Stage 8, expression of MtNAM was observed to decline in the carpel margins (Figure 3G), which had, by this time, fused together to close the gynoecium. At later developmental stages, MtNAM expression is present in presumptive tissue boundaries in the elongating ovule primordia (Figure 3H) and, following this, at the base of the expanding integuments of the ovule (Figure 3I). Similar NAM-ortholog expression patterns in floral organ and ovule primordia were previously shown in another species of Fabaceae, Pisum sativum (Blein et al., 2008).
These expression data reveal several underlying similarities in the expression of miR164-regulated NAM orthologs between A. thaliana and M. truncatula. These orthologs are highly expressed in both species at frontiers between and within floral organs, particularly during ovule development. These data do, however, reveal a difference in NAM expression in the carpel margins- no such expression was detected in the presumptive abaxial repla of the gynoecial tube at early stages of A. thaliana flower development, whereas NAM expression was detected in the carpel margins of the early M. truncatula gynoecium. This difference may relate to the contrasting modes of congenital and post-genital carpel margin fusion in A. thaliana and M. truncatula, respectively. Despite the differences observed, we concluded that the presence of MtNAM expression in M. truncatula carpel margins suggested that the NAM/miR164 module may be involved in the fusion of these structures, leading us to test this hypothesis experimentally.
Expression of a miR164-Resistant form of MtNAM Leads to a Breakdown in Carpel Margin Fusion and Other Developmental Fusion Events in Medicago truncatula Flowers
To test the role of the NAM/miR164 developmental module on carpel closure in M. truncatula, we produced transgenic plants expressing genomic constructs of MtNAM (MtNAMg-m4 and MtNAMg-wt), respectively, with or without four point mutations in their predicted miR164-binding sites, identical to those present in the CUC2g-m4 construct (Nikovics et al., 2006). Three independent transgenic MtNAMg-m4 calli were generated, two of which were successfully regenerated into fertile adult plants, as was one transgenic callus containing an MtNAMg-wt construct (Table 1). Phenotypic observations were made on T2 progeny representative of one of each of these transformed lines, and on untransformed plants for comparison (Table 2; Figure 4).
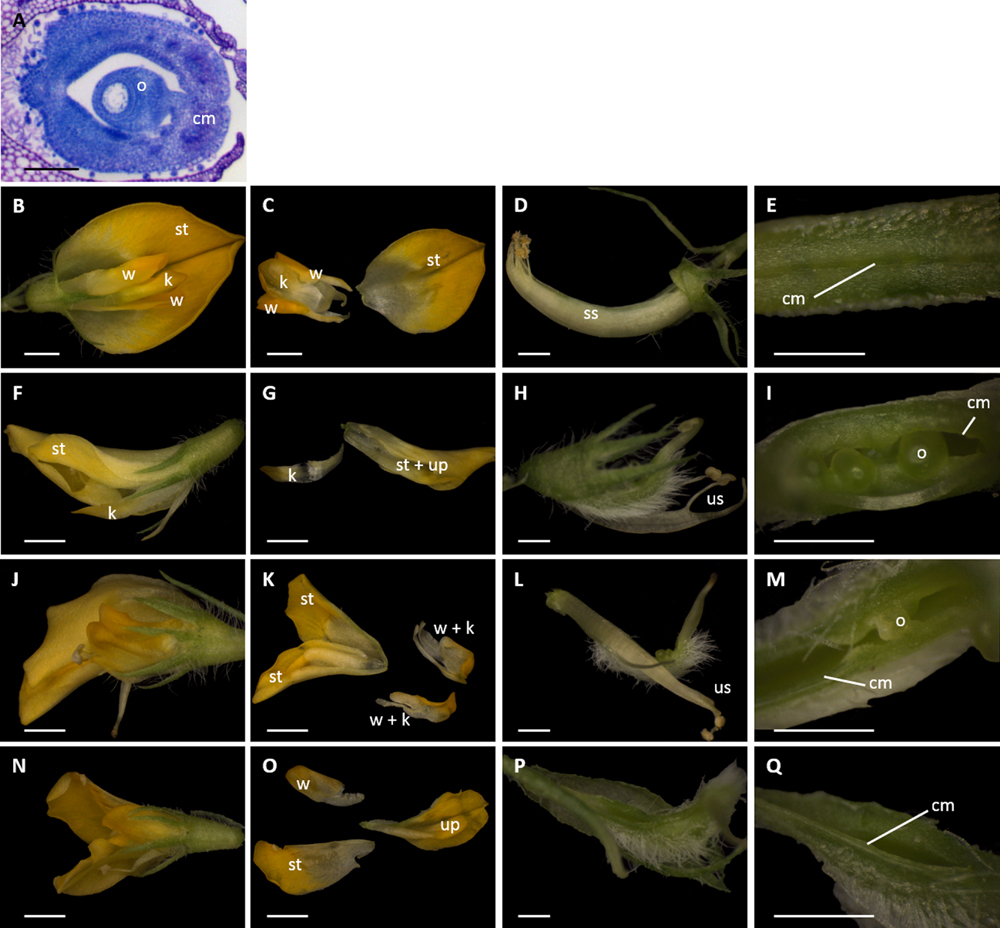
FIGURE 4. Dissections of M. truncatula flowers transformed with miR164-resistant (MtNAMg-m4) or wild-type control (MtNAMg-wt) constructs. (A) Transverse section of wild-type M. truncatula gynoecium stained with toluidine blue. (B–E) A typical flower of an MtNAMg-wt transformant showing (B) the intact flower, (C) petal morphology, (D) the sheath of anther filaments surrounding the gynoecium, and (E) the carpel margins. All structures in (B–E) appear identical to wild-type. (F–I), (J–M), and (N–Q) Three representative flowers from MtNAM-m4 transformants showing (F,J,N) the intact flower, (G,K,O) petal morphology, (H,L,P) after removal of the perianth, and (I,M,Q) the carpel margins. Defects in the corolla, androecium and gynoecium are apparent, including a marked breakdown in carpel margin fusion in most flower buds (e.g., I,M). cm, carpel margin; k, keel petal(s); o, ovule; ss, stamen sheath; st, standard petal; up, unidentified petal(s); us, unfused stamens; w, wing petal. Bars = 100 μm in (A), 1 mm in (B,C,F,G,J,K,N,O), and 0.5 mm in (D,E,H,I,L,M,P,Q).
Toluidene-blue staining was performed to highlight the ovule and the fused region of the carpel margins in the gynoecium of untransformed M. truncatula (Figure 4A). Transformation with MtNAMg-wt (Figures 4B–E) showed no effects on flower development compared to wild type M. truncatula. Accordingly, in MtNAMg-wt transformants, as in wild-type, five petals were produced, including two fused “keel” petals, two unfused “wing” petals, and a single “standard” petal (Figures 4B,C). As in the wild-type, all stamen filaments, with the exception of a single stamen positioned adjacent to the standard, were fused into a sheath surrounding the gynoecium (Figure 4D). The carpel margins of MtNAMg-wt transformants were also developmentally fused in the mature gynoecium, as in wild-type (Figure 4E).
By contrast, a range of mutant phenotypes were noted in flowers of plants transformed with the MtNAMg-m4 construct (Table 2; Figures 4F–Q). Two standard petals were produced in some flowers (Figure 4K), while in others, petals with altered morphology and fusion were produced, rendering difficult their identification as standard, wing or keel petals (Figures 4G,O). Unfused stamens were produced in some cases (Table 2; Figures 4H,L), while stamens were absent in others (Table 2; Figure 4Q). The carpel margins remained unfused in many flowers (Table 2; Figures 4I,M), revealing the ovules within these, though a small proportion of flowers did show completely fused carpel margins (Table 2; Figure 4Q).
These data indicate a range of roles of the NAM/miR164 developmental module in fusion events in the corolla, androecium, and gynoecium of M. truncatula flowers. Of particular interest to the current work, the elimination of post-transcriptional regulation of MtNAM in the gynoecium is shown to have a similar effect in M. truncatula to that shown on aux1-22 mutants of A. thaliana (Figure 2) by disrupting the fusion of carpel margins.
Discussion
A Role of the NAM/miR164 Module in the Fusion of Carpel Margins has Been Conserved at Least Since the MRCA of the Eurosids
In this study, we show that a previously characterized developmental module involving the post-transcriptional regulation of NAM orthologs by miR164 is involved not only in carpel fusion in syncarpous A. thaliana (Nikovics et al., 2006; Sieber et al., 2007), but also in the closure of the single carpels present in two species whose lineages diverged at the base of the eurosid clade, some 114–113 MYA. The two species concerned are A. thaliana itself, as aux1-22 mutants of A. thaliana produce single carpels, and M. truncatula, which is monocarpous in its wild-type form. We show that disruption of the NAM/miR164 module in both A. thaliana aux1-22 mutants (Figure 2) and a wild-type background of M. truncatula (Figure 4) produces single carpels that are no longer completely fused at their margins.
These data indicate that the NAM/miR164 module has conserved a role in developmental fusion events between carpel margins at least since the MRCA of the eurosids. The mapping of character states onto angiosperm phylogeny (Figure 1) indicates that the MRCA of the eurosids was syncarpous, and we may thus conclude that the NAM/miR164 module contributed to carpel fusion in that key ancestor, from which some 70 000 extant species are descended (Wang et al., 2009).
The NAM/miR164 Module Maintained its Role in Carpel Margin Fusion During a Transition from Syncarpy to Monocarpy in an Ancestor of Fabales
Character-state mapping (Figure 1) further indicates that the monocarpy present in Fabales (including M. truncatula) is a derived condition that occurred by reversion from syncarpy, present in earlier eurosids. In the present work, we show that the role of the NAM/miR164 module in carpel margin fusion was conserved during this developmental transition. Thus, our study strongly suggests that the NAM/miR164 module provides an underlying mechanism that is necessary for fusion events at the carpel margins of both syncarpous and monocarpous eurosids.
It is interesting to note that the aux1-22 mutation in A. thaliana causes a transition from a congenitally fused gynoecium of two carpels to a closed, monocarpous gynoecium. Thus, a single loss-of-function mutation in a gene involved in auxin signaling can bring about, in A. thaliana, a similar type of morphological transition to that which led to monocarpy in Fabales. The genetic simplicity of this transition suggests that reversions from syncarpy to monocarpy might occur frequently in natural populations. The general trend in the angiosperms, however, is for evolutionary transitions toward syncarpous gynoecia, which are believed to confer numerous selective advantages (Armbruster et al., 2002). Thus, while the loss of syncarpy may be a genetically “easy” transition to make, the fixation of this trait in populations by natural selection may occur much less frequently.
A Possible Role for the NAM/miR164 Module in the Timing of Carpel Fusion
In A. thaliana, the gynoecium forms as a radially symmetrical cylinder that later differentiates to show the positions of the carpel margins. By contrast, the single carpel of the M. truncatula gynoecium is plicate, and closes post-genitally by the fusion of preexisting carpel margins. In situ hybridization in this work (Figure 3) and other studies (Galbiati et al., 2013) failed to detect any expression of CUC1 or CUC2 in the carpel margins of A. thaliana. However, CUC2 is known to be highly expressed in the carpel margins of the unfused gynoecium at Stage 9 of flower development in mir164abc triple mutants (Sieber et al., 2007). Comparison of these data strongly suggests that the NAM/miR164 expression balance in A. thaliana lies heavily in favor of miR164 from the earliest stages of gynoecium development. By contrast, detectable levels of MtNAM were present in margin tissues at early stages of M. truncatula carpel development, and these levels were observed to decline at subsequent stages, as the margins fused (Figure 3). Thus, the different balances of NAM and miR164 expression observed at very early stages of A. thaliana and M. truncatula carpel development (Figure 3; Galbiati et al., 2013) correlate closely with the different timings of carpel closure observed in these species (Smyth et al., 1990; Benlloch et al., 2003).
Given the role of the NAM/miR164 module in carpel closure in both A. thaliana and M. truncatula (Figures 2 and 4), and the gene expression differences we have noted between the congenitally and post-genitally fused carpel margins of these two respective species, it would be interesting to compare the expression of NAM orthologs in a range of Fabales that show different spatial and temporal patterns of carpel closure. Candidate species for this analysis include Acacia celastrifolia and Inga bella (Paulino et al., 2014), in which the carpels include both congenitally fused (ascidiate) and later-fusing (plicate) zones, and Amberstia nobilis and Caesalpina spp. (Tucker and Kantz, 2001), in which the carpel margins remain unfused until after ovule initiation, much later than in most other Fabales. Such experiments, in quite closely related species showing marked differences in gynoecium anatomy, could provide strong correlative evidence of a role for the subtle modulation of gynoecium development by changes to the balance of the NAM/miR164 module. Notably, the NAM/miR164 expression balance at very early stages of carpel development may be important in determining whether carpel margins will fuse congenitally or postgenitally.
The Role of the NAM/miR164 Module in Carpel Evolution
As its genetic components are present in both gymnosperms and angiosperms (Axtell and Bartel, 2005; Larsson et al., 2012), the NAM/miR164 genetic module is clearly of ancient origin in seed plants. This module is involved in leaf, carpel, and ovule development in model angiosperms (Nikovics et al., 2006; Blein et al., 2008; Galbiati et al., 2013; Goncalves et al., 2015), while expression studies in Amborella trichopoda, the only living representative of Amborellales (see Figure 1), and hence the likely sister to all other living angiosperms, suggest that its role in ovule development, at least, has been conserved from the earliest stages of angiosperm evolution (Vialette-Guiraud et al., 2011a).
Like most basally diverging angiosperms, A. trichopoda is apocarpous and has ascidiate carpels. Thus, in both A. trichopoda and A. thaliana, the carpel margins are congenitally fused from the earliest stages of gynoecium development, albeit in the different contexts of apocarpy and syncarpy, respectively. No expression of the NAM ortholog from A. trichopoda, AtrNAM, was observed in the early carpel wall (Vialette-Guiraud et al., 2011a), as is the case for CUC1 and CUC2 in A. thaliana (Galbiati et al., 2013; Figure 3). Thus, it appears reasonable to postulate that the NAM/miR164 module operates in favor of the expression of miR164, and against that of NAM orthologs, from the earliest stages of gynoecium development in A. trichopoda, as it does in A. thaliana.
From the above observations, we hypothesize that the NAM/miR164 module may have played a role in the fusion of carpel margins in the MRCA of the living angiosperms, as it does in present-day model angiosperms. An important test of this hypothesis will depend on the development of plant transformation strategies in basally diverging angiosperms, which would allow, for example, the transformation of A. trichopoda with a miR164-resistant form of AtrNAM. Comparison of early diverging angiosperm lineages strongly suggests that the first flowering plants possessed ascidiate, rather than plicate carpels (Endress and Igersheim, 2000). Accordingly, we furthermore hypothesize, based on our gene expression analyses (Figure 3), that the origin of plicate carpels in various later-emerging angiosperm lineages may have depended on subtle modifications to the NAM/miR164 module that allowed a limited level of early expression of NAM orthologs in the carpel margins, as occurs in present-day M. truncatula.
Interestingly, it is known that in A. thaliana, the role of CUC2 in the closure of the gynoecium apex is under indirect negative control by the bHLH transcription factor SPATULA (SPT; Nahar et al., 2012). In addition, SPT is known to play a role in carpel fusion along the entire length of the gynoecium, which is revealed in double-mutant combinations with the YABBY transcription factor CRABS CLAW (crc-1 spt-2; Alvarez and Smyth, 1999). Like the NAM/miR164 module, it seems that SPT may have conserved its function in carpel development from the earliest stages of angiosperm evolution (Reymond et al., 2012). Thus, the establishment of negative regulation by SPT of a miR164-regulated NAM gene in a common ancestor of the angiosperms may have been a crucial step in the evolution of the closed carpel. Analysis of the pathway linking SPT, and its cofactors such as the HECATE transcription factors (Schuster et al., 2015), with the NAM/miR164 module in model angiosperms could provide insights into this possibility, and thus potentially indicate a molecular mechanism for the enclosure of the ovule with the carpel in the first angiosperms.
Author Contributions
AV-G performed all of the work except Medicago transformation, prepared the figures and collaborated with CS to plan and write the paper. AC assisted with in situ hybridizations of Arabidopsis. JG-M assisted with in situ hybridizations of Medicago. AE performed Medicago transformations. PR supervised Medicago transformations. CS supervised all work except Medicago transformation and collaborated with AV-G to plan and write the paper.
Funding
This work was supported by research grants ANR-13-BSV2-0009 “ORANGe” to CS and ANR-11-BSV2-0005 “Charmful” to PR. AV-G is funded through an ENS-Lyon research and teaching position.
Conflict of Interest Statement
The authors declare that the research was conducted in the absence of any commercial or financial relationships that could be construed as a potential conflict of interest.
Acknowledgments
We thank Téva Vernoux for supplying aux1-22 seed and Patrick Laufs for making available the CUC2g-m4 and CUC2g-wt constructs. We are grateful to Patrick Laufs and Mike Frohlich for helpful discussions.
References
Aida, M., Ishida, T., Fukaki, H., Fujisawa, H., and Tasaka, M. (1997). Genes involved in organ separation in Arabidopsis: an analysis of the cup-shaped cotyledon mutant. Plant Cell 9, 841–857. doi: 10.1105/tpc.9.6.841
Aida, M., Ishida, T., and Tasaka, M. (1999). Shoot apical meristem and cotyledon formation during Arabidopsis embryogenesis: interaction among the CUP-SHAPED COTYLEDON and SHOOT MERISTEMLESS genes. Development 126, 1563–1570.
Alvarez, J., and Smyth, D. R. (1999). CRABS CLAW and SPATULA, two Arabidopsis genes that control carpel development in parallel with AGAMOUS. Development 126, 2377–2386.
Armbruster, W. S., Debevec, E. M., and Willson, M. F. (2002). Evolution of syncarpy in angiosperms: theoretical and phylogenetic analyses of the effects of carpel fusion on offspring quantity and quality. J. Evol. Biol. 15, 657–672. doi: 10.1046/j.1420-9101.2002.00414.x
Axtell, M. J., and Bartel, D. P. (2005). Antiquity of microRNAs and their targets in land plants. Plant Cell 17, 1658–1673. doi: 10.1105/tpc.105.032185
Benlloch, R., Navarro, C., and Beltran, J. P. (2003). Floral development of the model legume Medicago truncatula: ontogeny studies as a tool to better characterize homeotic mutations. Sex. Plant Reprod. 15, 231–241. doi: 10.1007/s00487-002-0157-1
Bennett, M. J., Marchant, A., Green, H. G., May, S. T., Ward, S. P., Millner, P. A., et al. (1996). Arabidopsis AUX1 gene: a permease-like regulator of root gravitropism. Science 273, 948–950. doi: 10.1126/science.273.5277.948
Bilsborough, G. D., Runions, A., Barkoulas, M., Jenkins, H. W., Hasson, A., Galinha, C., et al. (2011). Model for the regulation of Arabidopsis thaliana leaf margin development. Proc. Natl. Acad. Sci. U.S.A. 108, 3424–3429. doi: 10.1073/pnas.1015162108
Blein, T., Pulido, A., Vialette-Guiraud, A., Nikovics, K., Morin, H., Hay, A., et al. (2008). A conserved molecular framework for compound leaf development. Science 322, 1835–1839. doi: 10.1126/science.1166168
Bremer, B., Bremer, K., Chase, M. W., Fay, M. F., Reveal, J. L., Soltis, D. E., et al. (2009). An update of the Angiosperm Phylogeny Group classification for the orders and families of flowering plants: APG III. Bot. J. Linn. Soc. 161, 105–121. doi: 10.1016/j.jep.2015.05.035
Cheng, X., Peng, J., Ma, J., Tang, Y., Chen, R., Mysore, K. S., et al. (2012). NO APICAL MERISTEM (MtNAM) regulates floral organ identity and lateral organ separation in Medicago truncatula. New Phytol. 195, 71–84. doi: 10.1111/j.1469-8137.2012.04147.x
Clough, S. J., and Bent, A. F. (1998). Floral dip: a simplified method for Agrobacterium-mediated transformation of Arabidopsis thaliana. Plant J. 16, 735–743. doi: 10.1046/j.1365-313x.1998.00343.x
Cosson, V., Eschstruth, A., and Ratet, P. (2015). Medicago truncatula transformation using leaf explants. Methods Mol. Biol. 1223, 43–56. doi: 10.1007/978-1-4939-1695-5_4
Endress, P. K., and Igersheim, A. (2000). Gynoecium structure and evolution in basal angiosperms. Int. J. Plant Sci. 161, S211–S223. doi: 10.1086/317572
Galbiati, F., Sinha Roy, D., Simonini, S., Cucinotta, M., Ceccato, L., Cuesta, C., et al. (2013). An integrative model of the control of ovule primordia formation. Plant J. 76, 446–455. doi: 10.1111/tpj.12309
Goncalves, B., Hasson, A., Belcram, K., Cortizo, M., Morin, H., Nikovics, K., et al. (2015). A conserved role for CUP-SHAPED COTYLEDON genes during ovule development. Plant J. 83, 732–742. doi: 10.1111/tpj.12923
Hellens, R., Mullineaux, P., and Klee, H. (2000). Technical focus: a guide to Agrobacterium binary Ti vectors. Trends Plant Sci. 5, 446–451. doi: 10.1016/S1360-1385(00)01740-4
Ishida, T., Aida, M., Takada, S., and Tasaka, M. (2000). Involvement of CUP-SHAPED COTYLEDON genes in gynoecium and ovule development in Arabidopsis thaliana. Plant Cell Physiol. 41, 60–67. doi: 10.1093/pcp/41.1.60
Kamiuchi, Y., Yamamoto, K., Furutani, M., Tasaka, M., and Aida, M. (2014). The CUC1 and CUC2 genes promote carpel margin meristem formation during Arabidopsis gynoecium development. Front. Plant Sci. 5:165. doi: 10.3389/fpls.2014.00165
Kirsch, R. D., and Joly, E. (1998). An improved PCR-mutagenesis strategy for two-site mutagenesis or sequence swapping between related genes. Nucleic Acids Res. 26, 1848–1850. doi: 10.1093/nar/26.7.1848
Koncz, C., and Schell, J. (1986). The promoter of TL-DNA Gene 5 controls the tissue-specific expression of chimeric genes carried by a novel type of Agrobacterium binary vector. Mol. Gen. Genet. 204, 383–396. doi: 10.1007/BF00331014
Larsson, E., Sundstrom, J. F., Sitbon, F., and von Arnold, S. (2012). Expression of PaNAC01, a Picea abies CUP-SHAPED COTYLEDON orthologue, is regulated by polar auxin transport and associated with differentiation of the shoot apical meristem and formation of separated cotyledons. Ann. Bot. 110, 923–934. doi: 10.1093/aob/mcs151
Mallory, A. C., Dugas, D. V., Bartel, D. P., and Bartel, B. (2004). MicroRNA regulation of NAC-domain targets is required for proper formation and separation of adjacent embryonic, vegetative, and floral organs. Curr. Biol. 14, 1035–1046. doi: 10.1016/j.cub.2004.06.022
Nahar, M. A.-U., Ishida, T., Smyth, D. R., Tasaka, M., and Aida, M. (2012). Interactions of CUP-SHAPED COTYLEDON and SPATULA genes control carpel margin development in Arabidopsis thaliana. Plant Cell Physiol. 53, 1134–1143. doi: 10.1093/pcp/pcs057
Nam, Y.-W., Penmetsa, R. V., Endre, G., Uribe, P., Kim, D., and Cook, D. R. (1999). Construction of a bacterial artificial chromosome library of Medicago truncatula and identification of clones containing ethylene-response genes. Theor. Appl. Genet. 98, 638–646. doi: 10.1007/s001220051115
Nikovics, K., Blein, T., Peaucelle, A., Ishida, T., Morin, H., Aida, M., et al. (2006). The balance between the MIR164A and CUC2 genes controls leaf margin serration in Arabidopsis. Plant Cell 18, 2929–2945. doi: 10.1105/tpc.106.045617
Paulino, J. V., Prenner, G., Mansano, V. F., and Teixeira, S. P. (2014). Comparative development of rare cases of a polycarpellate gynoecium in an otherwise monocarpellate family, Leguminosae. A. J. Bot. 101, 572–586. doi: 10.3732/ajb.1300355
Peaucelle, A., Morin, H., Traas, J., and Laufs, P. (2007). Plants expressing a miR164-resistant CUC2 gene reveal the importance of post-meristematic maintenance of phyllotaxy in Arabidopsis. Development 134, 1045–1050. doi: 10.1242/dev.02774
Reymond, M. C., Brunoud, G., Chauvet, A., Martinez-Garcia, J. F., Martin-Magniette, M.-L., Moneger, F., et al. (2012). A light-regulated genetic module was recruited to carpel development in Arabidopsis following a structural change to SPATULA. Plant Cell 24, 2812–2825. doi: 10.1105/tpc.112.097915
Schuster, C., Gaillochet, C., and Lohmann, J. (2015). Arabidopsis HECATE genes function in phytohormone control during gynoecium development. Development 142, 3343–3350. doi: 10.1242/dev.120444
Sieber, P., Wellmer, F., Gheyselinck, J., Riechmann, J. L., and Meyerowitz, E. M. (2007). Redundancy and specialization among plant microRNAs: role of the MIR164 family in developmental robustness. Development 134, 1051–1060. doi: 10.1242/dev.02817
Smyth, D., Bowman, J., and Meyerowitz, E. (1990). Early flower development in Arabidopsis. Plant Cell 2, 755–767. doi: 10.2307/3869174
Souer, E., vanHouwelingen, A., Kloos, D., Mol, J., and Koes, R. (1996). The NO APICAL MERISTEM gene of petunia is required for pattern formation in embryos and flowers and is expressed at meristem and primordia boundaries. Cell 85, 159–170. doi: 10.1016/S0092-8674(00)81093-4
Takada, S., Hibara, K., Ishida, T., and Tasaka, M. (2001). The CUP-SHAPED COTYLEDON1 gene of Arabidopsis regulates shoot apical meristem formation. Development 128, 1127–1135.
Tucker, S. C., and Kantz, K. E. (2001). Open carpels with ovules in Fabaceae. Int. J. Plant Sci. 162, 1065–1073. doi: 10.1086/321923
Vialette-Guiraud, A. C. M., Adam, H., Finet, C., Jasinski, S., Jouannic, S., and Scutt, C. P. (2011a). Insights from ANA-grade angiosperms into the early evolution of CUP-SHAPED COTYLEDON genes. Ann. Bot. 107, 1511–1519. doi: 10.1093/aob/mcr024
Vialette-Guiraud, A. C. M., Alaux, M., Legeai, F., Finet, C., Chambrier, P., Brown, S. C., et al. (2011b). Cabomba as a model for studies of early angiosperm evolution. Ann. Bot. 108, 589–598. doi: 10.1093/aob/mcr088
Wang, H., Moore, M. J., Soltis, P. S., Bell, C. D., Brockington, S. F., Alexandre, R., et al. (2009). Rosid radiation and the rapid rise of angiosperm-dominated forests. Proc. Natl. Acad. Sci. U.S.A. 106, 3853–3858. doi: 10.1073/pnas.0813376106
Keywords: Arabidopsis thaliana, Medicago truncatula, CUP SHAPED COTYLEDON, NO APICAL MERISTEM, miR164, gynoecium, carpel, syncarpy
Citation: Vialette-Guiraud ACM, Chauvet A, Gutierrez-Mazariegos J, Eschstruth A, Ratet P and Scutt CP (2016) A Conserved Role for the NAM/miR164 Developmental Module Reveals a Common Mechanism Underlying Carpel Margin Fusion in Monocarpous and Syncarpous Eurosids. Front. Plant Sci. 6:1239. doi: 10.3389/fpls.2015.01239
Received: 03 October 2015; Accepted: 20 December 2015;
Published: 13 January 2016.
Edited by:
Rainer Melzer, University College Dublin, IrelandReviewed by:
Stefan De Folter, Centro de Investigación y de Estudios Avanzados del Instituto Politécnico Nacional, MexicoBarbara Ambrose, The New York Botanical Garden, USA
Copyright © 2016 Vialette-Guiraud, Chauvet, Gutierrez-Mazariegos, Eschstruth, Ratet and Scutt. This is an open-access article distributed under the terms of the Creative Commons Attribution License (CC BY). The use, distribution or reproduction in other forums is permitted, provided the original author(s) or licensor are credited and that the original publication in this journal is cited, in accordance with accepted academic practice. No use, distribution or reproduction is permitted which does not comply with these terms.
*Correspondence: Charles P. Scutt, Y2hhcmxpZS5zY3V0dEBlbnMtbHlvbi5mcg==