- 1Key Laboratory of Cotton and Rapeseed, Ministry of Agriculture, Institute of Industrial Crops, Jiangsu Academy of Agricultural Sciences, Nanjing, China
- 2Jiangsu Collaborative Innovation Center for Modern Crop Production, Nanjing, China
- 3Provincial Key Laboratory of Agrobiology, Jiangsu Academy of Agricultural Sciences, Nanjing, China
The apetalous genotype is a morphological ideotype for increasing seed yield and should be of considerable agricultural use; however, only a few studies have focused on the genetic control of this trait in Brassica napus. In the present study, a recombinant inbred line, the AH population, containing 189 individuals was derived from a cross between an apetalous line ‘APL01’ and a normally petalled variety ‘Holly’. The Brassica 60 K Infinium BeadChip Array harboring 52,157 single nucleotide polymorphism (SNP) markers was used to genotype the AH individuals. A high-density genetic linkage map was constructed based on 2,755 bins involving 11,458 SNPs and 57 simple sequence repeats, and was used to identify loci associated with petalous degree (PDgr). The linkage map covered 2,027.53 cM, with an average marker interval of 0.72 cM. The AH map had good collinearity with the B. napus reference genome, indicating its high quality and accuracy. After phenotypic analyses across five different experiments, a total of 19 identified quantitative trait loci (QTLs) distributed across chromosomes A3, A5, A6, A9 and C8 were obtained, and these QTLs were further integrated into nine consensus QTLs by a meta-analysis. Interestingly, the major QTL qPD.C8-2 was consistently detected in all five experiments, and qPD.A9-2 and qPD.C8-3 were stably expressed in four experiments. Comparative mapping between the AH map and the B. napus reference genome suggested that there were 328 genes underlying the confidence intervals of the three steady QTLs. Based on the Gene Ontology assignments of 52 genes to the regulation of floral development in published studies, 146 genes were considered as potential candidate genes for PDgr. The current study carried out a QTL analysis for PDgr using a high-density SNP map in B. napus, providing novel targets for improving seed yield. These results advanced our understanding of the genetic control of PDgr regulation in B. napus.
Introduction
Oilseed rape (Brassica napus L., AACC, 2n = 38) is a widely planted oil crop worldwide. Rapeseed oil is not only a desirable edible oil, but is also used as a biofuel in many parts of the world (Hoekman, 2007). Additionally, oil-extracted meal from Brassica seeds is an excellent protein source for animal feed (Linnemann and Dijkstra, 2002). As the global demand for rapeseed products is continuously increasing, developing a high-yield variety is a main goal of B. napus breeding programs. An effective approach is to seek the morphological ideotype (Virk et al., 2004), and apetalous genotypes are of particular interest in breeding programs (Habekotté, 1997; Jiang and Becker, 2003).
The apetalous trait was first reported in a naturally occurring mutant of turnip (B. campestris L.) in Brassica (Ramanujam, 1940), and Buzza (1983) first detected an apetalous mutant in a spring oilseed rape. Since then, other apetalous flowers in Brassica species have been discovered or bred. There are several advantages to apetalous rape in yield. First and foremost, photosynthesis in cultivars without petals is more efficient, with the thick and brightly colored flowers preventing Brassica oilseeds from efficiently using solar energy (Jiang, 2007). The petals at the top layer of the normal flower type were reported to reflect or absorb up to 60% of incoming radiation (Mendham et al., 1981). Additionally, the apetalous cultivars have higher yield potentials than the normal type. The petal is not a photosynthesizing organ, but it consumes considerable amounts of photosynthesized assimilates during its formation and respiration (Jiang, 2007). Mendham et al. (1991) revealed that the yield of apetalous lines was higher than normal petaled cultivars. Finally, the apetalous type of rapeseeds might have a lower rate of infection from diseases distributed by petals, such as Sclerotinia sclerotiorum. Deciduous petals can transmit the Sclerotinia pathogen to healthy tissue, whereas the ascospores that land directly on the leaf surface do not germinate (Jamaux and Spire, 1999). Compared with normal petaled controls, apetalous genotypes have a much lower incidence and severity of Sclerotinia infection (Lefol and Morrall, 1996; Zhao and Wang, 2004). Moreover, a multitude of other diseases, such as Botrytis cinierea and Peronospora parasitica, may be distributed by petals (Lefol and Morrall, 1996). In summary, genotypes with apetalous flowers are a component of the high-yielding ideotype.
In B. napus, various genetic models of the apetalous trait, with different origins, are documented in many literatures. One study found that petalous flower development was controlled by one gene locus that exhibited incomplete dominance over apetalous flower development (Zhao and Wang, 2004). Most of the other studies revealed that the apetalous character in B. napus was regulated by recessive genes, possibly by two to four loci (Buzza, 1983; Lu and Fu, 1990; Kelly et al., 1995; Chen et al., 2006a; Zhang et al., 2007b,c). Generally, these loci independently control flower morphology; however, epistatic interactions between recessive alleles were also identified (Fray et al., 1997). In addition, the apetalous character is governed by the interaction of cytoplasmic and nuclear genes (Jiang and Becker, 2003). Although much attention has been paid to the inheritance of the apetalous character in B. napus, questions concerning the genetic basis remain open. Plants having less than a 10% petalous degree (PDgr) were considered apetalous (Buzza, 1983), but the distribution of PDgr in segregation generation is consecutive and should be treated as a quantitative trait (Zhang et al., 2007a). Quantitative trait loci (QTLs) mapping is a preliminary step and an effective approach to unravel the genetic architecture of complex quantitative traits and to identify QTLs for knowledge-based breeding (Mauricio, 2001). Fray et al. (1997) reported that five restriction fragment length polymorphism markers were significantly associated with one of two stamenoid petal loci, one each on A4 and C4. One random amplified polymorphic DNA marker that was tightly linked to a petal-controlled gene in B. napus was identified by Tan et al. (2003). Using a bulked segregant analysis approach, one sequence-related amplified polymorphism and one amplified fragment length polymorphism marker mapped on A4 were found to be linked to the gene controlling the petal-loss trait (Chen et al., 2006b). Based on a genetic map containing 219 markers, Zhang et al. (2007a) identified four QTLs, which were located on chromosomes A5, A6, A8, and C5, associated with the apetalous phenotype.
High-density maps could increase the precision of QTL localization and the estimation of QTL effects in biparental populations (Stange et al., 2013). Since the first molecular linkage map in B. napus was reported by Landry et al. (1991), various types of populations have been constructed for mapping QTLs associated with seed oil content, seed fatty acid concentrations, flowering time, seed yield and yield-related traits (Qiu et al., 2006; Quijada et al., 2006; Udall et al., 2006; Long et al., 2007; Basunanda et al., 2010; Chen et al., 2010; Cai et al., 2012; Ding et al., 2012; Wang et al., 2013; Wang et al., 2015a). However, most of these genetic linkage maps were constructed based on PCR markers with low densities. Single nucleotide polymorphisms (SNPs) are the most frequent polymorphism in the genomes of crops (Vignal et al., 2002), and they have been widely used in rice (Huang et al., 2010), wheat (Mochida et al., 2003), and maize (Ching et al., 2002). In B. napus, SNPs were also used for high-density genetic map construction and the fine mapping of important genes. Delourme et al. (2013) developed an integrated genetic map, which was comprised of 5,764 SNPs and 1,603 PCR markers, with a genetic length of 2,250 cM. Based on the 6 K SNP array harboring 5,306 probes for B. napus, Raman et al. (2014) constructed a genetic linkage map covered 2,514.8 cM, including 613 SNPs and 228 non-SNPs, and Cai et al. (2014) constructed a genetic map containing 2,115 markers (1,667 SNPs and 448 SSRs), with a length of 2,477.4 cM. Chen et al. (2013) constructed a SNP bin map containing 8,780 SNP loci and a presence/absence variation map containing 12,423 dominant loci. In 2012, the Brassica 60 K SNP BeadChip Array comprised of 52,157 SNP loci was produced (Snowdon and Iniguez Luy, 2012; Edwards et al., 2013), which was developed by an international consortium using preferentially single-locus SNPs contributed from genomic and transcriptomic sequencing in genetically diverse Brassica germplasm (Liu et al., 2013). This paved the way for the high-throughput and cost-effective construction of a high-density genetic B. napus map. Using the 60 K SNP BeadChip Array, Liu et al. (2013) constructed a linkage map containing 9,164 SNP markers covering 1,832.9 cM, and mapped the major QTL for seed color corresponding to a physical region of 620 kbp. Zhang et al. (2014) constructed a map covering a length of 2,139.5 cM with average distance of 1.6 cM between adjacent markers. Nevertheless, to the best of our knowledge, a QTL analysis for the apetalous trait using high-density SNP genetic linkage map in B. napus has not been performed.
The objectives of the present study were to: (1) construct a high-density genetic map using the Brassica 60 K Infinium SNP array and SSRs; and (2) investigate the QTLs for PDgr in B. napus across five experiments. The results will provide information useful for understanding the genetic control of apetalous in B. napus, and the major QTLs will lay a foundation for use in breeding programs to develop a variety with agronomic traits of interest for rapeseed production.
Materials and Methods
Plant Materials
The B. napus segregating recombinant inbred line (RIL) population used in this study was derived from a cross between ‘APL01’ and ‘Holly’ using the single seed descent method. The parent ‘APL01’ is an apetalous line, developed at the Institute of Industrial Crops, Jiangsu Academy of Agricultural Sciences, Nanjing, China. ‘APL01’ was selected from F6 generation of crosses between apetalous (Apeatlous No. 1) and normal petalous (Zhongshuang No. 4) rapeseed in 1998 (Zhang et al., 2002). ‘Apeatlous No. 1’ was bred from F8 generation of crosses between China rapeseed cultivar with smaller petals (SP103) and B. rapa variety with lower petals (LP153). ‘Zhongshuang No. 4’ was developed at Oil Crops Research Institute of the Chinese Academy of Agricultural Sciences, Wuhan, China. Except for PDgr, other agronomic traits in ‘APL01’ are quite normal. At early flowering stage, ‘APL01’ is absolutely apetalous, however, there may be one cripple petal only in a few flowers at late flowering stage. The genotype ‘Holly’ is a normally and completely petaled variety. The two parents showed the similar flowering time, which recorded from the sowing day to the day when the first flower had opened on half of the plants in the plot. A total of 550 F9 RIL lines were developed in 2014, and then a subset of 189 lines was randomly selected to compose the mapping population for the genetic linkage map construction. These were named the AH RIL population.
Field Trials and PDgr Measurements
The AH population, together with the two parents, was tested in five experiments. The materials were planted in a winter rapeseed area, Dali of Shaanxi Province (coded DL), in northwest China for one year (September-May of 2014–2015), and a semi-winter rapeseed area, Nanjing of Jiangsu Province (coded NJ), in eastern China for four years (September-May of 2011–2012, 2012–2013, 2013–2014, and 2014–2015). Year-location combinations were treated as experiments, for example, 14NJ indicates the experiment was conducted during 2014–2015 at the Nanjing location. The field experiments were conducted in a randomized complete block design with two replications in both NJ and DL. The experimental unit was a two-row plot with 20 plants per row and 40 cm between the rows. The field management followed the common agricultural practices.
At least five representational plants of each plot were selected to measure PDgr during the flowering stage. The percentage of petals of an individual plant was determined by counting the number of petals on the first 25 flowers to open (Buzza, 1983). The PDgr was calculated by the following formula as described by Buzza (1983):
in which P represents the number of petals for each flower that was counted, with a range of numerical value of 0–4, and n is the total number of flowers we investigated, n ≥ 50.The average of the PDgr in each RIL line was used as raw data in the analysis.
Statistical Analysis
Basic statistical analyses of PDgr were performed using SPSS 18.0 software (SPSS Inc., Chicago, IL, USA). The software package SEA-G3DH, with the mixed major gene and polygene inheritance model, was used to analyze the inheritance of the PDgr character in the AH population (Cao et al., 2013). The best fitting model was selected from 39 different models that were included in the software package according to Wang et al. (2015b). Because the PDgr was expressed as a percentage and did not fit the normal distribution model, all data were subjected to an arcsine transformation for the genetic model analysis. Genetic parameters were estimated using the best fitting model with the default settings in the software.
SNP and SSR Marker Analysis
The genotypes of the AH RIL population and two parental lines were analyzed using the Brassica 60 K SNP BeadChip Array, which successfully assays 52,157 Infinium Type II SNP loci in B. napus. This array was developed by the international Brassica SNP consortium in cooperation with Illumina Inc. San Diego, CA, USA. DNA sample preparation, hybridization to the BeadChip, washing, primer extension and staining were strictly carried out according to the Infinium HD Assay Ultra manual. Imaging of the arrays was performed using an Illumina HiSCAN scanner. Allele calling for each locus was performed using the GenomeStudio genotyping software v2011 (Illumina, Inc.). SNP markers used the names that were assigned by GenomeStudio, such as “Bn-A01-p25032772”. SSR primer pairs prefixed “CB” and “BRAS” were published by Piquemal et al. (2005); “Na”, “Ol” and “Ra” were developed by Lowe et al. (2004); “MR” were published by Uzunova and Ecke (1999) and “BnGMS” were developed by Cheng et al. (2009).
Construction of the Genetic Linkage Map and Alignment of the B. napus Reference Genome
All SNPs that were polymorphic between ‘APL01’ and ‘Holly’, as well as having less than 5% missing data, were used for the genetic linkage map construction. Of the 52,157 SNPs in the array, 17,414 SNPs met the above requirements and were selected for further analysis. SNP marker pairs with no recombination were classified into one genetic bin (one bin corresponded to all of the markers having the same genotype scoring data) using a Perl script. Then, the selected 17,414 SNPs were grouped into 3,422 SNP-bins, containing 1 to 2,079 SNPs in each bin. Combined with 81 polymorphic SSR markers between the two parents, 3,503 loci (3,422 SNP-bins and 81 SSRs) were subsequently applied in map construction using JoinMap software Version 4.0 (Van Ooijen, 2006). Centimorgan (cM) distances were calculated by the Kosambi function for map distance (Kosambi, 1943). Markers with a mean chi-square value ≥ 3.0 were excluded in all genetic groups to ensure the high quality of the map (Wang et al., 2013).
In addition, the probe sequences of the SNPs that assigned the A and C sub-genomes of B. napus, were queried using the BLAST algorithm against the B. napus reference genome sequence to locate chromosomal positions with highly stringent parameters (E value ≤ 1e-10) (Chalhoub et al., 2014). Alignments between the SNP bin map and the B. napus reference genome were used to validate the quality of the genetic map. If a locus was mapped to multiple paralogous positions in the B. napus reference genome, only the location that corresponded to the particular linkage group of the locus was selected for the collinearity analysis.
QTL Detection and Meta-Analysis
The software Windows QTL Cartographer 2.5 with a composite interval mapping model was used to estimate putative QTLs with additive effects (Zeng, 1994; Wang et al., 2007). The walking speed was set to 2 cM, and a window size of 10 cM with five background cofactors was used. The LOD threshold (2.8–3.1) for detection of significant QTLs was set by a 1,000-permutation test based upon a 5% experiment-wise error rate, and these QTLs were termed ‘identified QTLs’. Identified QTLs that were detected in different experiments with overlapping confidence intervals (CIs), may have been one single QTL. Then, identified QTLs were integrated into consensus QTLs using a meta-analysis method with the BioMercator V4.2 program (Arcade et al., 2004). If an identified QTL had no overlapping CIs with others, then it was also regarded as a consensus QTL (Wang et al., 2013).
The identified and consensus QTL nomenclature was based on the descriptions of McCouch et al. (1997) with minor modifications. For identified QTLs, a designation begins with the abbreviation “iq” (identified QTL), follow by the experiment and linkage group (A1–A10, C1–C9). If there was more than one identified QTL obtained in a linkage group, a serial number was added. For example, iq12NJ.A9-2 indicates the second identified QTL for PDgr on A9 in the 12NJ experiment. For consensus QTLs, a designation begins with the abbreviation “qPD” (q, QTL; PD, petalous degree). For example, QTL qPD.A9-2 indicates the second consensus QTL for PDgr on the A9 linkage group. In addition, candidate genes were identified by comparative mapping between the AH map and the B. napus reference genome based on the probe sequence of the SNPs. If the physical positions of aligned genes fell into the CI of a consensus QTL, then the orthologous candidate genes were assumed to be associated with the target QTL (Ding et al., 2012).
Results
Phenotypic Variation and Genetic Analysis for PDgr
‘APL01’ and ‘Holly’ are the two parental lines whose repeatability is very good in the five experiments. The mean values ± SE were 0.02% ± 0.03 and 99.99% ± 0.01 for ‘APL01’ and ‘Holly’, respectively. There was a wide range of variations and consecutive distributions in the AH population (Figure 1), suggesting that PDgr was governed by multiple genes. However, the phenotypic values did not fit the normal distribution in the majority of the RIL lines, mostly petalled, indicating that PDgr might be determined mainly by the major genes in B. napus.
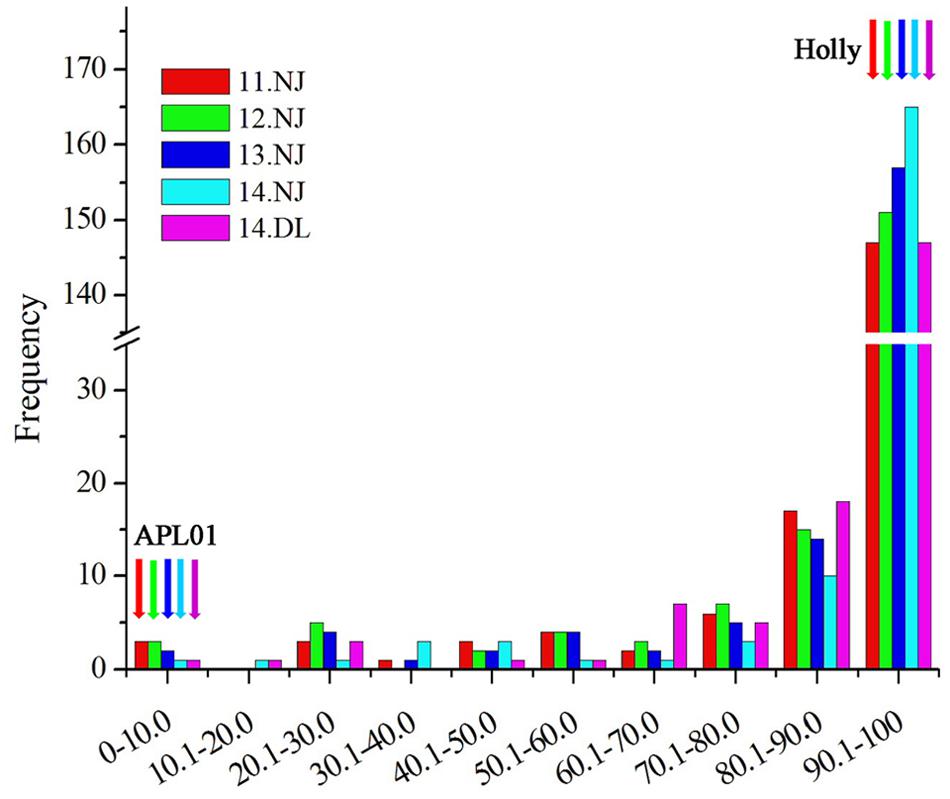
FIGURE 1. Distribution of the petalous degree (PDgr) of the AH RIL population in five experiments. The units of the x-axis are the percentage of the PDgr, and the units of the y-axis are the number of lines. PDgr in different experiments was discriminated using different colored boxes (11NJ, red; 12NJ, green; 13NJ blue; 14NJ, cyan; 14DL, magenta). The color arrows represent the location of the parental lines.
The mixed major gene and polygene inheritance model has been used to analyze the two parents and AH population for PDgr in the 11NJ and 12NJ experiments (Li et al., 2014). The PDgr of the AH population was controlled by the two additive major genes and the additive polygene model (MX2-Additive-A model) (Li et al., 2014). Using the same method, the best fitness genetic models for PDgr in 13NJ, 14NJ, and 14DL were analyzed in the present study. The MX2-Additive-A model was also the best fitness genetic model in the 13NJ and 14NJ experiments. However, the genetic model MX2-EA-A, which mixed two equal additive major genes with the additive polygene model, was the most suitable model for the 14DL experiment. The heritabilities of major genes ranged from 68.52% to 88.01% in the five experiments (Table 1), significantly higher than that of the polygenes (11.99–31.48%), indicating that PDgr in B. napus was determined by the combination of major genes and polygenes, but mainly by the major genes.
High-Density SNP Map Construction
Among the 3,503 loci (3,422 SNP-bins and 81 SSRs) that were used for map construction, 2,755 SNP-bins and 57 SSRs were assigned to 19 linkage groups, including 1,686 loci in the A sub-genome (A1–A10) and 1,126 in C sub-genome (C1–C9; Table 2, Supplementary Table S1). The number of SNP markers varied considerably across the different bins, ranging from 1 to 317, and 11,458 SNPs involved in the 2,812 loci were assigned to the genetic map (Table 2; Figure 2). A8 has the lowest number of SNPs with only 139 SNPs spanning 76.77 cM, and C2 has the most SNPs with 1,788 SNPs spanning 112.08 cM (Figure 2).
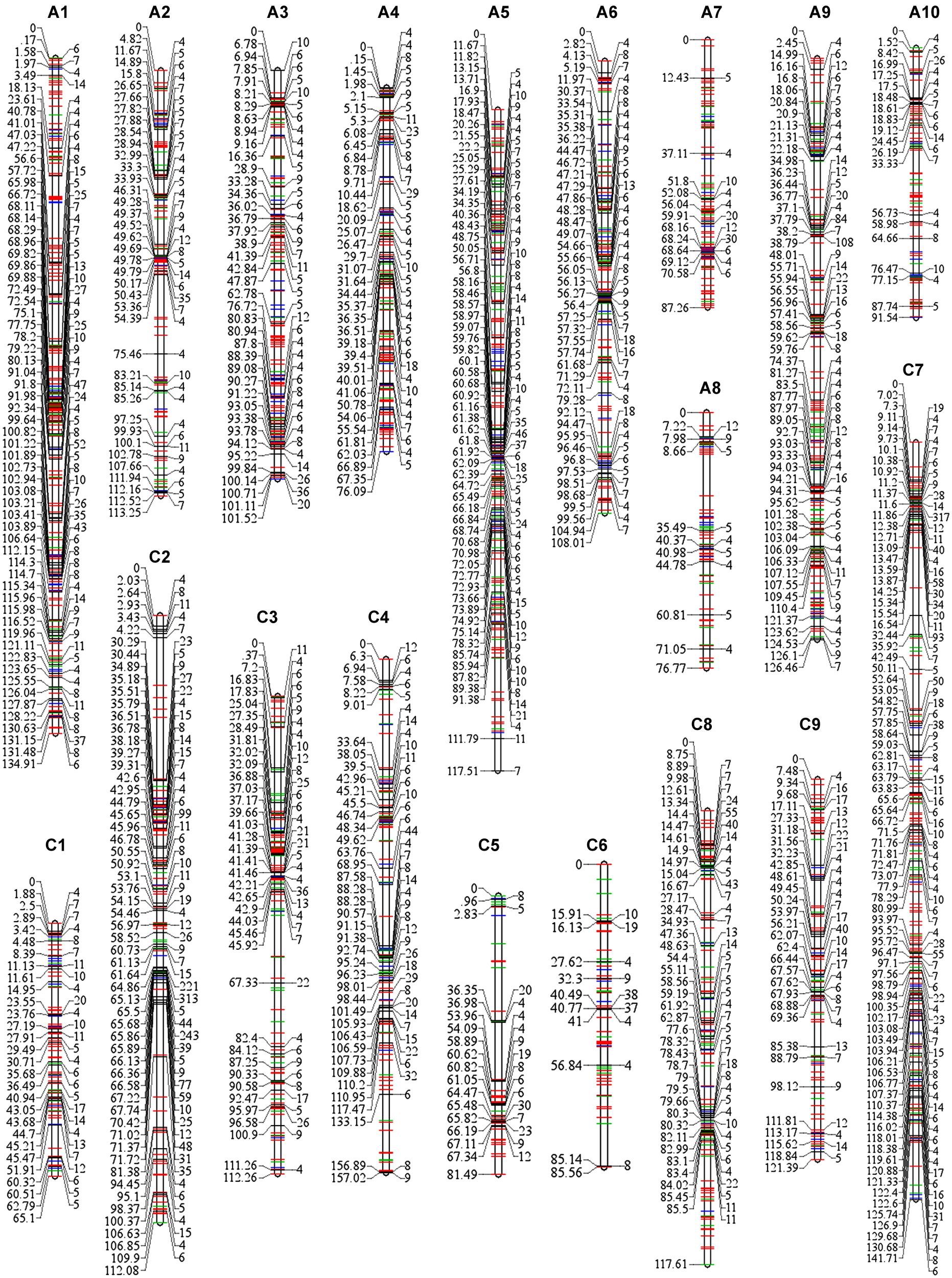
FIGURE 2. SNPs distribution in each linkage group of the map constructed using 189 RIL individuals. The 19 linkage groups are represented by vertical bars, designated as A1–A10 in the A sub-genome and C1–C9 in the C sub-genome. The number of SNPs in each bin is listed on the right side of the linkage groups, while the positions of the bins are shown on the left side of the linkage groups. Bins with less than three SNPs were represented by colored lines (red lines, bins involve one SNP; green lines, two SNPs; and blue lines, three SNPs), for simplicity the numbers and positions are not shown. Full details are provided in Supplementary Table S1.
The high-density map had a total length of 2,027.53 cM with an average marker interval of 0.72 cM, covering 1,033.31 and 994.22 cM of the A and C sub-genomes, respectively (Table 2). The average linkage group lengths of the A and C sub-genomes were similar at 103.3 and 110.5 cM, respectively. However, the lengths of each group showed great differences, ranging from 76.09 (A4) to 134.91 cM (A1) in the A sub-genome and 65.10 (C1) to 157.02 cM (C4) in the C sub-genome. In addition, no chromosome in the genetic map displayed gaps of more than 20 cM, while C5 showed the largest gap of 16.70 cM between Bn-scaff_16082_1-p33791 and Bn-scaff_15712_10-p52253 (Supplementary Table S1).
Alignment of the SNP Linkage Map to the B. napus Reference Genome
The probe sequences of all 2,755 SNP-bins that mapped to the 19 linkage groups were aligned to the B. napus reference genome to validate the genetic linkage maps (Figure 3). The results showed that 2,350 loci produced successful BLAST hits in the B. napus database, accounting for 85.30% of the 2,755 SNP-bins (85.63% for the A sub-genome and 84.80% for the C sub-genome).
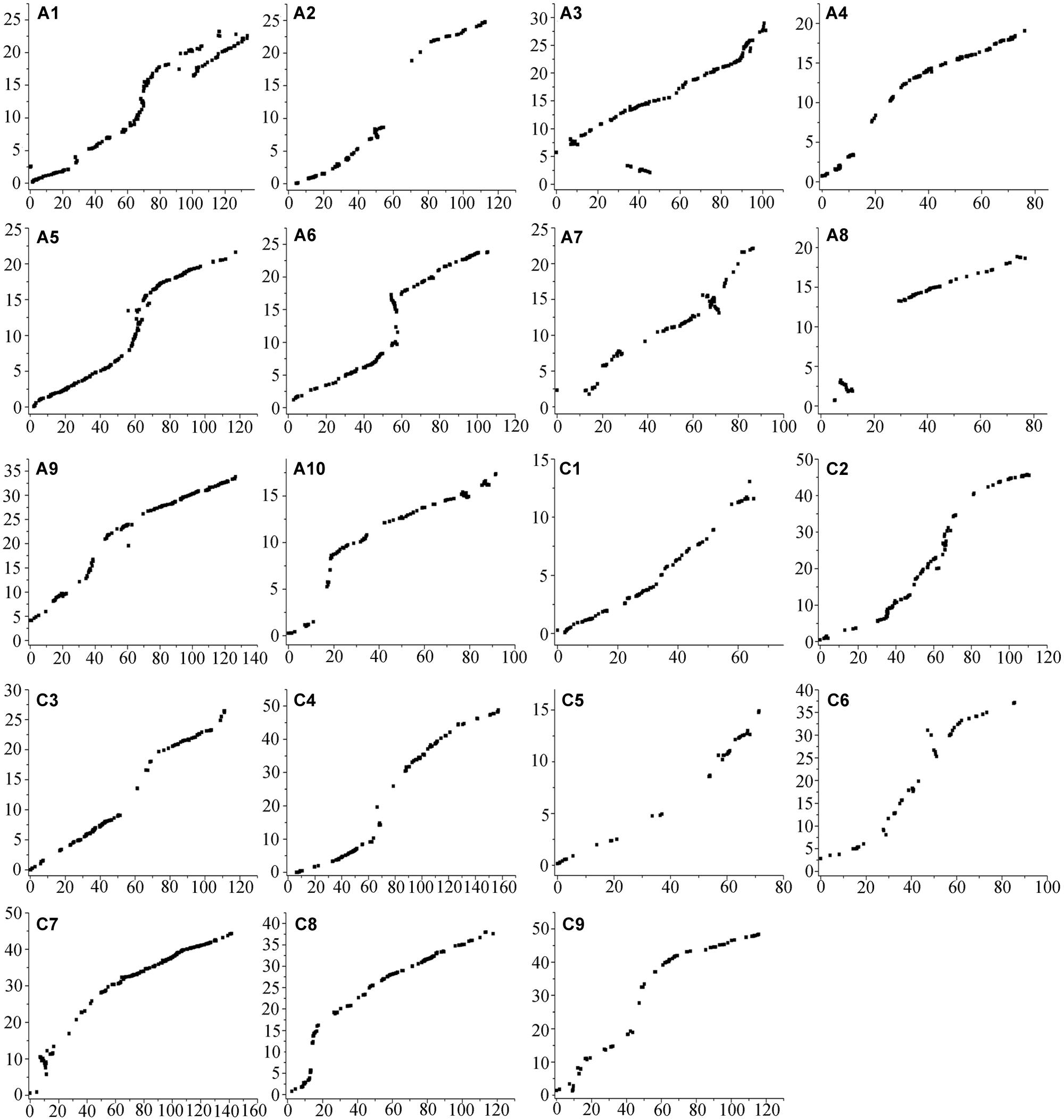
FIGURE 3. Alignments between the SNP linkage map and the Brassica napus reference genome sequence. The x-axis represents the genetic distance in centimorgan (cM) of each linkage group in the AH map constructed in the present study. The units of the y-axis are the physical distances (Mb) based on the B. napus reference genome sequence.
Alignments indicated that the linkage map constructed in the present study had good collinearity with the B. napus reference genome sequence (Figure 3), suggesting the high quality of the AH RIL map. However, several inconsistencies on A1, A3, A7, and A8 were detected between the map and the B. napus reference genome. For example, a large inconsistency involving 80 SNP-bins and spanning a region from 100.82 to 133.75 cM (16.27 Mb of physical region) on A1 might be caused by the existence of paralogous sequences. The inconsistency from the 34.36 to 45.37 cM on the A3 chromosome, which includes 38 SNP-bins and corresponds to ∼1.23 Mb of the physical interval, may have been caused by the presence of partial homologous sequences or fragment duplications. In addition, an inversion including 41 loci and spanning from 64.33 to 71.49 cM (2.49 Mb) was identified on the A7 chromosome, and an inversion with 20 loci from 4.09 to 12.40 cM (2.54 Mb) was also identified on A8.
The total genome size of B. napus is estimated to be 1,130 Mb, and ∼ 645.4 Mb of the genome assembly was collectively comprised by the scaffolds (Chalhoub et al., 2014). The A sub-genome of the AH map showed good coverage of the reference B. napus genome, representing 93.72% of the genome assembly length, while the C sub-genome showed a much lower coverage of 76.91% (Table 2). The main reason was that three linkage groups, C1, C3, and C5, only represent 33.45, 43.70, and 34.17%, respectively, of the corresponding chromosomes (Table 2; Figure 3). The remaining six linkage groups of the C sub-genome account for 92.21to 99.71% of the genome assembly length.
QTL Detection and Meta-Analysis for PDgr
Phenotypic data of PDgr in the AH population were obtained from the five different environments. QTLs for PDgr were analyzed based on the high-density SNP map, and then identified QTLs were integrated into consensus QTLs. Detailed information on identified and consensus QTLs are summarized in Table 3.
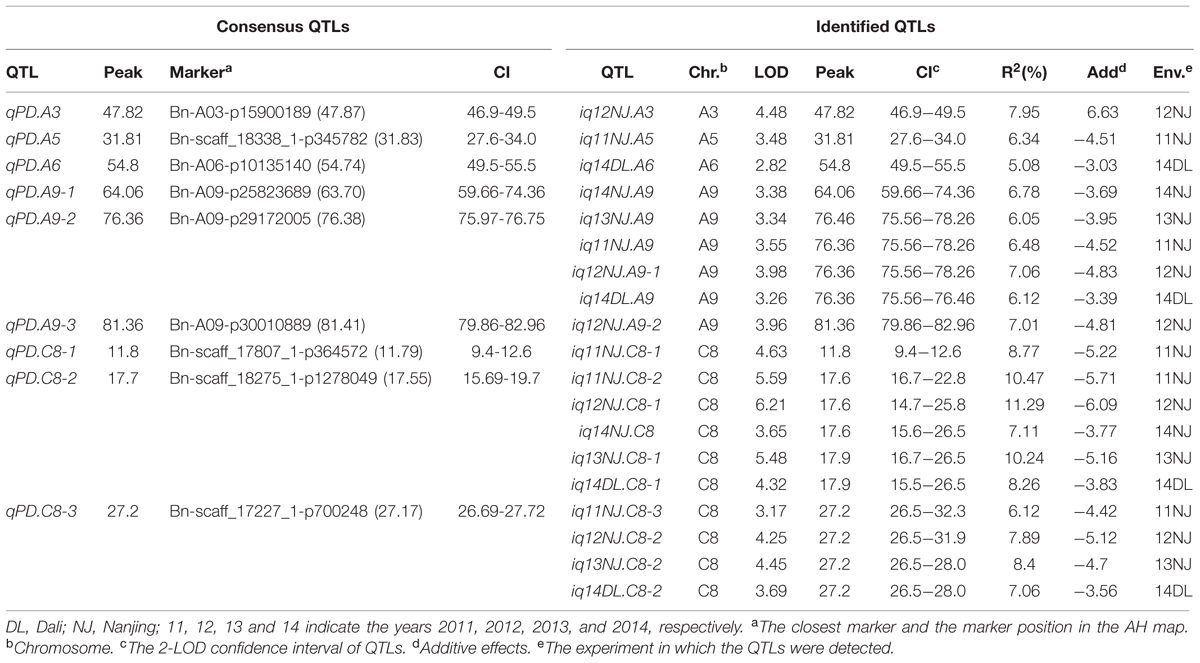
TABLE 3. A list of nine consensus QTLs for petalous degree obtained after the meta-analysis of 19 identified QTLs in five environments.
A total of 19 identified QTLs distributed across A3 (1 QTL), A5 (1 QTL), A6 (1 QTL), A9 (6 QTLs), and C8 (10 QTLs) chromosomes were detected in the present study (Table 3). These QTLs have additive effects ranging from -6.09 to 6.63, and singly explaining 5.08%–11.29% of the estimated phenotypic variation (PV). The number of identified QTLs detected in different experiments was quite different, ranging from two (14NJ) to five (11NJ). Among them, up to 10 identified QTLs were distributed on C8, implying that the major genes for PDgr might exist on the C8 chromosome. Eighteen of the 19 identified QTLs had negative additive effects, suggesting that the normally petalled parent ‘Holly’ contributed favorable alleles for increasing PDgr. Only one identified QTL, iq12NJ.A3, had the positive additive effect of 6.63, indicating that the positive alleles for higher phenotypic values were inherited from the apetalous parent ‘APL01’.
There were 13 identified QTLs with overlapping CIs, and these were further integrated into three consensus QTLs using a meta-analysis method (Table 3; Figure 4). As a result, the average CIs of these QTLs were reduced from 5.55 to 1.94 cM, which significantly increased the accuracy of the estimated positions of the meta-QTL. The other six non-overlapping QTLs were also considered as consensus QTLs. In total, nine consensus QTLs for PDgr were obtained in the present study. Among these consensus QTLs, one QTL (qPD.C8-2) with the closely linked marker Bn-scaff_18275_1-p1278049 of 0.15 cM, was consistently detected in all of the five experiments and had additive values in the range of –0.69 to –3.77 (Table 3). According to the description of Shi et al. (2009), if a consensus QTL presents at least once with PV ≥ 20% or at least twice with PV ≥ 10%, then the QTL can be regarded as a major QTL. The QTL qPD.C8-2 with PV ≥ 10% in 11NJ, 12NJ and 13NJ (10.47, 11.29, and 10.24%, respectively) was the major QTL, which might harbor major genes responsible for PDgr. In addition, two QTLs, qPD.A9-2 and qPD.C8-3, were expressed steadily in four experiments, with linked markers of Bn-A09-p29172005 and Bn-scaff_17227_1-p700248 and explained 6.05%–7.06% and 6.12%–8.40% of PV, respectively. The genes associated with these QTLs controlling PDgr may be less affected by environment, which is consistent with the results of the genetic analysis, which indicated that PDgr in the AH population has a high heritability (Table 1). A striking finding was that, except for the three abovementioned QTLs, no other QTL was detected in multiple experiments, and the remaining six QTLs were environment-specific QTLs detected only in one environment (Table 3).
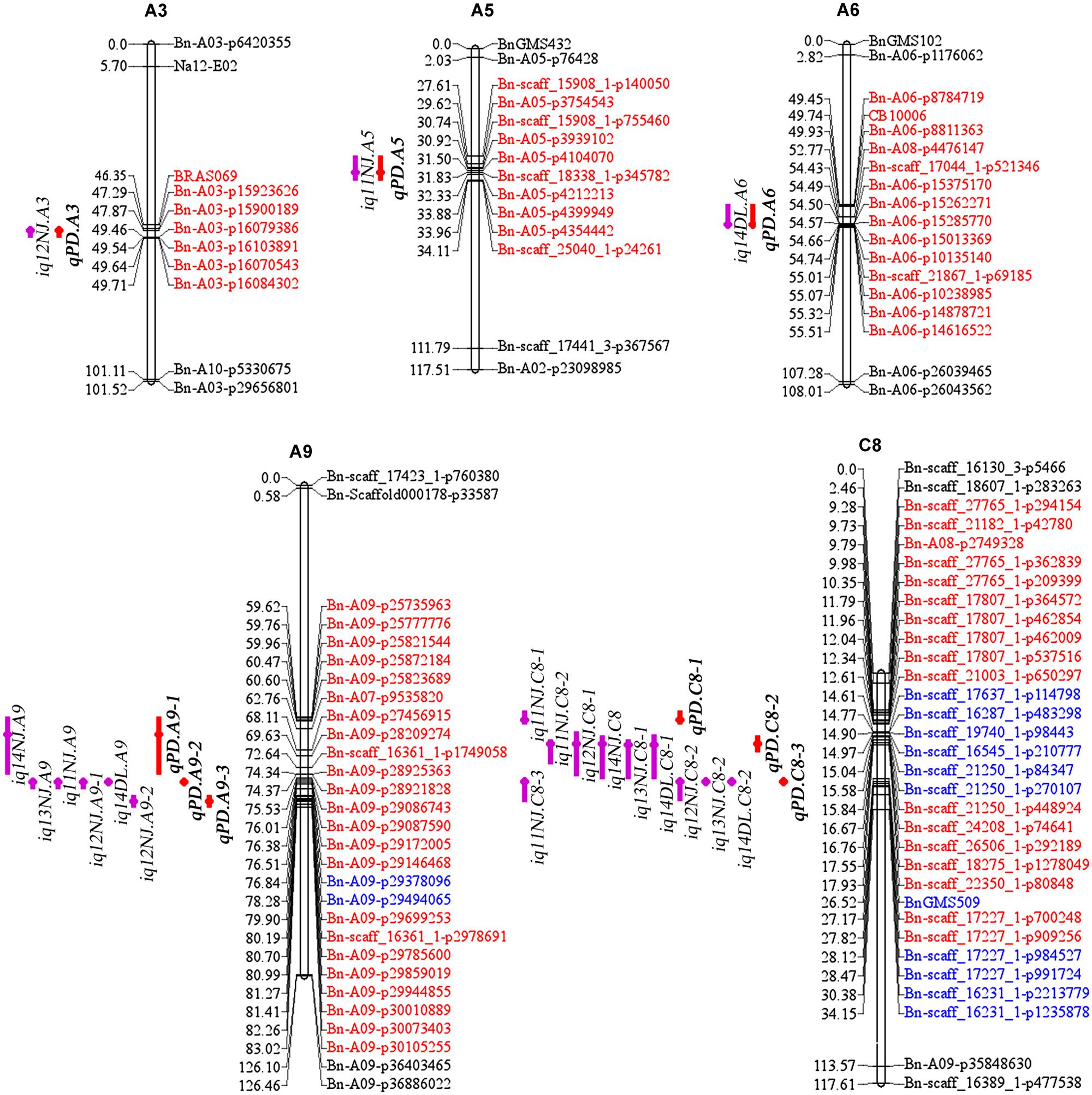
FIGURE 4. The locations of identified and consensus QTLs associated with PDgr in the AH map. The linkage groups are represented by vertical bars. The loci names are listed on the right side of the linkage groups, while the loci positions are shown on the left side of the linkage groups. For simplicity, only the markers underlying the QTL CIs and the terminal two markers of each linkage group are shown. QTLs for PDgr are represented by bars with different backgrounds on the left side of each linkage group (purple bars, identified QTLs and red bars, consensus QTLs). Red loci are underlying the CIs of consensus QTLs and blue loci are underlying the CIs of identified QTLs but not consensus QTLs.
Identification of Candidate Genes Responsible for PDgr in the AH Population
As mentioned above, qPD.A9-2, qPD.C8-2, and qPD.C8-3 were the three steady QTLs that showed significant effects in four, five, and four environments, respectively (Table 3). The three QTLs span regions of 75.97–76.75 cM, 15.69–19.7 cM, and 26.69–27.72 cM, with physical regions of 0.35, 2.65, and 0.26 Mb on chromosomes A9, C8, and C8, respectively. Based on the comparative mapping between the AH map and the B. napus reference genome, 58, 220, and 50 genes, respectively, were identified underlying the CIs of the three QTLs (Supplementary Table S2A). The other six consensus QTLs were only detected in certain geographical regions, suggesting that they were easily influenced by the environment, and genes underlying these QTLs were not analyzed in the present study.
Fifty-two genes regulating floral development were collected from 55 published studies that have been performed mainly in Arabidopsis (Supplementary Table S2B). These genes were further annotated with gene ontology (GO) terms, which were classified into three categories: biological process (BP, Figure 5A), cellular component (CC, Figure 5B), and molecular function (MF, Figure 5C). The 52 genes were classified into 92 functional groups, with the number of genes in each GO term ranging from 1 to 50 (Supplementary Table S2C). In the BP category, the most abundant GO terms were GO:0006355 (regulation of transcription, DNA-templated) and GO:0006351 (transcription, DNA-templated). In the CC category, GO:0005634 (nucleus) was the most abundant, followed by GO:0005737 (cytoplasm). In the MF category, GO:0005515 (protein binding) and GO:0003700 (sequence-specific DNA binding transcription factor activity) were the major GO terms.
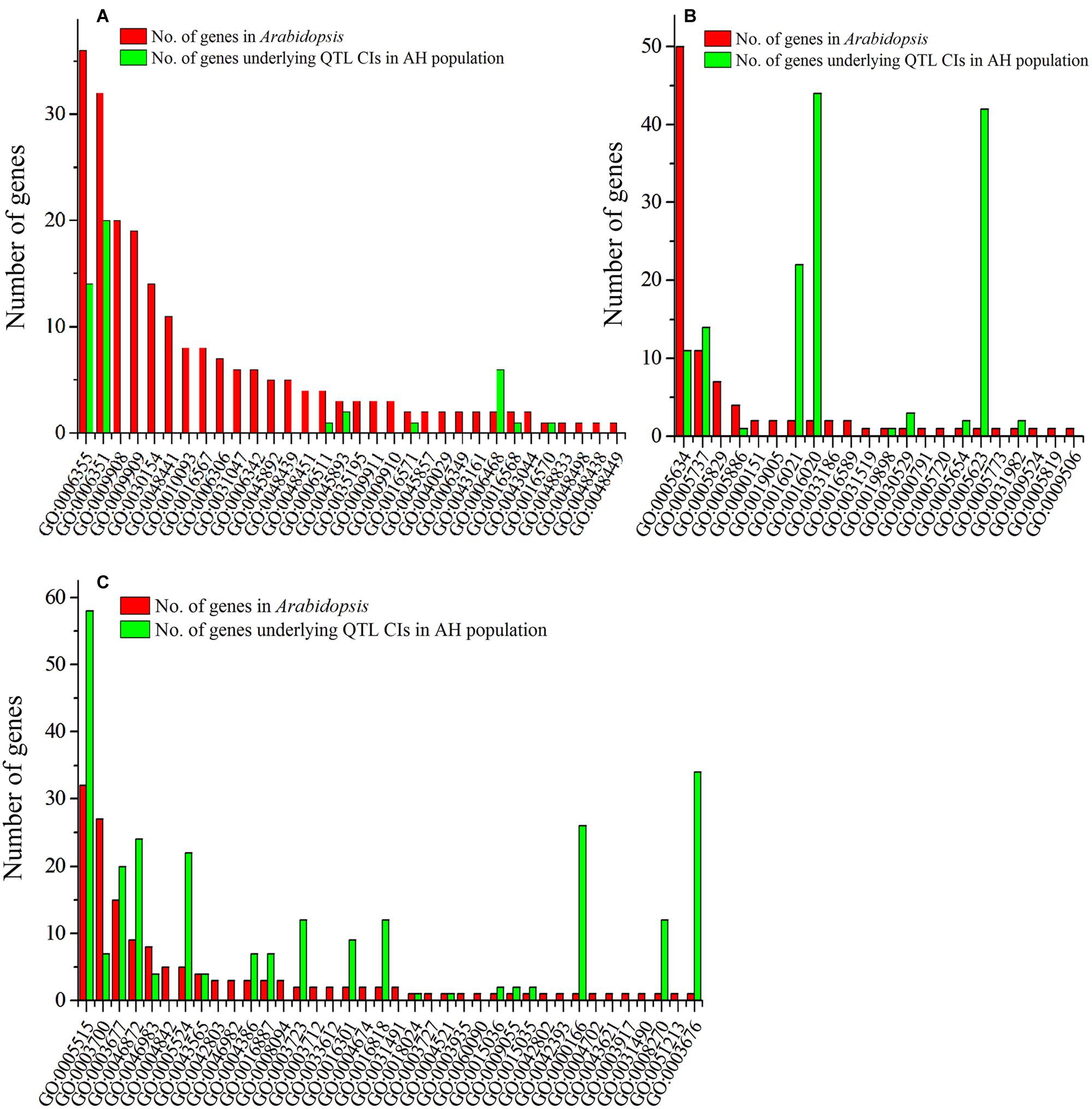
FIGURE 5. Gene ontology (GO) annotation of collected genes regulating petal development and the candidate genes of three steady QTLs. The x-axis represents the functional groups (GO accessions), and the y-axis represents the number of genes in each category. (A) Biological process; (B) cellular component; and (C) molecular function.
Gene ontology assignments were also used to classify the functions of the 328 genes that underlie the three steady QTLs. The results showed that 146 genes were distributed under 38 of the 92 GO terms (Figure 5, Supplementary Table S2C), and thus are potential candidate genes for PDgr in B. napus. Among these, 34 (14 and 20, respectively), 25 (11 and 14, respectively), and 64 (57 and 7, respectively) genes were classified in the top two GO terms in the BP, CC and MF categories to which the published genes for petal development were assigned, respectively (Figure 5, Supplementary Table S2C). Some candidate genes were assigned to more than one category. For example, BnaC08g10960D and BnaC08g14030D, which underlie the CIs of qPD.C8-2 and qPD.C8-3, respectively, were assigned to all three categories. In addition, seven, eight and five candidate genes were simultaneously assigned to BP and CC, BP and MF, CC and MF categories, respectively.
Discussion
The apetalous genotype is a morphological ideotype for increasing seed yield compared with the fully petalled variety in B. napus, which improves light penetration through the floral canopy and lowers the incidence of S. sclerotiorum infection (Mendham et al., 1981, 1991; Jamaux and Spire, 1999; Jiang, 2007). Having suitable parental genotypes is critical for identifying the inheritance and the genetic bases of a character; however, the lack of completely apetalous genetic resources has been a limiting factor for understanding its genetic control in B. napus. Several apetalous flowers in B. napus were used to investigate the genetic models for the apetalous character (Buzza, 1983; Lu and Fu, 1990; Kelly et al., 1995; Zhao and Wang, 2004; Chen et al., 2006a; Zhang et al., 2007b,c). In the present study, an apetalous line ‘APL01’ and a normally petalled variety ‘Holly’ were used to construct the AH RIL population and perform a genetic analysis of the apetalous trait. The results showed that PDgr in B. napus was controlled by the combination of two major genes and polygenes, which was consistent with previous studies (Buzza, 1983; Kelly et al., 1995; Jiang and Becker, 2003; Chen et al., 2006a; Zhang et al., 2007b). Other genetic models for apetalous in B. napus have also been reported, such as the apetalous character being controlled by only one gene locus (Zhao and Wang, 2004), and four pairs of recessive genes (Lu and Fu, 1990; Zhang et al., 2007c). These studies demonstrated that PDgr in B. napus is a quantitative trait.
Quantitative trait loci mapping is an effective approach to dissect the genetic mechanisms of quantitative traits, while high-density map can increase the precision of QTL localization and effects, especially for small and medium sized QTLs (Almeida et al., 2013; Stange et al., 2013). However, linkage mapping studies in B. napus were mostly based on low-density genetic maps constructed using SSR markers, or higher-density maps based on anonymous markers, such as amplified fragment length polymorphisms or sequence-related amplified polymorphisms (Sun et al., 2007). In the present study, a high-density SNP map was constructed with 2,812 loci involving 11,458 SNPs, covering a length of 2,027.53 cM and with an average marker interval of 0.72 cM, suggesting that it might be extremely useful in QTL detection. Compared with the previously published genetic maps constructed using SNPs in B. napus (Liu et al., 2013; Cai et al., 2014; Zhang et al., 2014), the AH map was one of the highest density maps with average distance between loci of less than 1.0 cM. In addition, the genome sequence of B. napus has been released (Chalhoub et al., 2014), which will facilitate the fine mapping of QTLs for quantitative traits and marker-assisted selection breeding in B. napus. A high-density SNP map is beneficial for the accurate alignment of the AH map to the physical chromosome segments in the assembled B. napus genomes. The results showed that the AH SNP map had good collinearity with the B. napus reference genome, indicating the high quality and accuracy of the map (Figure 3). Furthermore, the A sub-genome of the AH map showed a near-complete coverage of the B. napus genome (93.72%), considerably higher than the C sub-genome (76.91%; Table 2). One possible reason for the low coverage of the C sub-genome is that a form of distorted segregation in some chromosomes caused by rearrangements among homologous chromosomes in the parents occurred (Lu et al., 2013), resulting in the low coverage of C1 (33.45%), C3 (43.70%), and C5 (34.17%). The loci density in the A sub-genome (0.61 cM/marker) was also higher than that of the C sub-genome (0.88 cM/marker) in the AH map (Table 2), indicating the higher polymorphism rate in the A sub-genome. In agreement with these findings, Liu et al. (2013) constructed a map including 976 loci in the C genome and 1,819 loci in the A genome, with an average distance between markers of 0.53 cM in the A genome and 0.93 cM in the C genome.
A large population, a high-density genetic map and replicated experiments in multiple environments are three necessary factors for precise QTL detection (Yu et al., 2011; Wang et al., 2013). In the current study, a QTL analysis for PDgr was based on the high-density AH map and phenotypic data from five experiments, and nine consensus QTLs were identified on the A3, A5, A6, A9, and C8 chromosomes. Until now, only a few studies focused on the complex genetic mechanism of PDgr in B. napus. Several molecular markers associated with the petal-loss trait were located on A4 (Fray et al., 1997; Chen et al., 2006b) and C4 (Fray et al., 1997), and four QTLs for PDgr were located on A5, A6, A8, and C5 (Zhang et al., 2007a). These results suggest that the seven QTLs on A3, A9, and C8 in the present study were potential new QTLs, including the major QTL qPD.C8-2 detected in all of the five experiments and two steadily expressed QTLs (qPD.A9-2 and qPD.C8-3) identified in four experiments. The environment-specific QTLs qPD.A5 on A5 and qPD.A6 on A6 were not confirmed due to the lack of common markers between the different populations. The number of QTLs for PDgr in this study may be underestimated because the density of the AH genetic map was not saturated (Table 2), which may result in an incomplete resolution of QTLs. On the other hand, as AH population has been tested for 4 years in NJ, QTLs in DL might be underestimated based on the data of one year, and QTL detection could be improved in multiple experiments in DL location. These findings advanced our understanding of the genetic control of PDgr regulation in B. napus, and the three stable QTLs are helpful for fine mapping and cloning of QTLs, and will enable a marker-accelerated backcrossing programs.
A typical flower consists of four different types of organs arranged in four whorls, with sepals, petals, stamens and carpels in the outermost to innermost whorls. According to the ABC model, combinatorial interactions between the three classes of floral homeotic genes are affected in the four floral organs, with ‘A’, ‘A+B’, ‘B+C’, and ‘C’ specifying sepals, petals, stamens and carpels, respectively (Coen and Meyerowitz, 1991; Honma and Goto, 2001; Theißen and Saedler, 2001). The isolation of novel floral mutants in Arabidopsis and other species has led to an expansion of the ABC model to include the ‘D’ and ‘E’ functions. Thus, the ABCE model was proposed, which established that the organ-specific genes required the activity of SEPALLATA genes (Pinyopich et al., 2003; Krizek and Fletcher, 2005). These genes (termed class E genes), together with the class B and C genes, are required for the specification of organ identity in the petal (‘A+B+E’), stamen (‘B+C+E’), and carpel (‘C+E’) (Theißen and Saedler, 2001). Mutants with defects in the second and third whorls (‘B’ function) result in the homeotic conversion of petals to sepals and stamens to carpels (Causier et al., 2010). However, the apetalous line ‘APL01’ used in the present study had normal sepals, stamens and carpels, suggesting a more complex molecular mechanism of floral development in the allotetraploid species B. napus. In the present study, the genetic basis of floral development in B. napus was analyzed at the QTL level. Three QTLs (qPD.A9-2, qPD.C8-2, and qPD.C8-3) were stable across multiple environments, suggested that the genes controlling PDgr might underlying the CIs of the three QTLs. B. napus has a common ancestor with Arabidopsis, and a high degree of sequence similarities and chromosomal colinearities are expected because of the progenitors diverged about 20 million years ago (Yang et al., 1999; Koch et al., 2000). Generally, the allotetraploid genomes B. napus may typically contain six distinct alleles for each gene present within Arabidopsis (Lysak et al., 2005), with the likelihood that genes carry out the core biological processes will be probable orthologs. Based on the GO assignments, 52 genes regulating of floral development mainly in Arabidopsis were classified into 92 functional groups, and 146 genes underlying the CIs of the three QTLs were distributed under 38 of the 92 GO terms. These genes were considered as potential candidate genes responsible for PDgr in the AH population. The B-class genes, represented in Arabidopsis by the MADS-box genes APETALA3 (AP3) and PISTILLATA (PI), experienced gene duplication events (Kramer and Hall, 2005). In B. napus, two types of AP3 genes, B.AP3.a and B.AP3.b, share a high similarity in amino acid sequences, except for an eight residue difference located at the C-terminus, and, the B.AP3.a specified petal and stamen development and B.AP3.b only specified stamen development (Zhang et al., 2011). Surprisingly, no A, B, C or E class genes from the ABCE model were identified underlying the three stable QTL CIs, indicating that novel genes for PDgr in B. napus might exist. To gain a better understanding of how the three QTLs control PDgr in rapeseed, it is necessary to isolate these loci through a map-based cloning strategy in the future study. This study provided useful information for understanding the genetic control of floral development in B. napus.
Author Contributions
XW and KY carried out the QTL analysis and wrote the manuscript. HL, QP, FC, and WZ participated in the field experiment. SC and MH made helpful suggestions to the manuscript. JZ designed, led, and coordinated the overall study.
Conflict of Interest Statement
The authors declare that the research was conducted in the absence of any commercial or financial relationships that could be construed as a potential conflict of interest.
Acknowledgments
The work was supported by National Natural Science Foundation of China (31371660), ‘948’ Project of Ministry of Agriculture (2011-G23), the Industry Technology System of Rapeseed in China (CARS-13), Natural Science Foundation of Jiangsu Province (BK20151369), and Jiangsu Agriculture Science and Technology Innovation Fund (CX(14)5011).
Supplementary Material
The Supplementary Material for this article can be found online at: http://journal.frontiersin.org/article/10.3389/fpls.2015.01164
References
Almeida, G. D., Makumbi, D., Magorokosho, C., Nair, S., Borém, A., Ribaut, J., et al. (2013). QTL mapping in three tropical maize populations reveals a set of constitutive and adaptive genomic regions for drought tolerance. Theor. Appl. Genet. 126, 583–600. doi: 10.1007/s00122-012-2003-7
Arcade, A., Labourdette, A., Falque, M., Mangin, B., Chardon, F., Charcosset, A., et al. (2004). BioMercator: integrating genetic maps and QTL towards discovery of candidate genes. Bioinformatics 20, 2324–2326. doi: 10.1093/bioinformatics/bth230
Basunanda, P., Radoev, M., Ecke, W., Friedt, W., Becker, H. C., and Snowdon, R. J. (2010). Comparative mapping of quantitative trait loci involved in heterosis for seedling and yield traits in oilseed rape (Brassica napus L.). Theor. Appl. Genet. 120, 271–281. doi: 10.1007/s00122-009-1133-z
Buzza, G. (1983). The inheritance of an apetalous character in Canola (Brassica napus). Cruciferae Newsletter 8, 11–12.
Cai, G., Yang, Q., Yang, Q., Zhao, Z., Chen, H., Wu, J., et al. (2012). Identification of candidate genes of QTLs for seed weight in Brassica napus through comparative mapping among Arabidopsis and Brassica species. BMC Genet. 13:105. doi: 10.1186/1471-2156-13-105
Cai, G., Yang, Q., Yi, B., Fan, C., Edwards, D., Batley, J., et al. (2014). A complex recombination pattern in the genome of allotetraploid Brassica napus as revealed by a high-density genetic map. PLoS ONE 9:e109910. doi: 10.1371/journal.pone.0109910
Cao, X., Liu, B., and Zhang, Y. (2013). SEA: a software package of segregation analysis of quantitative traits in plants (in Chinese with an English abstract). J. Nanjing Agric. Univ. 36, 1–6.
Causier, B., Schwarz-Sommer, Z., and Davies, B. (2010). Floral organ identity: 20 years of ABCs. Semin. Cell Dev. Biol. 21, 73–79. doi: 10.1016/j.semcdb.2009.10.005
Chalhoub, B., Denoeud, F., Liu, S., Parkin, I. A., Tang, H., Wang, X., et al. (2014). Early allopolyploid evolution in the post-Neolithic Brassica napus oilseed genome. Science 345, 950–953. doi: 10.1126/science.1253435
Chen, B., Wu, X., Lu, G., Gao, G., Xu, K., and Li, X. (2006a). Inheritance for apetalous trait of two kinds of apetalous rapeseed mutants (in Chinese with an English abstract). Chin. J. Oil Crop Sci. 28, 263–267.
Chen, B., Wu, X., Lu, G., Gao, G., Xu, K., and Li, X. (2006b). Molecular mapping of the gene (s) controlling petal-loss trait in Brassica napus. Hereditas(Beijing) 28, 707–712.
Chen, G., Geng, J., Rahman, M., Liu, X., Tu, J., Fu, T., et al. (2010). Identification of QTL for oil content, seed yield, and flowering time in oilseed rape (Brassica napus). Euphytica 175, 161–174. doi: 10.1007/s10681-010-0144-9
Chen, X., Li, X., Zhang, B., Xu, J., Wu, Z., Wang, B., et al. (2013). Detection and genotyping of restriction fragment associated polymorphisms in polyploid crops with a pseudo-reference sequence: a case study in allotetraploid Brassica napus. BMC Genomics 14:346. doi: 10.1186/1471-2164-14-346
Cheng, X., Xu, J., Xia, S., Gu, J., Yang, Y., Fu, J., et al. (2009). Development and genetic mapping of microsatellite markers from genome survey sequences in Brassica napus. Theor. Appl. Genet. 118, 1121–1131. doi: 10.1007/s00122-009-0967-8
Ching, A., Caldwell, K. S., Jung, M., Dolan, M., Smith, O. S., Tingey, S., et al. (2002). SNP frequency, haplotype structure and linkage disequilibrium in elite maize inbred lines. BMC Genet. 3:19. doi: 10.1186/1471-2156-3-19
Coen, E. S., and Meyerowitz, E. M. (1991). The war of the whorls: genetic interactions controlling flower development. Nature 353, 31–37. doi: 10.1038/353031a0
Delourme, R., Falentin, C., Fomeju, B. F., Boillot, M., Lassalle, G., Andre, I., et al. (2013). High-density SNP-based genetic map development and linkage disequilibrium assessment in Brassica napus L. BMC Genomics 14:120. doi: 10.1186/1471-2164-14-120
Ding, G., Zhao, Z., Liao, Y., Hu, Y., Shi, L., Long, Y., et al. (2012). Quantitative trait loci for seed yield and yield-related traits, and their responses to reduced phosphorus supply in Brassica napus. Ann. Bot. 109, 747–759. doi: 10.1093/aob/mcr323
Edwards, D., Batley, J., and Snowdon, R. J. (2013). Accessing complex crop genomes with next-generation sequencing. Theor. Appl. Genet. 126, 1–11. doi: 10.1007/s00122-012-1964-x
Fray, M. J., Puangsomlee, P., Goodrich, J., Coupland, G., Evans, E. J., Arthur, A. E., et al. (1997). The genetics of stamenoid petal production in oilseed rape (Brassica napus) and equivalent variation in Arabidopsis thaliana. Theor. Appl. Genet. 94, 731–736. doi: 10.1007/s001220050472
Habekotté, B. (1997). Options for increasing seed yield of winter oilseed rape (Brassica napus L.) a simulation study. Field Crop Res. 54, 109–126. doi: 10.1016/S0378-4290(97)00041-5
Hoekman, B. (2007). Global Economic Prospects 2008: Technology Diffusion in the Developing World. Washington, DC: World Bank Publications, 40–41.
Honma, T., and Goto, K. (2001). Complexes of MADS-box proteins are sufficient to convert leaves into floral organs. Nature 409, 525–529. doi: 10.1038/35054083
Huang, X., Wei, X., Sang, T., Zhao, Q., Feng, Q., Zhao, Y., et al. (2010). Genome-wide association studies of 14 agronomic traits in rice landraces. Nat. Genet. 42, 961–967. doi: 10.1038/ng.695
Jamaux, D. I., and Spire, D. (1999). Comparison of responses of ascospores and mycelium by ELISA with anti-mycelium and anti-ascospore antisera for the development of a method to detect Sclerotinia sclerotiorum on petals of oilseed rape. Ann. Appl. Biol. 134, 171–179. doi: 10.1111/j.1744-7348.1999.tb05253.x
Jiang, L. (2007). Breeding for apetalous rape: inheritance and yield physiology. Adv. Bot. Res. 45, 217–231. doi: 10.1016/S0065-2296(07)45008-X
Jiang, L., and Becker, H. (2003). Inheritance of apetalous flowers in a mutant of oilseed rape. Crop Sci. 43:508. doi: 10.2135/cropsci2003.0508
Kelly, A., Fray, M., Arthur, E. A., Lydiate, D. J., and Evans, E. J. (1995). “The genetic control of petalless flowers and upright pods,” in Proceedings of the 9th International Rapeseed Congress, Cambridge, 4–8.
Koch, M. A., Haubold, B., and Mitchell-Olds, T. (2000). Comparative evolutionary analysis of chalcone synthase and alcohol dehydrogenase loci in Arabidopsis, Arabis, and related genera (Brassicaceae). Mol. Biol. Evol. 17, 1483–1498. doi: 10.1093/oxfordjournals.molbev.a026248
Kosambi, D. D. (1943). The estimation of map distances from recombination values. Ann. Eugen. 12, 172–175. doi: 10.1111/j.1469-1809.1943.tb02321.x
Kramer, E. M., and Hall, J. C. (2005). Evolutionary dynamics of genes controlling floral development. Curr. Opin. Plant. Biol. 8, 13–18. doi: 10.1016/j.pbi.2004.09.019
Krizek, B. A., and Fletcher, J. C. (2005). Molecular mechanisms of flower development: an armchair guide. Nat. Rev. Genet. 6, 688–698. doi: 10.1038/nrg1675
Landry, B. S., Hubert, N., Etoh, T., Harada, J. J., and Lincoln, S. E. (1991). A genetic map for Brassica napus based on restriction fragment length polymorphisms detected with expressed DNA sequences. Genome 34, 543–552. doi: 10.1139/g91-084
Lefol, C., and Morrall, R. A. A. (1996). Immunofluorescent staining of Sclerotinia ascospores on canola petals. Can. J. Plant Pathol. 18, 237–241. doi: 10.1080/07060669609500618
Li, H., Yu, K., Guo, T., and Zhang, J. (2014). Genetic analysis of apetalous trait in Brassica napus using mixed model of major gene and polygene (in Chinese with an English abstract). Jiangsu J. Agric. Sci. 30, 253–258.
Linnemann, A. R., and Dijkstra, D. S. (2002). Toward sustainable production of protein-rich foods: appraisal of eight crops for Western Europe. Part I. Analysis of the primary links of the production chain. Crit. Rev. Food Sci. Nutr. 42, 377–401. doi: 10.1080/20024091054193
Liu, L., Qu, C., Wittkop, B., Yi, B., Xiao, Y., He, Y., et al. (2013). A high-density SNP map for accurate mapping of seed fibre QTL in Brassica napus L. PLoS ONE 8:e83052. doi: 10.1371/journal.pone.0083052
Long, Y., Shi, J., Qiu, D., Li, R., Zhang, C., Wang, J., et al. (2007). Flowering time quantitative trait loci analysis of oilseed Brassica in multiple environments and genomewide alignment with Arabidopsis. Genetics 177, 2433–2444. doi: 10.1534/genetics.107.080705
Lowe, A. J., Moule, C., Trick, M., and Edwards, K. J. (2004). Efficient large-scale development of microsatellites for marker and mapping applications in Brassica crop species. Theor. Appl. Genet. 108, 1103–1112. doi: 10.1007/s00122-003-1522-7
Lu, W., Liu, J., Xin, Q., Wan, L., Hong, D., and Yang, G. (2013). A triallelic genetic male sterility locus in Brassica napus: an integrative strategy for its physical mapping and possible local chromosome evolution around it. Ann. Bot. 111, 305–315. doi: 10.1093/aob/mcs260
Lu, Z., and Fu, S. (1990). Inheritance of apetalous character in rape (Brassica napus L.) and its implication in breeding (in Chinese with an English abstract). Jiangsu J. Agric. Sci. 6, 30–36.
Lysak, M. A., Koch, M. A., Pecinka, A., and Schubert, I. (2005). Chromosome triplication found across the tribe Brassiceae. Genome Res. 15, 516–525. doi: 10.1101/gr.3531105
Mauricio, R. (2001). Mapping quantitative trait loci in plants: uses and caveats for evolutionary biology. Nat. Rev. Genet. 2, 370–381. doi: 10.1038/35072085
McCouch, S. R., Cho, Y. G., Yano, M., Paul, E., Blinstrub, M., Morishima, H., et al. (1997). Report on QTL nomenclature. Rice Genet. News 14, 11–131. doi: 10.1007/s10142-013-0328-1
Mendham, N. J., Rao, M. S. S., and Buzza, G. C. (1991). “The apetalous flower character as a component of a high yielding ideotype,” in Proceedings of the 8th International Rapeseed Congress, Saskatoon, SK, 596–600.
Mendham, N. J., Shipway, P. A., and Scott, R. K. (1981). The effects of delayed sowing and weather on growth, development and yield of winter oil-seed rape (Brassica napus). J. Agric. Sci. 96, 389–416. doi: 10.1017/S002185960006617X
Mochida, K., Yamazaki, Y., and Ogihara, Y. (2003). Discrimination of homoeologous gene expression in hexaploid wheat by SNP analysis of contigs grouped from a large number of expressed sequence tags. Mol. Genet. Genomics 270, 371–377. doi: 10.1007/s00438-003-0939-7
Pinyopich, A., Ditta, G. S., Savidge, B., Liljegren, S. J., Baumann, E., Wisman, E., et al. (2003). Assessing the redundancy of MADS-box genes during carpel and ovule development. Nature 424, 85–88. doi: 10.1038/nature01741
Piquemal, J., Cinquin, E., Couton, F., Rondeau, C., Seignoret, E., Doucet, I., et al. (2005). Construction of an oilseed rape (Brassica napus L.) genetic map with SSR markers. Theor. Appl. Genet. 111, 1514–1523. doi: 10.1007/s00122-005-0080-6
Qiu, D., Morgan, C., Shi, J., Long, Y., Liu, J., Li, R., et al. (2006). A comparative linkage map of oilseed rape and its use for QTL analysis of seed oil and erucic acid content. Theor. Appl. Genet. 114, 67–80. doi: 10.1007/s00122-006-0411-2
Quijada, P. A., Udall, J. A., Lambert, B., and Osborn, T. C. (2006). Quantitative trait analysis of seed yield and other complex traits in hybrid spring rapeseed (Brassica napus L.): 1. Identification of genomic regions from winter germplasm. Theor. Appl. Genet. 113, 549–561. doi: 10.1007/s00122-006-0323-1
Raman, H., Dalton-Morgan, J., Diffey, S., Raman, R., Alamery, S., Edwards, D., et al. (2014). SNP markers-based map construction and genome-wide linkage analysis in Brassica napus. Plant Biotechnol. J. 12, 851–860. doi: 10.1111/pbi.12186
Ramanujam, S. (1940). An apetalous mutation in turnip (Brassica campestris L.). Nature 145, 552–553. doi: 10.1038/145552a0
Shi, J., Li, R., Qiu, D., Jiang, C., Long, Y., Morgan, C., et al. (2009). Unraveling the complex trait of crop yield with quantitative trait loci mapping in Brassica napus. Genetics 182, 851–861. doi: 10.1534/genetics.109.101642
Snowdon, R. J., and Iniguez Luy, F. L. (2012). Potential to improve oilseed rape and canola breeding in the genomics era. Plant Breed. 131, 351–360. doi: 10.1111/j.1439-0523.2012.01976.x
Stange, M., Utz, H. F., Schrag, T. A., Melchinger, A. E., and Würschum, T. (2013). High-density genotyping: an overkill for QTL mapping? Lessons learned from a case study in maize and simulations. Theor. Appl. Genet. 126, 2563–2574. doi: 10.1007/s00122-013-2155-0
Sun, Z., Wang, Z., Tu, J., Zhang, J., Yu, F., McVetty, P. B. E., et al. (2007). An ultradense genetic recombination map for Brassica napus, consisting of 13551 SRAP markers. Theor. Appl. Genet. 114, 1305–1317. doi: 10.1007/s00122-006-0483-z
Tan, X., Li, D., Wang, H., and Guo, A. (2003). Identification of a RAPD marker linked to a petal-controlled gene in Brassica napus (in Chinese with an English abstract). Acta Bot. Boreal. Occident. Sin. 23, 561–565.
Theißen, G., and Saedler, H. (2001). Plant biology: floral quartets. Nature 409, 469–471. doi: 10.1038/35054172
Udall, J. A., Quijada, P. A., Lambert, B., and Osborn, T. C. (2006). Quantitative trait analysis of seed yield and other complex traits in hybrid spring rapeseed (Brassica napus L.): 2. Identification of alleles from unadapted germplasm. Theor. Appl. Genet. 113, 597–609. doi: 10.1007/s00122-006-0324-0
Uzunova, M. I., and Ecke, W. (1999). Abundance, polymorphism and genetic mapping of microsatellites in oilseed rape (Brassica napus L.). Plant Breed. 118, 323–326. doi: 10.1046/j.1439-0523.1999.00371.x
Van Ooijen, J. W. (2006). JoinMap 4. Software for the Calculation of Genetic Linkage Maps in Experimental Populations. Kyazma BV.
Vignal, A., Milan, D., SanCristobal, M., and Eggen, A. (2002). A review on SNP and other types of molecular markers and their use in animal genetics. Genet. Sel. Evol. 34, 275–305. doi: 10.1051/gse:2002009
Virk, P. S., Khush, G. S., and Peng, S. (2004). Breeding to enhance yield potential of rice at IRRI: the ideotype approach. Int. Rice Res. Notes 29, 5–9.
Wang, S., Basten, C. J., and Zeng, Z. B. (2007). Windows QTL Cartographer 2.5. Raleigh, NC: North Carolina State University.
Wang, X., Long, Y., Yin, Y., Zhang, C., Gan, L., Liu, L., et al. (2015a). New insights into the genetic networks affecting seed fatty acid concentrations in Brassica napus. BMC Plant Biol. 15:91. doi: 10.1186/s12870-015-0475-8
Wang, X., Wang, H., Long, Y., Liu, L., Zhao, Y., Tian, J., et al. (2015b). Dynamic and comparative QTL analysis for plant height in different developmental stages of Brassica napus L. Theor. Appl. Genet. 128, 1175–1192. doi: 10.1007/s00122-015-2498-9
Wang, X., Wang, H., Long, Y., Li, D., Yin, Y., Tian, J., et al. (2013). Identification of QTLs associated with oil content in a high-oil Brassica napus cultivar and construction of a high-density consensus map for QTLs comparison in B. napus. PLoS ONE 8:e80569. doi: 10.1371/journal.pone.0080569
Yang, Y., Lai, K., Tai, P., and Li, W. (1999). Rates of nucleotide substitution in angiosperm mitochondrial DNA sequences and dates of divergence between Brassica and other angiosperm lineages. J. Mol. Evol. 48, 597–604. doi: 10.1007/PL00006502
Yu, H., Xie, W., Wang, J., Xing, Y., Xu, C., Li, X., et al. (2011). Gains in QTL detection using an ultra-high density SNP map based on population sequencing relative to traditional RFLP/SSR markers. PLoS ONE 6:e17595. doi: 10.1371/journal.pone.0017595
Zhang, D., Hua, Y., Wang, X., Zhao, H., Shi, L., and Xu, F. (2014). A high-density genetic map identifies a novel major QTL for boron efficiency in oilseed rape (Brassica napus L.). PLoS ONE 9:e112089. doi: 10.1371/journal.pone.0112089
Zhang, J., Fu, S., Qi, C., Pu, H., and Wu, X. (2002). Preliminary report of breeding for near-isogenic lines of apetalous in rapeseed (B. napus L.) (in Chinese with an English abstract). Chin. J. Oil Crop Sci. 24, 14–16.
Zhang, J., Qi, C., Li, G., Pu, H., Chen, S., Chen, X., et al. (2007a). Genetic map construction and apetalousness QTLs identification in rapeseed (Brassica napus L.) (in Chinese with an English abstract). Acta. Agron. Sin. 33, 1246–1254.
Zhang, J., Qi, C., Pu, H., Chen, S., Chen, F., Chen, X., et al. (2007b). Genetic analysis of apetalous in Brassica napus L. using mixed model of major gene and polygene (in Chinese with an English abstract). Chin. J. Oil Crop Sci. 29, 227–232.
Zhang, R., Tang, X., Li, M., and Chen, D. (2007c). Study on genetics of apetalous line NF001 in rapeseed (B. napus L.) (in Chinese with an English abstract). Seed 26, 13–15.
Zhang, Y., Wang, X., Zhang, W., Yu, F., Tian, J., Li, D., et al. (2011). Functional analysis of the two Brassica AP3 genes involved in apetalous and stamen carpelloid phenotypes. PLoS ONE 6:e20930. doi: 10.1371/journal.pone.0020930
Keywords: Brassica napus L., apetalous, single nucleotide polymorphism, high-density map, quantitative trait locus, recombinant inbred line
Citation: Wang X, Yu K, Li H, Peng Q, Chen F, Zhang W, Chen S, Hu M and Zhang J (2015) High-Density SNP Map Construction and QTL Identification for the Apetalous Character in Brassica napus L. Front. Plant Sci. 6:1164. doi: 10.3389/fpls.2015.01164
Received: 10 September 2015; Accepted: 07 December 2015;
Published: 23 December 2015.
Edited by:
Juan Francisco Jimenez Bremont, Instituto Potosino de Investigacion Cientifica y Tecnologica, MexicoReviewed by:
Estefanía Carrillo, Instituto Nacional de Investigaciones Agropecuarias, EcuadorJavier Sanchez, University of Zürich, Switzerland
Copyright © 2015 Wang, Yu, Li, Peng, Chen, Zhang, Chen, Hu and Zhang. This is an open-access article distributed under the terms of the Creative Commons Attribution License (CC BY). The use, distribution or reproduction in other forums is permitted, provided the original author(s) or licensor are credited and that the original publication in this journal is cited, in accordance with accepted academic practice. No use, distribution or reproduction is permitted which does not comply with these terms.
*Correspondence: Jiefu Zhang, jiefu_z@163.com
†These authors have contributed equally to this work.