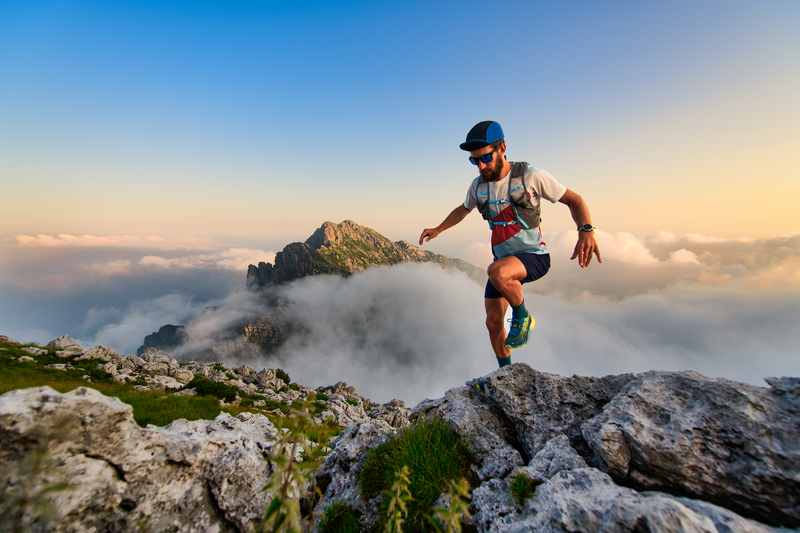
94% of researchers rate our articles as excellent or good
Learn more about the work of our research integrity team to safeguard the quality of each article we publish.
Find out more
REVIEW article
Front. Plant Sci. , 27 October 2015
Sec. Plant Nutrition
Volume 6 - 2015 | https://doi.org/10.3389/fpls.2015.00916
Boron (B) is an essential microelement for higher plants, and its deficiency is widespread around the world and constrains the productivity of both agriculture and forestry. In the last two decades, numerous studies on model or herbaceous plants have contributed greatly to our understanding of the complex network of B-deficiency responses and mechanisms for tolerance. In woody plants, however, fewer studies have been conducted and they have not well been recently synthesized or related to the findings on model species on B transporters. Trees have a larger body size, longer lifespan and more B reserves than do herbaceous plants, indicating that woody species might undergo long-term or mild B deficiency more commonly and that regulation of B reserves helps trees cope with B deficiency. In addition, the highly heterozygous genetic background of tree species suggests that they may have more complex mechanisms of response and tolerance to B deficiency than do model plants. Boron-deficient trees usually exhibit two key visible symptoms: depression of growing points (root tip, bud, flower, and young leaf) and deformity of organs (root, shoot, leaf, and fruit). These symptoms may be ascribed to B functioning in the cell wall and membrane, and particularly to damage to vascular tissues and the suppression of both B and water transport. Boron deficiency also affects metabolic processes such as decreased leaf photosynthesis, and increased lignin and phenol content in trees. These negative effects will influence the quality and quantity of wood, fruit and other agricultural products. Boron efficiency probably originates from a combined effect of three processes: B uptake, B translocation and retranslocation, and B utilization. Root morphology and mycorrhiza can affect the B uptake efficiency of trees. During B translocation from the root to shoot, differences in B concentration between root cell sap and xylem exudate, as well as water use efficiency, may play key roles in tolerance to B deficiency. In addition, B retranslocation efficiency primarily depends on the extent of xylem-to-phloem transfer and the variety and amount of cis-diol moieties in the phloem. The B requirement for cell wall construction also contribute to the B use efficiency in trees. The present review will provide an update on the physiological and molecular responses and tolerance mechanisms to B deficiency in woody plants. Emphasis is placed on the roles of B reserves that are more important for tolerance to B deficiency in trees than in herbaceous plants and the possible physiological and molecular mechanisms of differential B efficiency in trees. We propose that B may be used to study the relationship between the cell wall and the membrane via the B-bridge. Transgenic B-efficient tree cultivars have considerable potential for forestry or fruit rootstock production on low B soils in the future.
Boron (B) is essential for the growth and development of higher plants (Warington, 1923). In the past 90 years, numerous significant advances have been made in understanding the function of B in plants. The current understanding of the biological function of B in plants is that it plays a structural role in both the cell wall and plasma membrane. In the cell wall, B forms borate esters with apiose residues of the pectic domain rhamnogalacturonan II (RG-II; Kobayashi et al., 1996). This B–RG-II complex is essential for cell wall structure and function (O’Neill et al., 2004) because it contributes significantly to the control of cell wall porosity (Fleischer et al., 1999) and tensile strength (Ryden et al., 2003). A recent study reported that glycosylinositol phosphorylceramides (GIPCs), the major components of lipid rafts, participate in the formation of the GIPC–B–RG-II complex, suggesting the structural role of B in membranes (Voxeur and Fry, 2014).
Boron deficiency is a widespread problem in both agriculture and forestry, particularly on sandy and alkaline soils (Bell and Dell, 2008). Areas where low B soils are found include South and Southeast Asia, Eastern Australia and New Zealand, Africa, North and South America, and Northern Europe (reviewed by Lehto et al., 2010). Low B causes significant losses of yield or quality by influencing vegetative or reproductive growth in forest trees (Stone et al., 1982; White and Krause, 2001; Lehto et al., 2010), fruit trees (Raja et al., 2005; Kumar, 2011; Ganie et al., 2013; Liu et al., 2013b) and woody plants (Broschat, 2005; Tewari et al., 2010; Hajiboland et al., 2011a; Patnude and Nelson, 2012). However, compared to model or herbaceous plants, in woody trees the understanding of B-deficiency responses and tolerance mechanisms is limited. Recently, advances have been made about the regulation of B transport both in herbaceous and woody plants. For instance, B transport system and its transporters were better understood (Miwa et al., 2013; Tanaka et al., 2013; Chatterjee et al., 2014; Durbak et al., 2014; Hanaoka et al., 2014); and studies of the signal transduction of B starvation responses were initiated (González-Fontes et al., 2013; Quiles-Pando et al., 2013; González-Fontes et al., 2014). These new findings provide important insights for understanding B deficiency in woody plants. Here, the recent achievements regarding the response and tolerance mechanisms of B deficiency in woody plants, as well as relevant findings in model or herbaceous plants, are summarized, and perspectives are also proposed.
Boron-deficient trees exhibit various visible symptoms in both vegetative and reproductive organs. Boron deprivation initially reduces the elongation of growing points due to restricted cell wall deposition and then, in more extreme cases, induces the necrosis of these tissues owing to cell death. This negative effect directly reduces root growth, particularly the lateral roots (Mei et al., 2011; Zhou et al., 2014). Boron deficiency also supresses growth in the aerial parts, such as plant height and leaf area (Möttönen et al., 2001; Wojcik et al., 2008). If B deficiency lasts for many years, it results in the stunted appearance of trees. In some forest trees, long-term B deficiency can reduce the quality and utility of wood. Loblolly pine (Pinus taeda), for example, can grow normally for the first 3 years and then experience dieback under low B conditions (Vail et al., 1961). Similar symptoms of dieback are also observed in other tree species (Table 1). Deficient symptoms in leaves, including chlorosis, necrosis or malformation, have been reported in many tree and woody species (Table 1). When grape (Vitis vinifera) was cultured under low B conditions, diffuse yellowing of the young leaves, brownish areas of apical tendrils and cupping of the third–fourth leaves from the shoot tips were observed in the early stage (cultured for 1 month). With the increasing time, the leaves became more cupped and chlorotic, and the tendrils developed transverse cracks and necrosis (Scott and Schrader, 1947). Mulberry (Morus alba), whose leaves are used to feed silkworm, changed to cup-shaped leaves with bent and cracked veins under B limitation (Tewari et al., 2010). In addition, reproductive growth, especially flowering, fruit set and yield, is more sensitive to low B than is vegetative growth (Dell and Huang, 1997). In grape, flower and fruit cluster necrosis, and small “shot berries” that are round to pumpkin-shaped often appear due to B starvation (Christensen et al., 2006). The papaya (Carica papaya) fruit is often affected by B deficiency with latex secretion and deformity (Wang and Ko, 1975). Usually, milky latex secretion appears in the fruit surface at the early stage, after which the milky latex becomes brown. Finally, the fruit surface becomes rough and deformed (Wang and Ko, 1975).
Taken together, B deficiency symptoms in trees can be divided into two main groups. One is the inhibition, even necrosis, of growing points, such as the root tip, bud, flower, and young leaf. Light microscopy observation showed that cell death occurred in B-deficient Norway spruce (Picea abies) needle buds (Sutinen et al., 2006, 2007), probably due to the B function in the cell wall. The other type of symptom is the deformity of some organs, such as the shoot, leaf, and fruit. Relevant anatomical studies demonstrated that B deficiency could severely damage the vascular tissues and induce hypertrophy at the tissue/cellular level. A disorganized vascular tissue was induced by B deficiency in coffee (Coffea arabica), and discontinuities of both xylem and phloem vessels were observed in the B-deficient stem tip and young leaf (Rosolem and Leite, 2007). Boron deficiency also reduced the length of the xylem vessel in both the leaf and fruit vascular bundles and reduced the diameter of the xylem vessel in only the leaf vascular bundle in pumelo (Citrus grandis; Liu et al., 2013b). A consistent observation reported is that B deficiency can increase vascular cross sectional areas in Norway spruce needle (Sutinen et al., 2006, 2007), pumelo leaf and fruit vascular tissues (Liu et al., 2013b), and sweet orange (Citrus sinensis) leaf veins (Yang et al., 2013). These results suggest that B deficiency can increase the size but weaken the function of vascular tissue in trees.
Boron plays a crucial role in cell wall structure (O’Neill et al., 1996; Matoh, 1997; Brown et al., 2002; Goldbach and Wimmer, 2007). In B-deficient plants, the structures of the cell wall are strongly altered at both the macroscopic and microscopic levels (Loomis and Durst, 1992; Shorrocks, 1997). At the subcellular level, B starvation usually results in abnormally formed walls that are often thick and brittle as a consequence of altered mechanical properties and abnormal expansion (Brown et al., 2002). It has been suggested that B may be necessary for cell-to-wall adhesion and for the organization of the architectural integrity of the cell (Lord and Mollet, 2002; Bassil et al., 2004). This is further supported by an altered cell wall porosity and tensile strength under B deficiency (Fleischer et al., 1999; Ryden et al., 2003). In citrus and tea trees, B deprivation not only resulted in a heavily thickened and folded cell walls in roots (Zhou et al., 2015) but also increased the portion of the cell wall relative to the whole cell (Hajiboland et al., 2013b; Liu et al., 2013a) and induced changes in both the amount and assembly of its component polymers in leaves (Liu et al., 2014a). In forest trees, B deficiency impaired the primary cell wall, and interrupted the structural development of organs and whole plants, resulting in adverse impacts on tree formation, wood quality and cold tolerance (Lehto et al., 2010).
In general, the responses of membranes to B deficiency are faster than those of the cell wall (Goldbach et al., 2001; Brown et al., 2002). For example, within minutes of B deprivation, inhibition of plasma membrane-bound oxidoreductase activity was frequently observed (Barr et al., 1993). In addition, B-deficient plants often exhibit lower ion uptake rates in roots but higher efflux of potassium and organic compounds in leaves than B-sufficient plants (Pollard et al., 1977; Cakmak et al., 1995; Goldbach and Wimmer, 2007). The membrane-bound ATPase activity was reduced by B deficiency, but within 1 h after resupplying B, the activity was restored to the same level as that in B-sufficient bean (Phaseolus vulgaris) and maize (Zea mays) roots (Pollard et al., 1977). These results suggest that B deficiency might disturb the membrane transport process, the activity of membrane-located proteins, and the integrity and functioning of the plasma membrane (Brown et al., 2002; Goldbach and Wimmer, 2007; Camacho-Cristóbal et al., 2008b).
There is increasing evidence that B may play structural roles in the cell wall to cell membrane interface (O’Neill et al., 2001; Brown et al., 2002; Goldbach and Wimmer, 2007; Voxeur and Fry, 2014). Bassil et al. (2004) proposed that B may function in transvacuolar cytoplasmic strands and cell-to-wall adhesion. Recently, these predictions were partly confirmed by Voxeur and Fry (2014). These authors found that B can bind both the RG-II of the cell wall and the GIPCs of the cell membrane, thus forming a GIPC–B–RG-II complex (Voxeur and Fry, 2014). As a result, B serves as a bridge to connect the cell wall and the plasma membrane, which opens a possible avenue to probe the relationship between the cell wall and the membrane via the B-bridge. The GIPC–B–RG-II complex may also explain, at least partly, why both the cell wall and membrane are influenced by B deficiency.
Early detectable changes in B-deficient plants are considered to be reflected by the damage of the cell membrane (see Cell Wall and Membrane) or the disturbances of hormonal metabolism (Blevins and Lukaszewski, 1998; Martín-Rejano et al., 2011; Abreu et al., 2014; Camacho-Cristóbal et al., 2015), but the primary reaction remains unclear. However, the accumulation of phenols has repeatedly been observed in B-deficient plants (Cakmak and Römheld, 1997; Marschner, 2012). It is believed that the accumulation of phenolic compounds is an indirect long-term effect of B deficiency (Marschner, 2012). Boron starvation first damages the integrity of the cell wall and membrane, disrupting the phenol metabolism-related enzyme systems, such as phenylalanine-ammonium lyase (PAL; Cakmak et al., 1995; Brown et al., 2002), and thus results in the accumulation of phenols and related alterations of lignin synthesis from phenol alcohols (Pilbeam and Kirkby, 1983; Yang et al., 2013; Zhou et al., 2015). In tea and olive trees, significant accumulation of phenolic compounds has been detected in B-deficient leaves (Liakopoulos et al., 2005; Hajiboland et al., 2013a). The excessive accumulation of phenols probably leads to tissue necrosis. The poorly lignified branches of woody trees due to B deficiency may be unable to support the weight of leaves (Dell and Huang, 1997). Additionally, changes in phenol and lignin may also affect plant defense systems against herbivory and pathogens (Lehto et al., 2010).
There is no evidence that B plays a direct role in photosynthesis (Dell and Huang, 1997). However, B deficiency limits root growth and results in a weak vascular tissue, which may restrain water uptake and transport within the plant and further alter leaf function (e.g., the reduction of stomata number and abnormal shapes, reviewed by Wimmer and Eichert, 2013). Moreover, a wealth of information is available to suggest that B deficiency may indirectly affect photosynthesis by decreasing the photosynthetic area and altering the leaf constituents (Dell and Huang, 1997; Brown et al., 2002). As in herbaceous species (Dell and Huang, 1997), B deficiency of leaves in trees reduces the content of chlorophyll, CO2 assimilation and stomatal conductance, as well as the activities of photosynthetic enzymes and catalase, but enhances the production of reactive oxygen species (ROS) and intercellular CO2 concentration, thereby resulting in decreased photosynthetic capability (Han et al., 2008; Wojcik et al., 2008; Tewari et al., 2010). Moreover, the accumulation of soluble sugars in B-deficient leaves of trees may also produce feedback inhibition to net photosynthesis (Han et al., 2008; Ruuhola et al., 2011; Hajiboland et al., 2013b; Lu et al., 2014).
Interestingly, in woody perennial trees, B reserves play a significant role in response to B deficiency, particularly during the spring growth flush (Tromp, 1983; Spiegel-Roy and Goldschmidt, 1996). Boron reserves, also known as B storage, are defined as those B-containing substances that are not used directly in functioning but are primarily stored in the apoplast and cytoplasm of the tree until required (Tromp, 1983; Dannel et al., 2002; Du et al., 2002; Lehto et al., 2010). In contrast to herbaceous plants, trees are perennial and have a large body; therefore, trees may store adequate B to cope with B deficiency at a later stage. Generally, three B forms exist in plants: free B (B in the apoplast), semi-bound B (B in the cytoplasm), and bound B (B in the cell wall; Du et al., 2002). Previous studies have suggested that the forms of B reserves may be limited to free B and semi-bound B because bound B for cell wall construction is not retranslocated (Dannel et al., 2002; Matoh and Ochiai, 2005; Lehto et al., 2010; Liu et al., 2011; Wang et al., 2014).
Apart from the stage of growth (e.g., flowering and fruiting) and field management (e.g., leaf pruning; Goh et al., 2007), the amount of stored B in the plant is one of the most important factors that influence the B requirement of woody plants. Effective B application for trees is frequently observed, even though there is apparently sufficient B in leaves and no B-deficient symptoms evident (Hanson, 1991; Nyomora et al., 1999; Perica et al., 2001b; Sánchez and Righetti, 2005; Wells et al., 2008; Moura et al., 2013). Furthermore, the B-fertilized Norway spruce seedlings can maintain a foliar B concentration greater than the deficiency limit for 2–13 years, with larger trees having longer-lasting effects (Kilpeläinen et al., 2013; Riikonen et al., 2013). In addition, temporary B deficiency may occur in trees due to insufficient B reserves for redistribution within the plant, or limited B retranslocation in phloem, or to particular environmental conditions (e.g., low soil water and vapor pressure deficit; Bell, 2000). Hence, B application to enhance B storage in perennial organs, may result in increased B retranslocation, and thus improve the fruit set, yield, and wood quality of trees.
The effects of B application in woody plants are influenced by fertilization methods (Nyomora et al., 1999; Boaretto et al., 2011) and for foliar applications by leaf surface characteristics (Perica et al., 2001b; Will et al., 2012). Unlike annual plants, trees are perennial and commonly suffer from long-term B deficiency. For long-term B-deficient trees, the leaf structure may be more severely influenced than the root anatomy (see Plant Growth and Visual Symptoms), despite the faster response to B deficiency in roots than in shoots (Dell and Huang, 1997). This is because the root will not transport B to the shoot until its own essential requirements are met, as indicated by the fact that B retranslocation increases with an increase in B stored in the reserve tissues (the terms “B translocation” and “B retranslocation” used here refer to the processes of B transport in the xylem and phloem respectively, see details in Section B Translocation and Retranslocation; Boaretto et al., 2008; Lehto et al., 2010). In a limited-B-retranlocation species, the recovery of labeled B fertilizer in fruits was higher from soil application (21%) than for foliar application (7%; Boaretto et al., 2011). Interestingly, similar results were reported in a high-B-retranlocation species (Wojcik et al., 2008). Therefore, when applying B to B-deficient trees, application via the root system is more effective than foliar application, regardless of B retranslocation in plant species.
It has been suggested that stored B in older plant parts can be retranslocated to new tissues via the phloem during periods of rapid growth, and the extent of B retranslocation depends primarily on the B status and sugar alcohols in trees (Lehto et al., 2010). In sweet orange, 20–35% of the B content in new parts was retranslocated from plant B reserves (Boaretto et al., 2008). However, it should be noted that the proportion of B retranslocated varies more substantially among plant species than that of other essential plants nutrients (Brown and Shelp, 1997). For example, only 3.2% of newly acquired B by leaves was retranslocated in orange trees (Boaretto et al., 2011), whereas more than 70–80% of newly acquired B by leaves could be exported to young tissues in apple (Malus domestics), pear (Pyrus communis), prune (Prunus domestics), and sweet cherry (Prunus avium; Picchioni et al., 1995). Using the B concentration gradient along the shoot axis, and foliar 10B labeling, it has been demonstrated that 26 out of 31 deciduous tree species retranslocated B, while 10 out of 19 evergreen tree species did not retranslocated B in the phloem (Brown and Hu, 1998; Lehto et al., 2004b; Konsaeng et al., 2005). Furthermore, Aphalo et al. (2002) pointed out that the capacity for B retranslocation in conifer forests may be related to natural selection to avoid B stress in leaves with a long lifespan. These results indicate that the extent of B retranslocation may be greater in deciduous trees than in evergreen trees, probably due to the form and location of B reserves for each group of tree species during natural selection. The evolutionary advantage of B retranslocation is significant. Deciduous trees must retranslocate B from annual tissues to perennial tissues in a timely manner during the fall because the retranslocated B (also known as B reserve) plays a critical role in the growth of new organs in the subsequent spring. In contrast, evergreen trees do not experience defoliation and thus have less necessity to retranslocate B to new organs than do deciduous trees. This suggests that trees possess different adaptive strategies to B deficiency depending on climatic conditions.
In recent years, increasing numbers of papers on the molecular identification of B transporters in plants have been reported. More importantly, many B transporters are B-deficiency induced and their functions will become essential under low B conditions. To date, two types of B transporters have been identified: BORs, which perform a B export role in plant cells, and major intrinsic proteins (MIPs), including some boric acid channels (Miwa and Fujiwara, 2010; Reid, 2014). Both types of B transporters contribute to B uptake by roots (Takano et al., 2006; Durbak et al., 2014; Hanaoka et al., 2014), xylem loading and B distribution (Takano et al., 2001; Nakagawa et al., 2007; Tanaka et al., 2008) and B utilization within the plant under B-limited conditions (Miwa et al., 2013).
Arabidopsis thaliana BOR1, the first identified B exporter, plays a key role in xylem loading and B distribution within shoots (Takano et al., 2005). AtBOR1 is expressed in the columella, lateral root cap, epidermis, and endodermis in the root tip, and in the epidermis and endodermis in the elongation zone (Takano et al., 2010). AtBOR1 mRNA accumulation was not strongly expressed, while BOR1-GFP fusion protein accumulation was elevated under a limited B supply (Takano et al., 2005). AtBOR2 is an efflux-type B transporter that is localized to the plasma membrane and has been proposed to facilitate the effective cross linking of RG-II by B in the cell wall and root cell elongation. This protein is strongly expressed in the lateral root caps and epidermis of the elongation zones of roots and is essential for root cell elongation under low B conditions (Miwa et al., 2013). OsBOR1, one of four AtBOR1-like proteins in rice (Oryza sative), is a plasma-membrane-localized efflux transporter of B and is required for the normal growth of rice plants under B limitation (Nakagawa et al., 2007). OsBOR4, another AtBOR1-like protein in rice, is both highly and specifically expressed in pollen. With the same transporter type and subcellular location as those of OsBOR1, OsBOR4 is essential for normal pollen germination and/or tube elongation in reproductive processes (Tanaka et al., 2013). ZmRTE (ROTTEN EAR), an ortholog of AtBOR1 in maize, is also a B efflux transporter and required for inflorescence development and fertility under B-limited conditions (Chatterjee et al., 2014). In addition to these basic studies in model plants, homologs of AtBOR1 exist in tree species. Both VvBOR1 (Pérez-Castro et al., 2012) and Citrus macrophylla BOR1 (Cañon et al., 2013) are plasma-membrane-localized efflux B transporters. VvBOR1 is mainly expressed in the root but also in other tissues. The relative expression of this gene in root is 1.9 times higher than that in flowers. Boron-deficient grape vines display symptoms of shot berries (Christensen et al., 2006; Pérez-Castro et al., 2012). However, at the fruit setting stage, the transcript accumulation of VvBOR1 in shot berries is significantly less than that in normal berries (Pérez-Castro et al., 2012). VvBOR1 transcripts increase at anthesis and then gradually decrease until late development stages during berry development. CmBOR1 is expressed in the leaves, stem and flowers and shows the greatest level in the roots. A significantly increased expression of CmBOR1 is observed in shoots under B-deficiency conditions (Cañon et al., 2013).
The MIP superfamily in plants can be subdivided into five evolutionarily distinct sub-families, including nodulin-26-like intrinsic proteins (NIPs), plasma membrane intrinsic proteins (PIPs), small basic intrinsic proteins (SIPs), tonoplast intrinsic proteins (TIPs) and uncharacterized X intrinsic proteins (XIPs) (Ishibashi et al., 2011). Some MIP members are boric acid channels facilitating B influx into cells. In Arabidopsis, both NIP5;1 and NIP6;1 are localized in the plasma membrane, but their B transport functions are different: NIP5;1 is involved in the initial uptake process in root cells (Takano et al., 2006), while NIP6;1 may function in xylem-phloem B transfer into young growing tissues (Tanaka et al., 2008). Genetic research on OsNIP3;1 (Hanaoka et al., 2014) and ZmNIP3;1 (also called TASSEL-LESS1 protein, TLS1; Durbak et al., 2014) demonstrated that they function as boric acid channels and are required for vegetative and reproductive development. Three PIP members, ZmPIP1 (Dordas and Brown, 2001), Hordeum vulgare PIP1;3 and HvPIP1;4 (Fitzpatrick and Reid, 2009), increase the sensitivity of single cells to B, but their functions in whole plants are unclear. In citrus, an AtNIP5-like gene of trifoliate orange (Poncirus trifoliata), CiNIP5, is expressed mainly in the roots of citrus seedlings, and its transcripts increase significantly under B deficiency (An et al., 2012; Zhou et al., 2015).
Of the previously mentioned studies on B transporters, the majority involve model plants, whereas few were on tree species. In fact, the mechanism of B uptake, transport and distribution in woody plants is more complicated than current knowledge on model plants. For example, mycorrhiza exist in most herbaceous and woody plants and play an assistant role in the plant B uptake process (Lehto et al., 2004b; Ruuhola and Lehto, 2014). Unfortunately, no work has been reported on the existence of B transporters in mycorrhiza. Such studies would form a useful adjunct to research on B transporters in plants. However, the conserved function of B transporters is observed across model and woody species. VvBOR1, for example, restores the phenotype of Arabidopsis bor1-3 mutants under B deprivation (Pérez-Castro et al., 2012). Based on this consistency, the above new findings in model herbaceous plants could also provide a better understanding of the B deficiency response in woody plants. Moreover, to better understand the B transport system, studies are needed to identify and characterize potential B transporters in different tissue of trees, especially under B-deficiency conditions.
As described in Section “Cell Wall and Membrane,” B deficiency causes abnormally formed cell walls that are often thick and brittle (Brown et al., 2002). Generally, the cell wall thickening process requires two elements: polysaccharides (e.g., cellulose and xylans) and aromatic components (e.g., lignins; Goujon et al., 2003). It is therefore likely that B deficiency may affect the expression patterns of cell-wall-related genes: decreasing production of molecules that are related to cell wall elements synthesis and inhibiting molecules that are related to cell wall extensibility modification. When Arabidopsis roots are under short-term B deficiency, decreased transcript counts of cell wall modification-related genes are observed by transcriptomic analysis (Camacho-Cristóbal et al., 2008a). In Citrus species, B deficiency suppressed the expression of cell-wall-modifying enzyme genes in the roots (Zhou et al., 2015) but increased the expression of lignin biosynthesis pathway genes in both the roots (Zhou et al., 2015) and leaf veins (Yang et al., 2013). These results suggest that B deficiency affects the expression of cell-wall-related genes in both herbaceous and woody plants.
Signal transduction in B-deficient plants is becoming an increasingly interesting topic. Three hypotheses for the B deprivation signaling pathway that transmits the signal from the cell wall to the cytoplasm and nucleus are summarized in a recent review (González-Fontes et al., 2014): (i) the alteration in cell wall structure promotes signal transduction; (ii) the accumulation of ROS induces Ca2+ signaling and cell death; and (iii) a low intracellular B level activates transcription factors and target genes. The authors have proposed a major role of Ca2+ and Ca2+-related proteins in this pathway (González-Fontes et al., 2014). Subsequently, crucial evidence supporting a membrane structure role of B was provided (Voxeur and Fry, 2014). Biological membranes contain not only lipids and proteins but also particular domains (rafts). These rafts are enriched in sterol and sphingolipids and are depleted in unsaturated phospholipids (Mongrand et al., 2010). In recent years, the possible role of plant rafts as signal transduction platforms has been frequently proposed and well summarized (see more information in Mongrand et al., 2010, and Simon-Plas et al., 2011). Moreover, as major components of membrane rafts, GIPCs participate in the formation of a GIPC–B–RG-II complex (Voxeur and Fry, 2014). Here, we propose a hypothesis, based on the negative effect on growing root cells and on the faster responses of membranes than those of the cell wall to B deficiency, and on a B deprivation signaling pathway. The hypothesis is as follows: a lack of the GIPC–B–RG-II complex for cell wall and membrane construction in root meristem and elongation cells might trigger the signaling pathway via the activation of a potential membrane protein or protein complex, and then the induced cytoplasmic Ca2+ signal pathway activates the downstream transcription events. Although little genetic or molecular evidence supports this hypothesis, phosphoproteomic analysis and single protein function research in both herbaceous and woody plant species will shed light on this topic.
Boron efficiency as used here refers to the extent of variation in response to low B among genotypes within one species and among plant species (Rerkasem and Jamjod, 1997). Boron-efficient genotypes are those that are able to grow well in soils in which other genotypes are adversely affected by B deficiency, and the opposite is the case for B-inefficient genotypes (Graham, 1984; Rerkasem and Jamjod, 1997). As in herbaceous plants (Bellaloui and Brown, 1998; Stangoulis et al., 2001; Nachiangmai et al., 2004; Rerkasem and Jamjod, 2004; Zhang et al., 2014), differential B efficiencies have also been observed in a variety of trees (Rerkasem and Jamjod, 1997; Lehto et al., 2004b; Xiao et al., 2007; Mattiello et al., 2009; Sheng et al., 2009a,b). It is widely accepted that the wide range of B efficiency among genotypes is associated with B uptake rate (B uptake efficiency), B translocation and retranslocation (B transport efficiency), and B utilization within the plants (B use efficiency; Marschner, 2012).
At adequate to high B supply, B uptake occurs via passive diffusion across the lipid bilayer, whereas at low B supply, B in the external medium is initially taken up into the root symplasm through a passive facilitated transport process (Dannel et al., 2002; Miwa and Fujiwara, 2010). There are genotype-related differences in B uptake efficiency among trees. For example, under B-deficiency conditions, the sweet orange scion grafted on Carrizo citrange (Citrus sinensis ×Poncirus trifoliata) had a higher newly acquired B concentration in leaves than those grafted on trifoliate orange, suggesting that the rootstock Carrizo citrange has a greater B uptake efficiency than trifoliate orange (Liu et al., 2012). Boron uptake efficiency of trees has been suggested to be associated with root morphology (Mei et al., 2011) and mycorrhizas (Lehto et al., 2004b; Ruuhola and Lehto, 2014). Under low B conditions, B-efficient tree cultivars usually show less depression of root length and number (Mei et al., 2011) and thereby exhibit a higher B absorption rate (Wojcik et al., 2003; Han et al., 2012) compared to B-inefficient cultivars. Mycorrhizas, which often exist in symbiosis with trees, may also play an important role in B uptake efficiency. In silver birch (Betula pendula), B uptake rate was higher in Laccaria-inoculated than Laccaria-non-inoculated seedlings (Ruuhola and Lehto, 2014). At the molecular level, the AtNIP5;1 gene is a boric acid channel that is involved in the initial uptake process in root cells (Takano et al., 2006). The overexpressed lines for this gene have greater root elongation under B-limited conditions (Kato et al., 2009). These results suggest that greater accumulation of transcripts of the boric acid channel gene could increase tolerance to B starvation by enhancing the initial uptake process. This suggestion is further supported by the fact that the CiNIP5 transcript level in the roots of B-efficient Carrizo citrange increased continuously to 7.7 times at 48 h after B-deficiency treatment compared to the initial level, whereas that of B-inefficient fragrant citrus (Citrus junos) increased only to 4.4 times at 24 h and then decreased (An et al., 2012). A similar observation was also recently described in that the level of NIP5;1 mRNA in roots after 12 h of B deficiency was up-regulated 5.2 times for B-efficient Carrizo citrange, but only 3.8 times for B-inefficient trifoliate orange (Zhou et al., 2015).
At adequate to toxic B supply, a substantial retention of B in the root symplasm occurs at xylem loading; at low B supply, the B retained in the symplasm can be loaded into the xylem by an active transport system (Dannel et al., 2002). Once loaded into the xylem, B can be translocated from the root to shoot by transpiration under high B conditions, but by active transport processes at low B supply (Raven, 1980; Shelp et al., 1995; Eichert and Goldbach, 2010; Miwa and Fujiwara, 2010), both of which are influenced by water use efficiency (Wimmer and Eichert, 2013). In general, B translocation efficiency is evaluated by the ratio between B concentration in the root cell sap and xylem exudate using a stable isotope 10B tracer. Under B-deficiency conditions, B-efficient genotypes usually have relatively higher B concentrations in xylem exudates than B-inefficient genotypes, probably due to the greater ability to translocate B from the root to the shoot. For example, at low B supply, the B-efficient tomato (Solanum lycopersicum) cultivar ‘Rutgers’ had a higher B concentration in xylem exudate than the B-inefficient cultivar ‘T3238’, but a similar B concentration was found in the root cell sap of both genotypes (Brown and Jones, 1971). Furthermore, ‘Rutgers’ was more efficient than ‘T3238’ in translocating 10B from the root to the shoot (Bellaloui and Brown, 1998). For grafted trees, in addition to functioning in B uptake by roots, the rootstock may also play a role in B translocation from the root to the scion (Papadakis et al., 2003; Wojcik et al., 2003; Boaretto et al., 2008; Sheng et al., 2009b; Wang et al., 2014). Under B-deficiency conditions, the ratio of B concentration in the scion stem to the rootstock stem increased as the B efficiency of citrus combinations increased (Wang et al., 2014). This implies, at least in part, that B-efficient grafted combinations possess a greater ability to translocate B from the rootstock (root) to the scion (shoot). The B translocation efficiency in trees is likely related to water use efficiency in rootstocks and scions, and to connectivity in the grafting region, presumably due to the B transport in xylem associated with aquaporins (Wimmer and Eichert, 2013). Compared to the B-inefficient citrus rootstock trifoliate orange, the B-inefficient genotype Carrizo citrange is a vigorous rootstock that usually has a higher vessel diameter and hydraulic conductance (Saeed et al., 2010; Mei et al., 2011; Zhou et al., 2014). However, whether B translocation efficiency is associated with xylem vessel characteristics and water use efficiency still needs to be explored. At the molecular level, BOR1 proteins have been identified as B efflux transporters that are involved in B xylem translocation in the roots of Arabidopsis (Takano et al., 2001), rice (Nakagawa et al., 2007) and maize (Chatterjee et al., 2014). The strong expression of AtBOR1 improved growth in both Arabidopsis (Miwa et al., 2006) and tomato (Uraguchi et al., 2014) under B-deficiency conditions. These results suggest that the greater accumulation of B efflux transporter could increase tolerance to B starvation through an enhanced B xylem translocation process. This is further supported by grape VvBOR1 overexpression restoring the wild-type phenotype in an Arabidopsis bor1-3 mutant (Pérez-Castro et al., 2012) and citrus CmBOR1 overexpression increasing the tolerance to B deficiency in Arabidopsis (Cañon et al., 2013). Moreover, we recently found that ten aquaporin genes of roots were up-regulated in B-efficient Carrizo citrange, but only two of them were up-regulated in B-inefficient trifoliate orange at 24 h after B-deficiency treatment (Zhou et al., 2015). This result supports the proposal that the B-dependent regulation of aquaporins could affect the water status of the whole plant (Wimmer and Eichert, 2013). The plant aquaporin family functions in membrane channels and can facilitate the transport of water and other low molecular weight substances in the xylem (Ishibashi et al., 2011). Therefore, under B-deficiency conditions, Carrizo citrange may have a greater ability to absorb B and water and to transport them via the xylem to the shoot than trifoliate orange, thus tolerating B deficiency.
In contrast to the xylem, B retranslocation in the phloem can be achieved by two pathways: (i) xylem-to-phloem transfer along the stem, and (ii) retranslocation from the leaves (source) to the flowers or fruits (sink). Xylem-to-phloem transfer along the stem is particularly important for the B nutrition of plants during reproductive stage, due to the higher B demand and the lower transpiration rate of reproductive tissues than vegetative organs (Shelp et al., 1998; Huang et al., 2001). Moreover, B efficiency varies with plant growth period. For example, the wheat cultivar ‘Fang 60’ was B-efficient during reproductive growth, but vegetatively B-inefficient; the cultivar ‘SW41’ had the opposite trend of B efficiency compared with ‘Fang 60’ (Rerkasem and Jamjod, 1997). These results suggest that B efficiency of the plants at reproductive stage may be associated with the capacity for xylem-to-phloem transfer along the stem. The other pathway is the B retranslocation from the leaves to the flowers or fruits. After arriving at the source leaves, B can be retranslocated into the sinks (e.g., flowers, fruits) via the phloem by forming complexes with hydroxyl groups (the main pathway of B retranslocation; Brown and Shelp, 1997); otherwise, B will leak back into the xylem due to its high membrane permeability (Oertli and Richardson, 1970). Boron retranslocation is more marked at low B supply than at adequate or high B supply (Liakopoulos et al., 2009). Significant 10B retranslocation was found in the new stem and needles of Scots pine and Norway spruce seedlings after applying 10B to old needles, presumably as a consequence of B forming complexes with pinitol or inositol (Lehto et al., 2004a). In the olive tree leaf, mannitol may be involved in the promotion of B retranslocation under B limitation (Liakopoulos et al., 2009). Therefore, B retranslocation in the phloem is probably associated with the type and abundance of hydroxyl-bearing moieties, such as sugar alcohols (Perica et al., 2001a; Liakopoulos et al., 2009; Liu et al., 2014b). At the molecular level, B retranslocation may be associated with five B transporters (AtBOR6, AtBOR7, OsBOR4, VvBOR1, and AtNIP6;1) due to their expression in nodal regions of shoots or flowers (Tanaka et al., 2008; Fujiwara et al., 2010; Pérez-Castro et al., 2012; Tanaka et al., 2013). However, only AtNIP6;1 and OsBOR4 have been functionally identified (Tanaka et al., 2008, 2013). AtNIP6;1 is highly expressed in nodal regions of shoots, particularly the phloem. The nip6;1 mutants exhibit reduced expansion and low B concentrations of young rosette leaves under B-limited conditions (Tanaka et al., 2008). OsBOR4 is specifically expressed in pollen, and bor4 mutants show fertilization defect as a consequence of the reduced pollen tube elongation (Tanaka et al., 2013). These results suggest that AtNIP6;1 and OsBOR4 may function in B distribution into growing leaves or flowers. In addition, AtNIP6;1 may be responsible for xylem-to-phloem transfer of B due to its predominant expression in the phloem of the nodes. Moreover, B retranslocation has further been partly revealed by the transgenic lines of sorbitol-related gene, S6PDH. This gene controls the activity of sorbitol-6-phosphate dehydrogenase, a key enzyme for sorbitol biosynthesis (Bellaloui et al., 2003). The overexpression of S6PDH could improve the retranslocation of B in plants by increasing sorbitol biosynthesis and thus enhance the tolerance to B deficiency in tobacco (Nicotiana tabacum; Brown et al., 1999) and rice (Bellaloui et al., 2003).
In trees, differences in B retranslocation efficiency may exist among genotypes within one species. Mattiello et al. (2009) found that in B-deficient eucalypt, the ratio of 10B: 11B in 10B-applied leaves decreased from 3.225 at day 1–1.492 at day 17 in clone 129 (Eucalyptus grandis ×E. urophylla), whereas that ratio only decreased from 2.759–1.900 in clone 68 (E. grandis ×E. urophylla). Accordingly, the ratio in other leaves remained stable at 0.245–0.254 in clone 68, whereas in clone 129, the ratio increased from 0.245 to 0.425. This result suggests that clone 129 is better able to retranslocate B to other parts after foliar application under B-deficiency conditions. Similarly, we observed that two orange scion cultivars with the same rootstock showed a different growth performance under B-deficiency conditions, likely due to the differences in B retranslocation efficiency (Sheng et al., 2009a). Although it is widely accepted that different degrees of B retranslocation among species are related to the level of polyols in the phloem (Brown and Shelp, 1997), little research on the B-polyol relationship among genotypes within a species has been conducted. Further studies are needed to examine the relationship between B retranslocation and polyols among genotypes within one species. Here, four approaches are recommended to assess the B retranslocation efficiency: (i) to evaluate B retranslocation by applying the stable isotope 10B in aerial plant parts (Brown et al., 1992; Lehto et al., 2004a; Leite et al., 2007); (ii) to identify the types of hydroxyl groups in leaf tissue and the phloem sap (Liakopoulos et al., 2009); (iii) to isolate and characterize the B-polyol complexes from leaf tissue and phloem sap (Hu et al., 1997; Penn et al., 1997; Stangoulis et al., 2010); and (iv) to compare B retranslocation between polyol-related trangenic mutant and wild type (Brown et al., 1999; Bellaloui et al., 2003).
After long-distance transport, B enters into the targeted tissue, where it is required for the construction of the cell wall (O’Neill et al., 2001) and membranes (Voxeur and Fry, 2014) as well as for the metabolism of the cytoplasm (Brown et al., 2002). This process is known as B utilization. Boron use efficiency is related not only to the pectin content and composition of the cell wall (Hu et al., 1996; Kakegawa et al., 2005; Pan et al., 2012; Liu et al., 2013a), but also the cell membrane composition (Dordas and Brown, 2000). In general, B-efficient tree cultivars have a lower concentration of cell wall B at B-adequate supply but similar or greater concentration of cell wall B at B-limited supply, as compared to B-inefficient cultivars (Liu et al., 2013a; Wang et al., 2014). That is, B-efficient cultivars may possess a higher proportion of non-cell wall B which is available for cytoplasmic metabolism under B-adequate conditions, but a greater proportion of cell wall B which is required for the structure of cell wall under B-limited conditions (Hu et al., 1996; Pan et al., 2012). Moreover, the pectin composition of B-efficient cultivars differs from that of B-inefficient cultivars. For example, B-efficient Carrizo citrange had a higher concentration of CDTA-soluble pectin than B-inefficient trifoliate orange under B-limited conditions (Liu et al., 2013a). Boron use efficiency may also be associated with the cell membrane composition. The Arabidopsis chs1-1 mutant, which has a lower proportion of sterols than the wild type, showed a 30% higher B uptake, whereas the act1-1 mutant, which has an increased percentage of longer fatty acids, exhibited a 35% lower B uptake than the wild type (Dordas and Brown, 2000). At the molecular level, Miwa et al. (2013) demonstrated that the proportion of cross-linked RG-II in Arabidopsis bor2-1 and bor2-2 mutants (∼42.5 and ∼45.7%) was significantly lower than that of both wild type (∼54.0%) and bor1-3 mutant (∼52.8%) plants under B limitation. These results may suggest that B transport by the AtBOR2 protein from the symplast to the apoplast may be required for the effective cross linking of RG-II in the cell wall under B deficiency. Transgenic lines with enhanced expression of BOR2 showed improved root growth and better fertility under low B conditions, suggesting the potential utility of BOR2 expression in agricultural applications (Takada et al., 2014).
In summary, at a physiological level, B efficiency in trees is mainly attributed to four mechanisms: (i) the ability to absorb B from the soil/medium, which depends on root morphology and mycorrhiza; (ii) B translocation from root to shoot as indicated by the composition of root cell sap and xylem exudate and likely influenced by xylem vessel characteristics and water use efficiency; (iii) B retranslocation through xylem-to-phloem transfer and formation of complexes with hydroxyl groups in phloem; and (iv) the B requirement in cell wall construction and cell membrane composition. At a molecular level, the tolerance to B deficiency can be improved by the higher or stronger expression of NIPs, BORs, and S6PDH to facilitate B uptake, B translocation and retranslocation, as well as B utilization processes. These molecular results indicate that genetically modified trees with B-deficiency-tolerance-related genes may be useful in forestry or other tree industries in the future. Furthermore, the bor1 bor2 double mutants exhibited more severe growth defects under B-limited conditions than bor1 or bor2 single mutants in Arabidopsis (Miwa et al., 2013), indicating that B-deficiency-tolerance-related genes are probably dosage-dependent. That is, the differences in B efficiency probably originate from a combined effect of the four mechanisms mentioned above. However, previous studies were mainly limited to one single mechanism of B efficiency. Consequently, more systematic study is needed on the B deficiency tolerance mechanisms in trees, including uptake, translocation, retranslocation, and utilization, spanning investigations from the physiological to the molecular level.
Boron deficiency is frequently observed in woody plants. An adequate B supply for cultivated trees can be of great economic importance, contributing significantly to the yield and quality of fruit and forest trees. From a practical view point, there is a need to focus research on the significance of B reserves, which is especially important in trees, for which there may be no early warning symptoms of B deficiency. Species or genotypes with different B efficiency have provided ideal experimental material to elucidate some puzzling aspects of the mechanisms for tolerance to B deficiency. Recently, great progress has been achieved in uncovering the molecular mechanisms of B efficiency in herbaceous plants, which makes possible further exploration of the mechanisms of B deficiency tolerance in woody plants.
The authors declare that the research was conducted in the absence of any commercial or financial relationships that could be construed as a potential conflict of interest.
This review was financially supported by the National Modern Citrus Industry System, the Ministry of Education Innovation Team (IRT13065), the National Natural Science Foundation of China (NO. 31071761 and 31272121). The authors would like to thank Dr. Richard Bell and the reviewers for their valuable comments and suggestions to improve the quality of the original manuscript.
Abreu, I., Poza, L., Bonilla, I., and Bolaños, L. (2014). Boron deficiency results in early repression of a cytokinin receptor gene and abnormal cell differentiation in the apical root meristem of Arabidopsis thaliana. Plant Physiol. Biochem. 77, 117–121. doi: 10.1016/j.plaphy.2014.02.008
Agarwala, S., Nautiyal, B., Chatterjee, C., and Sharma, C. (1988). Manganese, zinc and boron deficiency in mango. Sci. Hortic. 35, 99–107. doi: 10.1016/0304-4238(88)90041-6
An, J. C., Liu, Y. Z., Yang, C. Q., Zhou, G. F., Wei, Q. J., and Peng, S. A. (2012). Isolation and expression analysis of CiNIP5, a citrus boron transport gene involved in tolerance to boron deficiency. Sci. Hortic. 142, 149–154. doi: 10.1016/j.scienta.2012.05.013
Aphalo, P. J., Schoettle, A. W., and Lehto, T. (2002). Leaf life span and the mobility of “non-mobile” mineral nutrients-the case of boron in conifers. Silva Fennica 36, 671–680. doi: 10.14214/sf.532
Asomaning, E., and Kwakwa, R. (1965). “Boron deficiency symptoms in cocoa fruits,” in Proceedings of the 2nd International Cocoa Research Conference, Bahia, 39–42.
Barr, R., Böttger, M., and Crane, F. (1993). The effect of boron on plasma membrane electron transport and associated proton secretion by cultured carrot cells. Biochem. Mol. Biol. Int. 31, 31–39.
Bassil, E., Hu, H., and Brown, P. H. (2004). Use of phenylboronic acids to investigate boron function in plants. Possible role of boron in transvacuolar cytoplasmic strands and cell-to-wall adhesion. Plant Physiol. 136, 3383–3395. doi: 10.1104/pp.104.040527
Bell, R. W. (2000). Temporary nutrient deficiency: a difficult case for diagnosis and prognosis by plant analysis. Commun. Soil Sci. Plant Anal. 31, 1847–1861. doi: 10.1080/00103620009370542
Bell, R. W., and Dell, B. (2008). Micronutrients for Sustainable Food, Feed, Fibre and Bioenergy Production. Paris: International Fertilizer Industry Association (IFA).
Bellaloui, N., and Brown, P. H. (1998). Cultivar differences in boron uptake and distribution in celery (Apium graveolens), tomato (Lycopersicon esculentum) and wheat (Triticum aestivum). Plant Soil 198, 153–158. doi: 10.1023/A:1004343031242
Bellaloui, N., Yadavc, R. C., Chern, M. S., Hu, H., Gillen, A. M., Greve, C., et al. (2003). Transgenically enhanced sorbitol synthesis facilitates phloem-boron mobility in rice. Physiol. Plant. 117, 79–84. doi: 10.1034/j.1399-3054.2003.1170110.x
Blevins, D. G., and Lukaszewski, K. M. (1998). Boron in plant structure and function. Annu. Rev. Plant Biol. 49, 481–500. doi: 10.1146/annurev.arplant.49.1.481
Blevins, D. G., Scrivner, C., Reinbott, T., and Schon, M. (1996). Foliar boron increases berry number and yield of two highbush blueberry cultivars in Missouri. J. Plant Nutr. 19, 99–113. doi: 10.1080/01904169609365110
Boaretto, R. M., Quaggio, J. A., Mattos, D., Muraoka, T., and Boaretto, A. E. (2011). Boron uptake and distribution in field grown citrus trees. J. Plant Nutr. 34, 839–849. doi: 10.1080/01904167.2011.544353
Boaretto, R. M., Quaggio, J. A., Mourão Filho, F. A., Giné, M. F., and Boaretto, A. E. (2008). Absorption and mobility of boron in young citrus plants. Commun. Soil Sci. Plant Anal. 39, 2501–2514. doi: 10.1080/00103620802358383
Broschat, T. K. (2005). Boron Deficiency in Palms. ENH1012. Fort Lauderdale, FL: University of Florida Extension Service, 6.
Brown, J., and Jones, W. (1971). Differential transport of boron in tomato (Lycopersicon esculenlum Mill.). Physiol. Plant. 25, 279–282. doi: 10.1111/j.1399-3054.1971.tb01443.x
Brown, P. H., Bellaloui, N., Hu, H., and Dandekar, A. (1999). Transgenically enhanced sorbitol synthesis facilitates phloem boron transport and increases tolerance of tobacco to boron deficiency. Plant Physiol. 119, 17–20. doi: 10.1104/pp.119.1.17
Brown, P. H., Bellaloui, N., Wimmer, M. A., Bassil, E., Ruiz, J., Hu, H., et al. (2002). Boron in plant biology. Plant Biol. 4, 205–223. doi: 10.1055/s-2002-25740
Brown, P. H., and Hu, H. (1998). Phloem boron mobility in diverse plant species. Plant Biol. 111, 331–335. doi: 10.1111/j.1438-8677.1998.tb00717.x
Brown, P. H., Picchioni, G., Jenkin, M., and Mu, H. (1992). Use of ICP-MS and 10B to trace the movement of boron in plants and soil. Commun. Soil. Sci. Plant Anal. 23, 2781–2807. doi: 10.1080/00103629209368773
Brown, P. H., and Shelp, B. J. (1997). Boron mobility in plants. Plant Soil 193, 85–101. doi: 10.1023/A:1004211925160
Burrell, A. (1940). The boron deficiency disease of apple. Ext. Bull. Cornell Agric. Exp. Stat. 428, 28.
Cakmak, I., Kurz, H., and Marschner, H. (1995). Short-term effects of boron, germanium and high light intensity on membrane permeability in boron deficient leaves of sunflower. Physiol. Plant. 95, 11–18. doi: 10.1111/j.1399-3054.1995.tb00801.x
Cakmak, I., and Römheld, V. (1997). Boron deficiency-induced impairments of cellular functions in plants. Plant Soil 193, 71–83. doi: 10.1023/A:1004259808322
Camacho-Cristóbal, J. J., Herrera-Rodríguez, M. B., Beato, V. M., Rexach, J., Navarro-Gochicoa, M. T., Maldonado, J. M., et al. (2008a). The expression of several cell wall-related genes in Arabidopsis roots is down-regulated under boron deficiency. Environ. Exp. Bot. 63, 351–358. doi: 10.1016/j.envexpbot.2007.12.004
Camacho-Cristóbal, J. J., Rexach, J., and Gonzalez-Fontes, A. (2008b). Boron in plants: deficiency and toxicity. J. Integr. Plant Biol. 50, 1247–1255. doi: 10.1111/j.1744-7909.2008.00742.x
Camacho-Cristóbal, J. J., Martín-Rejano, E. M., Herrera-Rodríguez, M. B., Navarro-Gochicoa, M. T., Rexach, J., and González-Fontes, A. (2015). Boron deficiency inhibits root cell elongation via an ethylene/auxin/ROS-dependent pathway in Arabidopsis seedlings. J. Exp. Bot. 66, 3831–3840. doi: 10.1093/jxb/erv186
Cañon, P., Aquea, F., Rodríguez-Hoces de La Guardia, A., and Arce-Johnson, P. (2013). Functional characterization of Citrus macrophylla BOR1 as a boron transporter. Physiol. Plant. 149, 329–339. doi: 10.1111/ppl.12037
Chatterjee, M., Tabi, Z., Galli, M., Malcomber, S., Buck, A., Muszynski, M., et al. (2014). The boron efflux transporter ROTTEN EAR is required for maize Inflorescence development and fertility. Plant Cell 26, 2962–2977. doi: 10.1105/tpc.114.125963
Christensen, L., Beede, R., and Peacock, W. (2006). Fall foliar sprays prevent boron-deficiency symptoms in grapes. Calif. Agric. 60, 100–103. doi: 10.3733/ca.v060n02p100
Cooling, E., and Jones, B. (1970). The importance of boron and NPK fertilizers to eucalyptus in the southern province. Zambia. East Afr. Agric. For. J. 36, 185–194.
Dannel, F., Pfeffer, H., and Römheld, V. (2002). Update on boron in higher plants-uptake, primary translocation and compartmentation. Plant Biol. 4, 193–204. doi: 10.1055/s-2002-25730
Dell, B., and Huang, L. (1997). Physiological response of plants to low boron. Plant Soil 193, 103–120. doi: 10.1023/A:1004264009230
Dordas, C., and Brown, P. H. (2000). Permeability of boric acid across lipid bilayers and factors affecting it. J. Membr. Biol. 175, 95–105. doi: 10.1007/s002320001058
Dordas, C., and Brown, P. H. (2001). Evidence for channel mediated transport of boric acid in squash (Cucurbita pepo). Plant Soil 235, 95–103. doi: 10.1023/A:1011837903688
Du, C. W., Wang, Y. H., Xu, F. S., Yang, Y. H., and Wang, H. Y. (2002). Study on the physiological mechanism of boron utilization efficiency in rape cultivars. J. Plant Nutr. 25, 231–244. doi: 10.1081/PLN-100108832
Durbak, A. R., Phillips, K. A., Pike, S., O’Neill, M. A., Mares, J., Gallavotti, A., et al. (2014). Transport of boron by the tassel-less1 aquaporin is critical for vegetative and reproductive development in maize. Plant Cell 26, 2978–2995. doi: 10.1105/tpc.114.125898
Eichert, T., and Goldbach, H. E. (2010). Transpiration rate affects the mobility of foliar-applied boron in Ricinus communis L. cv. Impala. Plant Soil 328, 165–174. doi: 10.1007/s11104-009-0094-y
Fitzpatrick, K. L., and Reid, R. J. (2009). The involvement of aquaglyceroporins in transport of boron in barley roots. Plant Cell Environ. 32, 1357–1365. doi: 10.1111/j.1365-3040.2009.02003.x
Fleischer, A., O’Neill, M. A., and Ehwald, R. (1999). The pore size of non-graminaceous plant cell walls is rapidly decreased by borate ester cross-linking of the pectic polysaccharide rhamnogalacturonan II. Plant Physiol. 121, 829–838. doi: 10.1104/pp.121.3.829
Fujiwara, T., Tanaka, M., and Miwa, K. (2010). “Optimisation of nutrient transport processes by plants-boron transport as an example,” in Proceedings of the 19th World Congress of Soil Science: Soil Solutions for a Changing World, eds R. J. Gilkes and N. Prakongkep (Brisbane, QLD: IUSS), 26–29.
Ganie, M. A., Akhter, F., Bhat, M., Malik, A., Junaid, J. M., Shah, M. A., et al. (2013). Boron-a critical nutrient element for plant growth and productivity with reference to temperate fruits. Curr. Sci. 104, 76–85.
Goh, K. J., Gan, H. H., Kee, K. K., Chew, P. S., and Teoh, K. C. (2007). “Boron requirement and distribution in the oil palm (Elaeis guineensis Jacq.) and some implications on manuring practices,” in Advances in Plant and Animal Boron Nutrition, eds F. S. Xu, H. E. Goldbach, P. H. Brown, R. W. Bell, T. Fujiwara, and C. D. Hunt (Amsterdam: Springer), 189–202.
Goldbach, H. E., and Wimmer, M. A. (2007). Boron in plants and animals: is there a role beyond cell wall structure? J. Plant Nutr. Soil Sci. 170, 39–48. doi: 10.1002/jpln.200625161
Goldbach, H. E., Yu, Q., Wingender, R., Schulz, M., Wimmer, M. A., Findeklee, P., et al. (2001). Rapid response reactions of roots to boron deprivation. J. Plant Nutr. Soil Sci. 164, 173–181.
González-Fontes, A., Navarro-Gochicoa, M. T., Camacho-Cristóbal, J. J., Herrera-Rodríguez, M. B., Quiles-Pando, C., and Rexach, J. (2014). Is Ca2+ involved in the signal transduction pathway of boron deficiency? New hypotheses for sensing boron deprivation. Plant Sci. 217, 135–139. doi: 10.1016/j.plantsci.2013.12.011
González-Fontes, A., Rexach, J., Quiles-Pando, C., Herrera-Rodríguez, M. B., Camacho-Cristóbal, J. J., and Navarro-Gochicoa, M. T. (2013). Transcription factors as potential participants in the signal transduction pathway of boron deficiency. Plant Signal. Behav. 8:e26114. doi: 10.4161/psb.26114
Goujon, T., Minic, Z., El Amrani, A., Lerouxel, O., Aletti, E., Lapierre, C., et al. (2003). AtBXL1, a novel higher plant (Arabidopsis thaliana) putative beta-xylosidase gene, is involved in secondary cell wall metabolism and plant development. Plant J. 33, 677–690. doi: 10.1046/j.1365-313X.2003.01654.x
Graham, R. D. (1984). Breeding for nutritional characteristics in cereals. Adv. Plant Nutr. 1, 57–102.
Hajiboland, R., Bahrami-Rad, S., and Bastani, S. (2013a). Phenolics metabolism in boron-deficient tea [Camellia sinensis (L.) O. Kuntze] plants. Acta Biol. Hung. 64, 196–206. doi: 10.1556/ABiol.64.2013.2.6
Hajiboland, R., Bahrami-Rad, S., Bastani, S., Tolrà, R., and Poschenrieder, C. (2013b). Boron re-translocation in tea (Camellia sinensis (L.) O. Kuntze) plants. Acta Physiol. Plant. 35, 2373–2381. doi: 10.1007/s11738-013-1272-3
Hajiboland, R., Bastani, S., and Bahrami-Rad, S. (2011a). Effect of light intensity on photosynthesis and antioxidant defense in boron deficient tea plants. Acta Biol. Szeg. 55, 265–272.
Hajiboland, R., Bastani, S., and Bahrami-Rad, S. (2011b). Photosynthesis, nitrogen metabolism and antioxidant defense system in B-deficient tea (Camellia sinensis (L.) O. Kuntze) plants. J. Sci. I. R. Iran 22, 311–320.
Han, J., Zhou, G. F., Li, Q. H., Liu, Y. Z., and Peng, S. A. (2012). Effects of magnesium, iron, boron deficiency on the growth and nutrition absorption of four major citrus rootstocks. Acta Horticulturae Sin. 39, 2105–2112.
Han, S., Chen, L. S., Jiang, H. X., Smith, B. R., Yang, L. T., and Xie, C. Y. (2008). Boron deficiency decreases growth and photosynthesis, and increases starch and hexoses in leaves of citrus seedlings. J. Plant Physiol. 165, 1331–1341. doi: 10.1016/j.jplph.2007.11.002
Hanaoka, H., Uraguchi, S., Takano, J., Tanaka, M., and Fujiwara, T. (2014). OsNIP3;1, a rice boric acid channel, regulates boron distribution and is essential for growth under boron-deficient conditions. Plant J. 78, 890–902. doi: 10.1111/tpj.12511
Hanson, E. J. (1991). Sour cherry trees respond to foliar boron applications. HortScience 26, 1142–1145.
Hu, H., Brown, P. H., and Labavitch, J. M. (1996). Species variability in boron requirement is correlated with cell wall pectin. J. Exp. Bot. 47, 227–232. doi: 10.1093/jxb/47.2.227
Hu, H., Penn, S. G., Lebrilla, C. B., and Brown, P. H. (1997). Isolation and characterization of soluble boron complexes in higher plants (The mechanism of phloem mobility of boron). Plant Physiol. 113, 649–655. doi: 10.1104/pp.113.2.649
Huang, L., Bell, R. W., and Dell, B. (2001). Boron supply into wheat (Triticum aestivum L. cv. Wilgoyne) ears whilst still enclosed within leaf sheaths. J. Exp. Bot. 52, 1731–1738. doi: 10.1093/jexbot/52.361.1731
Ishibashi, K., Kondo, S., Hara, S., and Morishita, Y. (2011). The evolutionary aspects of aquaporin family. Am. J. Integr. Comp. Physiol. 300, R566–R576. doi: 10.1152/ajpregu.90464
Jayasekara, K., and Loganathan, P. (1988). Boron deficiency in young coconut (Cocos nucifera L.) in Sri Lanka symptoms and corrective measures. Cocos 6, 31–37. doi: 10.4038/cocos.v6i1.2053
Kakegawa, K., Ishii, T., and Matsunaga, T. (2005). Effects of boron deficiency in cell suspension cultures of Populus alba L. Plant Cell Rep. 23, 573–578. doi: 10.1007/s00299-004-0878-8
Kato, Y., Miwa, K., Takano, J., Wada, M., and Fujiwara, T. (2009). Highly boron deficiency-tolerant plants generated by enhanced expression of NIP5;1, a boric acid channel. Plant Cell Physiol. 50, 58–66. doi: 10.1093/pcp/pcn168
Kilpeläinen, J., Räisänen, M., Mehtätalo, L., Sutinen, S., Rummukainen, A., Repo, T., et al. (2013). The longevity of Norway spruce responses to boron fertilisation. For. Ecol. Manag. 307, 90–100. doi: 10.1016/j.foreco.2013.06.054
Kobayashi, M., Matoh, T., and Azuma, J. (1996). Two chains of rhamnogalacturonan II are cross-linked by borate-diol ester bonds in higher plant cell walls. Plant Physiol. 110, 1017–1020. doi: 10.1104/pp.110.3.1017
Konsaeng, S., Dell, B., and Rerkasem, B. (2005). A survey of woody tropical species for boron retranslocation. Plant Prod. Sci. 8, 338–341. doi: 10.1626/pps.8.338
Kumar, R. (2011). Boron deficiency disorders in mango (Mangifera indica): field screening, nutrient composition and amelioration by boron application. Indian J. Agric. Sci. 51, 751–754.
Lehto, T., Lavola, A., Julkunen-Tiitto, R., and Aphalo, P. J. (2004a). Boron retranslocation in Scots pine and Norway spruce. Tree Physiol. 24, 1011–1017. doi: 10.1093/treephys/24.9.1011
Lehto, T., Räisänen, M., Lavola, A., Julkunen-Tiitto, R., and Aphalo, P. J. (2004b). Boron mobility in deciduous forest trees in relation to their polyols. New Phytol. 163, 333–339. doi: 10.1111/j.1469-8137.2004.01105.x
Lehto, T., Ruuhola, T., and Dell, B. (2010). Boron in forest trees and forest ecosystems. For. Ecol. Manag. 260, 2053–2069. doi: 10.1016/j.foreco.2010.09.028
Leite, V. M., Brown, P. H., and Rosolem, C. A. (2007). Boron translocation in coffee trees. Plant Soil 290, 221–229. doi: 10.1007/s11104-006-9154-8
Liakopoulos, G., Stavrianakou, S., Filippou, M., Fasseas, C., Tsadilas, C., Drossopoulos, I., et al. (2005). Boron remobilization at low boron supply in olive (Olea europaea) in relation to leaf and phloem mannitol concentrations. Tree Physiol. 25, 157–165. doi: 10.1093/treephys/25.2.157
Liakopoulos, G., Stavrianakou, S., Nikolopoulos, D., Karvonis, E., Vekkos, K. A., Psaroudi, V., et al. (2009). Quantitative relationships between boron and mannitol concentrations in phloem exudates of Olea europaea leaves under contrasting boron supply conditions. Plant Soil 323, 177–186. doi: 10.1007/s11104-009-9923-2
Liu, G. D., Dong, X. C., Liu, L. C., Wu, L. S., Peng, S. A., and Jiang, C. C. (2014a). Boron deficiency is correlated with changes in cell wall structure that lead to growth defects in the leaves of navel orange plants. Sci. Hortic. 176, 54–62. doi: 10.1016/j.scienta.2014.06.036
Liu, G. D., Dong, X. C., Liu, L. C., Wu, L. S., Peng, S. A., and Jiang, C. C. (2014b). Metabolic profiling reveals altered pattern of central metabolism in navel orange plants as a result of boron deficiency. Physiol. Plant 153, 513–524. doi: 10.1111/ppl.12279
Liu, G. D., Jiang, C. C., and Wang, Y. H. (2011). Distribution of boron and its forms in young ‘Newhall’ navel orange (Citrus sinensis Osb.) plants grafted on two rootstocks in response to deficient and excessive boron. Soil Sci. Plant Nutr. 57, 93–104. doi: 10.1080/00380768.2010.551299
Liu, G. D., Wang, R. D., Liu, L. C., Wu, L. S., and Jiang, C. C. (2013a). Cellular boron allocation and pectin composition in two citrus rootstock seedlings differing in boron-deficiency response. Plant Soil 370, 555–565. doi: 10.1007/s11104-013-1659-3
Liu, Y. Z., Li, S., Yang, C. Q., and Peng, S. A. (2013b). Effects of boron-deficiency on anatomical structures in the leaf main vein and fruit mesocarp of pummelo [Citrus grandis (L.) Osbeck]. Korean J. Hortic. Sci. 88, 693–700.
Liu, G. D., Wang, R. D., Wu, L. S., Peng, S. A., Wang, Y. H., and Jiang, C. C. (2012). Boron distribution and mobility in navel orange grafted on citrange and trifoliate orange. Plant Soil 360, 123–133. doi: 10.1007/s11104-012-1225-4
Lord, E. M., and Mollet, J. C. (2002). Plant cell adhesion: a bioassay facilitates discovery of the first pectin biosynthetic gene. Proc. Natl. Acad. Sci. U.S.A. 99, 15843–15845. doi: 10.1073/pnas.012685099
Lu, Y. B., Yang, L. T., Li, Y., Xu, J., Liao, T. T., Chen, Y. B., et al. (2014). Effects of boron deficiency on major metabolites, key enzymes and gas exchange in leaves and roots of Citrus sinensis seedlings. Tree Physiol. 34, 608–618. doi: 10.1093/treephys/tpu047
Ludbrook, W. (1940). Boron deficiency symptoms on pine seedlings in water culture. J. Counc. Sci. Ind. Res. 13, 186–190.
Martín-Rejano, E. M., Camacho-Cristobal, J. J., Herrera-Rodriguez, M. B., Rexach, J., Navarro-Gochicoa, M. T., and Gonzalez-Fontes, A. (2011). Auxin and ethylene are involved in the responses of root system architecture to low boron supply in Arabidopsis seedlings. Physiol. Plant. 142, 170–178. doi: 10.1111/j.1399-3054.2011.01459.x
Matoh, T., and Ochiai, K. (2005). Distribution and partitioning of newly taken-up boron in sunflower. Plant Soil 278, 351–360. doi: 10.1007/s11104-005-0372-2
Mattiello, E. M., Ruiz, H. A., Silva, I. R. D., Sarkis, J. E. S., Neves, J. C. L., and Pucci, M. M. (2009). Phloem mobility of boron in two eucalypt clones. Rev. Bras. Ciênc. Solo 33, 1695–1704. doi: 10.1590/S0100-06832009000600018
Mei, L., Sheng, O., Peng, S. A., Zhou, G. F., Wei, Q. J., and Li, Q. H. (2011). Growth, root morphology and boron uptake by citrus rootstock seedlings differing in boron-deficiency responses. Sci. Hortic. 129, 426–432. doi: 10.1016/j.scienta.2011.04.012
Miwa, K., and Fujiwara, T. (2010). Boron transport in plants: co-ordinated regulation of transporters. Ann. Bot. 105, 1103–1108. doi: 10.1093/aob/mcq044
Miwa, K., Takano, J., and Fujiwara, T. (2006). Improvement of seed yields under boron-limiting conditions through overexpression of BOR1, a boron transporter for xylem loading, in Arabidopsis thaliana. Plant J. 46, 1084–1091. doi: 10.1111/j.1365-313X.2006.02763.x
Miwa, K., Wakuta, S., Takada, S., Ide, K., Takano, J., Naito, S., et al. (2013). Roles of BOR2, a boron exporter, in cross linking of rhamnogalacturonan II and root elongation under boron limitation in Arabidopsis. Plant Physiol. 163, 1699–1709. doi: 10.1104/pp.113.225995
Mongrand, S., Stanislas, T., Bayer, E. M., Lherminier, J., and Simon-Plas, F. (2010). Membrane rafts in plant cells. Trends Plant Sci. 15, 656–663. doi: 10.1016/j.tplants.2010.09.003
Möttönen, M., Aphalo, P. J., and Lehto, T. (2001). Role of boron in drought resistance in Norway spruce (Picea abies) seedlings. Tree Physiol. 21, 673–681. doi: 10.1093/treephys/21.10.673
Moura, J., Prado, R., Benvindo, R., and Chaves Alencar, L. (2013). Applying boron to coconut palm plants: effects on the soil, on the plant nutritional status and on productivity boron to coconut palm trees. J. Soil Sci. Plant Nutr. 13, 79–85. doi: 10.4067/S0718-95162013005000008
Nachiangmai, D., Dell, B., Bell, R. W., Huang, L., and Rerkasem, B. (2004). Enhanced boron transport into the ear of wheat as a mechanism for boron efficiency. Plant Soil 264, 141–147. doi: 10.1023/B:PLSO.0000047757.39309.6f
Nakagawa, Y., Hanaoka, H., Kobayashi, M., Miyoshi, K., Miwa, K., and Fujiwara, T. (2007). Cell-type specificity of the expression of OsBOR1, a rice efflux boron transporter gene, is regulated in response to boron availability for efficient boron uptake and xylem loading. Plant Cell 19, 2624–2635. doi: 10.1105/tpc.106.049015
Nishina, M. S. (1991). Bumpy Fruit of Papaya as Related to Boron Deficiency (Commodity Fact Sheets. CFS-PA-4B). Honolulu (HI): University of Hawaii, 4.
Nyomora, A. M., Brown, P. H., and Krueger, B. (1999). Rate and time of boron application increase almond productivity and tissue boron concentration. HortScience 34, 242–245.
Nyomora, A. M., Brown, P. H., Pinney, K., and Polito, V. (2000). Foliar application of boron to almond trees affects pollen quality. J. Am. Soc. Hortic. Sci. 125, 265–270.
Oertli, J., and Richardson, W. (1970). The mechanism of boron immobility in plants. Physiol. Plant. 23, 108–116. doi: 10.1111/j.1399-3054.1970.tb06397.x
Ojeniyi, S., Egbe, N., and Omotosho, T. (1981). Boron nutrition of Amazon cocoa (Cacao theobroma) in Nigeria. I. Early results of fertilizer trials. Exp. Agric. 17, 399–402. doi: 10.1017/S0014479700011856
O’Neill, M. A., Eberhard, S., Albersheim, P., and Darvill, A. G. (2001). Requirement of borate cross-linking of cell wall rhamnogalacturonan II for Arabidopsis growth. Science 294, 846–849. doi: 10.1126/science.1062319
O’Neill, M. A., Ishii, T., Albersheim, P., and Darvill, A. G. (2004). Rhamnogalacturonan II: structure and function of a borate cross-linked cell wall pectic polysaccharide. Annu. Rev. Plant Biol. 55, 109–139. doi: 10.1146/annurev.arplant.55.031903.141750
O’Neill, M. A., Warrenfeltz, D., Kates, K., Pellerin, P., Doco, T., Darvill, A. G., et al. (1996). Rhamnogalacturonan-II, a pectic polysaccharide in the walls of growing plant cell, forms a dimer that is covalently cross-linked by a borate ester in vitro conditions for the formation and hydrolysis of the dimer. J. Biol. Chem. 271, 22923–22930. doi: 10.1074/jbc.271.37.22923
Pan, Y., Wang, Z. H., Yang, L., Wang, Z. F., Shi, L., Naran, R., et al. (2012). Differences in cell wall components and allocation of boron to cell walls confer variations in sensitivities of Brassica napus cultivars to boron deficiency. Plant Soil 354, 383–394. doi: 10.1007/s11104-011-1074-6
Papadakis, I., Dimassi, K., and Therios, I. (2003). Response of two citrus genotypes to six boron concentrations: concentration and distribution of nutrients, total absorption, and nutrient use efficiency. Aust. J. Agric. Res. 54, 571–580. doi: 10.1071/AR02163
Patnude, E., and Nelson, S. (2012). Boron Deficiency of Palms in Hawaii (Plant Disease; PD-83). Honolulu (HI): University of Hawaii, 6.
Penn, S. G., Hu, H., Brown, P. H., and Lebrilla, C. B. (1997). Direct analysis of sugar alcohol borate complexes in plant extracts by matrix-assisted laser desorption/ionization fourier transform mass spectrometry. Anal. Chem. 69, 2471–2477. doi: 10.1021/ac970101o
Pérez-Castro, R., Kasai, K., Gainza-Cortés, F., Ruiz-Lara, S., Casaretto, J. A., Peña-Cortés, H., et al. (2012). VvBOR1, the grapevine ortholog of AtBOR1, encodes an efflux boron transporter that is differentially expressed throughout reproductive development of Vitis vinifera L. Plant Cell Physiol. 53, 485–494. doi: 10.1093/pcp/pcs001
Perica, S., Bellaloui, N., Greve, C., Hu, H., and Brown, P. H. (2001a). Boron transport and soluble carbohydrate concentrations in olive. J. Am. Soc. Hortic. Sci. 126, 291–296.
Perica, S., Brown, P. H., Connell, J. H., Nyomora, A. M., Dordas, C., Hu, H., et al. (2001b). Foliar boron application improves flower fertility and fruit set of olive. HortScience 36, 714–716.
Picchioni, G. A., Weinbaum, S. A., and Brown, P. H. (1995). Retention and the kinetics of uptake and export of foliage-applied, labeled boron by apple, pear, prune, and sweet cherry leaves. J. Am. Soc. Hortic. Sci. 120, 28–35.
Pilbeam, D., and Kirkby, E. (1983). The physiological role of boron in plants. J. Plant Nutr. 6, 563–582. doi: 10.1080/01904168309363126
Pollard, A. S., Parr, A. J., and Loughman, B. C. (1977). Boron in relation to membrane function in higher plants. J. Exp. Bot. 28, 831–841. doi: 10.1093/jxb/28.4.831
Quiles-Pando, C., Rexach, J., Navarro-Gochicoa, M. T., Camacho-Cristóbal, J. J., Herrera-Rodríguez, M. B., and González-Fontes, A. (2013). Boron deficiency increases the levels of cytosolic Ca2+ and expression of Ca2+-related genes in Arabidopsis thaliana roots. Plant Physiol. Biochem. 65, 55–60. doi: 10.1016/j.plaphy.2013.01.004
Raja, M. E., Anil Kumar, S. C., and Raju, S. Y. (2005). Boron deficiency in mango (Mangifera indica L.): a cause delineation study in acidic soils of Maharashtra. Indian Soil Sci. Plant Nutr. 51, 751–754. doi: 10.1111/j.1747-0765.2005.tb00106.x
Raven, J. (1980). Short- and long-distance transport of boric acid in plants. New Phytol. 84, 231–249. doi: 10.1111/j.1469-8137.1980.tb04424.x
Reid, R. (2014). Understanding the boron transport network in plants. Plant Soil 385, 1–13. doi: 10.1007/s11104-014-2149-y
Rerkasem, B., and Jamjod, S. (1997). Genotypic variation in plant response to low boron and implications for plant breeding. Plant Soil 193, 169–180. doi: 10.1023/A:1004220226977
Rerkasem, B., and Jamjod, S. (2004). Boron deficiency in wheat: a review. Field Crops Res. 89, 173–186. doi: 10.1016/j.fcr.2004.01.022
Riikonen, J., Lehto, T., and Rikala, R. (2013). Effects of boron fertilization in the nursery or after planting on the performance of Norway spruce seedlings on boron-poor sites. New For. 44, 671–685. doi: 10.1007/s11056-013-9372-x
Rosolem, C. A., and Leite, V. M. (2007). Coffee leaf and stem anatomy under boron deficiency. Rev. Bras. Ciênc. Solo 31, 477–483. doi: 10.1590/S0100-06832007000300007
Ruuhola, T., Keinänen, M., Keski-Saari, S., and Lehto, T. (2011). Boron nutrition affects the carbon metabolism of silver birch seedlings. Tree Physiol. 31, 1251–1261. doi: 10.1093/treephys/tpr109
Ruuhola, T., and Lehto, T. (2014). Do ectomycorrhizas affect boron uptake in Betula pendula? Can. J. For. Res. 44, 1013–1019. doi: 10.1139/cjfr-2014-0115
Ryden, P., Sugimoto-Shirasu, K., Smith, A. C., Findlay, K., Reiter, W. D., and Mccann, M. C. (2003). Tensile properties of Arabidopsis cell walls depend on both a xyloglucan cross-linked microfibrillar network and rhamnogalacturonan II-borate complexes. Plant Physiol. 132, 1033–1040. doi: 10.1104/pp.103.021873
Saeed, M., Dodd, P. B., and Sohail, L. (2010). Anatomical studies of stems, roots and leaves of selected citrus rootstock varieties in relation to their vigour. J. Hortic. For. 2, 87–94.
Sánchez, E. E., and Righetti, T. L. (2005). Effect of postharvest soil and foliar application of boron fertilizer on the partitioning of boron in apple trees. HortScience 40, 2115–2117.
Scott, L., and Schrader, A. L. (1947). Effect of alternating conditions of boron nutrition upon growth and boron content of grape vines in sand culture. Plant Physiol. 22, 526. doi: 10.1104/pp.22.4.526
Shelp, B. J., Kitheka, A. M., Vanderpool, R. A., Van Cauwenberghe, O. R., and Spiers, G. A. (1998). Xylem-to-phloem transfer of boron in broccoli and lupin during early reproductive growth. Physiol. Plant. 104, 533–540. doi: 10.1034/j.1399-3054.1998.1040403.x
Shelp, B. J., Marentes, E., Kitheka, A. M., and Vivekanandan, P. (1995). Boron mobility in plants. Physiol. Plant. 94, 356–361. doi: 10.1023/a:1004211925160
Sheng, O., Song, S. W., Chen, Y. J., Peng, S. A., and Deng, X. X. (2009a). Effects of exogenous B supply on growth, B accumulation and distribution of two navel orange cultivars. Trees 23, 59–68. doi: 10.1007/s00468-008-0254-3
Sheng, O., Song, S. W., Peng, S. A., and Deng, X. X. (2009b). The effects of low boron on growth, gas exchange, boron concentration and distribution of ‘Newhall’ navel orange (Citrus sinensis Osb.) plants grafted on two rootstocks. Sci. Hortic. 121, 278–283. doi: 10.1016/j.scienta.2009.02.009
Shorrocks, V. M. (1997). The occurrence and correction of boron deficiency. Plant Soil 193, 121–148. doi: 10.1023/A:1004216126069
Simon-Plas, F., Perraki, A., Bayer, E., Gerbeau-Pissot, P., and Mongrand, S. (2011). An update on plant membrane rafts. Curr. Opin. Plant Biol. 14, 642–649. doi: 10.1016/j.pbi.2011.08.003
Smith, T. E., Asher, C. J., Stephenson, R. A., and Hetherington, S. E. (1997a). “Boron deficiency of avocado. 2. Effects on fruit size and ripening,” in Boron in Soils and Plants, eds R. W. Bell and B. Rerkasem (Amsterdam: Springer), 135–137.
Smith, T. E., Stephenson, R. A., Asher, C. J., and Hetherington, S. E. (1997b). “Boron deficiency of avocado. 1. Effects on pollen viability and fruit set,” in Boron in Soils and Plants, eds R. W. Bell and B. Rerkasem (Amsterdam: Springer), 131–133.
Spiegel-Roy, P., and Goldschmidt, E. E. (1996). The Biology of Citrus. Cambridge: Cambridge University Press.
Stangoulis, J. C., Brown, P. H., Bellaloui, N., Reid, R. J., and Graham, R. D. (2001). The efficiency of boron utilisation in canola. Funct. Plant Biol. 28, 1109–1114. doi: 10.1071/PP00164
Stangoulis, J. C., Tate, M., Graham, R. D., Bucknall, M., Palmer, L., Boughton, B., et al. (2010). The mechanism of boron mobility in wheat and canola phloem. Plant Physiol. 153, 876–881. doi: 10.1104/pp.110.155655
Stone, E., Hollis, C., and Barnard, E. (1982). Boron deficiency in a Southern pine nursery. South. J. Appl. For. 6, 108–112.
Sutinen, S., Aphalo, P. J., and Lehto, T. (2007). Does timing of boron application affect needle and bud structure in Scots pine and Norway spruce seedlings? Trees 21, 661–670. doi: 10.1007/s00468-007-0158-7
Sutinen, S., Vuorinen, M., and Rikala, R. (2006). Developmental disorders in buds and needles of mature Norway spruce, Picea abies (L.) Karst., in relation to needle boron concentrations. Trees 20, 559–570. doi: 10.1007/s00468-006-0071-5
Takada, S., Miwa, K., Omori, H., Fujiwara, T., Naito, S., and Takano, J. (2014). Improved tolerance to boron deficiency by enhanced expression of the boron transporter BOR2. Soil Sci. Plant Nutr. 60, 341–348. doi: 10.1080/00380768.2014.881705
Takano, J., Miwa, K., Yuan, L., Von Wirén, N., and Fujiwara, T. (2005). Endocytosis and degradation of BOR1, a boron transporter of Arabidopsis thaliana, regulated by boron availability. Proc. Natl. Acad. Sci. U.S.A. 102, 12276–12281. doi: 10.1073/pnas.0502060102
Takano, J., Tanaka, M., Toyoda, A., Miwa, K., Kasai, K., Fuji, K., et al. (2010). Polar localization and degradation of Arabidopsis boron transporters through distinct trafficking pathways. Proc. Natl. Acad. Sci. U.S.A. 107, 5220–5225. doi: 10.1073/pnas.0910744107
Takano, J., Wada, M., Ludewig, U., Schaaf, G., Von Wirén, N., and Fujiwara, T. (2006). The Arabidopsis major intrinsic protein NIP5;1 is essential for efficient boron uptake and plant development under boron limitation. Plant Cell 18, 1498–1509. doi: 10.1105/tpc.106.041640
Takano, J., Yamagami, M., Noguchi, K., Hayashi, H., and Fujiwara, T. (2001). Preferential translocation of boron to young leaves in Arabidopsis thaliana regulated by the BOR1 gene. Soil Sci. Plant Nutr. 47, 345–357. doi: 10.1080/00380768.2001.10408398
Tanaka, M., Wallace, I. S., Takano, J., Roberts, D. M., and Fujiwara, T. (2008). NIP6;1 is a boric acid channel for preferential transport of boron to growing shoot tissues in Arabidopsis. Plant Cell 20, 2860–2875. doi: 10.1105/tpc.108.058628
Tanaka, N., Uraguchi, S., Saito, A., Kajikawa, M., Kasai, K., Sato, Y., et al. (2013). Roles of pollen-specific boron efflux transporter, OsBOR4, in the rice fertilization process. Plant Cell Physiol. 54, 2011–2019. doi: 10.1093/pcp/pct136
Tewari, R. K., Kumar, P., and Sharma, P. N. (2010). Morphology and oxidative physiology of boron-deficient mulberry plants. Tree Physiol. 30, 68–77. doi: 10.1093/treephys/tpp093
Tromp, J. (1983). “Nutrient reserves in roots of fruit trees, in particular carbohydrates and nitrogen,” in Tree Root Systems and Their Mycorrhizas, eds D. Atkinson, K. K. S. Bhat, M. P. Coutts, P. A. Mason, and D. J. Read (London: Dr. W. Junk Publishers), 401–413.
Uraguchi, S., Kato, Y., Hanaoka, H., Miwa, K., and Fujiwara, T. (2014). Generation of boron-deficiency-tolerant tomato by overexpressing an Arabidopsis thaliana borate transporter AtBOR1. Front. Plant Sci. 5:125. doi: 10.3389/fpls.2014.00125
Vail, J., Parry, M., and Calton, W. (1961). Boron-deficiency dieback in pines. Plant Soil 14, 393–398. doi: 10.1007/BF01666296
Voxeur, A., and Fry, S. C. (2014). Glycosylinositol phosphorylceramides (GIPCs) from Rosa cell cultures are boron-bridged in the plasma membrane and form complexes with rhamnogalacturonan-II. Plant J. 79, 139–149. doi: 10.1111/tpj.12547
Wang, D. N., and Ko, W. (1975). Relationship between deformed fruit disease of papaya and boron deficiency. Phytopathology 65, 445–447. doi: 10.1094/Phyto-65-445
Wang, N. N., Yan, T. S., Fu, L. N., Zhou, G. F., Liu, Y. Z., and Peng, S. A. (2014). Differences in boron distribution and forms in four citrus scion-rootstock combinations with contrasting boron efficiency under boron-deficient conditions. Trees 28, 1589–1598. doi: 10.1007/s00468-014-1067-1
Warington, K. (1923). The effect of boric acid and borax on the broad bean and certain other plants. Ann. Bot. 37, 629–672.
Wells, M. L., Conner, P. J., Funderburk, J. F., and Price, J. G. (2008). Effects of foliar-applied boron on fruit retention, fruit quality, and tissue boron concentration of pecan. HortScience 43, 696–699.
White, J., and Krause, H. (2001). Short-term boron deficiency in a black spruce (Picea mariana [Mill.] BSP) plantation. For. Ecol. Manag. 152, 323–330. doi: 10.1016/S0378-1127(00)00615-0
Will, S., Eichert, T., Fernández, V., Müller, T., and Römheld, V. (2012). Boron foliar fertilization of soybean and lychee: effects of side of application and formulation adjuvants. J. Plant Nutr. Soil Sci. 175, 180–188. doi: 10.1002/jpln.201100107
Wimmer, M. A., and Eichert, T. (2013). Review: mechanisms for boron deficiency-mediated changes in plant water relations. Plant Sci. 203, 25–32. doi: 10.1016/j.plantsci.2012.12.012
Wojcik, P., Wojcik, M., and Klamkowski, K. (2008). Response of apple trees to boron fertilization under conditions of low soil boron availability. Sci. Hortic. 116, 58–64. doi: 10.1016/j.scienta.2007.10.032
Wojcik, P., Wojcik, M., and Treder, W. (2003). Boron absorption and translocation in apple rootstocks under conditions of low medium boron. J. Plant Nutr. 26, 961–968. doi: 10.1081/PLN-120020068
Xiao, J. X., Yan, X., Peng, S. A., and Fang, Y. W. (2007). Seasonal changes of mineral nutrients in fruit and leaves of ‘Newhall’ and ‘Skagg’s Bonanza’ navel oranges. J. Plant Nutr. 30, 671–690. doi: 10.1080/01904160701289347
Yang, C. Q., Liu, Y. Z., An, J. C., Li, S., Jin, L. F., Zhou, G. F., et al. (2013). Digital gene expression analysis of corky split vein caused by boron deficiency in ‘Newhall’ navel orange (Citrus sinensis Osbeck) for selecting differentially expressed genes related to vascular hypertrophy. PLoS ONE 8:e65737. doi: 10.1371/journal.pone.0065737
Zhang, D. D., Zhao, H., Shi, L., and Xu, F. S. (2014). Physiological and genetic responses to boron deficiency in Brassica napus: a review. Soil Sci. Plant Nutr. 60, 304–313. doi: 10.1080/00380768.2014.893537
Zhou, G. F., Liu, Y. Z., Sheng, O., Wei, Q. J., Yang, C. Q., and Peng, S. A. (2015). Transcription profiles of boron-deficiency-responsive genes in citrus rootstock root by suppression subtractive hybridization and cDNA microarray. Front. Plant Sci. 5:795. doi: 10.3389/fpls.2014.00795
Keywords: boron efficiency, boron reserves, cell wall, lignin, transporter, trees
Citation: Wang N, Yang C, Pan Z, Liu Y and Peng S (2015) Boron deficiency in woody plants: various responses and tolerance mechanisms. Front. Plant Sci. 6:916. doi: 10.3389/fpls.2015.00916
Received: 01 December 2014; Accepted: 12 October 2015;
Published: 27 October 2015.
Edited by:
Richard William Bell, Murdoch University, AustraliaReviewed by:
Dirceu Mattos Jr, Instituto Agronômico, BrazilCopyright © 2015 Wang, Yang, Pan, Liu and Peng. This is an open-access article distributed under the terms of the Creative Commons Attribution License (CC BY). The use, distribution or reproduction in other forums is permitted, provided the original author(s) or licensor are credited and that the original publication in this journal is cited, in accordance with accepted academic practice. No use, distribution or reproduction is permitted which does not comply with these terms.
*Correspondence: Shu’ang Peng, c2h1YW5ncGVuZzQyOEAxMjYuY29t
†These authors have contributed equally to this work.
Disclaimer: All claims expressed in this article are solely those of the authors and do not necessarily represent those of their affiliated organizations, or those of the publisher, the editors and the reviewers. Any product that may be evaluated in this article or claim that may be made by its manufacturer is not guaranteed or endorsed by the publisher.
Research integrity at Frontiers
Learn more about the work of our research integrity team to safeguard the quality of each article we publish.