- 1Jiangsu Key Laboratory for Bioresources of Saline Soils; Provincial Key Laboratory of Agrobiology, Institute of Agro-biotechnology, Jiangsu Academy of Agricultural Sciences, Nanjing, China
- 2Key Laboratory of Coastal Biology and Bioresources, Yantai Institute of Coastal Zone Research (YIC), Chinese Academy of Sciences (CAS), Yantai, China
- 3Institute of Technology, Yantai Academy of China Agriculture University, Yantai, China
Abiotic stresses adversely affect plant growth and agricultural productivity. According to the current climate prediction models, crop plants will face a greater number of environmental stresses, which are likely to occur simultaneously in the future. So it is very urgent to breed broad-spectrum tolerant crops in order to meet an increasing demand for food productivity due to global population increase. As one of the largest families of transcription factors (TFs) in plants, NAC TFs play vital roles in regulating plant growth and development processes including abiotic stress responses. Lots of studies indicated that many stress-responsive NAC TFs had been used to improve stress tolerance in crop plants by genetic engineering. In this review, the recent progress in NAC TFs was summarized, and the potential utilization of NAC TFs in breeding abiotic stress tolerant transgenic crops was also be discussed. In view of the complexity of field conditions and the specificity in multiple stress responses, we suggest that the NAC TFs commonly induced by multiple stresses should be promising candidates to produce plants with enhanced multiple stress tolerance. Furthermore, the field evaluation of transgenic crops harboring NAC genes, as well as the suitable promoters for minimizing the negative effects caused by over-expressing some NAC genes, should be considered.
Introduction
As sessile organisms, plants continuously suffer from a broad range of environmental stresses including abiotic and biotic stresses. Abiotic stresses such as drought, salinity, heat and cold, adversely affect plant growth and agriculture productivity, and cause more than 50% of worldwide yield loss for major crops every year (Boyer, 1982; Bray et al., 2000; Shao et al., 2009; Ahuja et al., 2010; Lobell et al., 2011). Further to this, plants are also attacked by a vast range of pests and pathogens, including fungi, bacteria, viruses, nematodes, and herbivorous insects (Hammond-Kosack and Jones, 2000). In addition, current climate prediction models indicate the deterioration of climate including an increasing average temperature, a changing distribution of annual precipitation, a rise of sea level, and so on. This will be concurrent with an increased frequency of drought, flood, heat wave, and salinization (Easterling et al., 2000; IPCC, 2007, 2008; Mittler and Blumwald, 2010). Climate change will also affect the spread of pests and pathogens. For example, the increasing temperature can facilitate pathogen spread (Bale et al., 2002; Luck et al., 2011; Nicol et al., 2011), and many abiotic stress can weaken the defense mechanism of plants and increase their susceptibility to pathogen infection (Amtmann et al., 2008; Goel et al., 2008; Mittler and Blumwald, 2010; Atkinson and Urwin, 2012). Taken together, crop plants will face a greater range and number of environmental stresses, which are likely to occur simultaneously. So it is very urgent to breed stress-tolerant crop varieties to satisfy an increasing demand for food productivity due to global population increase (Takeda and Matsuoka, 2008; Newton et al., 2011).
To cope with these recurrent environmental stresses, plants can activate a number of defense mechanisms which include signal perception, signal transduction through either ABA-dependent or ABA-independent pathways, stress-responsive gene expression, in turn the activation of physiological and metabolic responses (Xiong et al., 2002; Chaves et al., 2003; Yamaguchi-Shinozaki and Shinozaki, 2006; Perez-Clemente et al., 2013). To date, a large array of stress responsive genes have been identified in many plants, including Arabidopsis and rice. These genes are generally classified into two types (Shinozaki et al., 2003). One is functional genes encoding important enzymes and metabolic proteins (functional proteins), such as detoxification enzyme, water channel, late embryogenesis abundant (LEA) protein, which directly function to protect cells from stresses. The other is regulatory genes encoding various regulatory proteins including transcription factors (TFs) and protein kinases, which regulate signal transduction and gene expression in the stress response. In the signal transduction processes, TFs play pivotal roles in the conversion of stress signal perception to stress-responsive gene expression. TFs and their interacting cis-elements function in the promoter region of different stress-related genes acting as molecular switches for gene expression. In plants ~7% of the genome encodes for putative TFs, which often belong to large gene families, such as WRKY, bZIP, MYB, AP2/EREBP, and NAC families (Udvardi et al., 2007; Golldack et al., 2011). In light of the key importance of TFs in controlling a wide range of downstream events, lots of studies have aimed to identify and characterize various TFs involved in stress responses. However, these studies have mostly focused on understanding the responses of model plants and crops to a single stress such as drought, salinity, heat or cold, pathogen infection, and so on (Hirayama and Shinozaki, 2010; Chew and Halliday, 2011). Unlike the controlled conditions in the laboratory, crops and other plants are often simultaneously subjected to multiple stresses in the field conditions (Ahuja et al., 2010). Recent studies have showed that plant response to a combination of drought and heat is not a simple additive effect of the individual stress, and the combination of multiple stresses produces a unique pattern of gene expression, which is distinct from the study of either stress individually (Rizhsky et al., 2002, 2004; Prasch and Sonnewald, 2013; Rasmussen et al., 2013). Therefore, the results of studies performed under individual stress factors are not suitable for the complex field conditions, and it is crucial to characterize the response of plants to multiple stresses and identify multiple stress responsive genes by imposing multiple stresses simultaneously as an entirely new stress (Mittler, 2006). Maybe, manipulation of these multiple stress responsive genes, especially multifunctional TFs, will provide the opportunity to breed the broad-spectrum tolerant crops with high yields. Based on these considerations above, this paper reviews the progress of NAC TFs involved in plant abiotic stress responses, and also prospects the future study direction for the challenge of multiple environmental stresses in agriculture, particularly concerning their potential utilization for plant multiple stress tolerance in the field conditions.
NAC Transcription Factors in Plants
As one of the largest family of TFs in plants, the NAC TFs comprise a complex plant-specific superfamily and are present in a wide range of species. The NAC acronym is derived from three earliest characterized proteins with a particular domain (NAC domain) from petunia NAM (no apical meristem), Arabidopsis ATAF1/2 and CUC2 (cup-shaped cotyledon; Souer et al., 1996; Aida et al., 1997). By the availability of an ever-increasing number of complete plant genomes and EST sequences, large numbers of putative NAC genes have been identified in many sequenced species at genome-wide scale (As shown in Table 1), such as 117 in Arabidopsis, 151 in rice, 74 in grape, 152 in soybean, 204 in Chinese cabbage, 152 in maize, and so on. The large size of NAC family inevitably complicates the unraveling of their regulatory process.
The NAC family has been found to function in various processes including shoot apical meristem (Takada et al., 2001), flower development (Sablowski and Meyerowitz, 1998), cell division (Kim et al., 2006), leaf senescence (Breeze et al., 2011), formation of secondary walls (Zhong et al., 2010), and biotic and abiotic stress responses (Olsen et al., 2005; Christianson et al., 2010; Tran et al., 2010; Nakashima et al., 2012). Nonetheless, only a few of these genes have been characterized to date and most of the NAC family members have not yet been studied, even though these genes are likely to play important roles in plants, and a great deal of work will be required to determine the specific biological function of each NAC gene. The intensive study on model plants including Arabidopsis and rice reveals that a typical NAC protein contains a highly conserved N-terminal DNA-binding NAC domain and a variable transcriptional regulatory region in the C-terminal region. The NAC domain with ~150–160 amino acids is divided into five sub-domains (A to E; Ooka et al., 2003). The function of the NAC domain has been associated with nuclear localization, DNA binding, and the formation of homodimers or heterodimers with other NAC domain-containing proteins (Olsen et al., 2005). In contrast, the highly diverged C-terminal region functions as a transcription regulatory region, acting as a transcriptional activator or repressor, but it has frequent occurrence of simple amino acid repeats and regions rich in serine and threonine, proline and glutamine, or acidic residues (Olsen et al., 2005; Puranik et al., 2012). Some NAC TFs also contain transmembrane motifs in the C-terminal region which are responsible for anchoring to plasma membrane or endoplasmic reticulum, and these NAC TFs are membrane-associated and designated as NTLs (Seo et al., 2008; Seo and Park, 2010).
The expression of NAC genes can firstly be regulated at the level of transcription because there are some stress-responsive cis-acting elements contained in the promoter region such as ABREs (ABA-responsive elements), DREs (Dehydration-responsive elements), jasmonic acid responsive element and salicylic acid responsive element. Then the complex post-transcriptional regulation involves microRNA-mediated cleavage of genes or alternative splicing. NAC TFs also undergo intensive post-translational regulation including ubiquitinization, dimerization, phosphorylation or proteolysis (Nakashima et al., 2012; Puranik et al., 2012). These regulatory steps help NAC TFs playing multiple roles in the majority of plant processes as mentioned above. The NAC TFs regulate the transcription of downstream target genes by binding to a consensus sequence in their promoters. The NAC recognition sequence (NACRS) containing the CACG core-DNA binding motif has been identified in the promoter of the drought inducible EARLY RESPONSE TO DEHYDRATION1 (ERD1) gene in Arabidopsis (Simpson et al., 2003; Tran et al., 2004). The rice drought-inducible ONAC TFs also can bind to a similar NACRS, demonstrating that the NACRS might be conserved across plants at least for stress-inducible NAC TFs (Hu et al., 2006; Fang et al., 2008). In addition, other sequences have also been reported as NAC binding sites (NACBS), such as an Arabidopsis calmodulin-binding NAC with GCTT as core-binding motif (Kim et al., 2007), the iron deficiency-responsive IDE2 motif containing the core sequence CA(A/C)G(T/C) (T/C/A) (T/C/A) (Ogo et al., 2008) and the secondary wall NAC binding element (SNBE) with (T/A)NN(C/T) (T/C/G)TNNNNNNNA(A/C)GN(A/C/T) (A/T) as consensus sequence (Zhong et al., 2010). The sequences flanking the core site in promoter of target genes may define the binding specificity of different NAC TFs. Thus, the NAC TF family can recognize a vast array of DNA-Binding sequences and regulate multiple downstream target genes. These target genes regulated by NAC TFs comprise regulatory genes encoding regulatory proteins which function in signal transduction and regulation of gene expression and functional genes encoding proteins which are involved in osmolyte production, reactive oxygen species scavenging and detoxification, macromolecule protection and ubiquitination (Puranik et al., 2012). Taken together, the existence of NACRS in promoter of some of these genes makes them to be the potential direct targets, whereas those that do not have this motif may not be direct targets. In future more other novel NACRS remain to be elucidated by microarrays combined with chromatin immunoprecipitation (Taverner et al., 2004).
NAC Transcription Factors Function in Abiotic Stress
The NAC TFs play a vital role in the complex signaling networks during plant stress responses. Because of the large number of NAC TFs from different plants and their unknown roles, it is still a great challenge to uncover their roles in abiotic stress. Recently, whole-genome expression profiling and transcriptome studies have enabled researchers to identify a number of putative NAC TFs involved in abiotic stress responses. For example, 33 NAC genes changed significantly in Arabdopsis under salt treatment (Jiang and Deyholos, 2006), 38 NAC genes were involved in response to drought in soybean (Le et al., 2011), 40 NAC genes responded to drought or salt stress in rice (Fang et al., 2008), 32 NAC genes responded to at least two kinds of treatments in Chrysanthemum lavandulifolium (Huang et al., 2012). It appears that a significant proportion of NAC genes function in stress response according to the expression data from genome-wide transcriptome analyses in many plants. Phylogenetic analyses of NAC TFs showed that most of the stress responsive NAC TFs appeared to contain a closely homologous NAC domain (Ernst et al., 2004; Fang et al., 2008). Moreover, the stress-responsive NAC genes exhibit a large diversity in expression patterns, indicating their involvement in the regulation of a wide spectrum of responses to different abiotic stresses. The precise regulations of NAC genes during plant abiotic stress responses contribute to the establishment of complex signaling networks, and the important roles of NAC genes in plant abiotic stress responses make them promising candidates for the generation of stress tolerant transgenic plants. The functional studies of NAC TFs by over-expression techniques will directly improve our understanding of the regulatory functions of NAC members to abiotic stresses. Transgenic constructs over-expressing the selected NAC genes have been made in Arabidopsis, rice and other plants. Some successful examples are summarized in Table 2.
Conclusions and Perspectives
Considerable information has been gained about NAC TFs since the discovery of NAC TFs, but the research in this area is still in its infancy. Genome-wide identification and expression profiling will undoubtedly open new avenues for describing the key features of NAC TFs. As a result, our current understandings of the regulatory functions of the NAC TFs in various plant species will be definitely accelerated. In particular, the stress-responsive NAC TFs can be used as promising candidates for generation of stress tolerant transgenic plants possessing high productivity under adverse conditions. As a matter of fact, many transgenic studies have been proved successful by gene manipulation of NAC TFs for conferring different stresses tolerance to plants (As shown in Table 2), but there are still some problems to be solved. Firstly, the constitutive overexpression of NAC genes occasionally may lead to negative effects in transgenic plants such as dwarfing, late flowering and lower yields (Fujita et al., 2004; Nakashima et al., 2007; Hao et al., 2011; Liu et al., 2011b). Secondly, the transgenic plants overexpressing NAC genes may occasionally have antagonistic responses to different stresses. For example, drought tolerant Arabidopsis plants overexpressing ATAF1 were highly sensitive to ABA, high-salt, oxidative stress and necrotrophic fungus (B. cinerea; Wu et al., 2009). Overexpressing ANAC019 and ANAC055 not only increased drought tolerance but also decreased resistance to B. cinerea (Fujita et al., 2004; Bu et al., 2008). Thirdly, only a few of transgenic plants overexpressing NAC genes were evaluated in the field trials so far, and most of them were tested in greenhouse conditions and focused on plant vegetative stages rather than reproductive stages (Valliyodan and Nguyen, 2006). Lastly, most of the studies on NAC TFs only investigated the molecular mechanisms of individual occurring stress situations. Although recent studies have conducted multi-parallel stress experiments and identified different NAC TFs responding to single stress situations (Huang et al., 2012), the knowledge concerning responses to combinations of several stress factors is scarce, especially interactions among stress factors.
As everyone knows, one of the most important aims for plant stress research is to provide targets for the improvement of stress tolerance in crop plants. With the forecast changes in climatic conditions leading to a more complex stress environment in the fields, we will face new challenges in creating the multiple stress-tolerant crops. Breeding such plants will depend on understanding the crucial stress-regulatory networks and the potential effects of different combinations of adverse conditions. Studies of multiple stress responses in Arabidopsis have provided us with several possible avenues. Master regulatory genes such as members of the MYC, MYB, and NAC TF families that act in multiple abiotic stress responses are excellent candidates for manipulating multiple stress tolerance. So in the future, it is crucial to impose multiple stresses simultaneously that simulate natural field conditions and regard each set of stress combinations as an entirely new stress in order to identify the corresponding NAC TFs commonly induced by multiple stresses. Manipulation of these genes should be the major target of attempts to produce plants with enhanced multiple stress tolerance. Furthermore, the potential NAC genes which confer multiple abiotic stress tolerance in model plant species must be tested in crop plants and greater emphasis should be placed on the field evaluation of the transgenic crops harboring NAC genes, especially focusing on their reproductive success. Another lesson is the selection and/or improvement of suitable promoters (such as a stress-inducible promoter) which can maximize the positive effects and minimize the negative effects caused by over-expressing some NAC genes. In summary, NAC TFs are the key components of the signaling pathway in stress response which carry out their function by interacting with both downstream and upstream partners (Figure 1). Understanding the molecular mechanisms of NAC TFs networks integrating multiple stress responses will be essential for the development of broad-spectrum stress tolerant crop plants that can better cope with environmental challenges in future climates.
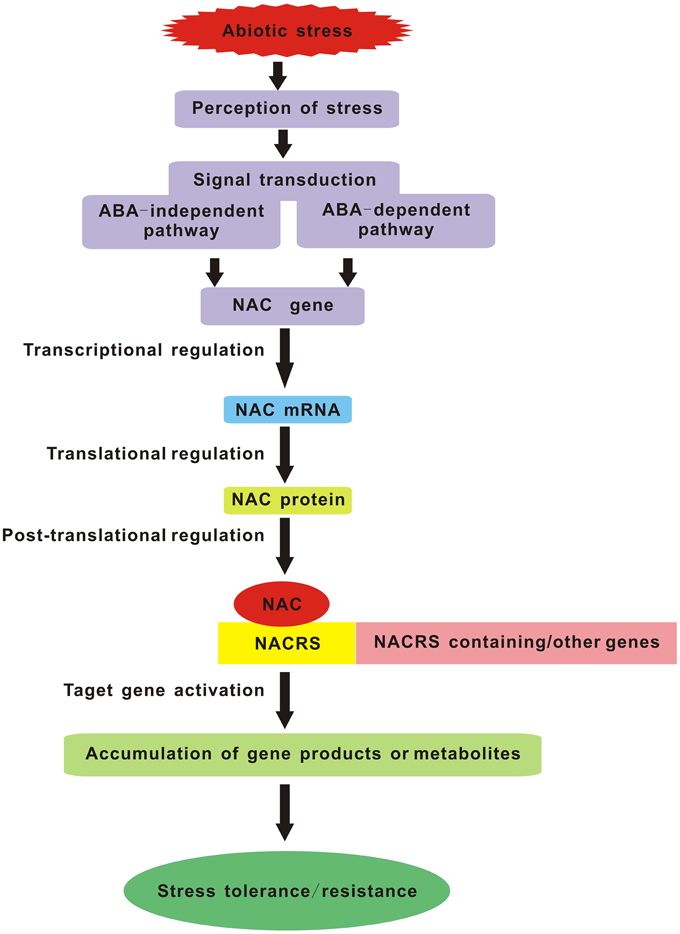
Figure 1. Schematic diagram of NAC TFs as key components in transcriptional regulatory networks during abiotic stress. NACRS is NAC recognition sequence.
Conflict of Interest Statement
The authors declare that the research was conducted in the absence of any commercial or financial relationships that could be construed as a potential conflict of interest.
Acknowledgments
This research was supported by Jiangsu Key Laboratory for Bioresources of Saline Soils (JKLBS2014006), the National Basic Research Program of China (2013CB430403), the National Natural Science Foundation of China (41171216), Jiangsu Autonomous Innovation Project of Agricultural Science and Technology [CX(15)1005], the Jiangsu Natural Science Foundation, China (BK20151364), Yantai Double-hundred Talent Plan (XY-003-02), and135 Development Plan of YIC-CAS.
References
Ahuja, I., de Vos, R. C., Bones, A. M., and Hall, R. D. (2010). Plant molecular stress responses face climate change. Trends Plant Sci. 15, 664–674. doi: 10.1016/j.tplants.2010.08.002
Aida, M., Ishida, T., Fukaki, H., Fujisawa, H., and Tasaka, M. (1997). Genes involved in organ separation in Arabidopsis: an analysis of the cup-shaped cotyledon mutant. Plant Cell 9, 841–857. doi: 10.1105/tpc.9.6.841
Amtmann, A., Troufflard, S., and Armengaud, P. (2008). The effect of potassium nutrition on pest and disease resistance in plants. Physiol. Plant. 133, 682–691. doi: 10.1111/j.1399-3054.2008.01075.x
Atkinson, N. J., and Urwin, P. E. (2012). The interaction of plant biotic and abiotic stresses: from genes to the field. J. Exp. Bot. 63, 3523–3543. doi: 10.1093/jxb/ers100
Bale, J. S., Masters, G. J., Hodkinson, I. D., Awmack, C., Bezemer, T. M., Brown, V. K., et al. (2002). Herbivory in global climate change research: direct effects of rising temperature on insect herbivores. Glob. Change Biol. 8, 1–16. doi: 10.1046/j.1365-2486.2002.00451.x
Boyer, J. S. (1982). Plant productivity and environment. Science 218, 443–448. doi: 10.1126/science.218.4571.443
Bray, E. A., Bailey-Serres, J., and Weretilnyk, E. (2000). “Responses to abiotic stresses,” in Biochemistry and Molecular Biology of Plants, eds W. Gruissem, B. Buchannan, and R. Jones (Rockville, MD: American Society of Plant Physiologists), 1158–1249.
Breeze, E., Harrison, E., McHattie, S., Hughes, L., Hickman, R., Hill, C., et al. (2011). High-resolution temporal profiling of transcripts during Arabidopsis leaf senescence reveals a distinct chronology of processes and regulation. Plant Cell 23, 873–894. doi: 10.1105/tpc.111.083345
Bu, Q., Jiang, H., Li, C. B., Zhai, Q., Zhang, J., Wu, X., et al. (2008). Role of the Arabidopsis thaliana NAC transcription factors ANAC019 and ANAC055 in regulating jasmonic acid-signaled defense responses. Cell Res. 18, 756–767. doi: 10.1038/cr.2008.53
Cenci, A., Guignon, V., Roux, N., and Rouard, M. (2014). Genomic analysis of NAC transcription factors in banana (Musa acuminata) and definition of NAC orthologous groups for monocots and dicots. Plant Mol. Biol. 85, 63–80. doi: 10.1007/s11103-013-0169-2
Chaves, M. M., Maroco, J., and Joao, S. P. (2003). Understanding plant responses to drought - from genes to the whole plant. Funct. Plant Biol. 30, 239–264. doi: 10.1071/FP02076
Chew, Y. H., and Halliday, K. J. (2011). A stress-free walk from Arabidopsis to crops. Curr. Opin. Biotechnol. 22, 281–286. doi: 10.1016/j.copbio.2010.11.011
Christianson, J. A., Dennis, E. S., Llewellyn, D. J., and Wilson, I. W. (2010). ATAF NAC transcription factors: regulators of plant stress signaling. Plant Signal. Behav. 5, 428–432. doi: 10.4161/psb.5.4.10847
Easterling, D. R., Meehl, G. A., Parmesan, C., Changnon, S. A., Karl, T. R., and Mearns, L. O. (2000). Climate extremes: observations, modeling, and impacts. Science 289, 2068–2074. doi: 10.1126/science.289.5487.2068
Ernst, H. A., Olsen, A. N., Larsen, S., and Lo Leggio, L. (2004). Structure of the conserved domain of ANAC, a member of the NAC family of transcription factors. EMBO Rep. 5, 297–303. doi: 10.1038/sj.embor.7400093
Fang, Y., You, J., Xie, K., Xie, W., and Xiong, L. (2008). Systematic sequence analysis and identification of tissue-specific or stress-responsive genes of NAC transcription factor family in rice. Mol. Genet. Genomics 280, 547–563. doi: 10.1007/s00438-008-0386-6
Fujita, M., Fujita, Y., Maruyama, K., Seki, M., Hiratsu, K., Ohme-Takagi, M., et al. (2004). A dehydration-induced NAC protein, RD26, is involved in a novel ABA-dependent stress-signaling pathway. Plant J. 39, 863–876. doi: 10.1111/j.1365-313X.2004.02171.x
Goel, A. K., Lundberg, D., Torres, M. A., Matthews, R., Akimoto-Tomiyama, C., Farmer, L., et al. (2008). The Pseudomonas syringae type III effector HopAM1 enhances virulence on water-stressed plants. Mol. Plant-Microbe Interact. 21, 361–370. doi: 10.1094/MPMI-21-3-0361
Golldack, D., Lüking, I., and Yang, O. (2011). Plant tolerance to drought and salinity: stress regulating transcription factors and their functional significance in the cellular transcriptional network. Plant Cell Rep. 30, 1383–1391. doi: 10.1007/s00299-011-1068-0
Ha, C. V., Esfahani, M. N., Watanabe, Y., Tran, U. T., Sulieman, S., Mochida, K., et al. (2014). Genome-wide identification and expression analysis of the CaNAC family members in chickpea during development, dehydration and ABA treatments. PLoS ONE 9:e114107. doi: 10.1371/journal.pone.0114107
Hammond-Kosack, K. E., and Jones, J. D. G. (2000). “Response to plant pathogens,” in Biochemistry and Molecular Biology of Plants, eds B. Buchannan, W. Gruissem, and R. Jones (Rockville, MD: American Society of Plant Physiologists), 1102–1157.
Hao, Y. J., Wei, W., Song, Q. X., Chen, H. W., Zhang, Y. Q., Wang, F., et al. (2011). Soybean NAC transcription factors promote abiotic stress tolerance and lateral root formation in transgenic plants. Plant J. 68, 302–313. doi: 10.1111/j.1365-313X.2011.04687.x
Hirayama, T., and Shinozaki, K. (2010). Research on plant abiotic stress responses in the post-genome era: past, present and future. Plant J. 61, 1041–1052. doi: 10.1111/j.1365-313X.2010.04124.x
Hu, H., Dai, M., Yao, J., Xiao, B., Li, X., Zhang, Q., et al. (2006). Overexpressing a NAM, ATAF, and CUC (NAC) transcription factor enhances drought resistance and salt tolerance in rice. Proc. Natl. Acad. Sci. U.S.A. 103, 12987–12992. doi: 10.1073/pnas.0604882103
Hu, R., Qi, G., Kong, Y., Kong, D., Gao, Q., and Zhou, G. (2010). Comprehensive analysis of NAC domain transcription factor gene family in Populus trichocarpa. BMC Plant Biol. 10:145. doi: 10.1186/1471-2229-10-145
Hu, W., Wei, Y., Xia, Z., Yan, Y., Hou, X., Zou, M., et al. (2015). Genome-wide identification and expression analysis of the NAC transcription factor family in cassava. PLoS ONE 10:e0136993. doi: 10.1371/journal.pone.0136993
Huang, H., Wang, Y., Wang, S., Wu, X., Yang, K., Niu, Y., et al. (2012). Transcriptome-wide survey and expression analysis of stress-responsive NAC genes in Chrysanthemum lavandulifolium. Plant Sci. 193–194, 18–27. doi: 10.1016/j.plantsci.2012.05.004
IPCC (2007). “Climate change 2007: the physical science basis,” in Contribution of Working Group I to the Fourth Assessment Report of the Intergovernmental Panel on Climate Change, eds S. Solomon, D. Qin, M. Manning, Z. Chen, M. Marquis, K. B. Averyt, et al. (Geneva: IPCC Secretariat).
IPCC (2008). “Climate change and water,” in Technical Paper of the Intergovernmental Panel on Climate Change, eds B. C. Bates, Z. W. Kundzewicz, J. Palutikof, and S. Wu (Geneva: IPCC Secretariat).
Jensen, M. K., Kjaersgaard, T., Nielsen, M. M., Galberg, P., Petersen, K., O'shea, C., et al. (2010). The Arabidopsis thaliana NAC transcription factor family: structure-function relationships and determinants of ANAC019 stress signalling. Biochem. J. 426, 183–196. doi: 10.1042/BJ20091234
Jeong, J. S., Kim, Y. S., Baek, K. H., Jung, H., Ha, S. H., Do Choi, Y., et al. (2010). Root-specific expression of OsNAC10 improves drought tolerance and grain yield in rice under field drought conditions. Plant Physiol. 153, 185–197. doi: 10.1104/pp.110.154773
Jiang, Y., and Deyholos, M. K. (2006). Comprehensive transcriptional profiling of NaCl-stressed Arabidopsis roots reveals novel classes of responsive genes. BMC Plant Biol. 6:25. doi: 10.1186/1471-2229-6-25
Kim, H. S., Park, B. O., Yoo, J. H., Jung, M. S., Lee, S. M., Han, H. J., et al. (2007). Identification of a calmodulin-binding NAC protein as a transcriptional repressor in Arabidopsis. J. Biol. Chem. 282, 36292–36302. doi: 10.1074/jbc.M705217200
Kim, Y. S., Kim, S. G., Park, J. E., Park, H. Y., Lim, M. H., Chua, N. H., et al. (2006). A membrane-bound NAC transcription factor regulates cell division in Arabidopsis. Plant Cell 18, 3132–3144. doi: 10.1105/tpc.106.043018
Le, D. T., Nishiyama, R., Watanabe, Y., Mochida, K., Yamaguchi-Shinozaki, K., Shinozaki, K., et al. (2011). Genome-wide survey and expression analysis of the plant-specific NAC transcription factor family in soybean during development and dehydration stress. DNA Res. 18, 263–276. doi: 10.1093/dnares/dsr015
Liu, Q. L., Xu, K. D., Zhao, L. J., Pan, Y. Z., Jiang, B. B., Zhang, H. Q., et al. (2011a). Overexpression of a novel chrysanthemum NAC transcription factor gene enhances salt tolerance in tobacco. Biotechnol. Lett. 33, 2073–2082. doi: 10.1007/s10529-011-0659-8
Liu, T. K., Song, X. M., Duan, W. K., Huang, Z. N., Liu, G. F., Li, Y., et al. (2014). Genome-wide analysis and expression patterns of NAC transcription factor family under different developmental stages and abiotic stresses in Chinese cabbage. Plant Mol. Biol. Rep. 32, 1041–1056. doi: 10.1007/s11105-014-0712-6
Liu, X., Hong, L., Li, X. Y., Yao, Y., Hu, B., and Li, L. (2011b). Improved drought and salt tolerance in transgenic Arabidopsis overexpressing a NAC transcriptional factor from Arachis hypogaea. Biosci. Biotechnol. Biochem. 75, 443–450. doi: 10.1271/bbb.100614
Lobell, D. B., Schlenker, W., and Costa-Roberts, J. (2011). Climate trends and global crop production since 1980. Science 333, 616–620. doi: 10.1126/science.1204531
Luck, J., Spackman, M., Freeman, A., Trębicki, P., Griffiths, W., Finlay, K., et al. (2011). Climate change and diseases of food crops. Plant Pathol. 60, 113–121. doi: 10.1111/j.1365-3059.2010.02414.x
Lu, M., Ying, S., Zhang, D. F., Shi, Y. S., Song, Y. C., Wang, T. Y., et al. (2012). A maize stress-responsive NAC transcription factor, ZmSNAC1, confers enhanced tolerance to dehydration in transgenic Arabidopsis. Plant Cell Rep. 31, 1701–1711. doi: 10.1007/s00299-012-1284-2
Mao, X., Zhang, H., Qian, X., Li, A., Zhao, G., and Jing, R. (2012). TaNAC2, a NAC-type wheat transcription factor conferring enhanced multiple abiotic stress tolerances in Arabidopsis. J. Exp. Bot. 63, 2933–2946. doi: 10.1093/jxb/err462
Mittler, R. (2006). Abiotic stress, the field environment and stress combination. Trends Plant Sci. 11, 15–19. doi: 10.1016/j.tplants.2005.11.002
Mittler, R., and Blumwald, E. (2010). Genetic engineering for modern agriculture: challenges and perspectives. Annu. Rev. Plant Biol. 61, 443–462. doi: 10.1146/annurev-arplant-042809-112116
Nakashima, K., Takasaki, H., Mizoi, J., Shinozaki, K., and Yamaguchi-Shinozaki, K. (2012). NAC transcription factors in plant abiotic stress responses. Biochim. Biophys. Acta 1819, 97–103. doi: 10.1016/j.bbagrm.2011.10.005
Nakashima, K., Tran, L. S., van Nguyen, D., Fujita, M., Maruyama, K., Todaka, D., et al. (2007). Functional analysis of a NAC-type transcription factor OsNAC6 involved in abiotic and biotic stress-responsive gene expression in rice. Plant J. 51, 617–630. doi: 10.1111/j.1365-313X.2007.03168.x
Newton, A. C., Johnson, S. N., and Gregory, P. J. (2011). Implications of climate change for diseases, crop yields and food security. Euphytica 179, 3–18. doi: 10.1007/s10681-011-0359-4
Nicol, J. M., Turner, S. J., Coyne, D. L., Nijs, L. D., Hockland, S., and Maafi, Z. T. (2011). “Current nematode threats to world agriculture,” in Genomics and Molecular Genetics of Plant-Nematode Interactions, eds J. Jones, G. Gheysen, and C. Fenoll (Berlin: Springer), 21–43. doi: 10.1007/978-94-007-0434-3_2
Nuruzzaman, M., Manimekalai, R., Sharoni, A. M., Satoh, K., Kondoh, H., Ooka, H., et al. (2010). Genome-wide analysis of NAC transcription factor family in rice. Gene 465, 30–44. doi: 10.1016/j.gene.2010.06.008
Ogo, Y., Kobayashi, T., Nakanishi Itai, R., Nakanishi, H., Kakei, Y., Takahashi, M., et al. (2008). A novel NAC transcription factor, IDEF2, that recognizes the iron deficiency-responsive element 2 regulates the genes involved in iron homeostasis in plants. J. Biol. Chem. 283, 13407–13417. doi: 10.1074/jbc.M708732200
Olsen, A. N., Ernst, H. A., Leggio, L. L., and Skriver, K. (2005). NAC transcription factors: structurally distinct, functionally diverse. Trends Plant Sci. 10, 79–87. doi: 10.1016/j.tplants.2004.12.010
Ooka, H., Satoh, K., Doi, K., Nagata, T., Otomo, Y., Murakami, K., et al. (2003). Comprehensive analysis of NAC family genes in Oryza sativa and Arabidopsis thaliana. DNA Res. 10, 239–247. doi: 10.1093/dnares/10.6.239
Pérez-Clemente, R. M., Vives, V., Zandalinas, S. I., López-Climent, M. F., Muñoz, V., and Gómez-Cadenas, A. (2013). Biotechnological approaches to study plant responses to stress. Biomed Res. Int. 2013:654120. doi: 10.1155/2013/654120
Prasch, C. M., and Sonnewald, U. (2013). Simultaneous application of heat, drought, and virus to Arabidopsis plants reveals significant shifts in signaling networks. Plant Physiol. 162, 1849–1866. doi: 10.1104/pp.113.221044
Puranik, S., Sahu, P. P., Mandal, S. N., B, V. S., Parida, S. K., and Prasad, M. (2013). Comprehensive genome-wide survey, genomic constitution and expression profiling of the NAC transcription factor family in foxtail millet (Setaria italica L.). PLoS ONE 8:e64594. doi: 10.1371/journal.pone.0064594
Puranik, S., Sahu, P. P., Srivastava, P. S., and Prasad, M. (2012). NAC proteins: regulation and role in stress tolerance. Trends Plant Sci. 17, 369–381. doi: 10.1016/j.tplants.2012.02.004
Ramegowda, V., Senthil-Kumar, M., Nataraja, K. N., Reddy, M. K., Mysore, K. S., and Udayakumar, M. (2012). Expression of a finger millet transcription factor, EcNAC1, in tobacco confers abiotic stress-tolerance. PLoS ONE 7:e40397. doi: 10.1371/journal.pone.0040397
Rasmussen, S., Barah, P., Suarez-Rodriguez, M. C., Bressendorff, S., Friis, P., Costantino, P., et al. (2013). Transcriptome responses to combinations of stresses in Arabidopsis. Plant Physiol. 161, 1783–1794. doi: 10.1104/pp.112.210773
Rizhsky, L., Liang, H., and Mittler, R. (2002). The combined effect of drought stress and heat shock on gene expression in tobacco. Plant Physiol. 130, 1143–1151. doi: 10.1104/pp.006858
Rizhsky, L., Liang, H., Shuman, J., Shulaev, V., Davletova, S., and Mittler, R. (2004). When defense pathways collide. The response of Arabidopsis to a combination of drought and heat stress. Plant Physiol. 134, 1683–1696. doi: 10.1104/pp.103.033431
Rushton, P. J., Bokowiec, M. T., Han, S., Zhang, H., Brannock, J. F., Chen, X., et al. (2008). Tobacco transcription factors: novel insights into transcriptional regulation in the Solanaceae. Plant Physiol. 147, 280–295. doi: 10.1104/pp.107.114041
Sablowski, R. W., and Meyerowitz, E. M. (1998). A homolog of NO APICAL MERISTEM is an immediate target of the floral homeotic genes APETALA3/PISTILLATA. Cell 92, 93–103. doi: 10.1016/S0092-8674(00)80902-2
Satheesh, V., Jagannadham, P. T., Chidambaranathan, P., Jain, P. K., and Srinivasan, R. (2014). NAC transcription factor genes: genome-wide identification, phylogenetic, motif and cis-regulatory element analysis in pigeonpea (Cajanus cajan (L.) Millsp.). Mol. Biol. Rep. 41, 7763–7773. doi: 10.1007/s11033-014-3669-5
Seo, P. J., Kim, S. G., and Park, C. M. (2008). Membrane-bound transcription factors in plants. Trends Plant Sci. 13, 550–556. doi: 10.1016/j.tplants.2008.06.008
Seo, P. J., and Park, C. M. (2010). A membrane-bound NAC transcription factor as an integrator of biotic and abiotic stress signals. Plant Signal. Behav. 5, 481–483. doi: 10.4161/psb.11083
Shahnejat-Bushehri, S., Mueller-Roeber, B., and Balazadeh, S. (2012). Arabidopsis NAC transcription factor JUNGBRUNNEN1 affects thermomemory-associated genes and enhances heat stress tolerance in primed and unprimed conditions. Plant Signal. Behav. 7, 1518–1521. doi: 10.4161/psb.22092
Shang, H., Li, W., Zou, C., and Yuan, Y. (2013). Analyses of the NAC transcription factor gene family in Gossypium raimondii Ulbr.: chromosomal location, structure, phylogeny, and expression patterns. J. Integr. Plant Biol. 55, 663–676. doi: 10.1111/jipb.12085
Shao, H. B., Chu, L. Y., Jaleel, C. A., Manivannan, P., Panneerselvam, R., and Shao, M. A. (2009). Understanding water deficit stress-induced changes in the basic metabolism of higher plants - biotechnologically and sustainably improving agriculture and the ecoenvironment in arid regions of the globe. Crit. Rev. Biotechnol. 29, 131–151. doi: 10.1080/07388550902869792
Shinozaki, K., Yamaguchi-Shinozakiy, K., and Sekiz, M. (2003). Regulatory network of gene expression in the drought and cold stress responses. Curr. Opin. Plant Biol. 6, 410–417. doi: 10.1016/S1369-5266(03)00092-X
Shiriga, K., Sharma, R., Kumar, K., Yadav, S. K., Hossain, F., and Thirunavukkarasu, N. (2014). Genome-wide identification and expression pattern of drought-responsive members of the NAC family in maize. Meta Gene 2, 407–417. doi: 10.1016/j.mgene.2014.05.001
Simpson, S. D., Nakashima, K., Narusaka, Y., Seki, M., Shinozaki, K., and Yamaguchi-Shinozaki, K. (2003). Two different novel cis-acting elements of erd1, a clpA homologous Arabidopsis gene function in induction by dehydration stress and dark-induced senescence. Plant J. 33, 259–270. doi: 10.1046/j.1365-313X.2003.01624.x
Sindhu, A., Chintamanani, S., Brandt, A. S., Zanis, M., Scofield, S. R., and Johal, G. S. (2008). A guardian of grasses: specific origin and conservation of a unique disease-resistance gene in the grass lineage. Proc. Natl. Acad. Sci. U.S.A. 105, 1762–1767. doi: 10.1073/pnas.0711406105
Singh, A. K., Sharma, V., Pal, A. K., Acharya, V., and Ahuja, P. S. (2013). Genome-wide organization and expression profiling of the NAC transcription factor family in potato (Solanum tuberosum L.). DNA Res. 20, 403–423. doi: 10.1093/dnares/dst019
Song, S. Y., Chen, Y., Chen, J., Dai, X. Y., and Zhang, W. H. (2011). Physiological mechanisms underlying OsNAC5-dependent tolerance of rice plants to abiotic stress. Planta 234, 331–345. doi: 10.1007/s00425-011-1403-2
Souer, E., van Houwelingen, A., Kloos, D., Mol, J., and Koes, R. (1996). The no apical meristem gene of Petunia is required for pattern formation in embryos and flowers and is expressed at meristem and primordia boundaries. Cell 85, 159–170. doi: 10.1016/S0092-8674(00)81093-4
Sperotto, R. A., Ricachenevsky, F. K., Duarte, G. L., Boff, T., Lopes, K. L., Sperb, E. R., et al. (2009). Identification of up-regulated genes in flag leaves during rice grain filling and characterization of OsNAC5, a new ABA-dependent transcription factor. Planta 230, 985–1002. doi: 10.1007/s00425-009-1000-9
Su, H. Y., Zhang, S. Z., Yin, Y. L., Zhu, D. Z., and Han, L. Y. (2015). Genome-wide analysis of NAM-ATAF1,2-CUC2 transcription factor family in Solanum lycopersicum. J. Plant Biochem. Biotechnol. 24, 176–183. doi: 10.1007/s13562-014-0255-9
Su, H., Zhang, S., Yuan, X., Chen, C., Wang, X. F., and Hao, Y. J. (2013). Genome-wide analysis and identification of stress-responsive genes of the NAM-ATAF1,2-CUC2 transcription factor family in apple. Plant Physiol. Biochem. 71, 11–21. doi: 10.1016/j.plaphy.2013.06.022
Takada, S., Hibara, K., Ishida, T., and Tasaka, M. (2001). The CUP-SHAPED COTYLEDON1 gene of Arabidopsis regulates shoot apical meristem formation. Development 128, 1127–1135.
Takeda, S., and Matsuoka, M. (2008). Genetic approaches to crop improvement: responding to environmental and population changes. Nat. Rev. Genet. 9, 444–457. doi: 10.1038/nrg2342
Tang, Y., Liu, M., Gao, S., Zhang, Z., Zhao, X., Zhao, C., et al. (2012). Molecular characterization of novel TaNAC genes in wheat and overexpression of TaNAC2a confers drought tolerance in tobacco. Physiol. Plant. 144, 210–224. doi: 10.1111/j.1399-3054.2011.01539.x
Taverner, N. V., Smith, J. C., and Wardle, F. C. (2004). Identifying transcriptional targets. Genome Biol. 5, 210. doi: 10.1186/gb-2004-5-3-210
Tran, L. S., Nakashima, K., Sakuma, Y., Simpson, S. D., Fujita, Y., Maruyama, K., et al. (2004). Isolation and functional analysis of Arabidopsis stress-inducible NAC transcription factors that bind to a drought-responsive cis-element in the early responsive to dehydration stress 1 promoter. Plant Cell 16, 2481–2498. doi: 10.1105/tpc.104.022699
Tran, L. S., Nishiyama, R., Yamaguchi-Shinozaki, K., and Shinozaki, K. (2010). Potential utilization of NAC transcription factors to enhance abiotic stress tolerance in plants by biotechnological approach. GM Crops 1, 32–39. doi: 10.4161/gmcr.1.1.10569
Udvardi, M. K., Kakar, K., Wandrey, M., Montanari, O., Murray, J., Andriankaja, A., et al. (2007). Legume transcription factors: global regulators of plant development and response to the environment. Plant Physiol. 144, 538–549. doi: 10.1104/pp.107.098061
Valliyodan, B., and Nguyen, H. T. (2006). Understanding regulatory networks and engineering for enhanced drought tolerance in plants. Curr. Opin. Plant Biol. 9, 189–195. doi: 10.1016/j.pbi.2006.01.019
Wang, N., Zheng, Y., Xin, H., Fang, L., and Li, S. (2013). Comprehensive analysis of NAC domain transcription factor gene family in Vitis vinifera. Plant Cell Rep. 32, 61–75. doi: 10.1007/s00299-012-1340-y
Wu, Y., Deng, Z., Lai, J., Zhang, Y., Yang, C., Yin, B., et al. (2009). Dual function of Arabidopsis ATAF1 in abiotic and biotic stress responses. Cell Res. 19, 1279–1290. doi: 10.1038/cr.2009.108
Wu, Z., Xu, X., Xiong, W., Wu, P., Chen, Y., Li, M., et al. (2015). Genome-wide analysis of the NAC gene family in physic nut (Jatropha curcas L.). PLoS ONE 10:e0131890. doi: 10.1371/journal.pone.0131890
Xiong, L., Schumaker, K. S., and Zhu, J. K. (2002). Cell signaling during cold, drought, and salt stress. Plant Cell 14(Suppl.), S165–S183. doi: 10.1105/tpc.000596
Xue, G. P., Way, H. M., Richardson, T., Drenth, J., Joyce, P. A., and McIntyre, C. L. (2011). Overexpression of TaNAC69 leads to enhanced transcript levels of stress up-regulated genes and dehydration tolerance in bread wheat. Mol. Plant 4, 697–712. doi: 10.1093/mp/ssr013
Yamaguchi-Shinozaki, K., and Shinozaki, K. (2006). Transcriptional regulatory networks in cellular responses and tolerance to dehydration and cold stresses. Annu. Rev. Plant Biol. 57, 781–803. doi: 10.1146/annurev.arplant.57.032905.105444
Yokotani, N., Ichikawa, T., Kondou, Y., Matsui, M., Hirochika, H., Iwabuchi, M., et al. (2009). Tolerance to various environmental stresses conferred by the salt-responsive rice gene ONAC063 in transgenic Arabidopsis. Planta 229, 1065–1075. doi: 10.1007/s00425-009-0895-5
You, J., Zhang, L., Song, B., Qi, X., and Chan, Z. (2015). Systematic analysis and identification of stress-responsive genes of the NAC gene family in Brachypodium distachyon. PLoS ONE 10:e0122027. doi: 10.1371/journal.pone.0122027
Zheng, X., Chen, B., Lu, G., and Han, B. (2009). Overexpression of a NAC transcription factor enhances rice drought and salt tolerance. Biochem. Biophys. Res. Commun. 379, 985–989. doi: 10.1016/j.bbrc.2008.12.163
Keywords: abiotic stress, multiple stresses, NAC, transcription factors, transgenic plant
Citation: Shao H, Wang H and Tang X (2015) NAC transcription factors in plant multiple abiotic stress responses: progress and prospects. Front. Plant Sci. 6:902. doi: 10.3389/fpls.2015.00902
Received: 29 June 2015; Accepted: 09 October 2015;
Published: 29 October 2015.
Edited by:
Girdhar Kumar Pandey, University of Delhi, IndiaReviewed by:
Chandrashekhar Pralhad Joshi, Michigan Technological University, USALam-Son Tran, RIKEN Center for Sustainable Resource Science, Japan
Copyright © 2015 Shao, Wang and Tang. This is an open-access article distributed under the terms of the Creative Commons Attribution License (CC BY). The use, distribution or reproduction in other forums is permitted, provided the original author(s) or licensor are credited and that the original publication in this journal is cited, in accordance with accepted academic practice. No use, distribution or reproduction is permitted which does not comply with these terms.
*Correspondence: Hongbo Shao, c2hhb2hvbmdib2NodUAxMjYuY29t
†These authors have contributed equally to this work.