- 1Department of Genetics, Osmania University, Hyderabad, India
- 2Leibniz Institute of Plant Genetics and Crop Plant Research, Gatersleben, Germany
- 3Grain Quality and Nutrition Center, International Rice Research Institute, Metro Manila, Philippines
Proline is a proteogenic amino acid and accumulates both under stress and non-stress conditions as a beneficial solute in plants. Recent discoveries point out that proline plays an important role in plant growth and differentiation across life cycle. It is a key determinant of many cell wall proteins that plays important roles in plant development. The role of extensins, arabinogalactan proteins and hydroxyproline- and proline-rich proteins as important components of cell wall proteins that play pivotal roles in cell wall signal transduction cascades, plant development and stress tolerance is discussed in this review. Molecular insights are also provided here into the plausible roles of proline transporters modulating key events in plant development. In addition, the roles of proline during seed developmental transitions including storage protein synthesis are discussed.
Introduction
Glutamate is an important amino acid and acts as a precursor for the biosynthesis of γ-aminobutyric acid, arginine, glutamine, and proline in plants as well as in other eukaryotes (Rhodes et al., 1986; Szekely et al., 2008; Sharma and Verslues, 2010). Proline can be distinguished among all other amino acids due to its unique structure with its α-amino group as a secondary amine and possesses distinctive cyclic structure which causes exceptional conformational rigidity to the protein structure (MacArthur and Thornton, 1991). It has also specific chemical properties like zwitterionic nature, neutral pH, high compatibility with cell milieu and extreme solubility as 14 kgs of it can be dissolved in 1 kg of water (LeRudulier et al., 1984). Though evidence has been presented for the synthesis of proline from glutamate and ornithine in higher plants (Delauney and Verma, 1993; Sharma et al., 2013), the role of ornithine as proline precursor is doubtful as pointed out by Funck et al. (2008). The pathway for proline biosynthesis in higher plants differs from that of bacteria (Kishor et al., 2005; Verbruggen and Hermans, 2008; Szabados and Savoure, 2010). The genes that encode the enzymes involved in proline biosynthesis like pyrroline-5-carboxylate synthetase (P5CS), pyrroline-5-carboxylate reductase (P5CR), and ornithine-δ-aminotransferase (OAT) and proline catabolism such as proline dehydrogenase (PDH) and pyrroline-5-carboxylate dehydrogenase (P5CDH) have been identified and isolated (Delauney and Verma, 1990; Hu et al., 1992; Delauney et al., 1993; Kiyosue et al., 1996; Strizhov et al., 1997; Deuschle et al., 2001; Funck et al., 2010). P5CS1 was first cloned from Vigna aconitifolia by complementation technique (Hu et al., 1992), and found to be a novel bifunctional enzyme (with activities of both γ-glutamy kinase and glutamic γ-semialdehyde dehydrogenase) that catalyzes glutamate to pyrroline-5 carboxylate, an intermediate in proline biosynthesis. This is a rate limiting step in proline biosynthesis. P5CS is encoded by two differentially regulated genes (P5CS1 and P5CS2) and P5CS2 was first cloned from Arabidopsis thaliana (Strizhov et al., 1997). While P5CS1 is abundantly expressed in most plant parts but not in dividing cells, P5CS2 is highly expressed at the transcriptional level in dividing cells (Strizhov et al., 1997). Besides acting as a proteogenic amino acid, it accumulates in large quantities and plays a role during abiotic stress tolerance in plants (Kishor et al., 1995, 2005; Sharma and Verslues, 2010). Thus, it is known that proline participates in the biosynthesis of primary metabolism, but also has special functions to carry as a metabolite during growth and development (Hare and Cress, 1997; Hare et al., 1999; Trovato et al., 2001; Mattioli et al., 2009a; Funck et al., 2012). Proline not only accumulates during abiotic stress, but also in different tissues of plants under non-stress conditions too (Kishor and Sreenivasulu, 2014). The role of proline as osmoprotectant in stress tolerance has been extensively reviewed (Verbruggen and Hermans, 2008; Szabados and Savoure, 2010). Though many amino acids constitute a major source of the components used for cellular growth and differentiation in higher plants, proline is distinct from others. The emerging roles of proline in plant growth and development from the perspective of proline homeostasis in mediating growth and development has been reviewed (Kishor and Sreenivasulu, 2014). Over and above, proline is an important source of cell wall matrix. As a component of cell wall proteins, it plays pivotal role in plant development but understanding of its diverse functions appears to be enigmatic. Cell walls contain hydroxyproline-rich O-glycoproteins (HRGPs) as complex macromolecules with varying structures and functions and encompass a broad category of extracellular proteins. HRGPs contain variations of Pro-Pro repeats (Cassab and Varner, 1988; Showalter, 1993) and are lightly glycosylated. Their classification is based on proline residue-containing proteins (Cassab and Varner, 1988; Showalter, 1993). It is a superfamily that is classified into extensins (EXTs) that are moderately glycosylated, arabinogalactan-proteins (AGPs) that are hyperglycosylated (Henrissat et al., 2001; Lamport et al., 2011) and Hyp/Pro-rich proteins (H/PRPs) that may not be glycosylated at all, or either weakly- or highly glycosylated. HRGPs undergo post-translational modifications like conversion of proline to hydroxyproline (Hyp) by a membrane-bound prolyl 4-hydroxylases (P4Hs) or glycosylation of HRGPs by glycosyltransferases (Rhee et al., 2003; Mayer and Jurgens, 2004; Wang and He, 2004). This sub-family is characterized by O-Hyp linked arabinosides and arabinogalactan polysaccharides (Lamport, 1967, 1977; Pope, 1977) which define the molecular surface. Arabinosylation and arabinogalactosylation would depend upon contiguous Hyp and clustered non-contiguous Hyp. What directs the Hyp glycosylation is a code based on peptide sequence. While Ser-Hyp4 motifs of EXTs are preferred sites for the addition of arabinoside, Ser-Hyp and Ala-Hyp repeats of the AGPs are the usual sites of arabinogalactan heteropolysaccharide addition (Kieliszewski and Shpak, 2001; Zhao et al., 2002; Held et al., 2004). Studies carried out by many suggest that species variation and tissue specific Hyp glycosylation occurs (Kieliszewski and Shpak, 2001; Zhao et al., 2002; Held et al., 2004). HRGPs appear to be not solubilized in cell walls as a response to the stress (Showalter, 1993; Fowler et al., 1999). They are expressed during wounding and pathogen attack in some tissues and implicated in different stages of plant growth and development, nodule formation, fertilization, cytokinesis, apoptosis, senescence, and cell wall lignification (Ye et al., 1991; Showalter, 1993, 2001; Nothnagel, 1997; Wu et al., 2001; Hall and Cannon, 2002; Lamport et al., 2006). Different roles of proline in plant ontogeny and during key transitions are summarized in Table 1. In the present endeavor, we aim at reviewing the progress of multidimensional functions of proline in plant ontogeny which is contributing to cell wall modifications and also during key transitional events especially reproductive development. In addition, the implications of proline during seed development and seed storage metabolism has been highlighted.
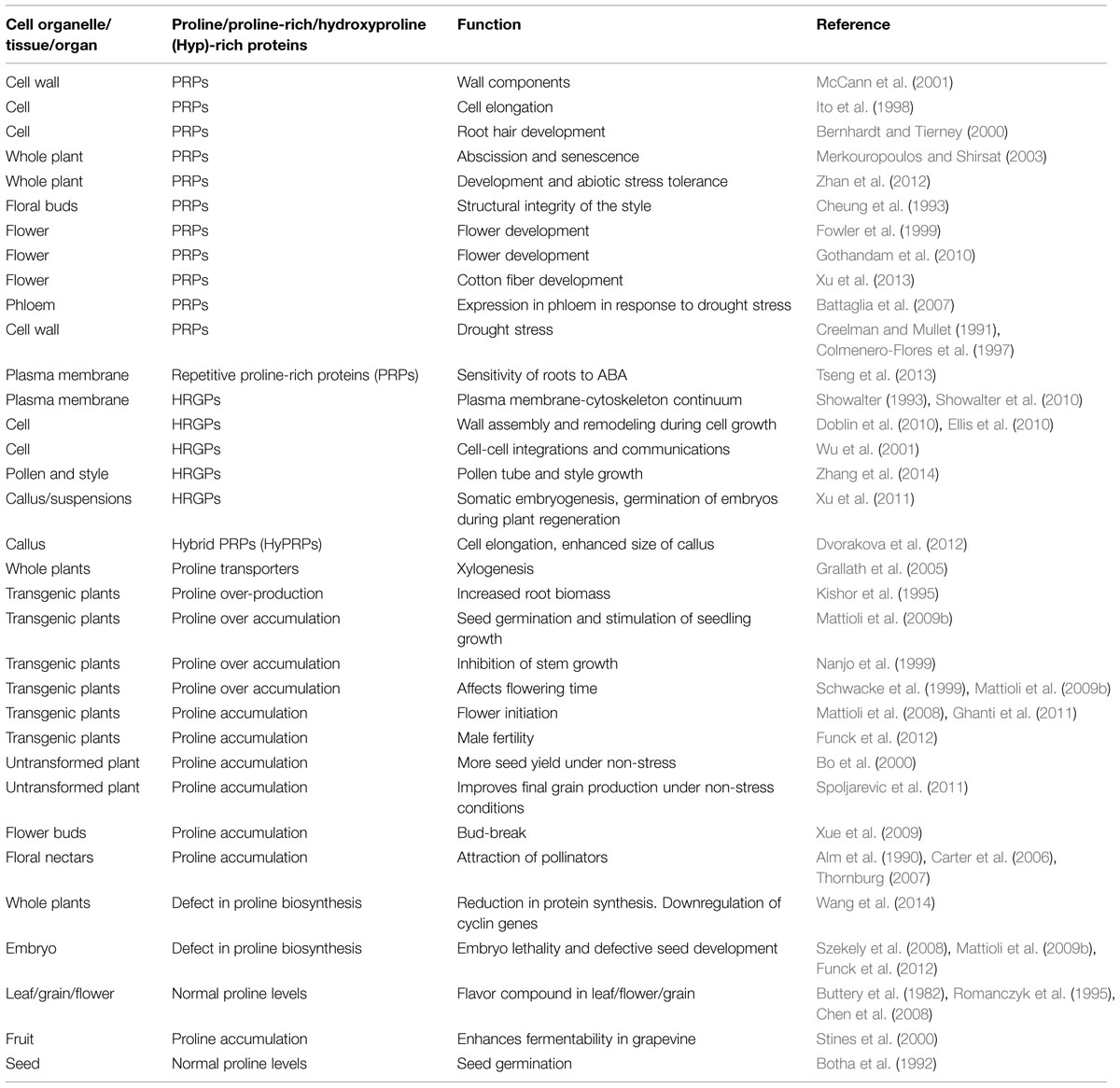
TABLE 1. Multidimensional roles of proline in different cell organelles, tissues, organs, and whole plants.
Significance of Proline in Developing Seeds and in Protein Synthesis
It is known that proline plays a vital role in regulating general protein synthesis in plants. Seed mutants with opaque phenotypes have been discovered in maize (Schmidt et al., 1987; Coleman et al., 1995; Kim et al., 2006). These mutants have been found to be associated with zein (a prolamin protein in seeds) synthesis (Holding et al., 2010; Wang et al., 2011). The auxotrophic recessive opaque mutant in maize, named as Proline responding1 (pro1), displays not only collapsed starchy endosperm morphology but also seedling lethality (Gavazzi et al., 1975; Ma and Nelson, 1975). Later, it was found out that mutants can be restored as normal phenotypes with the supply of exogenous proline (Racchi et al., 1978; Tonelli et al., 1984) and they have predicted that these mutants might have a defect in the biosynthesis of proline (Tonelli et al., 1986). Recently, Wang et al. (2014) reported positional cloning and functional characterization of proline responding1 gene (pro1). The conclusion of Wang et al. (2014) that maize Pro1 encodes a P5CS2 is based on the phylogenetic tree built on the P5CS full-length protein sequences taken from the genomes of A. thaliana, rice and maize. It has been found that this protein is localized in cytoplasm and expressed in roots, stems, silk, tassels, and kernels in these mutants and external feeding of proline rescued the viability of pro1 seedlings. They also unraveled the mechanism related to this mutation. In these pro1 mutants, lack of proline results in enhanced accumulation of uncharged tRNAproAGG. But, such an accumulation leads to a sequence of consequences, first the phosphorylation of eukaryotic initiation factor 2α (elF2α) in the mutant pro1 and subsequently a reduction in general protein synthesis. Thus, in pro1 mutants, lack of proline affects protein accumulation. Interestingly, Wang et al. (2014) further revealed that proline deficiency also leads to downregulation of cyclin genes at the transcriptional level and thus the cell cycle in the pro1 mutants is significantly affected. RT-PCR analysis revealed that CDC6 and MCM7 genes encoding proteins involved in the formation of prereplicative complex are downregulated in pro1 mutants, but external supply of proline induces the expression of A, B, and D cyclins, H2, H3, and H4 histones and DNA replication related genes in pro1 mutants (Wang et al., 2014). These studies point out that lack of proline affects cyclin genes at the transcriptional level and ultimately protein accumulation in seeds.
Proline Accumulation and Root Biomass
Though proline is produced at low levels in all tissues in unstressed conditions, it is actively transported to the roots under stress conditions. Further, it is compartimentalized into the mitochondria where it is degraded back to glutamate releasing energy in the form of FADH2 and NAD(P)H by two different enzymes (Szabados and Savoure, 2010). At low water potentials, maize primary roots have been shown to accumulate proline (Ober and Sharp, 1994). Maintenance of root elongation at low water potential depends on enhanced ABA content. When endogenous ABA levels were decreased either by using fluridone or vp5 mutant, proline concentrations also decreased in the root elongation zone. But, proline concentrations in fluridone treated roots were restored back by the addition of 7 μM ABA. These experiments demonstrate that increased ABA is necessary for the deposition of proline in maize primary root growth zone. Voetberg and Sharp (1991) also demonstrated that proline levels are high toward the root apex (120 m.mol growing at a water potential of -1.6 mpa), where elongation rates of roots are maintained under a range of water deficit conditions. They suggested that proline deposition in the growing root zone is important for osmotic adjustment. But, how proline deposition plays a role in the maintenance of root elongation at low water potentials is not clearly known. Verslues and Sharp (1999) found out that proline synthesis in maize root tips from [3H]glutamate and [14C]ornithine did not increase significantly at low water potential and accounted for only a small fraction of the proline deposition. Proline is also not catabolized and utilized in the root tips under low water potential. But, increased uptake was noticed suggesting increased transport of it. Removal of endosperm from the germinated seedlings decreased the proline accumulation in root tips at low water potentials in maize (Raymond and Smirnoff, 2002). They conclude that endosperm is the source of proline accumulation in the root tips of intact seedlings constituting 10% of the free amino acids released from endosperm. Labeling experiments also revealed that proline is transported from the scutellum to other parts of the seedlings and peaked in the root tips (Raymond and Smirnoff, 2002). Though the biosynthetic capacity of roots to produce proline is low, proline accumulation perhaps is regulated by the rates of its transport and utilization. A unique salt-inducible proline transporter was isolated from barley (Hordeum vulgare) and found to express strongly in root cap cells under salt stress (Ueda et al., 2001). Hence, it may play a role in the root tip region when plants are exposed to salt stress. Spollen et al. (2008) analyzed the spatial distribution of transcript changes in the maize primary root elongation zone at low water potential. They concluded that different signaling and metabolic response mechanisms (wall loosening proteins in region I, and for elements of ABA and ethylene signaling) are involved in the response to water stress in different regions of the maize primary root elongation zone. Further, overexpression of Vigna P5CS in tobacco (Kishor et al., 1995) and Sorghum bicolor (Reddy et al., 2015) resulted in better root biomass under salt stress conditions. Proline accumulation by overexpression of P5CSF129A, a mutated version of Vigna P5CS in transgenic chickpea (Ghanti et al., 2011) also resulted in root elongation and higher root biomass both under salt and drought stress conditions. It appears therefore, proline may play a direct or indirect role in enhancing root biomass in transgenics under stress conditions perhaps by supplying necessary energy [FADH2 and NAD(P)H] and nitrogen.
Several studies related to seed germination have suggested that oxidative pentose phosphate pathway (OPPP) is important in triggering seed germination (Botha et al., 1992; Hare and Cress, 1997). Since this pathway generates NADPH, the energy may be utilized for anabolic reactions during seed germination (Besse and Buchanan, 1997). Shetty (2004) also suggested a link between proline and OPPP. But, the molecular events leading to upregulation of OPPP and its precise role in triggering seed germination is not known completely. Hare et al. (2003) have analyzed the dehydrogenase enzymes involved in OPPP during Arabidopsis seed germination and found activation of this pathway along with fourfold increase in proline content before the emergence of the radicle. When AtP5CS1 gene was inserted in an antisense orientation into Arabidopsis, delayed emergence of radicle was noticed. Proline synthesis might replenish the NADP+ pool and therefore activate OPPP. This indicates a functional link between elevated proline biosynthesis and enhanced OPPP activity and coupling of both the pathways may be important in stimulating germination.
Effects of Proline Accumulation in Reproductive Tissues under Non-Stressed Conditions
Under normal physiological (non-stressed) conditions, plants accumulate large amounts of proline during the transition to flower initiation (Chiang and Dandekar, 1995; Schwacke et al., 1999; Mattioli et al., 2009b), a common phenomenon but often an overlooked fact. Similarly, proline content in tomato flowers was 60 times higher than any other vegetative tissue and >70% of total free amino acids was noticed in pollen grains (Schwacke et al., 1999). A wide range of plants like mature fruits of citrus (Clements and Leland, 1962), pollen grains of petunia and tomato (Zhang et al., 1982; Fujita et al., 1998), ovules of broad bean (Venekamp and Koot, 1984), inflorescences and siliques of Brassica napus (Flasinski and Rogozinska, 1985) also contain very high levels of proline. Accumulation of proline is due to upregulation of proline biosynthetic pathway gene P5CS, downregulation of ProDH and activation of a proline transporter T (ProT) in flowers (Savoure et al., 1995; Rentsch et al., 1996; Schwacke et al., 1999; Hayashi et al., 2000). Proline accumulation predominantly in reproductive tissues suggests that it may be associated with their development (Chiang and Dandekar, 1995; Schwacke et al., 1999). It is also transported to reproductive organs as has been pointed out by Rentsch et al. (1996) and Fischer et al. (1998). These findings suggest that proline may play a role during flower initiation and its subsequent development.
Overexpression of P5CS in tobacco resulted in higher accumulation of proline, and enhanced flowering with more number of flowers per plant and coflorescence (cluster of flowers) formation under stress conditions (Kishor et al., 1995). Over expression of P5CS1 and over-accumulation of proline lead to early flowering and bolting promotion during the early stages of plant development in Arabidopsis (Mattioli et al., 2008). But, during the later stages, downregulation occurred probably because of gene silencing, leading to low proline and bushy appearance of the plants. On the contrary, antisense expression of P5CS1 inhibited bolting in Arabidopsis (Nanjo et al., 1999). The p5cs1 loss-of-function mutants exhibit delay in flowering implying that P5CS1 plays an important role in flowering time (Mattioli et al., 2009b). They also noticed that down-regulation of P5CS1 caused reduction in proline content, impaired flowering, and stunted growth. Funck et al. (2012) made a detailed analysis of the proline biosynthetic pathway enzymes to dissect out their roles during vegetative and reproductive development of Arabidopsis. In their conditions, they found alteration in flowering time in p5cs1 mutants, but significant flowering delay in p5cs2 mutants. It is hypothesized that the flowering delay of the 35S-P5CS1 plants as reported by Mattioli et al. (2008) could be accounted for by the homology-based co-silencing of P5CS2 triggered by the expression of P5CS1. Samach et al. (2000) found that AtP5CS2 is a target of CONSTANS (CO), a transcriptional activator associated with flowering time. Besides, rolD oncogene from Agrobacterium rhizogenes is known to encode a functional ornithine cyclodeaminase enzyme in bacteria which ultimately converts ornithine to proline (Trovato et al., 2001). The main trait induced by overexpression of rolD gene in tobacco is precocity in flower setting and a strong enhancement of the flowering potential in tobacco (Mauro et al., 1996) and tomato (Bettini et al., 2003). These experiments thus point out that proline plays a vital role during flower transition, bolting, and in changing the architecture of inflorescence (coflorescence formation). However, we are not sure about the molecular events leading exactly how proline influences flowering time and changes the inflorescence architecture in higher plants when produced in optimum concentrations.
Proline Contribution to Plant Cell Wall Architecture in Root Development and Reproductive Organs
It is a fact that proline and Hyp are the major amino acid constituents of hydrolysates of cell wall proteins. As pointed out by Kaplan and Hagemann (1991), morphogenesis takes place in plants because of differential growth of organs at the cell wall. Therefore, studies on cell walls are vital though they are highly intricate and complex. Cell wall matrix encoding proteins are enriched with proline residues which are integrated in the form of HRGPs classified into three categories: (i) moderately glycosylated EXTs; (ii) hyperglycosylated arabinogalactanproteins (AGPs); and (iii) Hyp/proline (Pro)-Rich proteins (H/PRPs).
Role of Extensins in Cell Wall Modification During Root and Pollen Development
The extracellular matrices of plants and animals contain structural glycoproteins which are important scaffolding components. Some of these glycoproteins like collagens in animals and HRGPs of plants share functions like enhancing tensile strength (Shirsat et al., 1996) and contributing tissue integrity. In all the three subgroups, proline, and Hyp residues are abundant and play an important role in cell wall development. EXTs are self-assembling amphiphiles. They are modular in nature and contain Hyp and are usually characterized by pentameric Ser-Hyp peptide repeats. EXTs exhibit 35–65% glycosylation on Ser-(Hyp)n ≥ 2 motifs and the side chains contain oligosaccharides of arabinose and galactose (Showalter, 1993; Kieliszewski and Lamport, 1994). The genome of A. thaliana encodes 63 putative EXTs with high amino acid sequence similarity between them. Out of the 63 members, some are classical, while others are EXT-like chimeras and hybrid EXTs (Lamport et al., 2011). It is not clear whether they have redundant or specific functions to carry in different cell types of various plant tissues. EXTs exhibit repetitive nature of their sequences with many post-transcriptional modifications in plant genomes like signal peptide processing in the ER, hydroxylation of proline to Hyp residues by P4H, O-glycosylation on Hyp and Ser residues with chains of up to four linear Ara residues on each Hyp by arabinosyltransferases (Velasquez et al., 2011; Ogawa-Ohnishi et al., 2013), and cross-linking of glycosylated EXTs at the tyrosine residues in the cell wall (Jackson et al., 2001; Price et al., 2003; Lamport et al., 2011; Hijazi et al., 2014) forming intra- and inter- EXT linkages (Nunez et al., 2009; Dick-Perez et al., 2011). This helps EXTs to form a three-dimensional structure of protein and to interact with pectins in the cell walls (Dick-Perez et al., 2011). The coordination of EXTs with other components and their functions during cell wall architecture and plant development are not completely known. This is mostly because of their modular nature, existence in large numbers, hydroxylation by different P4Hs, complex structure, and redundant expression of these proteins in the same plant tissues (Genevestigator database, https://www.genevestigator.com). EXTs can also interact with pectins and serve as templates for cell wall deposition (Cannon et al., 2008; Lamport et al., 2011). Recently, Tan et al. (2013) found an ARABINOXYLAN PECTIN ARABINOGALACTAN PROTEIN1 (APAP1) in A. thaliana which consists of pectin and arabinoxylan that are covalently linked to an AGP. They suggested a role for APAP1 proteoglycan in plant cell wall architecture and function. However, the exact coordinated control of EXTs and pectin interaction during cell wall formation is unclear.
Several groups have used root hair as a model to study the functions of EXTs (Park et al., 2011; Velasquez et al., 2011). Mutants lacking the synthesis of wall polymer in the root hair are impaired in growth (Diet et al., 2006; Ringli, 2010; Park et al., 2011; Velasquez et al., 2012). Since posttranslational modifications of HRGPs are carried out by (P4Hs), this defines the subsequent O-glycosylation sites in EXTs (mostly arabinosylated) and polarized growth in root hairs. O-glycans enhance the HRGP solubility and resistance to proteolytic degradation (Kieliszewski et al., 1989; Shpak et al., 2001; Lamport et al., 2011). Velasquez et al. (2011) proved that O-glycosylation on EXTs is essential for cell-wall assembly and root hair elongation. Root hairs develop by tip growth akin to pollen tubes, axons, and fungal hyphae. Velasquez et al. (2015) recently carried out genetic analysis related to the regulation of P4H. They proved that P4H5 and P4H2 and P4H13 are pivotal for root hair tip growth. The impact of deficient proline hydroxylation on the cell wall architecture has also been shown by them. Their results demonstrate that peptidyl-proline hydroxylation on EXTs is required for cell wall assembly and therefore root hair elongation in Arabidopsis. The above works point out that changes in the O-glycosylation status impact the functions of EXTs during cell wall assembly, cell shape, and expansion. Genes that encode P4Hs are also linked to hypoxia, and P4H proteins are regarded as oxygen sensors under hypoxic stress. Zou et al. (2011) found out that P4H genes are subjected to alternative splicing in roots of maize seedlings under waterlogging conditions. The diverse transcripts generated due to alternative splicing are expressed at different levels clearly indicate that ZmP4H genes are under specific control by post-transcriptional regulation under waterlogging stress in maize. Hall and Cannon (2002) identified that cell wall Hyp-rich glycoprotein ROOT-SHOOT-HYPOCOTYL-DEFECTIVE (RSH) is necessary for normal embryo development in Arabidopsis. Xu et al. (2011) further found out the role of HRGPs during somatic embryogenesis of Musa sp. Their results suggest that HRGPs play a vital role in regeneration and germination of embryos during early plant development via somatic embryogenesis (Table 1). Proper localization and appropriate quantities of HRGPs seem to be critical for the formation and regeneration of somatic embryos. But, the exact mechanism by which HRGPs trigger somatic embryogenesis in callus or suspension cultures is not known. Thus, the functions proline-rich and Hyp-rich glycoproteins appear to be complex, but clearly associated with developmental regulation in different plant systems. Using protein blots and immunohistochemistry, EXTs have been found to be abundantly expressed in vivo for pollen tubes and transmitting tissues (Zhang et al., 2014). When inhibitors of HRGP were used, decreased pollen tube growth and stylar length were recorded. It appears that HRGPs especially EXTs play a critical role in the pollen tube and style cell growth. Wu et al. (2001) demonstrated that EXTs are involved in pollen recognition and fertilization. These evidences along with the works of Cannon et al. (2008), Ringli (2010), Lamport et al. (2011), and Velasquez et al. (2012) infer that cross-linked EXTs play pivotal roles during cell expansion and growth of pollen tube.
Arabinogalactan Protein Involvement in Vegetative and Reproductive Growth
Arabinogalactan proteins are Hyp-rich extracellular proteins with a high proportion of sugars (up to 90%). Among them, arabinose and galactose are predominant residues, but minor sugars like rhamnose, fucose, glucuronic, acid, and xylose are common (Nothnagel, 1997; Showalter, 2001). AGPs are characterized by extensive glycosylation with arabinose, galactose or both and analogous to animal proteoglycans (Clarke et al., 1979; Lord and Sanders, 1992). AGPs possess repetitive Alanine (Ala) or Serine (Ser) motifs that interact with Hyp molecules. They are chemically stable, and resistant to proteolytic enzymes in their native state due to the presence of carbohydrates (Fincher et al., 1983). AGPs contain glycosylphosphatidylinositols (GPIs) which tethers them to the plasma membranes in plants (Schultz et al., 2004). The biosynthesis, structure, expressions, and functions of AGPs have been well studied and reviewed (Showalter, 2001; Ellis et al., 2010; Tan et al., 2012; Lamport and Várnai, 2013; Nguema-Ona et al., 2013; Knoch et al., 2014). Cassab (1998) pointed out that carbohydrate groups (D-galactose and L-arabinose) are mostly linked by O-glycosylation to the hydroxyl group of Ser and Hyp of the AGP backbone. However, diversity exists in the degree and pattern of O-glycosylation depending on the type and length of glycan chains attached to the proteins. Many studies suggest that AGPs play important roles in vegetative and reproductive growth. AGPs were observed throughout the plant kingdom, in leaves, stems, roots, floral parts, and seeds (Fincher et al., 1983; Nothnagel, 1997). They were found in plasma membranes, cell walls, intracellular multivesicular bodies, and also as secretions to intercellular spaces (Nothnagel, 1997).
Some AGPs are also implicated with redundant architectural functions of cell walls (Bosch et al., 2001). Several lines of evidence indicate that AGPs are associated with growth and differentiation in lower as well as in higher plants. For example, when inhibitors of AGP biosynthesis were used, the growth of leaf primordia was suppressed in a liverwort (Basile and Basile, 1993). Yariv reagent inhibited cell division in cell suspension cultures and roots in carrot and Arabidopsis, respectively, (Willats and Knox, 1996; Ding and Zhu, 1997). This indicated that AGPs are involved in cellular growth and development. While certain AGPs promote somatic embryogenesis in Daucus carota, (Kreuger and van Holst, 1993), and Picea abies (Egertsdotter and von Arnold, 1995), others inhibit it as noticed by Toonen et al. (1997) in carrot.
AGPs were noticed in xylem, stylar transmitting tissues and cell suspension cultures. In targeting certain AGPs to the specific cell surface locations, the role of certain sequence determinants in the carbohydrate or protein need to be determined. Their multi-location (organs, tissues, and cell-types) occurrence indicates specific functions of AGPs in different tissues like flowers and other cell types (Pennell and Roberts, 1990). Many AGPs possess a C-terminal Ole e 1 domain (pollen allergens, named after a secreted protein isolated from Olea europeae pollen; Villalba et al., 1994). Developmental and tissue specific expressions were noticed for several AGPs in different plants (Pennell et al., 1991; Chen et al., 1994; Gao and Showalter, 2000), consistent with the assumption that they play a role in plant development. Altered expression of AGP in pea plants has changed the sexual development as shown by Pennell and Roberts (1990). Some AGPs like stylar transmitting tissue-specific PRPs (TTS proteins) play vital roles in reproductive growth and development like pollen-pistil interactions (Bosch et al., 2001; Hancock et al., 2005). When the gene knockdown experiments were carried out, TTS proteins have been found important for pollen–pistil interactions. This may be possible for TTS proteins which act as cell surface adhesives (Wu et al., 2000). AGPs are also implicated in cellular signaling events (Schultz et al., 1998). They are the important components in plant exudates and gums, as shown in the case of gum arabic extracted from Acacia senegal (Serpe and Nothnagel, 1999) and used as an additive in the food industry. Type II arabinogalactans have also been found to stimulate animal immune systems and thus may be useful in medicine as well (Yamada and Kiyohara, 1999). Further, chimeric proteins containing AGP domains may interact with polysaccharides. In this connection, the experiments of Griffiths et al. (2014) have proved that CELLULOSE SYNTHASE5 (CESA5) and the SALT-OVERLY SENSITIVE5 (SOS5), a Fasciclin-AGP are required for mucilage adherence in seeds of A. thaliana. They conclude that SOS5 mediates adherence through pectin (Griffiths et al., 2014). AGPs are involved in cell–cell/intercellular communications involved in tracheary element differentiation (Motose et al., 2001) and thus AGPs are found to be associated with the differentiation of xylem and programmed cell death (Schindler et al., 1995; Gao and Showalter, 2000). Thus, AGPs perform several functions in different aspects of plant growth and development, though the underlying molecular mechanisms are not clearly known.
Pursuit of Hyp/Pro-Rich Proteins (H/PRPs) in Plant Development
Hyp/Pro-rich proteins belong to the HRGP superfamily like that of EXTs and AGPs. Based on repetitive motifs and domain organization, PRP proteins are classified into three subtypes; (a) repetitive PRPs, (b) non-repetitive PRPs, and (c) multi-domain hybrid PRPs (Jose-Estanyol and Puigdomenech, 2000). Proline residues in PRPs are hydroxylated and form Hyps which are then glycosylated as mentioned earlier. But, O-glycosylation of H/PRPs and their interactions with polysaccharides is not completely known. Twelve H/PRP proteins have been identified so far in Arabidopsis thaliana, carrot, cotton, tobacco, common bean, capsicum, and petunia (Hijazi et al., 2014). Bradley et al. (1992), and Frueauf et al. (2000) pointed out that H/PRPs may be cross-linked in cell walls, but evidence is lacking for such an assumption.
Pro-rich proteins are involved in cell elongation (Ito et al., 1998), abscission and senescence (Merkouropoulos and Shirsat, 2003). As shown in Table 1, HRGPs are essential for the structure and function of cell wall as well as plasma membrane-cytoskeleton continuum (Showalter, 1993; Showalter et al., 2010). It is highly interesting to note that the PRP genes show developmental stage-, organ-, tissue-, and even cell-specific expressions (Hong et al., 1989; Ye and Varner, 1991; Wyatt et al., 1992). Hong et al. (1987, 1989) reported the characterization of three developmentally regulated proline-rich cell wall protein genes from Glycine max. Marked differences were observed in the pattern of expression of PRPs in different organs like hypocotyls, roots, stems (Wyatt et al., 1992), immature embryos (Jose-Estanyol et al., 1992), immature seed coats and developing seeds and in cells that are undergoing lignifications (Vignols et al., 1999). Fowler et al. (1999) characterized and expressed four proline-rich cell wall protein genes in Arabidopsis encoding two distinct subsets of multiple domain proteins. Their studies support a model for the involvement of PRPs in specifying cell-type specific wall structures, and provide the basis for a genetic approach to dissect the function of PRPs during growth and development. While S. bicolor PRP1 (SbPRP1) mRNA is abundant in elongating regions of hypocotyl epidermal cells, SbPRP2 mRNA is more expressed in phloem cells. Further, it has been found that SbPRP3 mRNA is localized in the endodermoid layer of cells in the hypocotyl elongating region (Wyatt et al., 1992). Bernhardt and Tierney (2000) demonstrated that expression of AtPRP3, a proline-rich structural cell wall protein is regulated by cell-type-specific developmental pathways involved in root hair (single cells that develop by tip growth and are specialized in absorption of nutrients) formation. They noticed enhanced expression of AtPRP3/β-glucuronidase (GUS) in roots of transgenic seedlings treated with 1-aminocyclopropane-1-carboxylic acid (ACC) or α-naphthaleneacetic acid, two compounds known to promote root hair formation. The results indicate that AtPRP3 is regulated by developmental pathways involved in root hair formation (Bernhardt and Tierney, 2000). Overexpression of GhPRP5 in A. thaliana resulted in smaller cell size, but knock-down of GhPRP5 expression by RNA interference in cotton increased the fiber length (Xu et al., 2013). Their data suggest that this protein participates in modulating fiber development of cotton.
Involvement of PRPs as Signaling Proteins in Reproductive Tissues
Pro-rich proteins perform a whole spectrum of functions like providing structural integrity to mediate cell–cell integrations and communications (Wu et al., 2001). They are also involved and play a vital role in wall assembly and remodeling during cell growth and development (Ellis et al., 2010; Doblin et al., 2010). Gibbon et al. (1998) characterized several profilin isoforms from maize pollen and showed that their function depends on interaction with proline-rich motifs. They found out that profilin isoforms expressed in a single cell can have different effects on actin in living cells and the poly-L-proline binding function of profilin may have important consequences for the regulation of actin cytoskeletal dynamics. Two stylar transmitting tissue specific PRPs (TTS-1 and TTS-2) have also been reported in tobacco (Cheung et al., 1993). TTS-1 and TTS-2 mRNAs have been induced in floral buds, during the later stages of flower development especially when style elongation is rapid and are active at anthesis (Cheung et al., 1993). The occurrence of PRPs in transmitting tissues has been predicted to play a vital role in maintaining the structural integrity of the style. Transgenic Arabidopsis overexpressing Atext1 displayed altered inflorescence and affected stem thickening and height (Roberts and Shirsat, 2006). However, the functions of all PRPs are not completely known during different developmental stages, thus leaving a gap in our understanding of PRPs.
Role of PRPs in Cell Wall Modification under Stress
Genes that encode PRPs and the localization of these proteins in plant cell walls have been studied (Cassab and Varner, 1988; Showalter and Varner, 1989; Cassab, 1998). During growth, differentiation and also during different environmental stress conditions, cells may expand up to 200 times their original length. Water stress could result in alterations in cell volume and shape, as well need to deal with loss of turgor (Wakabayashi et al., 1997). Therefore, cell walls must possess tensile strength to withstand the turgor pressures. This involves very large chemical and biochemical modifications of cell wall constituents including cell wall proteins representing 1 to 2% of the genome in plants such as Arabidopsis (Somerville et al., 2004). Cell wall protein transcripts are also affected under salt, drought, and temperature stress conditions (Covarrubias et al., 1995). PRPs are insolubilized in the cell walls with the involvement of H2O2-mediated oxidative cross linking and it precedes the expression of transcription-dependent defences. Though cell wall bound PRPs may play a role in the structural integrity of plant cells, participate in defence related activities, and plant cell surface interactions as pointed out by Roberts (1989), Varner and Lin (1989), interestingly, the mRNA levels of PRPs increase in response to water scarcity in higher plants (Creelman and Mullet, 1991; Colmenero-Flores et al., 1997).
Battaglia et al. (2007) characterized two proline-rich glycoproteins of 33 and 36 kDa (p33 and p36) which are found in the cell wall soluble fraction of common bean (Phaseolus vulgaris). They observed the highest accumulation of these proteins in growing regions, predominantly in phloem tissues in response to drought indicating that cell wall modifications are induced in actively growing cells of common bean. Gothandam et al. (2010) reported a flower specific PRP from rice (OsPRP3) that is expressed during the late stages of flower development. While AtPRP3 is associated with root hair formation, overexpression of Oryza sativa PRP3 (OsPRP3) showed an increase in cold tolerance compared to the wild-type plants. They showed that this OsPRP3 enhances cell wall integrity in the cold tolerant plants. Knockout mutants displayed defects in floral organogenesis suggesting a role for PRPs in reproductive tissues. Tseng et al. (2013) reported ABA-induced expression of a family of four genes, REPETETIVE PROLINE-RICH PROTEIN (RePRP) in rice. These genes encode proline-rich glycoproteins with highly repetitive PX1PX2 motifs, RePRP1, and RePRP2. Their work also revealed that ABA treatment increases RePRP expression and that these proteins are localized to the plasma membrane. It has been found that knockdown of the expression of RePRP1 and RePRP2 decreases the sensitivity of roots to ABA. Thus, these experiments reveal that RePRP proteins play an essential role in ABA/stress regulation of root growth and development. It has also been noticed that rice RePRPs interact with arabinogalactan polysaccharide in a dosage-dependent manner (Tseng et al., 2013). Zhan et al. (2012) recently identified a PRP called SICKLE (SIC) in Arabidopsis. This protein is critical for not only development in Arabidopsis but also in imparting abiotic stress tolerance. The loss-of-function sic-1 mutants displayed reduction in plant height, delay in flowering, and abnormal inflorescence phyllotaxy. These mutants accumulated reduced levels of a subset of miRNAs and transacting siRNAs but enhanced levels of primary miRNAs than the controls. The sic-1 mutant plants are also sensitive to cold and salt stresses (Zhan et al., 2012). Therefore, it appears that SIC is a PRP associated with the biogenesis of some miRNAs and degradation of some spliced introns and is vital for plant development.
Hybrid Proline-Rich Proteins (HyPRPs) are Crucial Players in Cell Elongation
Out of the three subclasses of PRPs, one of them contains several copies of POVEKPOVXK motif (Hong et al., 1990), while the other two subclasses show a hybrid structure and are called hybrid proline-rich proteins (HyPRPs). Plant HyPRPs represent putative cell wall proteins consisting of a repetitive proline-rich N-terminal domains and a conserved C-terminal domain. They are unique to seed plants and analysis of the families from different plant species suggests rapid diversification of their sequences and expression patterns (Dvorakova et al., 2007). Their functions are not clearly known, but their occurrence indicates that they may be involved in basic cellular processes (Dvorakova et al., 2012). To understand the functions of HyPRPs, Dvorakova et al. (2012) modulated the expression of three HyPRP genes in tobacco and potato. Transgenic plants displayed cell elongation, and enhanced size of calli. HyPRPs contain two regions, and one of them is rich in proline and the other in cystein residues (Deutch and Winicov, 1995). Further, it has been shown that the third sub-class, namely NHyPRPs displays high percentage of proline residues organized in repetitive sequence motifs in C-terminus region (Castonguay et al., 1994; Menke et al., 2000). Thus, it appears that HyPRPs are important players in plant cell elongation, though the molecular mechanism is not yet elucidated.
Proline-Rich Extensin-Like Receptor Kinases (PERKs)
A family of receptor kinase-like proteins has been discovered that are involved in cell wall signaling. In A. thaliana, 11 members of the PERK family have been discovered which share some features of EXTs. Altered expression of PERK receptor kinases resulted in changes of plant growth and floral organ formation (Haffani et al., 2006). In a novel work, it has been elucidated by Bai et al. (2009) that the gene PERK4 (coding for a protein kinase located in the plasma membrane) is required for the ABA-dependent influx of Ca2+ and normal ABA sensitivity in seeds and roots of A thaliana. Their experiments suggest that PERK4 is associated in ABA perception and also might interact with wall polymers. Thus, PRPs are implicated in cell wall signal transduction cascades. Further work is necessary since it is not yet clear about how many PERK proteins are associated with this process and also their additional roles if any during growth and stress tolerance.
Proline Deficiency Affects the Biosynthesis of Cell Wall Matrix Proteins
The experiments conducted by Cooper et al. (1994) demonstrated initially in tobacco that HRGPs are critical for cell morphology and osmotic stability. Strong evidence has come later from Nanjo et al. (1999) who created Arabidopsis plants with antisense P5CS. Interestingly, the transgenics displayed morphological defects in leaves and elongation of inflorescences. Further, they observed that protein biosynthesis was normal in proline deficient plants, but structural proteins localized in the cell walls were strikingly altered (Nanjo et al., 1999) indicating a direct evidence for a key role of proline in cell walls. Specifically, proline and Hyp contents were reduced in the cell wall preparations of Arabidopsis transgenic leaves. They pointed out that PRPs and HRGPs are responsible for morphological abnormalities observed in the leaves of antisense Arabidopsis plants since these proteins provide mechanical support for cells under stressed conditions. Such a role in cell morphology and the mechanical support for the PRPs and HRGPs also has been demonstrated by Showalter (1993) and Munoz et al. (1998). Thus, proline-related growth abnormalities are correlated well with the effects of proline on plant cell wall development. Wang et al. (2014) found out that proline deficiency causes downregulation of cyclin genes and affects general protein synthesis. However, they did not find out its effect on the cell wall proteins. It would be interesting to find out if there would be any changes that take place in H/PRPs associated with cell wall development. Several overexpressions and knockout mutations of plants containing PRPs and HRGPs are needed to demonstrate the exact role(s) of these proteins during cell wall synthesis and growth.
Proline Transporters Play an Important Role in Plant Development Including Xylogenesis
Proline transporters have been isolated from several species and three from Arabidopsis (AtProTs) so far (Rentsch et al., 1996; Schwacke et al., 1999; Fujiwara et al., 2010; Lehmann et al., 2011). Transcript levels of Arabidopsis ProT2, mangrove ProT homologues, barley HvProT as well as proline levels are elevated under salt stress (Rentsch et al., 1996; Hibino et al., 2001; Ueda et al., 2001; Waditee et al., 2002). Like in bacteria, proline transporters also act as glycinebetaine transporters in plants but with different affinities. Further, they also transport other compatible solutes like γ-aminobutyric acid (GABA) in plants (Grallath et al., 2005; Fujiwara et al., 2010). Though intracellular localization, substrate selectivity, and affinity of the three AtProTs are similar, they exhibited differential expression patterns in various plant organs indicating differential transport of these molecules. By fusing transporter genes with GUS, they demonstrated that AtProT1, AtProT2, and AtProT3 are localized in lignified tissues like phloem of all organs, epidermis, and cortex of roots and in epidermis of leaves respectively, (Grallath et al., 2005). ProT1 isolated from tomato has shown to be expressed specifically in pollen grains (Schwacke et al., 1999). Further, Lehmann et al. (2010) clearly showed a relation between uneven distribution of proline in the lower epidermis and rest of the leaf with the expression levels of AtProT3. In spite of the fact, that single, double, and triple knockout mutants of the AtProT1, AtProT2, and AtProT3 genes responsible for proline transport in plants have been isolated and characterized, these mutants did not reveal any differences when compared to wild type plants (Lehmann et al., 2011). This underlays the complex situation and therefore needs further elucidation. The functions of these transporters might be highly redundant and possibly complemented by other transporters, since transporters mediating proline uptake across the plasma membrane have also been noticed in the amino acid transporter (ATF) or amino acid/auxin permease (AAAP) and in the amino acid-polyamine-choline (APC) families (Rentsch et al., 2007). Along with glutamate, amino acid permease (AAP) family mediates proton coupled uptake of neutral amino acids such as proline (Frommer et al., 1993; Lee and Pallas, 2007; Schmidt et al., 2007). Couturier et al. (2010) isolated AAP11 from Populus trichocarpa and overexpressed it in poplar and yeast. PtAAP11 was highly expressed in differentiating xylem cells in different organs. Further, functional characterization revealed that it is a high affinity amino ATF, more particularly for proline. Therefore, the authors suggested that PtAAP11 may play an important role in xylogenesis by providing proline which is required for xylem cell wall proteins in poplar. Lysine-histidine transporter (LHT) family transports neutral and acidic amino acids including proline (Lee and Tegeder, 2004). Contrary to the AAP and LHT families, ProTs transport proline and other compatible solutes like GABA but not other proteogenic amino acids (Rentsch et al., 1996). The fact that both low and high affinity-proline transporters exist in plants implicate that they play a role in general transfer of nitrogen and also proline for its specific functions in different cells and tissues. Selective transport of proline by ProTs and also their expression analysis during drought/salt stresses or in pollen grains suggests that they play a vital role in proline homeostasis both under stress and non-stress conditions (Rentsch et al., 1996; Ueda et al., 2001; Waditee et al., 2002; Lehmann et al., 2010). The list of proline/betaine transporters and transport mutants known from different plants is shown in Table 2. In summary, proline transporters (AAPs) play an important role in plant development by providing proline as a source of nitrogen and energy which is required for lignifications, xylem differentiation and cell wall modification during plant development (as a component of plant cell wall proteins). But the exact mechanisms how proline can mediate these functions are not known.
Mutations in p5cs2 and p5cr Cause Embryo Lethality and Affect Seed Development
The amount of free proline and its metabolism modulates transition to flowering, pollen, and embryo development. Several studies found that immature/mature seeds of Vicia faba and Arabidopsis accumulate proline before ripening (Venekamp and Koot, 1984; Chiang and Dandekar, 1995; Schmidt et al., 2007). In support of it, concomitant expression of genes encoding enzymes of proline metabolism was observed in seeds (Armengaud et al., 2004; Deuschle et al., 2004; Hur et al., 2004; Szekely et al., 2008). It was noticed that P5CS2 is expressed mostly in actively dividing meristematic cells, developing tissues, callus, and cell suspension cultures. p5cs2 mutations cause embryo abortion during late stages of seed development though both P5CS mRNAs are detectable throughout embryonic development under warmer climate (Szekely et al., 2008). Their experiments indicated exogenous proline can rescue p5cs2 mutants by ex vivo cultivation of developing seeds, but the mutant plants undergo aberrant development and become sterile. It has also been reported that homozygous p5cs2 mutant plants died before the onset of flowering. Therefore, specific role of P5CS2 in reproductive development could not be analyzed (Szekely et al., 2008; Mattioli et al., 2009b). When Arabidopsis plants were simultaneously silenced or co-suppressed with P5CS1 and P5CS2 genes, they resulted in retarded growth, delayed flowering and reduced apical dominance (Nanjo et al., 1999; Mattioli et al., 2008). The above results indicate that Arabidopsis P5CS1 is insufficient for compensation of developmental defects caused by knockout of P5CS2. Expression of P5CS2-GFP was observed in leaf primordia, where the levels of P5CS1-GFP levels are very low. P5CS2-GFP also displayed cell-type specific subcellular localization pattern compared to P5CS1-GFP in root tips, leaves, flower organs, and embryonic cells (Szekely et al., 2008). Taken together, the data demonstrate that these two genes have non-redundant functions in plants. In A. thaliana, Funck et al. (2012) did not observe developmental defects in single mutants devoid of P5CS1 gene. p5cs1/p5cs2 double mutants displayed pollen sterility, while egg cells are fertile. Thus, these results emphasize the role(s) of P5CS in pollen fertility. p5cs2 T-DNA insertion lines have been described as embryo lethal or conditionally embryo lethal. It has been found that P5CS2 is not essential for sexual reproduction (Funck et al., 2012). They obtained homozygous p5cs2 mutants that are viable and could produce fertile seeds by in vitro culture of immature mutant seeds on MS medium supplemented with 60 mM sucrose and 2 mM proline. This allowed generating homozygous plants that are phenotypically normal, but showed reduced growth compared to the wild type plants. Under short day and low-light conditions, these plants produced viable seeds. Funck et al. (2012) pointed out that embryo lethality in heterozygous atp5cs2 plants might be due to premature desiccation of homozygous embryos that develop more slowly than heterozygous embryos. In a contrast to p5cs1/p5cs2 double mutants, normal pollen fertility was observed in the absence of a functional P5CR gene indicating that P5CR is not needed for pollen fertility. These results implicate that P5CS1/P5CS2 genes are crucial for pollen fertility, but not P5CR. It has been found that p5cr mutations cause embryonic lethality, however. Disruption of P5CR gene using T-DNA insertions resulted in embryo defective mutation emb2722 (Meinke et al., 2008) in Arabidopsis. They reported arrest of the embryo development after the second division of the embryo proper in p5cr mutants. All attempts to rescue putative homozygous p5cr mutant embryos in vitro by proline feeding failed. Their efforts to promote embryo development of homozygous seeds in siliques of heterozygous parents in situ by feeding the proline exogenously or induction of internal proline accumulation through salt stress were not fruitful. Thus, activities of both P5CS and P5CR enzymes have been found essential for sexual reproduction in plants.
Conclusion
Proline not only participates in protein synthesis, but regulates several important functions like osmotic adjustment and protection of proteins during stress conditions. Its multifarious functions are always enigmatic in plants. However, it is increasingly becoming evident that proline modulates a wide-array of functions like regulation of cyclin genes at the transcriptional level, cell wall elongation and modifications, xylogenesis, stem elongation, root, and shoot growth, inflorescence architecture, embryo formation/seed development, and seed germination during the life cycle of a plant. Deficiency in proline biosynthesis leads to abnormal plants and cell wall defects, thus implicating its role in structural proteins. But, clear evidence is lacking for attributing several specific functions to this amino acid. We do not know yet how the genes like P5CS1, P5CS2, P5CR, OAT, PDH, and P5CDH interact with other genes and we still do not have an interactome map of the network of genes. Unless such maps are available along with their experimental validation, we cannot pinpoint the exact metabolic functions of proline other than its role in primary metabolism in protein biosynthesis.
Author Contributions
All the authors of the manuscript meet the essential criteria of the publication. All authors have read and approved the manuscript.
Conflict of Interest Statement
The authors declare that the research was conducted in the absence of any commercial or financial relationships that could be construed as a potential conflict of interest.
Acknowledgments
PK is thankful to the CSIR, New Delhi, for awarding the Emeritus Fellowship (No.21(0934)12/EMR-II). PH is grateful to the CSIR, New Delhi, for providing fellowship in the form of Senior Research Fellowship.
References
Alm, J., Ohnmeiss, T. E., Lanza, J., and Vriesenga, L. (1990). Preference of cabbage white butterflies and honey bees for nectar that contains amino acids. Oecologia 84, 53–57. doi: 10.1007/BF00665594
Armengaud, P., Thiery, L., Buhot, N., March, G., and Savoure, A. (2004). Transcriptional regulation of proline biosynthesis in Medicago truncatula reveals developmental and environmental specific features. Physiol. Plant. 120, 442–450. doi: 10.1111/j.0031-9317.2004.00251.x
Bai, L., Zhang, G., Zhou, Y., Zhang, Z., Wang, W., Du, Y., et al. (2009). Plasma membrane-associated proline-rich extensin-like receptor kinase 4, a novel regulator of Ca signaling, is required for abscisic acid responses in Arabidopsis thaliana. Plant J. 60, 314–327. doi: 10.1111/j.1365-313X.2009.03956.x
Basile, D. V., and Basile, M. R. (1993). The role and control of the place-dependent suppression of cell division in plant morphogenesis and phylogeny. Mem. Torrey Botanical. Club 25, 63–68.
Battaglia, M., Solorzano, R. M., Hernandez, M., Ortiz, S. C., Gomez, B. G., Marquez, J., et al. (2007). Proline-rich cell wall proteins accumulate in growing regions and phloem tissue in response to water deficit in common bean seedlings. Planta 225, 1121–1133. doi: 10.1007/s00425-006-0423-9
Bernhardt, C., and Tierney, M. L. (2000). Expression of AtPRP3, a proline-rich structural cell wall protein from Arabidopsis, is regulated by cell-type-specific developmental pathways involved in root hair formation. Plant Physiol. 122, 705–714. doi: 10.1104/pp.122.3.705
Besse, I., and Buchanan, B. B. (1997). Thioredoxin-linked plant and animal processes: the new generation. Bot. Bull. Acad. Sin. 38, 1–11. doi: 10.1046/j.1469-8137.1997.00784.x
Bettini, P., Michelotti, S., Bindi, D., Giannini, R., Capuana, M., and Buiatti, M. (2003). Pleiotropic effect of the insertion of the Agrobacterium rhizogenes rolD gene in tomato (Lycopersicon esculentum Mill). Theore. Appl. Genet. 107, 831–836. doi: 10.1007/s00122-003-1322-0
Bo, Z., Xiao-Ping, Z., and Zhang-Cheng, Z. (2000). Studies on pollen free proline content and seed yield of Gordonia acuminata in different communities. Acta Phytoecol. Sin. 24, 617–620.
Bosch, M., Sommer-Knudsen, J., Derksen, J., and Mariani, C. (2001). Class III pistil-specific extensin-like proteins from tobacco have characteristics of arabinogalactan proteins. Am. Soc. Plant Phys. 125, 2180–2188. doi: 10.1104/pp.125.4.2180
Botha, F. C., Potgieter, G. P., and Botha, A. M. (1992). Respiratory metabolism and gene expression during seed germination. Plant Growth Regul. 11, 211–224. doi: 10.1007/BF00024560
Bradley, D. J., Kjellbom, P., and Lamb, C. J. (1992). Elicitor-induced and wound induced oxidative cross-linking of a proline rich plant cell wall protein: a novel, rapid defense response. Cell 70, 21–30. doi: 10.1016/0092-8674(92)90530-P
Buttery, R. G., Ling, L. C., and Juliano, B. O. (1982). 2-Acetyl-1-pyrroline: an important aroma component of cooked rice. Chem. Industry (London) 12, 958–959.
Cannon, M. C., Terneus, K., Hall, Q., Tan, L., Wang, Y., Wegenhart, B. L., et al. (2008). Self-assembly of the plant cell wall requires an extensin scaffold. Proc. Natl. Acad. Sci. U.S.A. 105, 2226–2231. doi: 10.1073/pnas.0711980105
Carter, C., Shafir, S., Yehonatan, L., Palmer, R. G., and Thornburg, R. (2006). A novel role for proline in plant floral nectars. Naturwissenschaften 93, 72–79. doi: 10.1007/s00114-005-0062-1
Cassab, G. I. (1998). Plant cell wall proteins. Annu. Rev. Plant Physiol. Plant Mol. Biol. 49, 281–309. doi: 10.1146/annurev.arplant.49.1.281
Cassab, G. I., and Varner, J. E. (1988). Cell wall proteins. Ann. Rev. Plant Physiol. Plant Mol. Biol. 39, 321–353. doi: 10.1146/annurev.pp.39.060188.001541
Castonguay, Y., Laberge, S., Nadeau, P., and Vezina, L. P. (1994). A cold induced gene from Medicago sativa encodes a bimodular protein similar to developmentally regulated protein. Plant Mol. Biol. 24, 799–804. doi: 10.1007/BF00029861
Chen, C. G., Pu, Z. Y., Moritz, R. L., Simpson, R. J., Bacic, A., Clarke, A. E., et al. (1994). Molecular cloning of a gene encoding an arabinogalactan-protein from pear (Pyrus communis) cell suspension culture. Proc. Natl. Acad. Sci. U.S.A. 91, 10305–10309. doi: 10.1073/pnas.91.22.10305
Chen, L., and Bush, D. R. (1997). LHT1, a lysine- and histidinespecific amino acid transporter in Arabidopsis. Plant Physiol. 115, 1127–1134. doi: 10.1104/pp.115.3.1127
Chen, S., Yang, Y., Shi, W., Ji, Q., He, F., Zhang, Z., et al. (2008). Badh2, encoding betaine aldehyde aldehyde dehydrogenase, inhibits the biosynthesis of 2-acetyl-1-pyrroline, a major component in rice fragrance. Plant Cell 20, 1850–1861. doi: 10.1105/tpc.108.058917
Cheung, A. Y., May, B., Kawata, E. E., Gu, Q., and Wu, H.-M. (1993). Characterization of cDNAs for stylar transmitting tissue-specific proline-rich proteins in tobacco. Plant J. 3, 151–160. doi: 10.1046/j.1365-313X.1993.t01-7-00999.x
Chiang, H. H., and Dandekar, A. M. (1995). Regulation of proline accumulation in Arabidopsis thaliana (L.) Heynh during development and in response to desiccation. Plant Cell Environ. 18, 1280–1290. doi: 10.1111/j.1365-3040.1995.tb00187.x
Clarke, A., Gleeson, P., Harrison, S., and Knox, R. B. (1979). Pollen-stigma interactions: identification and characterization of surface components with recognition potential (cell surface receptors/plant glycoproteins/adhesion/concanavalin A/tridacnin). Proc. Natl. Acad. Sci. U.S.A. 76, 3358–3362. doi: 10.1073/pnas.76.7.3358
Clements, R. L., and Leland, H. V. (1962). An ion-exchange study of the free amino acids in the juices of six varieties of citrus. J. Food Sci. 27, 20–25. doi: 10.1111/j.1365-2621.1962.tb00051.x
Coleman, C. E., Lopes, M. A., Gillikin, J. W., Boston, R. S., and Larkins, B. A. (1995). A defective signal peptide in the maize high lysine mutant floury 2. Proc. Natl. Acad. Sci. U.S.A. 92, 6828–6831. doi: 10.1073/pnas.92.15.6828
Colmenero-Flores, J. M., Campos, F., Garciarrubio, A., and Covarrubias, A. A. (1997). Characterization of Phaseolus vulgaris cDNA clones responsive to water deficit: identification of a novel late embryogenesis abundant-like protein. Plant Mol. Biol. 35, 393–405. doi: 10.1023/A:1005802505731
Cooper, J. B., Heuser, J. E., and Varner, J. E. (1994). 3,4-dehydroproline inhibits cell wall assembly and cell division in tobacco protoplasts. Plant Physiol. 104, 747–752. doi: 10.1104/pp.104.2.747
Couturier, J., Fay, E. D., Fitz, M., Wipf, D., Blaudez, D., and Chalot, M. (2010). PtAAP11, a high affinity amino acid transporter specifically expressed in differentiating xylem cells of poplar. J. Exp. Bot. 61, 1671–1682. doi: 10.1093/jxb/erq036
Covarrubias, A. A., Ayala, J. W., Reyes, J. L., Hernandez, M., and Garciarrubio, A. (1995). Cell-wall proteins induced by water deficit in bean (Phaseolus vulgaris L.) seedlings. Plant Physiol. 107, 1119–1128. doi: 10.1104/pp.107.4.1119
Creelman, R. A., and Mullet, J. E. (1991). Water deficit modulates gene expression in growing zones of soybean seedlings. Analysis of differentially expressed cDNAs, a new β-tubulin gene, and expression of genes encoding cell wall proteins. Plant Mol. Biol. 17, 591–608. doi: 10.1007/BF00037046
Delauney, A. J., Hu, C. A. A., Kishor, P. B. K., and Verma, D. P. S. (1993). Cloning of ornithine δ-aminotransferase cDNA from Vigna aconitifolia by trans-complementation in Escherichia coli and regulation of proline biosynthesis. J. Biol. Chem. 268, 18673–18678.
Delauney, A. J., and Verma, D. P. S. (1990). A soybean Δ1-pyrroline-5-carboxylate reductase gene was isolated by functional complementation in Escherichia coli and is found to be osmoregulated. Mol. Gen. Genet. 221, 299–305. doi: 10.1007/BF00259392
Delauney, A. J., and Verma, D. P. S. (1993). Proline biosynthesis and osmoregulation in plants. Plant J. 4, 215–223. doi: 10.1046/j.1365-313X.1993.04020215.x
Deuschle, K., Funck, D., Forlani, G., Stransky, H., Biehl, A., Leister, D., et al. (2004). The role of Δ1-pyrroline-5-carboxylate dehydrogenase in proline degradation. Plant Cell 16, 3413–3425. doi: 10.1105/tpc.104.023622
Deuschle, K., Funck, D., Hellman, H., Daschner, K., Binder, S., and Frommer, W. B. (2001). A nuclear gene encoding mitochondrial Δ1-pyrroline-5-carboxylate dehydrrogenase and its potential role in protection from proline toxicity. Plant J. 27, 345–356. doi: 10.1046/j.1365-313X.2001.01101.x
Deutch, C. E., and Winicov, I. (1995). Post-transcriptional regulation of a salt-inducible alfalfa gene encoding a putative chimeric proline-rich cell wall protein. Plant Mol. Biol. 27, 411–418. doi: 10.1007/BF00020194
Dick-Perez, M., Zhang, Y., Hayes, J., Salazar, A., Zabotina, O. A., and Hong, M. (2011). Structure and interactions of plant cell-wall polysaccharides by two and three-dimensional magic-angle-spinning solid-state NMR. Biochemistry 50, 989–1000. doi: 10.1021/bi101795q
Diet, A., Link, B., Seifert, G. J., Schellenberg, B., Wagner, U., Pauly, M., et al. (2006). The Arabidopsis root hair cell wall formation mutant lrx1 is suppressed by mutations in the RHM1 gene encoding a UDP-L-rhamnose synthase. Plant Cell 18, 1630–1641. doi: 10.1105/tpc.105.038653
Ding, L., and Zhu, J.-K. (1997). A role for arabinogalactan-proteins in root epidermal cell expansion. Planta 203, 289–294. doi: 10.1007/s004250050194
Doblin, M. S., Pettolino, F., and Bacic, A. (2010). Plant cell walls: the skeleton of the plant world. Funct. Plant Biol. 37, 357–381. doi: 10.1071/FP09279
Dvorakova, L., Cvrckova, F., and Fischer, L. (2007). Analysis of the hybrid proline-rich protein families from seven plant species suggests rapid diversification of their sequences and expression patterns. BMC Genomics 8:412. doi: 10.1186/1471-2164-8-412
Dvorakova, L., Srba, M., Opatrny, Z., and Fischer, L. (2012). Hybrid proline-rich proteins: novel players in plant cell elongation? Ann. Bot. 109, 453–462. doi: 10.1093/aob/mcr278
Egertsdotter, U., and von Arnold, S. (1995). Importance of arabinogalactan proteins for the development of somatic embryos of Norway spruce (Picea abies). Physiol. Plant. 93, 334–345. doi: 10.1111/j.1399-3054.1995.tb02237.x
Ellis, M., Egelund, J., Schultz, C. J., and Bacic, A. (2010). Arabinogalactanproteins: key regulators at the cell surface? Plant Physiol. 153, 403–419. doi: 10.1104/pp.110.156000
Fincher, G. B., Stone, B. A., and Clarke, A. E. (1983). Arabinogalactan-proteins: structure, biosynthesis, and function. Ann. Rev. Plant Physiol. 34, 47–70. doi: 10.1146/annurev.pp.34.060183.000403
Fischer, W. N., Andre, B., Rentsch, D., Krolkiewicz, S., Tegedar, M., Breitkreuz, K., et al. (1998). Amino acid transport in plants. Trends Plant Sci. 3, 188–195. doi: 10.1016/S1360-1385(98)01231-X
Fischer, W. N., Kwart, M., Hummel, S., and Frommer, W. B. (1995). Substrate specificity and expression profile of amino acid transporters (AAPs) in Arabidopsis. J. Biol. Chem. 270, 16315–16320. doi: 10.1074/jbc.270.27.16315
Flasinski, S., and Rogozinska, J. (1985). Effect of water deficit on proline accumulation, protein and chlorophyll content during flowering and seed formation in winter rape (Brassica napus L. var. oleifera). Acta Agrobot. 38, 11–21.
Foster, J., Lee, Y. H., and Tegeder, M. (2008). Distinct expression of members of the LHT amino acid transporter family in flowers indicates specific roles in plant reproduction. Sexual Plant Rep. 21, 143–152. doi: 10.1007/s00497-008-0074-z
Fowler, T. J., Bernhardt, C., and Tierney, M. L. (1999). Characterization and expression of four proline-rich cell wall protein genes in arabidopsis encoding two distinct subsets of multiple domain proteins. Plant Physiol. 121, 1081–1091. doi: 10.1104/pp.121.4.1081
Frommer, W. B., Hummel, S., and Riesmeier, J. W. (1993). Expression cloning in yeast of a cDNA encoding a broad specificity amino acid permease from Arabidopsis thaliana. Proc. Natl. Acad. Sci. U.S.A. 90, 5944–5948. doi: 10.1073/pnas.90.13.5944
Frommer, W. B., Hummel, S., Unseld, M., and Ninnemann, O. (1995). Seed and vascular expression of a high-affinity transporter for cationic amino acids in Arabidopsis. Proc. Natl. Acad. Sci. U.S.A. 92, 12036–12040. doi: 10.1073/pnas.92.26.12036
Frueauf, J. B., Dolata, M., Leykam, J. F., Lloyd, E., Gonzales, M., Vandenbosch, K., et al. (2000). Peptides isolated from cell walls of Medicago truncatula nodules and uninfected root. Phytochem. 55, 429–438. doi: 10.1016/S0031-9422(00)00336-8
Fujita, T., Maggio, A., Garcia-Rios, M., Bressan, R. A., and Csonka, L. N. (1998). Comparative analysis of the regulation of expression and structures of two evolutionarily divergent genes for Δ1-pyrroline-5-carboxylate synthetase. Plant Physiol. 118, 661–674. doi: 10.1104/pp.118.2.661
Fujiwara, T., Mitsuya, S., Miyake, H., Hattori, T., and Takabe, T. (2010). Characterization of novel glycinebetaine/proline transporter gene expressed in the mestome sheath and lateral root cap cells in barley. Planta 232, 133–143. doi: 10.1007/s00425-010-1155-4
Funck, D., Eckard, S., and Muller, G. (2010). Non-redundant functions of two proline dehydrogenase isoforms in Arabidopsis. BMC Plant Biol. 10:70. doi: 10.1186/1471-2229-10-70
Funck, D., Stadelhofer, B., and Koch, W. (2008). Ornithine-δ-aminotransferase is essential for arginine catabolism but not for proline biosynthesis. BMC Plant Biol. 8:40 doi: 10.1186/1471-2229-8-40
Funck, D., Winter, G., Baumgarten, L., and Forlani, G. (2012). Requirement of proline synthesis during Arabidopsis reproductive development. BMC Plant Biol. 12:191. doi: 10.1186/1471-2229-12-191
Gao, M., and Showalter, A. M. (2000). Immunolocalization of LeAGP-1, a modular arabinogalactan-protein, reveals its developmentally regulated expression in tomato. Planta 210, 865–874. doi: 10.1007/s004250050691
Gavazzi, G., Nava-Racchi, M., and Tonelli, C. (1975). A mutation causing proline requirement in Zea mays. Theor. Appl. Genet. 46, 339–345. doi: 10.1007/BF00281675
Ghanti, K. K. S., Sujata, K. G., Kumar, B. M. V., Karba, N. N., Reddy, K. J., Rao, M. S., et al. (2011). Heterologous expression of P5CS gene in chickpea enhances salt tolerance without affecting yield. Biol. Plant. 55, 634–640. doi: 10.1007/s10535-011-0161-0
Gibbon, B. C., Zonia, L. E., Kovar, D. R., Hussey, P. J., and Staiger, C. J. (1998). Pollen profiling function depends on interaction with proline-rich motifs. Plant Cell 10, 981–993. doi: 10.1105/tpc.10.6.981
Gothandam, K. M., Nalini, E., Karthikeyan, S., and Shin, J. S. (2010). OsPRP3, a flower specific proline-rich protein of rice, determines extracellular matrix structure of floral organs and its overexpression confers cold-tolerance. Plant Mol. Biol. 72, 125–135. doi: 10.1007/s11103-009-9557-z
Grallath, S., Weimar, T., Meyer, A., Gumy, C., Suter-Grotemeyer, M., Neuhaus, J., et al. (2005). The AtProT family. Compatible solute transporters with similar substrate specificity but differential expression patterns. Plant Physiol. 137, 117–126. doi: 10.1104/pp.104.055079
Griffiths, J. S., Tsai, A. Y., Xue, H., Voiniciuc, C., Šola, K., Seifert, G. J., et al. (2014). SALT-OVERLY SENSITIVE5 mediates Arabidopsis seed coat mucilage adherence and organization through pectins. Plant Physiol. 165, 991–1004. doi: 10.1104/pp.114.239400
Haffani, Y. Z., Silva-Gagliardi, N. F., Sewter, S. K., Grace Aldea, M., Zhao, Z., Nakhamchik, A., et al. (2006). Altered expression of PERK receptor kinases in Arabidopsis leads to changes in growth and floral organ formation. Plant Signal. Behav. 1, 251–260. doi: 10.4161/psb.1.5.3324
Hall, Q., and Cannon, M. (2002). The cell wall hydroxyproline-rich glycoprotein RSH is essential for normal embryo development in Arabidopsis. Plant Cell 14, 1161–1172. doi: 10.1105/tpc.010477
Hancock, C. N., Kent, L., and McClure, B. A. (2005). The stylar 120 kDa glycoprotein is required for S-specific pollen rejection in Nicotiana. Plant J. 43, 716–723. doi: 10.1111/j.1365-313X.2005.02490.x
Hare, P. D., and Cress, W. (1997). Metabolic implications of stress induced proline accumulation in plants. Plant Growth Reg. 21, 79–102. doi: 10.1023/A:1005703923347
Hare, P. D., Cress, W. A., and Van Staden, J. (1999). Proline synthesis and degradation: a model system for elucidating stress-related signal transduction. J. Exp. Bot. 50, 413–434. doi: 10.1093/jxb/50.333.413
Hare, P. D., Cress, W. A., and Van Staden, J. (2003). A regulatory role for proline metabolism in stimulating Arabidopsis thaliana seed germination. Plant Growth Reg. 39, 41–50. doi: 10.1023/A:1021835902351
Hayashi, F., Ichino, T., Osanai, M., and Wada, K. (2000). Oscillation and regulation of proline content by P5CS and ProDH gene expressions in the light/dark cycles in Arabidopsis thaliana. Plant Cell Physiol. 41, 1096–1101. doi: 10.1093/pcp/pcd036
Held, M. A., Tan, L., Kamyab, A., Hare, M., Shpak, E., and Kieliszewksi, M. J. (2004). Di-isodityrosine is the intermolecular cross-link of isodityrosine-rich extensin analogs cross-linked in vitro. J. Biol. Chem. 279, 55474–55482. doi: 10.1074/jbc.M408396200
Henrissat, B., Coutinho, P. M., and Davies, G. J. (2001). A census of carbohydrate-active enzymes in the genome of Arabidopsis thaliana. Plant Mol. Biol. 47, 55–72. doi: 10.1023/A:1010667012056
Hibino, T., Meng, Y. L., Kawamitsu, Y., Uehara, N., Matsuda, N., Tanaka, Y., et al. (2001). Molecular cloning and functional characterization of two kinds of betaine-aldehyde dehydrogenase in betaine-accumulating mangrove Avicennia marina (Forsk.) Vierh. Plant Mol. Biol. 45, 353–363. doi: 10.1023/A:1006497113323
Hijazi, M., Roujol, D., Nguyen-Kim, H., Del Rocio Cisneros Castillo, L., Saland, E., Jamet, E., et al. (2014). Arabinogalactan protein 31 (AGP31), a putative network-forming protein in Arabidopsis thaliana cell walls? Ann. Bot. 114, 1087–1097. doi: 10.1093/aob/mcu038
Hirner, B., Fischer, W. N., Rentsch, D., Kwart, M., and Fommer, W. B. (1998). Developmental control of H+/amino acid permease gene expression during seed development of Arabidopsis. Plant J. 14, 535–544. doi: 10.1046/j.1365-313X.1998.00151.x
Holding, D. R., Meeley, R. B., Hazebroek, J., Selinger, D., Gruis, F., Jung, R., et al. (2010). Identification and characterization of the maize arogenate dehydrogenase gene family. J. Exp. Bot. 61, 3663–3673. doi: 10.1093/jxb/erq179
Hong, J. C., Nagao, R. T., and Key, J. L. (1987). Characterization and sequence analysis of a developmentally regulated putative cell wall protein gene isolated from soybean. J. Biol. Chem. 262, 8367–8376.
Hong, J. C., Nagao, R. T., and Key, J. L. (1989). Developmentally regulated expression of soybean proline-rich cell wall protein genes. Plant Cell 1, 937–943. doi: 10.1105/tpc.1.9.937
Hong, J. C., Nagao, R. T., and Key, J. L. (1990). Characterization of a proline-rich cell wall protein gene family of soybean. A comparative analysis. J. Biol. Chem. 265, 2470–2475.
Hu, C. A. A., Delauney, A. J., and Verma, D. P. S. (1992). A bifunctional enzyme (Δ1-pyrroline-5-carboxylate synthetase) catalyzes the first two steps in proline biosynthesis in plants. Proc. Natl. Acad. Sci. U.S.A. 89, 9354–9358. doi: 10.1073/pnas.89.19.9354
Hur, J., Jung, K. H., Lee, C. H., and An, G. (2004). Stress-inducible OsP5CS2 gene is essential for salt and cold tolerance in rice. Plant Sci. 167, 417–426. doi: 10.1016/j.plantsci.2004.04.009
Igarashi, Y., Yoshiba, Y., Takeshita, T., Nomura, S., Otomo, J., Yamaguchi-Shinozaki, K., et al. (2000). Molecular cloning and characterization of a cDNA encoding proline transporter in rice. Plant Cell Physiol. 41, 750–756. doi: 10.1093/pcp/41.6.750
Ito, M., Kodama, H., Komamine, A., and Watanabe, A. (1998). Expression of extensin genes is dependent on the stage of the cell cycle and cell proliferation in suspension-cultured Catharanthus roseus cells. Plant Mol. Biol. 36, 343–351. doi: 10.1023/A:1005913818129
Jackson, P. A., Galinha, C. I., Pereira, C. S., Fortunato, A., Soares, N. C., Amancio, S. B., et al. (2001). Rapid deposition of extensin during the elicitation of grapevine callus cultures is specifically catalyzed by a 40-kilodalton peroxidase. Plant Physiol. 127, 1065–1076. doi: 10.1104/pp.010192
Jose-Estanyol, M., and Puigdomenech, P. (2000). Plant cell wall glycoproteins and their genes. Plant Physiol. Biochem. 38, 97–108. doi: 10.1016/S0981-9428(00)00165-0
Jose-Estanyol, M., Ruiz-Avila, L., and Puigdomenench, P. (1992). A maize embryo-specific gene encodes a proline-rich and hydrophobic protein. Plant Cell 4, 413–423. doi: 10.1105/tpc.4.4.413
Kaplan, D. R., and Hagemann, W. (1991). The relationship of cell and organism in vascular plants. Bioscience 41, 693–703. doi: 10.2307/1311764
Kieliszewski, M. J., and Lamport, D. T. A. (1994). Extensin: repetitive motifs, functional sites, post-translation codes and phylogeny. Plant J. 5, 157–172. doi: 10.1046/j.1365-313X.1994.05020157.x
Kieliszewski, M. J., Leykam, J. F., and Lamport, D. T. (1989). Trypsin cleaves lysylproline in a hydroxyproline-rich glycoprotein from Zea mays. Pept. Res. 2, 246–248.
Kieliszewski, M. J., and Shpak, E. (2001). Synthetic genes for the elucidation of glycosylation codes for arabinogalactan-proteins and other hydroxyproline-rich glycoproteins. Cell Mol. Life Sci. 58, 1386–1398. doi: 10.1007/PL00000783
Kim, C. S., Gibbon, B. C., Gillikin, J. W., Larkins, B. A., Boston, R. S., and Jung, R. (2006). The maize mucronate mutation is a deletion in the 16-kDa gamma-zein gene that induces the unfolded protein response. Plant J. 48, 440–451. doi: 10.1111/j.1365-313X.2006.02884.x
Kishor, P. B. K., Hong, Z., Miao, G. H., Hu, C. A. A., and Verma, D. P. S. (1995). Overexpression of Δ1-pyrroline-5-carboxylate synthetase increases proline production and confers osmotolerance in transgenic plants. Plant Physiol. 108, 1387–1394.
Kishor, P. B. K., Sangam, S., Amrutha, R. N., Laxmi, P. S., Naidu, K. R., Rao, K. R. S. S., et al. (2005). Regulation of proline biosynthesis, degradation, uptake and transport in higher plants: Its implications in plant growth and abiotic stress tolerance. Curr. Sci. 88, 424–438.
Kishor, P. B. K., and Sreenivasulu, N. (2014). Is proline accumulation per se correlated with stress tolerance or is proline homoeostasis a more critical issue? Plant Cell Environ. 37, 300–311. doi: 10.1111/pce.12157
Kiyosue, T., Yoshiba, Y., Yamaguchi-Shinozaki, K., and Shinozaki, K. (1996). A nuclear gene encoding mitochondrial proline dehydogenase, an enzyme involved in proline metabolism, is upregulated by proline but downregulated by dehydration in Arabidopsis. Plant Cell 8, 1323–1335. doi: 10.1105/tpc.8.8.1323
Knoch, E., Dilokpimol, A., and Geshi, N. (2014). Arabinogalactan proteins: focus on carbohydrate active enzymes. Front. Plant Sci. 5:198. doi: 10.3389/fpls.2014.00198
Kreuger, M., and van Holst, G. (1993). Arabinogalactan proteins are essential in somatic embryogenesis of Daucus carota L. Planta 189, 243–248. doi: 10.1007/BF00195083
Kwart, M., Hirner, B., Hummel, S., and Frommer, W. B. (1993). Differential expression of two related amino-acid transporters with differing substrate specificity in Arabidopsis thaliana. Plant J. 4, 993–1002. doi: 10.1046/j.1365-313X.1993.04060993.x
Lamport, D. T. A. (1967). Hydroxyproline-O-glycosidic linkage of the plant cell wall glycoprotein extensin. Nature 216, 1322–1324. doi: 10.1038/2161322a0
Lamport, D. T. A. (1977). “Structure, biosynthesis and signifi cance of cell wall glycoproteins,” in Recent Advances in Phytochemistry, 11th Edn, eds F. A. Loewus and V. C. Runeckles (New York: Plenum Press), 79–115.
Lamport, D. T. A., Kieliszewski, M. J., Chen, Y., and Cannon, M. C. (2011). Role of the extensin superfamily in primary cell wall architecture. Plant Physiol. 156, 11–19. doi: 10.1104/pp.110.169011
Lamport, D. T. A., Kieliszewski, M. J., and Showalter, A. M. (2006). Salt stress upregulates periplasmic arabinogalactan proteins: using salt stress to analyse AGP function. New Phytol. 169, 479–492. doi: 10.1111/j.1469-8137.2005.01591.x
Lamport, D. T. A., and Várnai, P. (2013). Periplasmic arabinogalactan glycoproteins act as a calcium capacitor that regulates plant growth and development. New Phytol. 197, 58–64. doi: 10.1111/nph.12005
Lee, J. A., and Pallas, D. C. (2007). Leucine carboxyl methyltransferase-1 is necessary for normal progression through mitosis in mammalian cells. J. Biol. Chem. 282, 30974–30984. doi: 10.1074/jbc.M704861200
Lee, Y. H., and Tegeder, M. (2004). Selective expression of a novel high-affinity transport system for acidic and neutral amino acids in the tapetum cells of Arabidopsis flowers. Plant J. 40, 60–74. doi: 10.1111/j.1365-313X.2004.02186.x
Lehmann, S., Funck, D., Szabados, L., and Rentsch, D. (2010). Proline metabolism and transport in plant development. Amino Acids 39, 949–962. doi: 10.1007/s00726-010-0525-3
Lehmann, S., Gumy, C., Blatter, E., Boeffel, S., Fricke, W., and Rentsch, D. (2011). In planta function of compatible solute transporters of the AtProT family. J. Exp. Bot. 62, 787–796. doi: 10.1093/jxb/erq320
LeRudulier, D., Strom, A. R., Dandekar, A. M., Smith, L. T., and Valentaine, R. C. (1984). Molecular biology of osmoregulation. Science 224, 1064–1068. doi: 10.1126/science.224.4653.1064
Lord, E. M., and Sanders, L. C. (1992). Roles for the extracellular matrix in plant development and pollination: a special case of cell movement in plants. Dev. Biol. 153, 16–28. doi: 10.1016/0012-1606(92)90088-X
Ma, Y., and Nelson, O. E. (1975). Amino acid composition and storage proteins in two new high-lysine mutants of maize. Cereal Chem. 52, 412–419.
MacArthur, M. W., and Thornton, J. M. (1991). Influence of proline residues on protein conformation. J. Mol. Biol. 218, 397–412. doi: 10.1016/0022-2836(91)90721-H
Mattioli, R., Costantino, P., and Trovato, M. (2009a). Proline accumulation in plants-Not only stress. Plant Sig. Behav. 4, 1016-1018. doi: 10.4161/psb.4.11.9797
Mattioli, R., Falascb, G., Sabatini, S., Altamura, M. M., Costantino, P., and Trovato, M. (2009b). The proline biosynthetic genes P5CS1 and P5CS2 play overlapping roles in Arabidopsis flower transition but not in embryo development. Physiol. Plant. 137, 72–85. doi: 10.1111/j.1399-3054.2009.01261.x
Mattioli, R., Marchese, D., D’Angeli, S., Altamura, M. M., Costantino, P., and Trovato, M. (2008). Modulation of intracellular proline levels affects flowering time and inflorescence architecture in Arabidopsis. Plant Mol. Biol. 66, 277–288. doi: 10.1007/s11103-007-9269-1
Mauro, M. L, Trovato, M., De Paolis, A., Gallelli, A., Costantino, P., and Altamura, M. M. (1996). The plant oncogene rolD stimulates flowering in transgenic tobacco plants. Dev. Biol. 180, 693–700. doi: 10.1006/dbio.1996.0338
Mayer, U., and Jurgens, G. (2004). Cytokinesis: lines of division taking shape. Curr. Opin. Plant Biol. 7, 599–604. doi: 10.1016/j.pbi.2004.07.008
McCann, M. C., Bush, M., Milioni, D., Sado, P., Stacey, N. J., Catchpole, G., et al. (2001). Approaches to understanding the functional architecture of the plant cell wall. Phytochemistry 57, 811–821. doi: 10.1016/S0031-9422(01)00144-3
Meinke, D., Muralla, R., Sweeney, C., and Dickerman, A. (2008). Identifying essential genes in Arabidopsis thaliana. Trends Plant Sci. 13, 483–491. doi: 10.1016/j.tplants.2008.06.003
Menke, U., Renault, N., and Mueller-Roeber, B. (2000). StGCPRP, a potato gene strongly expressed in stomatal guard cells, defines a novel type of repetitive proline-rich proteins. Plant Physiol. 122, 677–686. doi: 10.1104/pp.122.3.677
Merkouropoulos, G., and Shirsat, A. H. (2003). The unusual Arabidopsis extensin gene Atext1 is expressed throughout plant development and is induced by a variety of biotic and abiotic stresses. Planta 217, 356–366. doi: 10.1007/s00425-003-1002-y
Motose, H., Sugiyama, M., and Fukuda, H. (2001). An arabinogalactan protein(s) is a key component of a fraction that mediates local intercellular communication involved in tracheary element differentiation of Zinnia mesophyll cells. Plant Cell Physiol. 42, 129–137. doi: 10.1093/pcp/pce014
Munoz, F. J., Dopico, B., and Labrador, E. (1998). A cDNA encoding a proline-rich protein from Cicer arietinum. Changes in expression during development and abiotic stress. Physiol. Plant. 102, 582–590. doi: 10.1034/j.1399-3054.1998.1020413.x
Nanjo, T., Kobayashi, M., Yoshiba, Y., Sanada, Y., Wada, K., Tsukaya, H., et al. (1999). Biological functions of proline in morphogenesis and osmotolerance revealed in antisense transgenic Arabidopsis thaliana. Plant J. 18, 185–193. doi: 10.1046/j.1365-313X.1999.00438.x
Nguema-Ona, E., Vicré-Gibouin, M., Cannesan, M. A., and Driouich, A. (2013). Arabinogalactan proteins in root-microbe interactions. Trends Plant Sci. 18, 440–449. doi: 10.1016/j.tplants.2013.03.006
Nothnagel, E. A. (1997). Proteoglycans and related components in plant cells. Inter. Rev. Cytol. 174, 195–291. doi: 10.1016/S0074-7696(08)62118-X
Nunez, A., Fishman, M. L., Fortis, L. L., Cooke, P. H., and Hotchkiss, A. T. J. (2009). Identification of extensin protein associated with sugar beet pectin. J. Agric. Food Chem. 57, 10951–10958. doi: 10.1021/jf902162t
Ober, E. S., and Sharp, R. E. (1994). Proline accumulation in maize (Zea mays L.) primary roots at low water potentials. (I Requirement for increased levels of abscisic acid). Plant Physiol. 105, 981–987.
Ogawa-Ohnishi, M., Matsushita, W., and Matsubayashi, Y. (2013). Identification of three hydroxyproline O-arabinosyltransferases in Arabidopsis thaliana. Nat. Chem. Biol. 9, 726–730. doi: 10.1038/nchembio.1351
Okumoto, S., Koch, W., Tegeder, M., Fischer, W. N., Biehl, A., Leister, D., et al. (2004). Root phloem-specific expression of the plasma membrane amino acid proton co-transporter AAP3. J. Exp. Bot. 55, 2155–2168. doi: 10.1093/jxb/erh233
Okumoto, S., Schmidt, R., Tegeder, M., Fischer, W. N., Rentsch, D., Frommer, W. B., et al. (2002). High affinity amino acid transporters specifically expressed in xylem parenchyma and developing seeds of Arabidopsis. J. Biol. Chem. 277, 45338–45346. doi: 10.1074/jbc.M207730200
Park, S., Szumlanski, A. L., Gu, F., Guo, F., and Nielsen, E. (2011). A role for CSLD3 during cell-wall synthesis in apical plasma membranes of tip-growing root-hair cells. Nat. Cell Biol. 13, 973–980. doi: 10.1038/ncb2294
Pennell, R. I., Janniche, L., Kjellbom, P., Scofield, G. N., Peart, J. M., and Roberts, K. (1991). Developmental regulation of a plasma membrane arabinogalactan protein epitope in oilseed rape flowers. Plant Cell 3, 1317–1326. doi: 10.1105/tpc.3.12.1317
Pennell, R. I., and Roberts, K. (1990). Sexual development in the pea is presaged by altered expression of arabinogalactan protein. Nature 344, 547–549. doi: 10.1038/344547a0
Pope, D. G. (1977). Relationships between hydroxyproline-containing proteins secreted into the cell wall and medium by suspension- cultured Acer pseudoplatanus cells. Plant Physiol. 59, 894–900. doi: 10.1104/pp.59.5.894
Price, N. J., Pinheiro, C., Soares, C. M., Ashford, D. A., Ricardo, C. P., and Jackson, P. A. (2003). A biochemical and molecular characterization of LEP1, an extensin peroxidase from lupin. J. Biol. Chem. 278, 41389–41399. doi: 10.1074/jbc.M304519200
Racchi, M. L., Gavazzi, G., Monti, D., and Manitto, P. (1978). An analysis of the nutritional requirements of thepromutants in Zea mays. Plant Sci. Lett. 13, 357–364. doi: 10.1016/0304-4211(78)90213-4
Raymond, M. J., and Smirnoff, N. (2002). Proline metabolism and transport in maize seedlings at low water potential. Ann. Bot. 89, 813–823. doi: 10.1093/aob/mcf082
Reddy, P. S., Jogeswar, G., Rasineni, G. K., Maheswari, M., Reddy, A. R., Varshney, R. K., et al. (2015). Proline over-accumulation alleviates salt stress and protects photosynthetic and antioxidant enzyme activities in transgenic sorghum [Sorghum bicolor (L.) Moench]. Plant Physiol. Biochem. 94, 104–113. doi: 10.1016/j.plaphy.2015.05.014
Rentsch, D., Hirner, B., Schmelzer, E., and Frommer, W. B. (1996). Salt-stress induced proline transporters and salt-repressed broad specificity amino acid permeases identified by suppression of a yeast amino acid permease-targeting mutant. Plant Cell 8, 1437–1446. doi: 10.1105/tpc.8.8.1437
Rentsch, D., Schmidt, S., and Tegeder, M. (2007). Transporters for uptake and allocation of organic nitrogen compounds in plants. FEBS Lett. 581, 2281–2289. doi: 10.1016/j.febslet.2007.04.013
Rhee, S. Y., Osborne, E., Poindexter, P. D., and Somerville, C. R. (2003). Microspore separation in the quartet 3 mutants of Arabidopsis is impaired by a defect in a developmentally regulated polygalacturonase required for pollen mother cell wall degradation. Plant Physiol. 133, 1170–1180. doi: 10.1104/pp.103.028266
Rhodes, D., Handa, S., and Bressan, R. A. (1986). Metabolic changes associated with adaptation of plant cells to water stress. Plant Physiol. 82, 890–903. doi: 10.1104/pp.82.4.890
Ringli, C. (2010). The hydroxyproline-rich glycoprotein domain of the Arabidopsis LRX1 requires Tyr for function but not for insolubilization in the cell wall. Plant J. 63, 662–669. doi: 10.1111/j.1365-313X.2010.04270.x
Roberts, K. (1989). The plant extracellular matrix. Cur. Opin. Cell Biol. 1, 1020–1027. doi: 10.1016/0955-0674(89)90074-4
Roberts, K., and Shirsat, A. H. (2006). Increased extension levels in Arabidopsis affect inflorescence stem thickening and height. J. Exp. Bot. 57, 537–545. doi: 10.1093/jxb/erj036
Romanczyk, L. J. Jr., McClelland, C. A., Post, L. S., and Aitken, W. M. (1995). Formation of 2-acetyl-1-pyrroline by several Bacillus cereus strains isolated from cocoa fermentation boxes. J. Agric. Food Chem. 43, 469–475. doi: 10.1021/jf00050a040
Samach, A., Onouchi, H., Gold, S. E., Ditta, G. S., Schwarz-Sommer, Z., Yanofsky, M. F., et al. (2000). Distinct roles of CONSTANS target genes in reproductive development of Arabidopsis. Science 288, 1613–1616. doi: 10.1126/science.288.5471.1613
Sauer, N., and Tanner, W. (1985). Selection and characterization of Chlorella mutants deficient in amino acid transport. Plant Physiol. 79, 760–764. doi: 10.1104/pp.79.3.760
Savoure, A., Jaoua, S., Hua, X., Ardiles, W., Montagu, M. V., and Verbruggen, N. (1995). Isolation, characterization, and chromosomal location of a gene encoding the Δ1-pyrroline-5-carboxylate synthetase in Arabidopsis thaliana. FEBS Lett. 372, 13–19. doi: 10.1016/0014-5793(95)00935-3
Schindler, T., Bergfeld, R., and Schopfer, P. (1995). Arabinogalactan proteins in maize coleoptiles: developmental relationship to cell death during xylem differentiation but not to extension growth. Plant J. 5, 157–172. doi: 10.1046/j.1365-313X.1995.07010025.x
Schmidt, R. J., Burr, F. A., and Burr, B. (1987). Transposon tagging and molecular analysis of the maize regulatory locus opaque-2. Science 238, 960–963. doi: 10.1126/science.2823388
Schmidt, R., Stransky, H., and Koch, W. (2007). The amino acid permease AAP8 is important for early seed development in Arabidopsis thaliana. Planta 226, 805–813. doi: 10.1007/s00425-007-0527-x
Schultz, C. J., Ferguson, K. L., Lahnstein, J., and Bacic, A. (2004). Post-translational modifications of arabinogalactan-peptides of Arabidopsis thaliana: endoplasmic reticulum and glycosylphosphatidylinositol-anchor signal cleavage sites and hydroxylation of proline. J. Biol. Chem. 279, 45503–45511. doi: 10.1074/jbc.M407594200
Schultz, C., Gilson, P., Oxley, D., Youl, J., and Bacic, A. (1998). GPI-anchors on arabinogalactan-proteins: implications for signalling in plants. Trends Plant Sci. 3, 426–431. doi: 10.1016/S1360-1385(98)01328-4
Schwacke, R., Grallath, S., Breitkreuz, K. E., Stransky, H., Frommer, W. B., and Rentsch, D. (1999). LeProT1, a transporter for proline, glycine betaine and γ-aminobutyric acid in tomato pollen. Plant Cell 11, 377–391. doi: 10.1105/tpc.11.3.377
Serpe, M. D., and Nothnagel, E. A. (1999). Arabinogalactan-proteins in the multiple domains of the plant cell surface. Adv. Bot. Res. 30, 207–289. doi: 10.1016/S0065-2296(08)60229-3
Sharma, S., Shinde, S., and Verslues, P. E. (2013). Functional characterization of an ornithine cyclodeaminase-like protein of Arabidopsis thaliana. BMC Plant Biol. 13:182. doi: 10.1186/1471-2229-13-182
Sharma, S., and Verslues, P. E. (2010). Mechanisms independent of abscisic acid (ABA) or proline feedback have a predominant role in transcriptional regulation of proline metabolism during low water potential and stress recovery. Plant Cell Environ. 33, 1838–1851. doi: 10.1111/j.1365-3040.2010.02188.x
Shen, Y. G., Zhang, W. K., Yan, D. Q., Du, B. X., Zhang, J. S., and Chen, S. Y. (2002). Overexpression of proline transporter gene isolated from halophyte confers salt tolerance in Arabidopsis. Acta Bot. Sin. 44, 956–962.
Shetty, K. (2004). Role of proline-linked pentose phosphate pathway in biosynthesis of plant phenolics for functional food and environmental applications: a review. Process Biochem. 39, 789–803. doi: 10.1016/S0032-9592(03)00088-8
Shirsat, A. H., Wieczorek, D., and Kozbial, P. (1996). A gene for Brassica napus extensin is differentially expressed on wounding. Plant Mol. Biol. 30, 1291–1300. doi: 10.1007/BF00019559
Showalter, A. M. (1993). Structure and function of plant cell wall proteins. Plant Cell 5, 9–23. doi: 10.1105/tpc.5.1.9
Showalter, A. M. (2001). Arabinogalactan-proteins: structure, expression, and function. Cell. Mol. Life Sci. 58, 1399–1417. doi: 10.1007/PL00000784
Showalter, A. M., Keppler, B., Lichtenberg, J., Gu, D., and Welch, L. R. (2010). A bioinformatics approach to the identification, classification, and analysis of hydroxyproline-rich glycoproteins. Plant Physiol. 153, 485–513. doi: 10.1104/pp.110.156554
Showalter, A. M., and Varner, J. E. (1989). “Plant hydroxyproline-rich glycoproteins,” in The Biochemistry of Plants, ed. M. Abraham (New York: Academic Press), 485–520.
Shpak, E., Barbar, E., Leykam, J. F., and Kieliszewski, M. J. (2001). Contiguous hydroxyproline residues direct hydroxyproline arabinosylation in Nicotiana tabacum. J. Biol. Chem. 276, 11272–11278. doi: 10.1074/jbc.M011323200
Somerville, C., Bauer, S., Brininstool, G., Facette, M., Hamann, T., Milne, J., et al. (2004). Toward a systems approach to understanding plant cell walls. Science 306, 2206–2211. doi: 10.1126/science.1102765
Spoljarevic, M., Agic, D., Lisjak, M., Gumze, A., Wilson, I. D., Hancock, J. T., et al. (2011). The relationship of proline content and metabolism on the productivity of maize plants. Plant Sign. Behav. 6, 251–257. doi: 10.4161/psb.6.2.14336
Spollen, W. G., Tao, W., Valliiyodan, B., Chen, K., Hejlek, L. G., Kim, J. J., et al. (2008). Spatial distribution of transcript changes in the maize primary root elongation zone at low water potential. BMC Plant Biol. 8:32. doi: 10.1186/1471-2229-8-32
Stines, A. P., Grubb, J., Gockowiak, H., Henschke, P. A., Hoj, P. B., and van Heeswijck, R. (2000). Proline and arginine accumulation in developing berries of Vitis vinifera L. in Australian vineyards: Influence of vine cultivar, berry maturity and tissue type. Aust. J. Grape Wine Res. 6, 150–158. doi: 10.1111/j.1755-0238.2000.tb00174.x
Strizhov, N., Abraham, E., Okresz, L., Blickling, S., Zilberstein, A., Schell, J., et al. (1997). Differential expression of two P5CS genes controlling proline accumulation during salt-stress requires ABA and is regulated by ABA1, ABI1 and AXR2 in Arabidopsis. Plant J. 12, 557–569. doi: 10.1111/j.0960-7412.1997.00557.x
Szabados, L., and Savoure, A. (2010). Proline: a multifunctional amino acid. Trends Plant Sci. 15, 89–97. doi: 10.1016/j.tplants.2009.11.009
Szekely, G., Abraham, E., Cseplo, A., Rigo, G., Zsigmond, L., Csiszar, J., et al. (2008). Duplicated P5CS genes of Arabidopsis play distinct roles in stress regulation and developmental control of proline biosynthesis. Plant J. 53, 11–28. doi: 10.1111/j.1365-313X.2007.03318.x
Tan, L., Eberhard, S., Pattathil, S., Warder, C., Glushka, J., Yuan, C., et al. (2013). An Arabidopsis cell wall proteoglycan consists of pectin and arabinoxylan covalently linked to an arabinogalactan protein. Plant Cell 25, 270–287. doi: 10.1105/tpc.112.107334
Tan, L., Showalter, A. M., Egelund, J., Hernandez-Sanchez, A., Doblin, M. S., and Bacic, A. (2012). Arabinogalactan-proteins and the research challenges for these enigmatic plant cell surface proteoglycans. Front. Plant Sci. 3:140. doi: 10.3389/fpls.2012.00140
Tegeder, M., and Ward, J. M. (2012). Molecular evolution of plant AAP and LHT amino acid transporters. Front Plant Sci. 3:21. doi: 10.3389/fpls.2012.00021
Thornburg, R. W. (2007). “Molecular biology of the Nicotiana floral nectar,” in Nectaries and Nectar, eds S. W. Nicolson, M. Nepi, and E. Pacini (Heidelberg: Springer-Verlag), 265–287.
Toka, I., Planchais, S., Cabassa, C., Justin, A. M., De Vos, D., Richard, L., et al. (2010). Mutations in the hyperosmotic stress-responsive mitochondrial BASIC AMINOACID CARRIER2 enhance proline accumulation in Arabidopsis. Plant Physiol. 152, 1851–1862. doi: 10.1104/pp.109.152371
Tonelli, C., Gavazzi, G., Manzocchi, L., Di Fonzo, N., and Soave, C. (1986). Opaque 6 allelic to pro1 mutant. Maize Newslett. 60, 100.
Tonelli, C., Racchi, M. L., Viani, I., and Gavazzi, G. (1984). In vitro development of proline requiring mutant maize kernels. Atti. Assoc. Genet. Italy 30:231.
Toonen, M. A. J., Schmidt, E. D. L., Van Kammen, A., and De Vries, S. C. (1997). Promotive and inhibitory effects of diverse arabinogalactan proteins on Daucus carota L. somatic embryogenesis. Planta 203, 188–195. doi: 10.1007/s004250050181
Trovato, M., Maras, B., Linhares, F., and Costantino, P. (2001). The plant oncogenic rolD encodes a functional ornithine cyclodeaminase. Proc. Nat. Acad. Sci. U.S.A. 98, 13449–13453. doi: 10.1073/pnas.231320398
Tseng, I. C., Hong, C. Y., Yu, S. M., and Ho, T. H. (2013). Abscisic acid- and stress-induced highly proline-rich glycoproteins regulate root growth in rice. Plant Physiol. 163, 118–134. doi: 10.1104/pp.113.217547
Ueda, A., Shi, W., Sanmiya, K., Shono, M., and Takabe, T. (2001). Functional analysis of salt-inducible proline transporter of barley roots. Plant Cell Physiol. 42, 1282–1289. doi: 10.1093/pcp/pce166
Ueda, A., Shi, W., Shimada, T., Miyake, H., and Takabe, T. (2008). Altered expression of barley proline transporter causes different growth responses in Arabidopsis. Planta 227, 277–286. doi: 10.1007/s00425-007-0615-y
Varner, J. E., and Lin, L. S. (1989). Plant cell wall architecture. Cell 56, 231–239. doi: 10.1016/0092-8674(89)90896-9
Velasquez, S. M., Ricardi, M. M., Dorosz, J. G., Fernandez, P. V., Nadra, A. D., Pol-Fachin, L., et al. (2011). O-glycosylated cell wall proteins are essential in root hair growth. Science 332, 1401–1403. doi: 10.1126/science.1206657
Velasquez, S. M., Ricardi, M. M., Poulsen, C. P., Oikawa, A., Dilokpimol, A., Halim, A., et al. (2015). Complex regulation of prolyl-4-hydroxylases impacts root hair expansion. Mol. Plant 8, 734–746. doi: 10.1016/j.molp.2014.11.017
Velasquez, S. M., Salgado Salter, J., Petersen, B. L., and Estevez, J. M. (2012). Recent advances on the post-translational modifications of EXTs and their roles in plant cell walls. Front. Plant Sci. 3:93. doi: 10.3389/fpls.2012.00093
Venekamp, J. H., and Koot, J. T. M. (1984). The distribution of free amino acids, especially of proline, in the organs of field bean plants. Vicia faba L., during development in the field. J. Plant Physiol. 116, 343–349. doi: 10.1016/S0176-1617(84)80113-3
Verbruggen, N., and Hermans, C. (2008). Proline accumulation in plants: a review. Amino Acids 35, 753–759. doi: 10.1007/s00726-008-0061-6
Verbruggen, N., Villarroel, R., and Van Montagu, M. (1993). Osmoregulation of a pyrroline-5-carboxylate reductase gene in Arabidopsis. Plant Physiol. 103, 771–781. doi: 10.1104/pp.103.3.771
Verslues, P. E., and Sharp, R. E. (1999). Proline accumulation in maize (Zea mays L.) primary roots at low water potentials. II. Metabolic source of increased proline deposition in the elongation zone. Plant Physiol. 119, 1349–1360. doi: 10.1104/pp.119.4.1349
Vignols, F., Jose-Estanyol, M., Caparros-Ruiz, D., Rigau, J., and Puigdomenech, P. (1999). Involvement of a maize proline-rich protein in secondary cell wall formation as deduced from its specific mRNA localization. Plant Mol. Biol. 39, 945–952. doi: 10.1023/A:1006129703262
Villalba, M., Batanero, E., Monsalve, R. I., Gonzalez, M. A., Lahoz, C., and Rodriguez, R. (1994). Cloning and expression of Ole e I, the major allergen from olive tree pollen. Polymorphism analysis and tissue specificity. J. Biol. Chem. 269, 15217–15222.
Voetberg, G. S., and Sharp, R. E. (1991). Growth of maize primary root at low water potentials. III. Role of increased proline deposition in osmotic adjustment. Plant Physiol. 96, 1125–1130. doi: 10.1104/pp.96.4.1125
Waditee, R., Hibino, T., Tanaka, Y., Nakamura, T., Incharoensakdi, A., Hayakawa, S., et al. (2002). Functional characterization of betaine/proline transporters in betaine-accumulating mangrove. J. Biol. Chem. 277, 18378–18382. doi: 10.1074/jbc.M112012200
Wakabayashi, K., Hoson, T., and Kamisaka, S. (1997). Changes in amounts and molecular mass distribution of cell-wall polysaccharides of wheat (Triticum aestivum L.) coleoptiles under water stress. J. Plant Physiol. 151, 33–40. doi: 10.1016/S0176-1617(97)80033-8
Wang, G., Sun, X., Wang, G., Wang, F., Gao, Q., Sun, X., et al. (2011). Opaque7 encodes an acyl-activating enzyme-like protein that affects storage protein synthesis in maize endosperm. Genetics 189, 1281–1295. doi: 10.1534/genetics.111.133967
Wang, G., Zhang, J., Wang, G., Fan, X., Sun, X., Qin, H., et al. (2014). Proline responding1 plays a critical role in regulating general protein synthesis and the cell cycle in maize. Plant Cell 26, 2582–2600. doi: 10.1105/tpc.114.125559
Wang, Z. Y., and He, J. X. (2004). Brassinosteroid signal transduction - choices of signals and receptors. Trends Plant Sci. 9, 91–96. doi: 10.1016/j.tplants.2003.12.009
Willats, W. G. T., and Knox, J. P. (1996). A role for arabinogalactan-proteins in plant cell expansion: evidence from studies on the interaction of glucosyl Yariv reagent with seedlings of Arabidopsis thaliana. Plant J. 9, 919–925. doi: 10.1046/j.1365-313X.1996.9060919.x
Wu, H., de Graaf, B., Mariani, C., and Cheung, A. Y. (2001). Hydroxyproline-rich glycoproteins in plant reproductive tissues: structure, functions and regulation. Cell. Mol. Life Sci. 58, 1418–1429. doi: 10.1007/PL00000785
Wu,H-M., Wong, E., Ogdahl, J., and Cheung, A. Y. (2000). A pollen tube growth-promoting arabinogalactan protein from Nicotiana alata is similar to the tobacco TTS protein. Plant J. 22, 165–176. doi: 10.1046/j.1365-313x.2000.00731.x
Wyatt, R. E., Nagao, R. T., and Key, J. L. (1992). Patterns of soybean proline-rich protein gene expression. Plant Cell. 4, 99–110. doi: 10.1105/tpc.4.1.99
Xu, C., Takc, T., Burbach, C., Menzel, D., and Samaj, J. (2011). Developmental localization and the role of hydroxyproline rich glycoproteins during somatic embryogenesis of banana (Musa spp. AAA). BMC Plant Biol. 11:38. doi: 10.1186/1471-2229-11-38
Xu, W. L., Zhang, D. J., Wu, Y. F., Qin, L. X., Huang, G. Q., Li, J., et al. (2013). Cotton PRP5 gene encoding a proline-rich protein is involved in fiber development. Plant Mol. Biol. 82, 353–365. doi: 10.1007/s11103-013-0066-8
Xue, X., Liu, A., and Hua, X. (2009). Proline accumulation and transcriptional regulation of proline biosynthesis and degradation in Brassica napus. BMB Rep. 42, 28–34. doi: 10.5483/BMBRep.2009.42.1.028
Yamada, H., and Kiyohara, H. (1999). “Complement-activating polysaccharides from medicinal herbs,” in Immunomodulatory Agents from Plants, ed. H. Wagner (Reinach: Birkhäuser Basel), 161–202. doi: 10.1007/978-3-0348-8763-2_7
Yamada, N., Promden, W., Yamane, K., Tamagake, H., Hibino, T., Tanaka, Y., et al. (2009). Preferential accumulation of betaine uncoupled to choline monooxygenase in young leaves of sugar beet-importance of long-distance translocation of betaine under normal and salt stressed conditions. J. Plant Physiol. 166, 2058–2070. doi: 10.1016/j.jplph.2011.03.007
Yamada, N., Sakakibara, S., Tsutsumi, K., Waditee, R., Tanaka, Y., and Takabe, T. (2011). Expression and substrate specificity of betaine/proline transporters suggest a novel choline transport mechanism in sugar beet. J. Plant Physiol. 168, 1609–1616. doi: 10.1016/j.jplph.2011.03.007
Ye, Z. H., Song, Y. R., Marcus, A., and Varner, J. E. (1991). Comparative localization of three classes of cell wall proteins. Plant J. 1, 175–183. doi: 10.1111/j.1365-313X.1991.00175.x
Ye, Z. H., and Varner, J. E. (1991). Tissue-specific expression of cell wall proteins in developing soybean tissues. Plant Cell 3, 23–37. doi: 10.1105/tpc.3.1.23
Zhan, X., Wang, B., Li, H., Liu, R., Kalia, R. K., Zhu, J. K., et al. (2012). Arabidopsis proline-rich protein important for development and abiotic stress tolerance is involved in microRNA biogenesis. Proc. Nat. Acad. Sci. U.S.A. 109, 18198–18203. doi: 10.1073/pnas.1216199109
Zhang, H. Q., Croes, A., and Linskens, H. (1982). Protein synthesis in germinating pollen of Petunia: role of proline. Planta 154, 199–203. doi: 10.1007/BF00387864
Zhang, X., Ma, H., Qi, H., and Zhao, J. (2014). Roles of hydroxyproline-rich glycoproteins in the pollen tube and style cell growth of tobacco (Nicotiana tabacum L.). J. Plant Physiol. 171, 1036–1045. doi: 10.1016/j.jplph.2014.02.010
Zhao, Z. D., Tan, L., Showalter, A. M., Lamport, D. T. A., and Kieliszewski, M. J. (2002). Tomato LeAGP-1 arabinogalactan-protein purified from transgenic tobacco corroborates the Hyp contiguity hypothesis. Plant J. 31, 431–444. doi: 10.1046/j.1365-313X.2002.01365.x
Keywords: proline, plant ontogeny, proline-rich proteins, hydroxylproline-rich glycoproteins, hybrid proline-rich proteins
Citation: Kavi Kishor PB, Hima Kumari P, Sunita MSL and Sreenivasulu N (2015) Role of proline in cell wall synthesis and plant development and its implications in plant ontogeny. Front. Plant Sci. 6:544. doi: 10.3389/fpls.2015.00544
Received: 17 April 2015; Accepted: 06 July 2015;
Published: 20 July 2015.
Edited by:
Sakiko Okumoto, Virginia Polytechnic Institute and State University, USAReviewed by:
Naser A. Anjum, University of Aveiro, PortugalSantiago Signorelli, Universidad de la República, Uruguay
Maurizio Trovato, Sapienza – Università di Roma, Italy
Copyright © 2015 Kavi Kishor, Hima Kumari, Sunita and Sreenivasulu. This is an open-access article distributed under the terms of the Creative Commons Attribution License (CC BY). The use, distribution or reproduction in other forums is permitted, provided the original author(s) or licensor are credited and that the original publication in this journal is cited, in accordance with accepted academic practice. No use, distribution or reproduction is permitted which does not comply with these terms.
*Correspondence: Polavarapu B. Kavi Kishor, Department of Genetics, Osmania University, Hyderabad 500007, India, pbkavi@yahoo.com