- 1Molecular Genetics Department, Center for Research in Agricultural Genomics, Consortium CSIC-IRTA-UAB-UB, Parc de Recerca Universitat Autònoma de Barcelona, Barcelona, Spain
- 2Instituto de Biología Molecular y Celular de Plantas, Consejo Superior de Investigaciones Científicas – Universidad Politécnica de Valencia, Valencia, Spain
- 3Departamento de Genética, Universidad de Córdoba, Córdoba, Spain
The architecture of the inflorescence, the shoot system that bears the flowers, is a main component of the huge diversity of forms found in flowering plants. Inflorescence architecture has also a strong impact on the production of fruits and seeds, and on crop management, two highly relevant agronomical traits. Elucidating the genetic networks that control inflorescence development, and how they vary between different species, is essential to understanding the evolution of plant form and to being able to breed key architectural traits in crop species. Inflorescence architecture depends on the identity and activity of the meristems in the inflorescence apex, which determines when flowers are formed, how many are produced and their relative position in the inflorescence axis. Arabidopsis thaliana, where the genetic control of inflorescence development is best known, has a simple inflorescence, where the primary inflorescence meristem directly produces the flowers, which are thus borne in the main inflorescence axis. In contrast, legumes represent a more complex inflorescence type, the compound inflorescence, where flowers are not directly borne in the main inflorescence axis but, instead, they are formed by secondary or higher order inflorescence meristems. Studies in model legumes such as pea (Pisum sativum) or Medicago truncatula have led to a rather good knowledge of the genetic control of the development of the legume compound inflorescence. In addition, the increasing availability of genetic and genomic tools for legumes is allowing to rapidly extending this knowledge to other grain legume crops. This review aims to describe the current knowledge of the genetic network controlling inflorescence development in legumes. It also discusses how the combination of this knowledge with the use of emerging genomic tools and resources may allow rapid advances in the breeding of grain legume crops.
Introduction: Inflorescence Architecture
One of the most interesting features of plant development is the fact that all aerial parts of the plant body are generated from the activity of the shoot apical meristem (SAM). The SAM is located at the tip of the plant shoot and contains a central pool of stem cells that are able to self-maintain together with peripheral dividing cells required for organ initiation (Steeves and Sussex, 1989). During the vegetative phase, the SAM generates leaf primordia with axillary vegetative shoots, in a sequential manner until floral transition is attained. Upon floral transition, the SAM becomes an inflorescence meristem that, either directly or in flower-bearing shoots, produces the floral meristems that form the flowers. The position where meristems are formed in the inflorescence apex and the activity of those meristems determines to a high degree the architecture of the inflorescence, the part of the plant that bears the flowers (Weberling, 1989a; Benlloch et al., 2007; Prusinkiewicz et al., 2007).
A basic classification divides inflorescences into two groups, depending on whether the primary inflorescence axis terminates into a flower or not. According to this classification, determinate inflorescences are those where, after floral transition, the SAM acquires the identity of a floral meristem, which forms a terminal flower (TFL; Weberling, 1989a). This type of inflorescence includes extremely simple architectures, such as that of Tulipa sp, to more complex forms such as the cymes, found for instance, in some Solanaceae species (Lippman et al., 2008), where after formation of the TFL by the primary axis growth continues from lateral axes that repeat this pattern (Figure 1; Weberling, 1989a). On the contrary, in indeterminate inflorescences the SAM is never converted into a floral meristem and the inflorescence meristem continues producing floral meristems until senescence, as for example, occurs in the model plant species Arabidopsis thaliana (Figure 1; Weberling, 1989a; Benlloch et al., 2007).
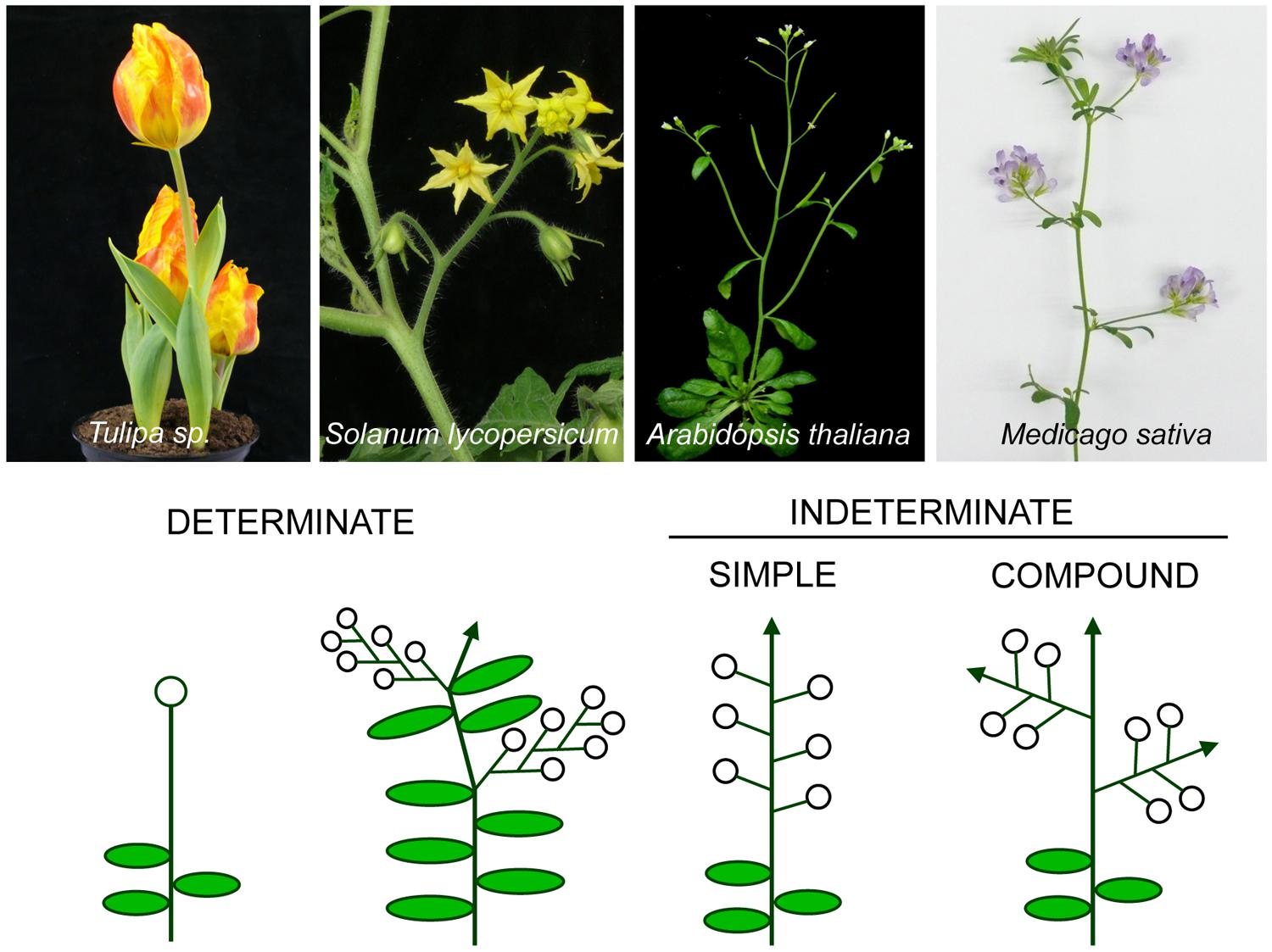
FIGURE 1. Different types of inflorescence architecture. Images of plant species representative of main inflorescence types (top) and the corresponding diagrams (below) of the architecture of their inflorescences. Open circles represent flowers and arrows represent indeterminate shoots.
According to another main classification, Arabidopsis is also an example of a simple inflorescence, as its flowers are directly formed in the primary inflorescence axis. In contrast, other plants have evolved to a more complex architecture and have compound inflorescences (Figure 1; Weberling, 1989a). In compound inflorescences, the flowers are not formed in the primary inflorescence axes but, instead, they are formed in secondary or higher order axes (Figure 1). Compound inflorescences are typical, for instance, of grasses and legumes (Weberling, 1989b; Kellogg, 2007).
The architecture of the inflorescence, which conditions how many flowers (and therefore, fruits and seeds) are produced, and their position in the plant, has a profound impact on key agronomical aspects such as crop management, yield and yield stability. For instance, in crops such as tomato and grain legumes, determinate varieties have been traditionally selected because they show favorable traits for an efficient cultivation and harvest. Determinate varieties often display a shorter flowering time and earlier maturation and they are usually more compact, facilitating large-scale harvesting (Tian et al., 2010; Park et al., 2014). Taken into account the great economic importance of grain legumes, which include broadly used species for food and feed, it is of great interest to understand the genetic bases of inflorescence architecture in these species. In this context, genes controlling inflorescence development are instrumental for the generation of breeding and biotechnological tools to design new legume crops better adapted to different environmental conditions.
In this review, we describe the current knowledge on the genetic control of inflorescence architecture in grain legumes, and discuss the biotechnological potential of this knowledge for the development and selection of more productive and sustainable legume crop varieties.
Genetic Network Controlling Meristem Identity in the Arabidopsis Inflorescence
A main factor that shapes inflorescence architecture is the identity of the meristems produced in the inflorescence apex, which determines the relative position where flowers are formed.
Arabidopsis thaliana is one of the best-known examples of simple indeterminate inflorescences. In Arabidopsis, upon floral transition, the vegetative meristem becomes an inflorescence meristem, which produces floral meristems laterally (Figures 1 and 2). The development of the Arabidopsis inflorescence can be mostly explained by the function and mutual regulation of three genes: TERMINAL FLOWER 1 (TFL1), LEAFY (LFY), and APETALA 1 (AP1) (Shannon and Meeks-Wagner, 1993; Liljegren et al., 1999; Blazquez et al., 2006). These three genes act as opposing forces maintaining the balance between inflorescence and floral meristem identity at the inflorescence apex (Ratcliffe et al., 1999; Blazquez et al., 2006).
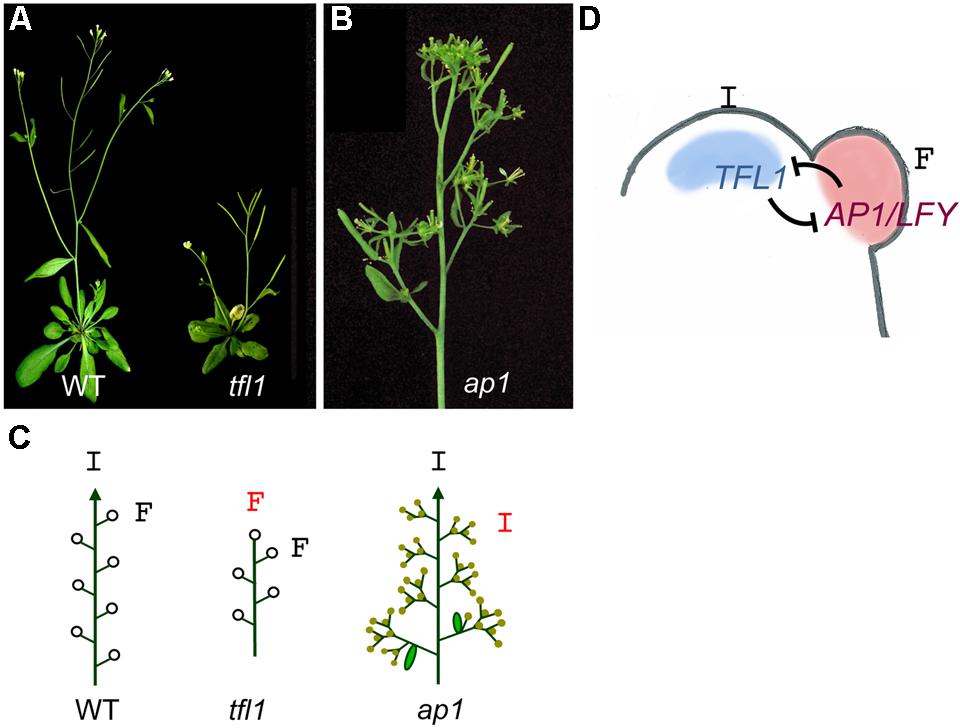
FIGURE 2. Meristem identity genes in Arabidopsis. (A) Images of wild-type (WT) and tfl1 mutant plants. While in the WT the main inflorescence and the lateral inflorescences (appearing in the axil of cauline leaves) show indeterminate growth, in the tfl1 mutant the main inflorescence ends into a terminal flower (a fruit in this image) and lateral branches are replaced by solitary flowers. (B) Inflorescence of an ap1 mutant. Individual flowers are replaced by branched structures. (C) Diagrams of meristem identity in the inflorescences of the wild-type and the tfl1 and ap1 mutants. In tfl1, the indeterminate inflorescence apex (I) is replaced by a terminal flower (F) while in ap1, the flowers are replaced by inflorescence-like structures. Arrowheads, indeterminate shoot; open circles, flowers, closed circles, abnormal flowers. (D) Model for specification of meristem identity in the simple inflorescence of Arabidopsis. In the Arabidopsis inflorescence apex, TFL1 expression in the inflorescence meristem (I) and AP1 and LFY expression in the floral meristem (F) are required for these meristems to acquire their identity. Expression of these genes in their correct domains is maintained by mutual repressive interactions.
LEAFY
The LFY and AP1 genes are essential for the specification of floral meristem identity in Arabidopsis. LFY codes for a plant-specific transcription factor that is expressed at very early stages in the flanks of the inflorescence meristem, at the floral “anlagen” (the groups of cells that will form the floral meristems) directing these incipient primordia to a floral meristem fate (Schultz and Haughn, 1991; Huala and Sussex, 1992; Weigel et al., 1992; Maizel et al., 2005). Hence, meristems produced by the SAM after floral transition in loss-of-function lfy mutants have problems to acquire floral identity and retain features typical of inflorescence meristems. This results in replacement of the first flowers on the inflorescence stem by shoots. Nevertheless, at later stages of development, the inflorescencs of lfy mutants produce flower-like structures, which show that, in addition to LFY, other genes participate in the specification of floral meristem identity.
APETALA1
The AP1 gene codes for a MADS-box transcription factor also required for floral meristem identity specification (Mandel et al., 1992; Weigel and Meyerowitz, 1993). AP1 transcription is directly activated by LFY at stage 1 floral meristems (Wagner et al., 1999). In ap1 mutants the first flowers on the inflorescence stem are replaced by shoots and their flowers display severe morphological and homeotic alterations. The sepals of the ap1 mutant flowers are replaced by bract-like organs and, in the axils of these organs, secondary flowers are produced, which again may produce axillary flowers (Figure 2; Irish and Sussex, 1990; Bowman et al., 1993). The formation of bract-like organs and ramified flowers indicates a partial reversion from floral fate to inflorescence. This incomplete reversion suggests that other genes may act redundantly with AP1 in the specification of floral meristem fate. In Arabidopsis, this redundant function is played by the CAULIFLOWER (CAL) gene, a paralogue of AP1 that is also expressed in early floral meristems as a result of LFY direct activation (Bowman et al., 1993; Mandel and Yanofsky, 1995; William et al., 2004). The combination of ap1 and cal mutations results in a complete absence of floral meristem identity acquisition. Thus, in double ap1 cal mutants, inflorescence meristems produce new meristems that completely fail to acquire floral fate behaving like new inflorescence meristems that continue to divide, producing proliferating structures with cauliflower morphology (Bowman et al., 1993; Kempin et al., 1995; Mandel and Yanofsky, 1995).
TERMINAL FLOWER 1
The TFL1 gene encodes for a phosphatidyl-ethanolamine-binding protein (PEBP) and it is expressed in a subset of cells of the SAM at low level during vegetative stage (Figure 2; Bradley et al., 1997; Ohshima et al., 1997). TFL1 expression increases after floral transition and TFL1 protein acts as a signal controlling inflorescence meristem identity. Mutations in the TFL1 gene cause a conversion of the inflorescence meristems into floral meristems, producing the abrupt termination of the main inflorescence stem in a TFL and the substitution of lateral branches by solitary axillary flowers (Figure 2; Shannon and Meeks-Wagner, 1991; Alvarez et al., 1992; Schultz and Haughn, 1993). Therefore, the tfl1 mutation changes the Arabidopsis inflorescence from an indeterminate to a determinate type. In agreement with its expression in the vegetative meristem, TFL1 also has a role controlling the length of the vegetative phase, acting as a repressor of flowering. Thus, tfl1 mutant plants flower earlier that the wild type, with a reduction in the number of leaves and branches produced in the main stem (Shannon and Meeks-Wagner, 1991; Schultz and Haughn, 1993).
Analyses of genetic interactions between these mutants, together with the expression patterns of TFL1, LFY, and AP1, led to a model for the control of meristem identity in the inflorescence of Arabidopsis (Figure 2; Liljegren et al., 1999; Ratcliffe et al., 1999; Blazquez et al., 2006). According to this model, inflorescence meristem identity in Arabidopsis is maintained by the activity of TFL1, which represses AP1 and LFY genes in the inflorescence meristem, preventing early inflorescence termination. In fact, in tfl1 mutants, AP1 and LFY are ectopically expressed in the inflorescence meristems, which in turn acquire floral fate and produce terminal and axillary flowers (Mandel et al., 1992; Weigel et al., 1992; Bradley et al., 1997). Conversely, LFY and AP1 are expressed in the meristems produced at the flanks of the inflorescence meristem, which thus acquire floral identity and form the flowers. LFY and AP1 repress TFL1 in the newly formed floral meristems, allowing up-regulation of floral organ identity genes and hence the formation of flowers (Parcy et al., 1998; Liljegren et al., 1999; Wagner et al., 1999; Kaufmann et al., 2010). This simple model of mutual repression between TFL1 and LFY/AP1 elegantly explains the maintenance of the indeterminate inflorescence meristem in Arabidopsis and the formation of floral meristems at its flanks.
Genetic Network Controlling Meristem Identity in the Legume Inflorescence
As mentioned above, legumes are characterized by a compound indeterminate inflorescence (Weberling, 1989b; Benlloch et al., 2007; Prenner, 2013; Hofer and Noel Ellis, 2014). The ontogeny of the compound inflorescence has been described in detail in pea (Pisum sativum; Singer et al., 1999). Briefly, the SAM undergoes a transition from a vegetative meristem to a primary inflorescence (I1) meristem, with indeterminate growth. This I1 meristem, instead of producing floral meristems at its flanks, as in the case of Arabidopsis, produces secondary inflorescence meristems (I2), which in turn will generate floral meristems (F). In pea, the I2 usually produces 1-2 floral meristems before it ceases growing, forming a residual organ or stub (Figure 3). Therefore, the appearance of the I2 meristem supposes an additional level of complexity in the legume inflorescence, as compared to Arabidopsis, and different genes have been coopted to orchestrate the development of the compound inflorescence in legumes.
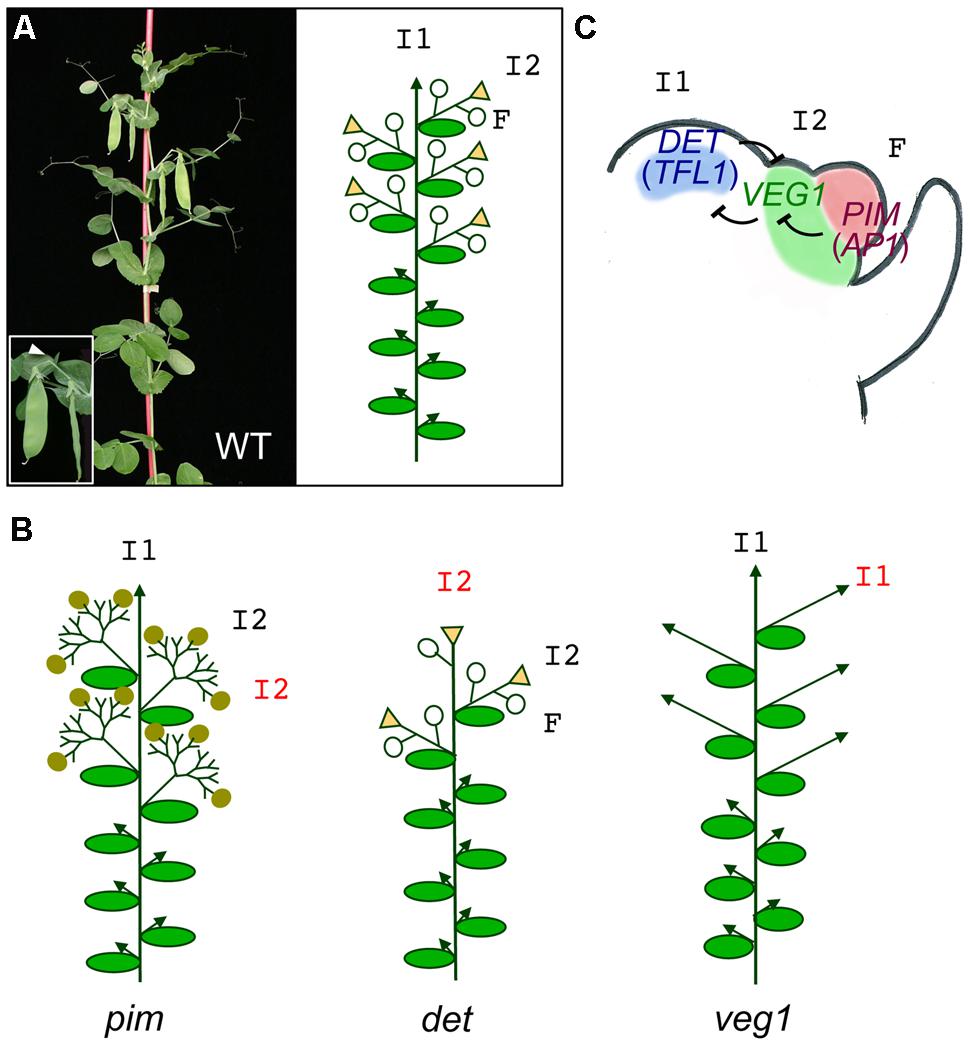
FIGURE 3. Meristem identity genes in pea. (A) Picture and diagram of a pea WT plant. The main primary inflorescence (I1) shows indeterminate growth (arrowhead). Upper nodes of the plant contain secondary inflorescences (I2) which produce 1–2 flowers (F, open circles) and terminate into a stub (triangles). The inset shows a close up of a secondary inflorescence with two flowers (pods) and the stub (arrowhead). (B) Diagrams of meristem identity of the pim, det, and veg1 mutants. In the pim mutant, flowers are replaced by proliferating I2s with abnormal flowers (closed circles). In the det mutant, the primary inflorescence is replaced by a terminal secondary inflorescence. In the veg1 mutant, the I2s are replaced by vegetative branches with I1 identity. (C) Model for specification of meristem identity in the compound pea inflorescence. In the pea inflorescence apex, DET expression in the primary inflorescence meristem (I2), VEG1 in the secondary inflorescence meristem (I2) and PIM in the floral meristem (F) are required for these meristems to acquire their identity. Expression of these genes in their correct domains is maintained by a network of mutual repressive interactions.
Among legumes, pea is the species where genetics of inflorescence development is best understood. In this section, we will review the current knowledge on the genes controlling floral meristem identity, as well as primary and secondary inflorescence meristem identity, primarily in this species and then extending to what is known for other legume species.
Floral Meristem Identity
In pea, floral meristem identity is controlled by the homologs of the LFY and AP1 genes from Arabidopsis. The PROLIFERATING INFLORESCENCE MERISTEM (PIM/PEAM4) and UNIFOLIATA (UNI) genes have been characterized as homologs to AP1 and LFY, respectively (Hofer et al., 1997; Berbel et al., 2001; Taylor et al., 2002). The function of PIM and UNI in the control of inflorescence architecture is similar to their counterparts in Arabidopsis, although some functional differences are also found, possibly accounting for the more complex inflorescence and flower development exhibited by legumes.
PROLIFERATING INFLORESCENCE MERISTEM
The pea MADS-box gene PIM/PEAM4 is specifically expressed in floral meristems (Berbel et al., 2001, 2012; Taylor et al., 2002). Its pattern of expression is similar to AP1, being uniformly expressed in floral meristems at early stages and restricted to the sepal and petal primordia in later stages. Overexpression of PIM in Arabidopsis causes early flowering and, often, the formation of a TFL and replacement of branches by axillary flowers. These phenotypic alterations are also observed in 35S::AP1 plants and indicate that PIM specifies floral meristem identity, being its expression in the inflorescence meristem sufficient to convert it into a floral meristem. pim mutants do not show alterations in vegetative traits and I1 and I2 meristems are correctly specified. However, pim I2 meristems, rather than producing floral meristems, produce new I2 meristems in a reiterative manner (Figure 3; Taylor et al., 2002; Berbel et al., 2012), somehow resembling the proliferative inflorescences of the Arabidopsis ap1 cal double mutant (Kempin et al., 1995). Likewise, the I2 meristems of the pim mutants are eventually able to produce floral meristems; in these cases the meristems only acquire partial floral fate, as indicated by the production of flowers with bract-like organs and other floral identity defects (Taylor et al., 2002).
The function of PIM seems to be conserved in other grain legumes. PIM homologs have also been described in Lotus japonicus, LjAP1a and LjAP1b, and in Medicago truncatula, MtPIM (Dong et al., 2005; Benlloch et al., 2006). These homologs show an expression pattern during floral meristem initiation and development very similar to that of PIM. The Medicago mtpim mutant also exhibits a proliferating inflorescence phenotype, somehow more severe than the pea mutants, where floral meristems are replaced by proliferating I2 meristems (Benlloch et al., 2006).
UNIFOLIATA
Another gene with a key function in the in the initiation of floral meristems in pea is the LFY homolog UNI (Hofer et al., 1997). In the loss-of-function uni mutants, floral meristems are not correctly specified and rather than flowers they produce proliferating structures, mainly formed by sepals and carpels. These structures derive from flowers with severe loss of determinacy, where supernumerary flowers reiteratively arise in the axil of sepals, apparently replacing petals and stamens (Hofer et al., 1997). This phenotype partly resembles that of lfy mutants in Arabidopsis, whose flowers never form petals or stamens. Nevertheless, the uni phenotype is less severe than that of lfy mutants as, in the strict sense, replacement of flowers by branches is not observed in uni mutants; instead, the proliferating uni flowers rather resemble the branched flowers of Arabidopsis ap1 mutants. Mutants in the UNI homologs of L. japonicus, LjLFY and M. truncatula, SINGLE LEAFLET1 (SGL1) have also been described and both produce flowers with a very similar phenotype to those of uni (Dong et al., 2005; Wang et al., 2008a).
Expression of UNI in pea floral meristems is detected in developing floral organ primordia, declining as they expand. In L. japonicus and M. truncatula the UNI homologs also show expression in young floral organ primordia. In addition, although uni mutants do not show apparent defects in I2 meristem specification, expression of UNI genes was also described in I2 meristems in pea and M. truncatula.
UNI has an additional function in the control of the compound leaf development in pea, as shown by the phenotype of uni mutants, where the complexity of the leaves is strongly reduced (Hofer et al., 1997; Gourlay et al., 2000). The number of leaflets is reduced in uni mutant and tendrils are not formed. This function seems conserved in other grain legumes from the IRCL clade, as mutants in the L. japonicus and M. truncatula UNI homologs, LjLFY and SLG1, also show a strong reduction in the complexity of their leaves (Dong et al., 2005; Wang et al., 2008a). In this context, compound leaves are interpreted as “partially indeterminate” and have been proposed that UNI would have a role in the control of determinacy not only of floral meristems but also of leaf primordia (Hofer and Noel Ellis, 2014).
I1 Meristem Identity
DETERMINATE
As described above, grain legumes have an indeterminate inflorescence, where the I1 meristem does not form a TFL but continues producing lateral I2s until it ceases growing (Figures 1 and 3). Pea mutants in the DETERMINATE (DET) gene have a determinate inflorescence that produces 1-2 normal lateral I2s and an apparent TFL, resembling the Arabidopsis tfl1 mutant (Singer et al., 1990). However, a closer analysis reveals that the I1 meristem of det mutants do not directly form a (terminal) flower but, instead, it develops as a stem that produces a flower in a lateral position and terminates into a stub, like the I2s. This shows that in pea det mutants, rather than the conversion of the inflorescence meristem into floral meristem observed in Arabidopsis tfl1 mutants, what really takes place is the conversion of the I1 meristem into an I2 meristem (Figure 3; Singer et al., 1990). The molecular identification of the DET gene showed that indeed it corresponds to a homolog of the Arabidopsis TFL1 gene, which was named PsTFL1a (Foucher et al., 2003). DET/PsTFL1a is expressed only after floral transition, in the I1 meristem, in agreement with its function as an I1 meristem identity gene (Foucher et al., 2003; Berbel et al., 2012).
Mutants with determinate inflorescences have been described in other grain legumes. In the last years, the underlying mutations of some of these phenotypes have been identified, and these have been shown to affect DET/PsTFL1a homologs (Avila et al., 2007; Liu et al., 2010; Repinski et al., 2012; Dhanasekar and Reddy, 2014; Mir et al., 2014). An exception to this is the soybean dt2 mutants, which show a semideterminate phenotype that is not caused by a mutation in a TFL1-like gene. The function of the Dt2 gene will be discussed in next sections.
Another difference between the pea det mutant and the Arabidopsis tfl1 mutant is that tfl1 mutations, in addition to determination of the inflorescence, cause early flowering in Arabidopsis. Foucher et al. (2003) also showed that the early flowering phenotype of recessive mutations in the pea LATE FLOWERING loci (LF, described by Weller and Ortega, in this Research Topic) was due to mutations in another TFL1-like gene, PsTFL1c, a paralogue of DET/PsTFL1a. Interestingly, the pea det lf double mutant plants are early flowering and determinate, which strongly resembles the phenotype of Arabidopsis tfl1 mutants. This has lead to the attractive idea that the TFL1 function, which in Arabidopsis controls both the vegetative and the inflorescence phases (Ratcliffe et al., 1998), in pea would be divided between two genes, DET and LF (Foucher et al., 2003).
I2 Meristem Identity
Particularly interesting is the specification of the secondary inflorescence (I2) meristem, as the formation of these meristems is crucial for the development of higher order inflorescences and hence for the formation of the characteristic legume compound inflorescences. Secondary inflorescence (I2) meristems do not form in simple inflorescences, indicating that new genetic functions must have appeared in evolution to direct the acquisition of I2 meristem identity. The identification of these novel genetic functions, and the characterization of their conservation across legumes, is important for a better understanding of compound inflorescence development.
VEGETATIVE1
The genetic basis of I2 meristem identity acquisition was elucidated by the analysis of a pea mutant in the VEGETATIVE1 (VEG1) locus. veg1 mutant plants present a extreme non-flowering phenotype: no flowers or floral organs are produced in veg1 plants under any growing condition (Gottschalk, 1979; Reid and Murfet, 1984). Characterization of primary inflorescence markers in veg1 mutant discarded the possibility that the floral transition was blocked or delayed in this mutant. Instead, the non-flowering phenotype of veg1 is explained by a blockage on I2 meristem identity acquisition. Transition from vegetative to I1 meristem apparently takes place but the I1 meristem produces lateral meristems that, unable to acquire I2 identity, continue to develop as I1s, producing vegetative branches that replace I2 inflorescences (Figure 3; Gottschalk, 1979; Reid and Murfet, 1984; Berbel et al., 2012). In agreement with this, DET expression, which as discussed above specifies I1 meristem identity, was found in the lateral meristems produced at the flanks of the apical I1 meristem of the veg1 plants, indicating that in wild-type (WT) pea, VEG1 is required to confer I2 identity to these lateral meristems and that to achieve that, VEG1 directly or indirectly represses DET expression in these meristems (Berbel et al., 2012).
VEG1 was shown to correspond to PsFULc, a MADS-box gene belonging to the AGL79 clade of the AP1/SQUA/FUL genes (Berbel et al., 2012). In agreement with its proposed function in the control of I2 meristem identity, VEG1/PsFULc gene is expressed after floral transition in the inflorescence apex, specifically in I2 meristems, just before PIM upregulation and floral meristem development, and its expression is not detected in I1 or in floral meristems.
VEGETATIVE2 and GIGAS
Two other genes are considered to participate in the control of I2 meristem identity in pea: GIGAS and VEGETATIVE2 (Murfet and Reid, 1993; Beveridge and Murfet, 1996; Reid et al., 1996).
Plants with severe mutations in the GIGAS locus show an extreme non-flowering phenotype under long-day (LD) conditions. Similar to veg1, gigas mutants show apparently normal vegetative development, and later in development, the induction of inflorescence markers, such as upregulation of DET and bud outgrowth (Beveridge and Murfet, 1996; Hecht et al., 2011), indicating that transition from vegetative to I1 meristem also takes place in gigas mutants. However, expression of PIM and VEG1 is never induced under LD in the inflorescence of the gigas mutants, which indicates that I2 specification does not take place (Hecht et al., 2011; Berbel et al., 2012). GIGAS corresponds to PsFTa1, one of the pea homologs of the FLOWERING LOCUS T (FT) gene in Arabidopsis (Hecht et al., 2011). FT has been identified as a major component of the florigen, the floral promoting signal that travels from the leaf to the apex and initiates the floral transition (reviewed in Pin and Nilsson, 2012). FT, in a complex with the bZIP transcription factor FD, directly upregulates the expression of floral genes, such as AP1 (Abe et al., 2005; Wigge et al., 2005; Pin and Nilsson, 2012). However, in pea, and in most legume species, there are several FT genes, comprising three distinct clades; analysis of pea FT genes has revealed a much more complex regulation of photoperiodic flowering in pea compared to Arabidopsis, with different FT homologs expressed in leaf and/or apex, and displaying different responsiveness to photoperiod (described by Weller and Ortega, 2015, in this Research Topic).
Loss-of-function mutations in VEG2 also cause a phenotype related to I2 meristem development. Thus, the veg2-1 mutant displays a non-flowering phenotype similar to veg1, while veg2-2, a weaker allele, shows a delay in flowering and a conversion of I2 inflorescences into flower-bearing branch-like structures with indeterminate growth, which resemble the primary I1 inflorescence of WT plants (Murfet and Reid, 1993). VEG2 has been recently shown to correspond to PsFDa, a pea orthologue of FD (Sussmilch et al., 2015). As in gigas mutants, expression of PIM and VEG1 is never detected in the “inflorescence” apex of veg2-1 mutant. As the VEG2/FDa protein is able to interact with GIGAS/FTa1, a likely possibility is that GIGAS and VEG2 form a transcriptional complex responsible for the upregulation of VEG1, which would not take place in gi or veg2 mutants, thus explaining the absence of I2 development observed in these mutants.
Model for Inflorescence Meristem Identity in Legumes
A genetic model for the specification of meristem identity in the pea compound inflorescences has been proposed based on the genetic studies described above and the existing knowledge on Arabidopsis inflorescence development (Figure 3).
In this model, primary and secondary inflorescence meristem identity is regulated by DET (TFL1 homolog) and VEG1, respectively. DET and VEG1 repress each other expression, ensuring the balance between the indeterminate development of the apical primary inflorescence (I1) and the formation of secondary inflorescence (I2) meristems at its flanks. The identity of the floral meristems produced by the I2 is controlled by PIM, the homolog of AP1. PIM expression in the newly formed floral meristems represses VEG1 in this tissue, allowing floral development to proceed.
Consistent with this model, the missexpression of VEG1 in the inflorescence apex of det mutant plants would cause the apical meristem to acquire I2 identity and to develop as a terminal I2. Conversely, in a veg1 mutant, DET is ectopically expressed in the lateral meristems produced by the I1 and these lateral meristems then fail to acquire I2 identity, developing as I1 inflorescences. Finally, as it was also observed that loss-of-function of PIM leads to a missexpression of VEG1 in the newly formed “floral” meristems, this ectopic VEG1 expression would explain why these meristems do not acquire floral fate but instead develop as I2 meristems. This simple model based on the DET/VEG1/PIM regulatory module (Figure 3) is a more complex version of the TFL1/LFY/AP1 model (Figure 2) that explains the development of the Arabidopsis simple inflorescence. With the introduction of a novel genetic function, represented by VEG1, which is placed in between the mutual antagonistic activities of DET and PIM, the pea model explains elegantly how the transient I2 meristem appears and, hence, the development of the compound inflorescence in legumes.
Though most grain legumes have compound inflorescences with a similar architecture to pea (Weberling, 1989b; Benlloch et al., 2007; Prenner, 2013; Hofer and Noel Ellis, 2014), the conservation of the DET/VEG1/PIM regulatory network among the legume family remains to be proved. Diverse evidence suggests that, indeed, the properties and architecture of this network could be conserved among grain legumes. The determinate growth habit caused by mutations in DET/TFL1-homologs in other grain legumes indicates that DET function is conserved in these species (Liu et al., 2010; Tian et al., 2010; Kwak et al., 2012; Repinski et al., 2012; Dhanasekar and Reddy, 2014). Also, the characterization of MtPIM, the M. truncatula homolog of PIM, indicates conservation of this function in Medicago (Benlloch et al., 2006). To our knowledge, there are no reported examples of VEG1 loss-of-function mutants outside pea. Nevertheless, the dominant semideterminate inflorescence phenotype in the dt2 soybean mutants has been recently associated to the overexpression of a FULc/VEG1 homolog in the inflorescence apex of the mutant (Ping et al., 2014). According to the proposed model, elevated expression of a VEG1 gene in the apical I1 meristem should repress DET expression and cause determination, hence the phenotype of the dt2 mutants is consistent with the proposed repression of DET by VEG1 also being conserved in other grain legumes.
Genetic Control of the Activity of Meristems in the Legume Inflorescence
Apart from meristem identity, which determines the relative position where flowers are formed in the inflorescence apex, a second factor with a key influence on the architecture of the inflorescence is the activity of the meristems. In plants with an indeterminate growth habit, such as grain legumes, the SAMs produce lateral structures, branches and flowers, while they remain active. Therefore, the number of secondary inflorescences (I2) produced by the primary inflorescence (I1), and the number of flowers produced by the secondary inflorescences, depends on for how long the I1 and I2 meristems, respectively, remain active.
The number of flowers produced in each I2, as well as the number of I2s produced by the primary inflorescence, is characteristic of each species or cultivar (Murfet, 1985; Annicchiarico and Iannucci, 2008; Smýkal et al., 2014). Thus, for instance, while pea usually produce between 1 and 2 flowers per secondary inflorescence, the secondary inflorescences of Mellilotus officinalis (yellow sweet clover) usually have 20–30 flowers.
Number of Flowers per Secondary Inflorescence, Activity of the I2 Meristem
In spite of its possible influence on seed yield of crops, there are relatively few studies about the genetic control of flower number per I2 in legumes, which depends on the activity of the I2 meristem.
In the case of pea, where I2 meristems usually produce 1–2 flowers, several studies have identified loci responsible for limiting the number of floral meristems initiated by the I2 meristem before stub formation. Classical studies indicate that this trait is controlled by two genes, Fn and Fna, whose single recessive mutations cause an increase in the number of flowers per I2, being higher in the double recessive genotypes, the so-called multipod phenotype (White, 1917; Lamprecht, 1947). More recent studies describe that mutations in NEPTUNE (NEP), a gene represented by two recessive alleles, also causes a multipod phenotype (Singer et al., 1999), although the possible allelic relationship between NEP and the Fn and Fna genes has not been reported. In addition to these genetic factors, the number of flowers per I2 is also affected by growing conditions (Hole and Hardwick, 1976; Murfet, 1985; Singer et al., 1999) and mutations in the flowering time genes HIGH RESPONSE (HR) and STERILE NODES (SN), involved in photoperiod response, also strongly influence this trait, with the number of flowers being decreased by recessive sn alleles and increased by dominant HR alleles (Murfet, 1985; Reid et al., 1996; Weller et al., 2012; Liew et al., 2014).
Chickpea (Cicer arietinum) is the grain legume where genetics of number of flowers per I2 is possibly better understood. Most chickpea genotypes have only one flower per I2, and it has been proposed that this solitary flower could be a reduction of a multi-flowered ancestor (Prenner, 2013). Natural mutations that produce double triple and multi-flowers per I2 have been reported (Knights, 1987; Singh and Chaturvedi, 1998; Gaur and Gour, 2002). Two loci have been identified that control this trait: Sfl and Cym. Plants with a Sfl-Cym genotype are single-flowered, while the recessive sfld and sflt alleles cause a double-flower and triple-flower phenotype, respectively, the sfld allele being dominant over sflt (Srinivasan et al., 2006). The multi-flower phenotype is found in plants homozygous for the recessive allele in the Cym gene, which produces apparently cymose secondary inflorescences (Gaur and Gour, 2002).
As in the case of pea, number of flowers per I2 in chickpea is also affected by environmental conditions. Thus, sfld allele showed higher penetrance and expressivity under soil moisture stress conditions (Sheldrake et al., 1978). Interestingly, more flowers do not directly mean more pods, and triple-flower plants can only develop two pods per I2, because one of the three flowers, with a different morphology, does not set pod (Srinivasan et al., 2006). In the same way, multi-flower plants can produce up to nine flowers per node but do not form more than four or five pods per I2 (Gaur and Gour, 2002; Srinivasan et al., 2006).
Number of I2 Nodes in the Primary Inflorescence, Activity of the I1 Meristem
The flowering time genes SN and HR not only control the activity of the I2 meristem in pea, but also affect the duration of I1 meristem activity, since the number of I2 nodes produced before I1 meristem arrest is decreased by recessive sn alleles and increased by dominant HR alleles (Reid et al., 1996). Interestingly, some studies suggested that duration of I1 meristem activity could be uncoupled from flowering time (Reid, 1980).
In some cases, the number of I2 (flowering) nodes has been found to limit yield in legume crops (Roche et al., 1998; Kahlon et al., 2011), which indicates that the number of flowering nodes is a trait with the potential to improve yield. However, genes that specifically control the number of I2 nodes produced by the I1 meristem have not been identified so far.
Inflorescence Traits Amenable to Improvement in Legume Crops
Inflorescence traits amenable to improvement in legumes could be divided into two categories: (1) traits related to the identity of the meristems in the inflorescence apex, and (2) traits related to the activity of the inflorescence meristems. In this section, we discuss genetic and/or biotechnological strategies to modify inflorescence traits that might be applicable in breeding programs either to synchronize maturity facilitating mechanical harvesting or to improve and stabilize yields.
Traits Related to Inflorescence Meristem Identity
At least two main meristem-identity related traits might be amenable to improvement: determinate growth habit and inhibition of flowering (Figure 4).
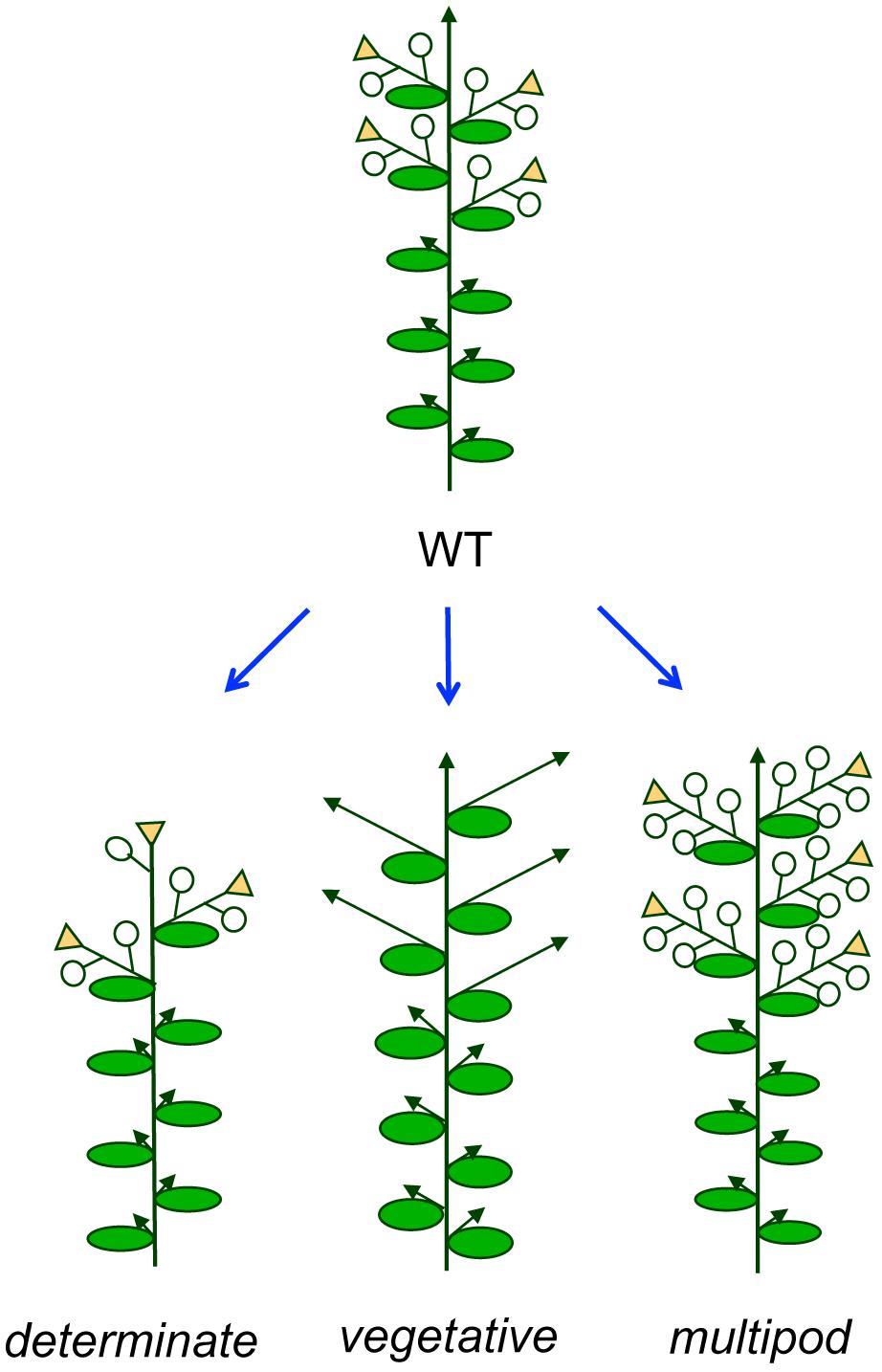
FIGURE 4. Possible modifications of the pea inflorescence architecture. Different plant architectures deriving from modifications of the inflorescence, with potential to improve crop performance in legumes. Open circles represent flowers and arrows represent indeterminate shoots.
Determinate Cultivars
As mentioned before, while wild accessions of most grain legumes have an indeterminate growth habit, where the main (primary) inflorescence meristem continues growing and producing lateral (secondary) inflorescences until its senescence, in many legume crop species determinate varieties exist, in which the growth of the primary inflorescence meristem is interrupted, soon after onset of flowering, by the production of a terminal inflorescence (Figures 3 and 4; Singer et al., 1990; Tian et al., 2010; Kwak et al., 2012). Determinate growth habit leads to a reduction in the flowering period, which can be beneficial under certain growing conditions. Thus, under rain-fed conditions the coincidence of growth duration of crop varieties to soil–moisture–availability is essential to reach high seed yields (Siddique et al., 2003) or to avoid lodging in some crops (Duc et al., 2014). Also, determinate cultivars are sometimes preferred because this growth habit facilitates mechanical harvesting (Kelly, 2001; Boote et al., 2003). As described above, recessive mutations in homologs of the Arabidopsis TFL1 gene underlie this determinate trait, at least in the grain legume crops where the genetic basis of the phenotype has been elucidated (Foucher et al., 2003; Avila et al., 2007; Liu et al., 2010; Repinski et al., 2012; Dhanasekar and Reddy, 2014).
In several legume crops, cultivars with semideterminate growth habit exist, where cessation of growth of the main inflorescence shoot occurs later than in determinate varieties. Advantages of these cultivars are that they produce a larger number of secondary inflorescences (and, therefore, pods) than determinate ones and, at the same time; they are less prone to lodging because they are shorter than indeterminate cultivars (Bernard, 1972; Kapoor and Gupta, 1991). In soybean, semideterminate growth habit has been linked to a dominant mutation leading to high expression in the apex of the main inflorescence of DT2, a homolog of the pea I2 meristem identity gene VEG1 (Ping et al., 2014).
According to what is known, it would seem that isolation of mutants in TFL1 homologs should be the most direct way to obtain determinate varieties of grain legume crop species. In contrast, it is not clear that a strategy to obtain semideterminate varieties through mutation will be possible because the causative mutation(s) of semideterminacy in soybean has not been fully elucidated yet, and also because they are gain-of-function mutations, most likely in regulatory regions of the Dt2 gene, which may be difficult to translate to other legume species (Ping et al., 2014). An alternative strategy that might allow obtaining semideterminate varieties in grain legumes would be the generation of plants overexpressing Dt2/VEG1.
Vegetative Non-Flowering Cultivars
In the case of forage legume crops, such as alfalfa and clovers, breeding is focused in the increase of total biomass (Hayes et al., 2013). An increase of the vegetative portion of the plant can be obtained through a delay in flowering or by inhibition of formation of secondary inflorescences (Figure 4). The genetic control of flowering time in legumes is discussed in another review in this number (see Weller and Ortega, 2015, in this Research Topic).
As described above, in the loss-of-function mutants in the VEG1 gene in pea, formation of secondary inflorescences (I2s) is inhibited, as these structures are transformed into vegetative branches (I1s) (Figure 3) and, consistent with that, pea plants where VEG1 was transiently silenced through virus-induced-silencing (VIGS) produced more vegetative nodes than the WT control (Berbel et al., 2012). As the function of VEG1 and other factors involved in the same genetic route, as GIGAS or VEG2, might be conserved in other grain legumes a possible strategy to inhibit flowering in forage legume species could be the isolation of mutants with reduced function of the homologs of these genes (Hecht et al., 2011; Berbel et al., 2012; Sussmilch et al., 2015).
On the other hand, and according to the proposed genetic model, the non-flowering phenotype of pea veg1 mutants appears to be caused by ectopic expression of the TFL1-homolog DET gene in all the meristems in the inflorescence apex, which transforms the branches produced in the “inflorescence” apex into primary inflorescences (I1s) and inhibit the formation of flowers (Hecht et al., 2011; Berbel et al., 2012). Therefore, an alternative strategy could be the overexpression of DET/TFL1 homologs that should reduce VEG1 activity and might result in inhibition or delay of flowering. This would represent a similar situation to the overexpression of TFL1 genes in Arabidopsis or tobacco, which leads to an extreme inhibition of flowering (Ratcliffe et al., 1998; Amaya et al., 1999).
Traits Related to the Activity of the Inflorescence Meristems
Multiflower/Multipod Pod Cultivars
The number of flowers per I2 (multipod/multiflower) is an inflorescence trait related to the activity of the inflorescence meristem that might be amenable to improvement in grain legumes (Figure 4). The possibility of increasing the number of pods appears an attractive option to increase yield in grain legumes. In this sense, in the case of chickpea it has been reported that the double-flower trait has the potential to increase yield (Kumar et al., 2000) or to have a positive effect on yield stability (Rubio et al., 2004). However, translation of this trait to grain legumes different from pea or chickpea is currently limited because the genes responsible of the multiflower/multipod trait have not been identified. Progress on the mapping of the chickpea SFL gene, responsible of the double- and triple-flower phenotypes, has been reported, which places SFL on LG6, (Rajesh et al., 2002; Gaur et al., 2011). To date, no linkage analysis has been reported for CYM, the chickpea gene responsible of the multi-flower phenotype.
Apart from the isolation of mutants for the multiflower/multipod genes, an alternative strategy that also might lead to an increased number of flowers per I2 node could be to manipulate the expression of genes that control general meristem activity. In Arabidopsis, a main determinant of shoot and inflorescence meristem activity is the WUSCHEL (WUS) gene, which codes for a homeobox-type transcription factor that induces stem-cell identity and is essential to maintain the population of stem cells in the meristems. WUS expression in the center of the meristems is directly correlated with an active state of stem cells within the meristem, and when WUS expression disappears meristems arrest. In wus loss-of-function mutants very few flowers are formed and these have a reduced number of floral organs (Mayer et al., 1998). Opposite phenotypes are observed when WUS expression is increased as, for instance, in clavata mutants that produce more flowers and these flowers have an increased number of floral organs (Schoof et al., 2000).
WUS homologs have been described in legumes and the available data suggest that they play the same function in meristem activity as in Arabidopsis (Wong et al., 2010). Therefore, it is conceivable that directing the expression of WUS to the I2 meristem, for example with the VEG1 promoter, might lead to an increased activity of the I2 meristem and, therefore to a higher production of flowers.
Perspectives for Legume Inflorescence Improvement, the Help of Genomics
As shown in the previous sections, we now have a rather good knowledge of the genetic networks that control major traits related to inflorescence architecture in legumes. In some legume species, particularly in pea, key regulators of inflorescence architecture have been isolated and functionally characterized and we can make rather reliable predictions of which of these genes should be used, and how, to improve inflorescence architecture in grain legumes for easier crop management and a higher and stable yield. Nonetheless, much work still needs to be done and genomics and related disciplines will be of great help to speed up progress in this area.
In addition to the sequenced genomes of the model species L. japonicus and M. truncatula (Sato et al., 2008; Young et al., 2011), genome sequencing has extended in the last years to crop legumes, mainly thanks to the advances in next generation sequencing (NGS) technologies. Thus, genome sequences are now available for soybean, chickpea, and pigeonpea (Schmutz et al., 2010; Varshney et al., 2012, 2013) and those of other important legume crops, such as pea, lentil or peanut are currently underway (Varshney et al., 2014a). The availability of genome sequences and other genomic resources should greatly facilitate the translation of basic knowledge obtained in a few legume models to the breeding of legume crops, and the reader is referred to recently published reviews on this subject for more details (Bolger et al., 2014; Varshney et al., 2014a,b).
Access to the genome sequence of crop legumes will allow easy identification and cloning of the homologs of inflorescence architecture regulators previously identified in model grain legumes such as pea or Medicago. Indeed, homologous genes of the major inflorescence regulators can be found in the sequence databases of several grain legumes (Table 1), therefore facilitating the identification of suitable alleles to breed inflorescence traits in the legume crop of interest.
Next generation sequencing technologies should also greatly ease the identification of candidate genes for architectural traits whose genetic basis remains unknown. The recently developed mapping-by-sequencing approaches are now starting to be routinely used to rapidly identify candidates for causal mutations in model organisms and, as the NGS technologies become more powerful and cheaper, their use is extending to crop species in spite of their usually larger genomes (Schneeberger et al., 2009; Abe et al., 2012; James et al., 2013). These methods bear great promise for the isolation of novel architecture-related genes identified from forward genetics or natural variation that eventually can be incorporated to breeding strategies.
In addition, different reverse genetic and genomic tools that can be used to validate the function of candidate genes for architectural traits are now available in several model and non-model legume species. First, mutant populations for the retrotransposons Tnt1, in M. truncatula and Lore1 in L. japonicus are now routinely used for identification of mutants for genes of interest through reverse genetics (Cheng et al., 2011; Urbański et al., 2012). Second, the virus induced gene silencing (VIGS) methods are available to several legume species such as pea, soybean, common bean, Latirus odorata and M. truncatula (Constantin et al., 2004; Grønlund et al., 2008; Zhang et al., 2010). VIGS is now allowing to successfully analyzing gene function in several legumes, including species that are recalcitrant to genetic transformation (Wang et al., 2008b; Liu et al., 2010). Finally, Targeting Induced Local Lesions In Genomes (TILLING) and EcoTILLING, which allow identification of point mutations and allelic variability in specific genes, can be applied to any species where mutagenised EMS-populations are available or to germplasm collections (Colbert et al., 2001; Comai et al., 2004; Tsai et al., 2011). TILLING platforms are effective reverse genetics tools that already have been proven highly successful for functional analysis of developmental regulators in legumes (Hofer et al., 2009; Berbel et al., 2012). These platforms are currently available for pea, M. truncatula, L. japonicus and chickpea (Perry et al., 2003; Dalmais et al., 2008; Le Signor et al., 2009; Varshney et al., 2014a), and will likely be developed for other grain legumes as well, providing a rich source of allelic variation for breeding purposes with potential to be used in virtually any diploid crop legume.
Finally, “designer” alleles also appear to be within reach through the recently developed genome editing techniques mediated by the CRISPR-Cas system. CRISPR has been showed to efficiently work in several plants like Arabidopsis and rice, where it was possible to engineer site-directed mutations in the genes of interest (Li et al., 2013; Shan et al., 2013; Lozano-Juste and Cutler, 2014). However, while extremely powerful, the use of CRISPR mutagenesis is currently limited to genetically transformable species.
In summary, the increasing availability of genomic tools and resources offers a unique opportunity to accelerate breeding of inflorescence architecture and, in general, of agronomic important traits in legumes. The combination of the increased understanding of the genetic networks controlling legume inflorescence architecture and the use of these genomic tools and resources, promises a rapid progress in obtention of new legume varieties with improved performance, which will be instrumental in developing a sustainable agriculture for the future.
Conflict of Interest Statement
The authors declare that the research was conducted in the absence of any commercial or financial relationships that could be construed as a potential conflict of interest.
Acknowledgments
We thank Cristina Ferrándiz for critical reading and help preparing the figures. We also apologize to those authors whose work we have inadvertently omitted, or could not review at length due to space limitations. Research in the Madueño lab is supported by grants form the Spanish Ministerio de Economía y Competitividad (BFU2012-38929), the Generalitat Valenciana (ACOMP/2014/109) and the EU (LEGATO project, GA n°FP7-613551) and in the Millán lab by the INIA (RTA2013-00025, co-financed by the EU, ERDF 2014-2020). RB is supported by a postdoctoral IE Marie-Curie Fellowship (FP7-PEOPLE-2011-IEF-299639-Molecular Clock) and LA by a Ph.D. fellowship from Syrian Ministry of High Education.
References
Abe, A., Kosugi, S., Yoshida, K., Natsume, S., Takagi, H., Kanzaki, H., et al. (2012). Genome sequencing reveals agronomically important loci in rice using MutMap. Nat. Biotechnol. 30, 174–178. doi: 10.1038/nbt.2095
Abe, M., Kobayashi, Y., Yamamoto, S., Daimon, Y., Yamaguchi, A., Ikeda, Y., et al. (2005). FD, a bZIP protein mediating signals from the floral pathway integrator FT at the shoot apex. Science 309, 1052–1056. doi: 10.1126/science.1115983
Alvarez, J., Guli, C. L., Yu, X. H., and Smyth, D. R. (1992). terminal flower: a gene affecting inflorescence development in Arabidopsis thaliana. Plant J. 2, 103–116. doi: 10.1111/j.1365-313X.1992.00103.x
Amaya, I., Ratcliffe, O. J., and Bradley, D. J. (1999). Expression of CENTRORADIALIS (CEN) and CEN-like genes in tobacco reveals a conserved mechanism controlling phase change in diverse species. Plant Cell 11, 1405–1418. doi: 10.1105/tpc.11.8.1405
Annicchiarico, P., and Iannucci, A. (2008). Adaptation strategy, germplasm type and adaptive traits for field pea improvement in Italy based on variety responses across climatically contrasting environments. Field Crops Res. 108, 133–142. doi: 10.1016/j.fcr.2008.04.004
Avila, C. M., Atienza, S. G., Moreno, M. T., and Torres, A. M. (2007). Development of a new diagnostic marker for growth habit selection in faba bean (Vicia faba L.) breeding. Theor. Appl. Gen. 115, 1075–1082. doi: 10.1007/s00122-007-0633-y
Benlloch, R., Berbel, A., Serrano-Mislata, A., and Madueo, F. (2007). Floral initiation and inflorescence architecture: a comparative view. Ann. Bot. 100, 659–676. doi: 10.1093/aob/mcm146
Benlloch, R., D’erfurth, I., Ferrandiz, C., Cosson, V., Beltran, J. P., Cañas, L. A., et al. (2006). Isolation of mtpim proves Tnt1 a useful reverse genetics tool in Medicago truncatula and uncovers new aspects of AP1-like functions in legumes. Plant Physiol. 142, 972–983. doi: 10.1104/pp.106.083543
Berbel, A., Ferrandiz, C., Hecht, V., Dalmais, M., Lund, O. S., Sussmilch, F. C., et al. (2012). VEGETATIVE1 is essential for development of the compound inflorescence in pea. Nat. Commun. 3:797. doi: 10.1038/ncomms1801
Berbel, A., Navarro, C., Ferrandiz, C., Cañas, L. A., Madueño, F., and Beltran, J. P. (2001). Analysis of PEAM4, the pea AP1 functional homolog, supports a model for AP1-like genes controlling both floral meristem and floral organ identity in different plant species. Plant J. 25, 441–451. doi: 10.1046/j.1365-313x.2001.00974.x
Bernard, R. L. (1972). Two Genes affecting stem termination in soybeans. Crop Sci. 12, 235–239. doi: 10.2135/cropsci1972.0011183X001200020028x
Beveridge, C. A., and Murfet, I. C. (1996). The gigas mutant in pea is deficient in the floral stimulus. Physiol. Plant. 96, 637–645. doi: 10.1111/j.1399-3054.1996.tb00237.x
Blazquez, M. A., Ferrandiz, C., Madueño, F., and Parcy, F. (2006). How floral meristems are built. Plant Mol. Biol. 60, 855–870. doi: 10.1007/s11103-006-0013-z
Bolger, M. E., Weisshaar, B., Scholz, U., Stein, N., Usadel, B., and Mayer, K. F. (2014). Plant genome sequencing — applications for crop improvement. Curr. Opin. Biotechnol. 26, 31–37. doi: 10.1016/j.copbio.2013.08.019
Boote, K. J., Jones, J. W., Batchelor, W. D., Nafziger, E. D., and Myers, O. (2003). Genetic coefficients in the CROPGRO–soybean model. Agron. J. 95, 32–51. doi: 10.2134/agronj2003.0032
Bowman, J. L., Alvarez, J., Weigel, D., Meyerowitz, E. M., and Smyth, D. R. (1993). Control of flower development in Arabidopsis thaliana by APETALA1 and interacting genes. Development 119, 721–743.
Bradley, D., Ratcliffe, O., Vincent, C., Carpenter, R., and Coen, E. (1997). Inflorescence commitment and architecture in Arabidopsis. Science 275, 80–83. doi: 10.1126/science.275.5296.80
Cheng, X., Wen, J., Tadege, M., Ratet, P., and Mysore, K. S. (2011). Reverse genetics in Medicago truncatula using Tnt1 insertion mutants. Methods Mol. Biol. 678, 179–190. doi: 10.1007/978-1-60761-682-5_13
Chi, Y., Huang, F., Liu, H., Yang, S., and Yu, D. (2011). An APETALA1-like gene of soybean regulates flowering time and specifies floral organs. J. Plant Physiol. 168, 2251–2259. doi: 10.1016/j.jplph.2011.08.007
Colbert, T., Till, B. J., Tompa, R., Reynolds, S., Steine, M. N., Yeung, A. T., et al. (2001). High-throughput screening for induced point mutations. Plant Physiol. 126, 480–484. doi: 10.1104/pp.126.2.480
Comai, L., Young, K., Till, B. J., Reynolds, S. H., Greene, E. A., Codomo, C. A., et al. (2004). Efficient discovery of DNA polymorphisms in natural populations by Ecotilling. Plant J. 37, 778–786. doi: 10.1111/j.0960-7412.2003.01999.x
Constantin, G. D., Krath, B. N., Macfarlane, S. A., Nicolaisen, M., Elisabeth Johansen, I., and Lund, O. S. (2004). Virus-induced gene silencing as a tool for functional genomics in a legume species. Plant J. 40, 622–631. doi: 10.1111/j.1365-313X.2004.02233.x
Dalmais, M., Schmidt, J., Le Signor, C., Moussy, F., Burstin, J., Savois, V., et al. (2008). UTILLdb, a Pisum sativum in silico forward and reverse genetics tool. Genome Biol. 9, R43. doi: 10.1186/gb-2008-9-2-r43
Dhanasekar, P., and Reddy, K. S. (2014). A novel mutation in TFL1 homolog affecting determinacy in cowpea (Vigna unguiculata). Mol. Genet. Genomics 290, 55–65. doi: 10.1007/s00438-014-0899-0
Dong, Z.-C., Zhao, Z., Liu, C.-W., Luo, J.-H., Yang, J., Huang, W.-H., et al. (2005). Floral patterning in Lotus japonicus. Plant Physiol. 137, 1272–1282. doi: 10.1104/pp.104.054288
Duc, G., Agrama, H., Bao, S., Berger, J., Bourion, V., De Ron, A. M., et al. (2014). Breeding annual grain legumes for sustainable agriculture: new methods to approach complex traits and target new cultivar ideotypes. Crit. Rev. Plant Sci. 34, 381–411. doi: 10.1080/07352689.2014.898469
Foucher, F., Morin, J., Courtiade, J., Cadioux, S., Ellis, N., Banfield, M. J., et al. (2003). Determinate and late flowering are two terminal flower1/centroradialis homologs that control two distinct phases of flowering initiation and development in Pea. Plant Cell 15, 2742–2754. doi: 10.1105/tpc.015701
Gaur, P. M., and Gour, V. K. (2002). A gene producing one to nine flowers per flowering node in chickpea. Euphytica 128, 231–235. doi: 10.1023/A:1020845815319
Gaur, R., Sethy, N., Choudhary, S., Shokeen, B., Gupta, V., and Bhatia, S. (2011). Advancing the STMS genomic resources for defining new locations on the intraspecific genetic linkage map of chickpea (Cicer arietinum L.). BMC Genom. 12:117. doi: 10.1186/1471-2164-12-117
Gottschalk, W. (1979). A Pisum gene preventing transition from the vegetative to the reproductive stage. Pisum Newslett. 11:11.
Gourlay, C. W., Hofer, J. M., and Ellis, T. H. (2000). Pea compound leaf architecture is regulated by interactions among the genes UNIFOLIATA, cochleata, afila, and tendril-lessn. Plant Cell 12, 1279–1294. doi: 10.1105/tpc.12.8.1279
Grønlund, M., Constantin, G., Piednoir, E., Kovacev, J., Johansen, I. E., and Lund, O. S. (2008). Virus-induced gene silencing in Medicago truncatula and Lathyrus odorata. Virus Res. 135, 345–349. doi: 10.1016/j.virusres.2008.04.005
Guo, X., Zhao, Z., Chen, J., Hu, X., and Luo, D. (2006). A putative CENTRORADIALIS/TERMINAL FLOWER 1-like gene, Ljcen1, plays a role in phase transition in Lotus japonicus. J. Plant Physiol. 163, 436–444. doi: 10.1016/j.jplph.2005.04.037
Hayes, B. J., Cogan, N. O. I., Pembleton, L. W., Goddard, M. E., Wang, J., Spangenberg, G. C., et al. (2013). Prospects for genomic selection in forage plant species. Plant Breed. 132, 133–143. doi: 10.1111/pbr.12037
Hecht, V., Laurie, R. E., Vander Schoor, J. K., Ridge, S., Knowles, C. L., Liew, L. C., et al. (2011). The pea GIGAS gene is a FLOWERING LOCUS T homolog necessary for graft-ransmissible specification of flowering but not for responsiveness to photoperiod. Plant Cell 23, 147–161. doi: 10.1105/tpc.110.081042
Hofer, J. M. I., and Noel Ellis, T. H. (2014). Developmental specialisations in the legume family. Curr. Opin. Plant Biol. 17, 153–158. doi: 10.1016/j.pbi.2013.11.014
Hofer, J., Turner, L., Hellens, R., Ambrose, M., Matthews, P., Michael, A., et al. (1997). UNIFOLIATA regulates leaf and flower morphogenesis in pea. Curr. Biol. 7, 581–587. doi: 10.1016/S0960-9822(06)00257-0
Hofer, J., Turner, L., Moreau, C., Ambrose, M., Isaac, P., Butcher, S., et al. (2009). Tendril-less regulates tendril formation in pea leaves. Plant Cell 21, 420–428. doi: 10.1105/tpc.108.064071
Hole, C. C., and Hardwick, R. C. (1976). Development and control of the number of flowers per node in Pisum sativum L. Ann. Bot. 40, 707–722.
Huala, E., and Sussex, I. M. (1992). LEAFY interacts with floral homeotic genes to regulate Arabidopsis floral development. Plant Cell 4, 901–913. doi: 10.1105/tpc.4.8.901
Irish, V. F., and Sussex, I. M. (1990). Function of the apetala-1 gene during Arabidopsis floral development. Plant Cell 2, 741–753. doi: 10.1105/tpc.2.8.741
James, G. V., Patel, V., Nordström, K. J., Klasen, J. R., Salomé, P. A., Weigel, D., et al. (2013). User guide for mapping-by-sequencing in Arabidopsis. Genome Biol. 14, R61. doi: 10.1186/gb-2013-14-6-r61
Kahlon, C. S., Board, J. E., and Kang, M. S. (2011). An analysis of yield component changes for new vs. old soybean cultivars. Agron. J. 103, 13–22. doi: 10.2134/agronj2010.0300
Kapoor, R. K., and Gupta, S. C. (1991). Inheritance of growth habit in pigeonpea. Crop Sci. 31, 1456–1459. doi: 10.2135/cropsci1991.0011183X003100060012x
Kaufmann, K., Wellmer, F., Muiño, J. M., Ferrier, T., Wuest, S. E., Kumar, V., et al. (2010). Orchestration of floral initiation by APETALA1. Science 328, 85–89. doi: 10.1126/science.1185244
Kellogg, E. A. (2007). Floral displays: genetic control of grass inflorescences. Curr. Opin. Plant Biol. 10, 26–31. doi: 10.1016/j.pbi.2006.11.009
Kelly, J. D. (2001). Remaking bean plant architecture for efficient production. Adav. Agron. 71, 109–143. doi: 10.1016/S0065-2113(01)71013-9
Kempin, S. A., Savidge, B., and Yanofsky, M. F. (1995). Molecular basis of the cauliflower phenotype in Arabidopsis. Science 267, 522–525. doi: 10.1126/science.7824951
Knights, E. (1987). The double-podded gene in chickpea improvement. Int. Chickpea Newslett. 17, 6–7.
Kong, F., Liu, B., Xia, Z., Sato, S., Kim, B. M., Watanabe, S., et al. (2010). Two coordinately regulated homologs of FLOWERING LOCUS T are involved in the control of photoperiodic flowering in soybean. Plant Physiol. 154, 1220–1231. doi: 10.1104/pp.110.160796
Kumar, J., Srivastava, R. K., and Ganesh, M. (2000). Penetrance and expressivity of the gene for double podding in chickpea. J. Heredity 91, 234–236. doi: 10.1093/jhered/91.3.234
Kwak, M., Toro, O., Debouck, D. G., and Gepts, P. (2012). Multiple origins of the determinate growth habit in domesticated common bean (Phaseolus vulgaris). Ann. Bot. 110, 1573–1580. doi: 10.1093/aob/mcs207
Lamprecht, H. (1947). The inheritance of the number of flowers per inflorescence and the origin of Pisum, illustrated by polymeric genes. Agri. Hort Genetica 5, 16–25.
Laurie, R. E., Diwadkar, P., Jaudal, M., Zhang, L., Hecht, V., Wen, J., et al. (2011). The medicago FLOWERING LOCUS T homolog, MtFTa1, is a key regulator of flowering time. Plant Physiol. 156, 2207–2224. doi: 10.1104/pp.111.180182
Le Signor, C., Savois, V., Aubert, G., Verdier, J., Nicolas, M., Pagny, G., et al. (2009). Optimizing TILLING populations for reverse genetics in Medicago truncatula. Plant Biotechnol. J. 7, 430–441. doi: 10.1111/j.1467-7652.2009.00410.x
Li, J.-F., Norville, J. E., Aach, J., Mccormack, M., Zhang, D., Bush, J., et al. (2013). Multiplex and homologous recombination-mediated genome editing in Arabidopsis and Nicotiana benthamiana using guide RNA and Cas9. Nat. Biotechnol. 31, 688–691. doi: 10.1038/nbt.2654
Liew, L. C., Hecht, V., Sussmilch, F. C., and Weller, J. L. (2014). The pea photoperiod response gene STERILE NODES Is an ortholog of LUX ARRHYTHMO. Plant Physiol. 165, 648–657. doi: 10.1104/pp.114.237008
Liljegren, S. J., Gustafson-Brown, C., Pinyopich, A., Ditta, G. S., and Yanofsky, M. F. (1999). Interactions among APETALA1, LEAFY, and TERMINAL FLOWER1 specify meristem fate. Plant Cell 11, 1007–1018. doi: 10.1105/tpc.11.6.1007
Lippman, Z. B., Cohen, O., Alvarez, J. P., Abu-Abied, M., Pekker, I., Paran, I., et al. (2008). The making of a compound inflorescence in tomato and related nightshades. PLoS Biol. 6:e288. doi: 10.1371/journal.pbio.0060288
Liu, B., Watanabe, S., Uchiyama, T., Kong, F., Kanazawa, A., Xia, Z., et al. (2010). The soybean stem growth habit gene Dt1 is an ortholog of arabidopsis TERMINAL FLOWER1. Plant Physiol. 153, 198–210. doi: 10.1104/pp.109.150607
Lozano-Juste, J., and Cutler, S. R. (2014). Plant genome engineering in full bloom. Trends Plant Sci. 19, 284–287. doi: 10.1016/j.tplants.2014.02.014
Maizel, A., Busch, M. A., Tanahashi, T., Perkovic, J., Kato, M., Hasebe, M., et al. (2005). The floral regulator LEAFY evolves by substitutions in the DNA binding domain. Science 308, 260–263. doi: 10.1126/science.1108229
Mandel, M. A., Gustafson-Brown, C., Savidge, B., and Yanofsky, M. F. (1992). Molecular characterization of the Arabidopsis floral homeotic gene APETALA1. Nature 360, 273–277. doi: 10.1038/360273a0
Mandel, M. A., and Yanofsky, M. F. (1995). A gene triggering flower formation in Arabidopsis. Nature 377, 522–524. doi: 10.1038/377522a0
Mayer, K. F., Schoof, H., Haecker, A., Lenhard, M., Jürgens, G., and Laux, T. (1998). Role of WUSCHEL in regulating stem cell fate in the Arabidopsis shoot meristem. Cell 95, 805–815. doi: 10.1016/S0092-8674(00)81703-1
Mir, R. R., Kudapa, H., Srikanth, S., Saxena, R. K., Sharma, A., Azam, S., et al. (2014). Candidate gene analysis for determinacy in pigeonpea (Cajanus spp.). Theor. Appl. Gen. 127, 2663–2678. doi: 10.1007/s00122-014-2406-8
Murfet, I. C., and Reid, J. B. (1993). “Developmental mutants,” in Peas: Genetics, Molecular Biology And Biotechnology eds R. Casey and D. R. Davies (Wallinford: CAB International), 165–216.
Ohshima, S., Murata, M., Sakamoto, W., Ogura, Y., and Motoyoshi, F. (1997). Cloning and molecular analysis of the Arabidopsis gene terminal flower 1. Mol. Gen. Gen. 254, 186–194. doi: 10.1007/s004380050407
Ono, N., Ishida, K., Yamashino, T., Nakanishi, H., Sato, S., Tabata, S., et al. (2010). Genomewide characterization of the light-responsive and clock-controlled output pathways in Lotus japonicus with special emphasis of its uniqueness. Plant Cell Physiol. 51, 1800–1814. doi: 10.1093/pcp/pcq140
Parcy, F., Nilsson, O., Busch, M. A., Lee, I., and Weigel, D. (1998). A genetic framework for floral patterning. Nature 395, 561–566. doi: 10.1038/26903
Park, S. J., Jiang, K., Tal, L., Yichie, Y., Gar, O., Zamir, D., et al. (2014). Optimization of crop productivity in tomato using induced mutations in the florigen pathway. Nat. Genet. 46, 1337–1342. doi: 10.1038/ng.3131
Perry, J. A., Wang, T. L., Welham, T. J., Gardner, S., Pike, J. M., Yoshida, S., et al. (2003). A TILLING reverse genetics tool and a web-accessible collection of mutants of the legume Lotus japonicus. Plant Physiol. 131, 866–871. doi: 10.1104/pp.102.017384
Pin, P. A., and Nilsson, O. (2012). The multifaceted roles of FLOWERING LOCUS T in plant development. Plant Cell Environ. 35, 1742–1755. doi: 10.1111/j.1365-3040.2012.02558.x
Ping, J., Liu, Y., Sun, L., Zhao, M., Li, Y., She, M., et al. (2014). Dt2 Is a gain-of-function MADS-domain factor gene that specifies semideterminacy in soybean. Plant Cell. 26, 2831–2842. doi: 10.1105/tpc.114.126938
Prenner, G. (2013). Papilionoid inflorescences revisited (Leguminosae-Papilionoideae). Ann. Bot. 112, 1567–1576. doi: 10.1093/aob/mcs258
Prusinkiewicz, P., Erasmus, Y., Lane, B., Harder, L. D., and Coen, E. (2007). Evolution and development of inflorescence architectures. Science 316, 1452–1456. doi: 10.1126/science.1140429
Rajesh, P., Tullu, A., Gil, J., Gupta, V., Ranjekar, P., and Muehlbauer, F. (2002). Identification of an STMS marker for the double-podding gene in chickpea. Theor. Appl. Genet. 105, 604–607. doi: 10.1007/s00122-002-0930-4
Ratcliffe, O. J., Amaya, I., Vincent, C. A., Rothstein, S., Carpenter, R., Coen, E. S., et al. (1998). A common mechanism controls the life cycle and architecture of plants. Development 125, 1609–1615.
Ratcliffe, O. J., Bradley, D. J., and Coen, E. S. (1999). Separation of shoot and floral identity in Arabidopsis. Development 126, 1109–1120.
Reid, J. B. (1980). Apical senescence in Pisum: a direct or indirect role for the flowering genes ? Ann. Bot. 45, 195–201.
Reid, J. B., and Murfet, I. C. (1984). Flowering in Pisum: a fifth locus, veg. Ann. Bot. 53, 369–382.
Reid, J. B., Murfet, I. C., Singer, S. R., Weller, J. L., and Taylor, S. A. (1996). Physiological-genetics of flowering in Pisum. Semin. Cell Dev. Biol. 7, 455–463. doi: 10.1006/scdb.1996.0057
Repinski, S. L., Kwak, M., and Gepts, P. (2012). The common bean growth habit gene PvTFL1y is a functional homolog of Arabidopsis TFL1. Theor. Appl. Gen. 124, 1539–1547. doi: 10.1007/s00122-012-1808-8
Roche, R., Jeuffroy, M., and Ney, B. (1998). A model to simulate the final number of reproductive nodes in pea (Pisum sativum L.). Ann. Bot. 81, 545–555. doi: 10.1006/anbo.1998.0592
Rubio, J., Flores, F., Moreno, M. T., Cubero, J. I., and Gil, J. (2004). Effects of the erect/bushy habit, single/double pod and late/early flowering genes on yield and seed size and their stability in chickpea. Field Crops Res. 90, 255–262. doi: 10.1016/j.fcr.2004.03.005
Sato, S., Nakamura, Y., Kaneko, T., Asamizu, E., Kato, T., Nakao, M., et al. (2008). Genome structure of the legume, Lotus japonicus. DNA Res. 15, 227–239. doi: 10.1093/dnares/dsn008
Schmutz, J., Cannon, S. B., Schlueter, J., Ma, J., Mitros, T., Nelson, W., et al. (2010). Genome sequence of the palaeopolyploid soybean. Nature 463, 178–183. doi: 10.1038/nature08670
Schneeberger, K., Ossowski, S., Lanz, C., Juul, T., Petersen, A. H., Nielsen, K. L., et al. (2009). SHOREmap: simultaneous mapping and mutation identification by deep sequencing. Nat. Methods 6, 550–551. doi: 10.1038/nmeth0809-550
Schoof, H., Lenhard, M., Haecker, A., Mayer, K. F., Jürgens, G., and Laux, T. (2000). The stem cell population of Arabidopsis shoot meristems in maintained by a regulatory loop between the CLAVATA and WUSCHEL genes. Cell 1006, 635–644. doi: 10.1016/S0092-8674(00)80700-X
Schultz, E. A., and Haughn, G. W. (1991). LEAFY, a homeotic gene that regulates inflorescence development in Arabidopsis. Plant Cell 3, 771–781. doi: 10.1105/tpc.3.8.771
Schultz, E. A., and Haughn, G. W. (1993). Genetic analysis of the floral initiation process (FLIP) in Arabidopsis. Development 119, 745–765.
Shan, Q., Wang, Y., Li, J., Zhang, Y., Chen, K., Liang, Z., et al. (2013). Targeted genome modification of crop plants using a CRISPR-Cas system. Nat. Biotechnol. 31, 686–688. doi: 10.1038/nbt.2650
Shannon, S., and Meeks-Wagner, D. R. (1991). A mutation in the Arabidopsis TFL1 gene affects inflorescence meristem development. Plant Cell 3, 877–892. doi: 10.1105/tpc.3.9.877
Shannon, S., and Meeks-Wagner, D. R. (1993). Genetic interactions that regulate inflorescence development in Arabidopsis. Plant Cell 5, 639–655. doi: 10.1105/tpc.5.6.639
Sheldrake, A. R., Saxena, N. P., and Krishnamurthy, L. (1978). The expression and influence on yield of the ‘double-podded’ character in chickpeas (Cicer arietinum L.). Field Crops Res. 1, 243–253. doi: 10.1016/0378-4290(78)90029-1
Siddique, K. H. M., Loss, S. P., and Thomson, B. D. (2003). “Cool season grain legumes in dryland Mediterranean environments of Western Australia: significance of early flowering,” in Management of Agricultural Drought, ed. N. Saxena (Enfield, NH: Enfield Publishers), 151–161.
Singer, S. R., Hsiung, L. P., and Huber, S. C. (1990). Determinate (det) mutant of Pisum sativum (Leguminosae: Papilionoideae) exhibits an indeterminate growth pattern. Am. J. Bot. 77, 1330–1335. doi: 10.2307/2444593
Singer, S., Sollinger, J., Maki, S., Fishbach, J., Short, B., Reinke, C., et al. (1999). Inflorescence architecture: a developmental genetics approach. Bot. Rev. 65, 385–410. doi: 10.1007/BF02857756
Singh, K., and Chaturvedi, S. (1998). Genetics of triple floweredness in chickpea. Indian J. Pulses Res. 11, 15–17.
Smýkal, P., Coyne, C. J., Ambrose, M. J., Maxted, N., Schaefer, H., Blair, M. W., et al. (2014). Legume crops phylogeny and genetic diversity for science and breeding. Crit. Rev. Plant Sci. 34, 43–104. doi: 10.1080/07352689.2014.897904
Srinivasan, S., Gaur, P. M., Chaturvedi, S. K., and Rao, B. V. (2006). Allelic relationships of genes controlling number of flowers per axis in chickpea. Euphytica 152, 331–337. doi: 10.1007/s10681-006-9219-z
Steeves, T. A., and Sussex, I. M. (1989). Patterns in Plant Development. Cambridge: Cambridge University Press. doi: 10.1017/CBO9780511626227
Sussmilch, F. C., Ana Berbel, A., Hecht, V., Vander Schoor, J. K., Ferrándiz, C., Madueño, F., et al. (2015). The pea VEGETATIVE2 gene is an FD homolog that is essential for flowering and compound inflorescence development. Plant Cell 27, 1046–1060. doi: 10.1105/tpc.115.136150
Taylor, S. A., Hofer, J. M. I., Murfet, I. C., Sollinger, J. D., Singer, S. R., Knox, M. R., et al. (2002). PROLIFERATING INFLORESCENCE MERISTEM, a MADS-Box Gene that regulates floral meristem identity in pea. Plant Physiol. 129, 1150–1159. doi: 10.1104/pp.001677
Tian, Z., Wang, X., Lee, R., Li, Y., Specht, J. E., Nelson, R. L., et al. (2010). Artificial selection for determinate growth habit in soybean. Proc. Natl. Acad. Sci. U.S.A. 107, 8563–8568. doi: 10.1073/pnas.1000088107
Tsai, H., Howell, T., Nitcher, R., Missirian, V., Watson, B., Ngo, K. J., et al. (2011). Discovery of rare mutations in populations: TILLING by sequencing. Plant Physiol. 156, 1257–1268. doi: 10.1104/pp.110.169748
Urbański, D. F., Małolepszy, A., Stougaard, J., and Andersen, S. U. (2012). Genome-wide LORE1 retrotransposon mutagenesis and high-throughput insertion detection in Lotus japonicus. Plant J. 69, 731–741. doi: 10.1111/j.1365-313X.2011.04827.x
Varshney, R. K., Chen, W., Li, Y., Bharti, A. K., Saxena, R. K., Schlueter, J. A., et al. (2012). Draft genome sequence of pigeonpea (Cajanus cajan), an orphan legume crop of resource-poor farmers. Nat. Biotechnol. 30, 83–89. doi: 10.1038/nbt.2022
Varshney, R. K., Kudapa, H., Pazhamala, L., Chitikineni, A., Thudi, M., Bohra, A., et al. (2014a). Translational genomics in agriculture: some examples in grain legumes. Crit. Rev. Plant Sci. 34, 169–194. doi: 10.1080/07352689.2014.897909
Varshney, R. K., Terauchi, R., and Mccouch, S. R. (2014b). Harvesting the promising fruits of genomics: applying genome sequencing technologies to crop breeding. PLoS Biol. 12:e1001883. doi: 10.1371/journal.pbio.1001883
Varshney, R. K., Song, C., Saxena, R. K., Azam, S., Yu, S., Sharpe, A. G., et al. (2013). Draft genome sequence of chickpea (Cicer arietinum) provides a resource for trait improvement. Nat. Biotechnol. 31, 240–246. doi: 10.1038/nbt.2491
Wagner, D., Sablowski, R. W., and Meyerowitz, E. M. (1999). Transcriptional activation of APETALA1 by LEAFY. Science 285, 582–584. doi: 10.1126/science.285.5427.582
Wang, H., Chen, J., Wen, J., Tadege, M., Li, G., Liu, Y., et al. (2008a). Control of compound leaf development by FLORICAULA/LEAFY Ortholog SINGLE LEAFLET1 in medicago truncatula. Plant Physiol. 146, 1759–1772. doi: 10.1104/pp.108.117044
Wang, Z., Luo, Y., Li, X., Wang, L., Xu, S., Yang, J., et al. (2008b). Genetic control of floral zygomorphy in pea (Pisum sativum L.). Proc. Natl. Acad. Sci. U.S.A. 105, 10414–10419. doi: 10.1073/pnas.0803291105
Weberling, F. (1989a). Morphology of Flowers and Inflorescences. Trans. R. J. Pankhurst. Cambridge: Cambridge University. Press.
Weberling, F. (1989b). “Structure and evolutionary tendencies of inflorescences in the Leguminosae,” in Advances in Legume Biology, eds C. H. Stirton and J. L. Zarucchi (St. Louis, MO: Missouri Botanical Gardens), 35–58.
Weigel, D., Alvarez, J., Smyth, D. R., Yanofsky, M. F., and Meyerowitz, E. M. (1992). LEAFY controls floral meristem identity in Arabidopsis. Cell 69, 843–859. doi: 10.1016/0092-8674(92)90295-N
Weigel, D., and Meyerowitz, E. M. (1993). Activation of floral homeotic genes in Arabidopsis. Science 261, 1723–1726. doi: 10.1126/science.261.5129.1723
Weller, J. L., Liew, L. C., Hecht, V. F. G., Rajandran, V., Laurie, R. E., Ridge, S., et al. (2012). A conserved molecular basis for photoperiod adaptation in two temperate legumes. Proc. Natl. Acad. Sci. U.S.A. 109, 21158–21163. doi: 10.1073/pnas.1207943110
Weller, J. L., and Ortega, R. (2015). Genetic control of flowering time in legumes. Front. Plant Sci. 6:207. doi: 10.3389/fpls.2015.00207
White, O. E. (1917). Studies of inheritance in Pisum. II. The present state of knowledge of heredity and variation in pea. Proc. Am. Philos. Soc. 56, 487–588.
Wigge, P. A., Kim, M. C., Jaeger, K. E., Busch, W., Schmid, M., Lohmann, J. U., et al. (2005). Integration of spatial and temporal information during floral induction in Arabidopsis. Science 309, 1056–1059. doi: 10.1126/science.1114358
William, D. A., Su, Y., Smith, M. R., Lu, M., Baldwin, D. A., and Wagner, D. (2004). Genomic identification of direct target genes of LEAFY. Proc. Natl. Acad. Sci. U.S.A. 101, 1775–1780. doi: 10.1073/pnas.0307842100
Wong, C. E., Khor, S. Y., Bhalla, P. L., and Singh, M. B. (2010). Novel spatial expression of soybean WUSCHEL in the incipient floral primordia. Planta 233, 553–560. doi: 10.1007/s00425-010-1320-9
Young, N. D., Debellé, F., Oldroyd, G. E. D., Geurts, R., Cannon, S. B., Udvardi, M. K., et al. (2011). The Medicago genome provides insight into the evolution of rhizobial symbioses. Nature 480, 520–524. doi: 10.1038/nature10625
Keywords: legumes, pea, inflorescence architecture, meristem identity, AP1, TFL1, VEG1
Citation: Benlloch R, Berbel A, Ali L, Gohari G, Millán T and Madueño F (2015) Genetic control of inflorescence architecture in legumes. Front. Plant Sci. 6:543. doi: 10.3389/fpls.2015.00543
Received: 05 March 2015; Accepted: 06 July 2015;
Published: 21 July 2015.
Edited by:
Marta Santalla, Mision Biologica de Galicia, Consejo Superior de Investigaciones Cientificas, SpainReviewed by:
Matthew R. Willmann, University of Pennsylvania, USAElena M. Kramer, Harvard University, USA
Muriel Quinet, Université catholique de Louvain, Belgium
Copyright © 2015 Benlloch, Berbel, Ali, Gohari, Millán and Madueño. This is an open-access article distributed under the terms of the Creative Commons Attribution License (CC BY). The use, distribution or reproduction in other forums is permitted, provided the original author(s) or licensor are credited and that the original publication in this journal is cited, in accordance with accepted academic practice. No use, distribution or reproduction is permitted which does not comply with these terms.
*Correspondence: Francisco Madueño, Instituto de Biología Molecular y Celular de Plantas, Consejo Superior de Investigaciones Científicas – Universidad Politécnica de Valencia, Avenida Los Naranjos s/n, Valencia 46022, Spain, madueno@ibmcp.upv.es