- 1Plant Nutrition Department, Centro de Edafología y Biología Aplicada del Segura (CEBAS-CSIC), Murcia, Spain
- 2Food Science and Technology Department, Centro de Edafología y Biología Aplicada del Segura (CEBAS-CSIC), Murcia, Spain
- 3Department of Biosciences, CNR Biophysics Institute, University of Milan, Milano, Italy
Members of the Brassicaceae are known for their contents of nutrients and health-promoting phytochemicals, including glucosinolates. Exposure to salinity increases the levels of several of these compounds, but their role in abiotic stress response is unclear. The effect of aliphatic glucosinolates on plant water balance and growth under salt stress, involving aquaporins, was investigated by means of Arabidopsis thaliana mutants impaired in aliphatic glucosinolate biosynthesis, which is controlled by two transcription factors: Myb28 and Myb29. The double mutant myb28myb29, completely lacking aliphatic glucosinolates, was compared to wild type Col-0 (WT) and the single mutant myb28. A greater reduction in the hydraulic conductivity of myb28myb29 was observed under salt stress, when compared to the WT and myb28; this correlated with the abundance of both PIP1 and PIP2 aquaporin subfamilies. Also, changes in root architecture in response to salinity were genotype dependent. Treatment with NaCl altered glucosinolates biosynthesis in a similar way in WT and the single mutant and differently in the double mutant. The results indicate that short-chain aliphatic glucosinolates may contribute to water saving under salt stress.
Introduction
Glucosinolates are a group of plant secondary metabolites containing nitrogen and sulfur, mainly found in the Brassicaceae family (Zareba and Serradelf, 2004; Finley, 2005). Based on their side-chain structure and amino acid precursors, glucosinolates can be classified into aliphatic, aromatic, and indolic (Fahey et al., 2001). In Arabidopsis thaliana (Arabidopsis), nearly 40 different glucosinolates have been identified, mostly of the aliphatic or indolic type (Reichelt et al., 2002; Brown et al., 2003).
It has been reported that two MYB transcription factors, MYB28 and MYB29, were essential for aliphatic glucosinolate biosynthesis (Hirai et al., 2007; Gigolashvili et al., 2007b; Sonderby et al., 2007; Beekwilder et al., 2008; Malitsky et al., 2008). Thus, the levels of short-chain aliphatic glucosinolates are roughly halved in quantity in both the myb28 and myb29 single mutants in A. thaliana, but long-chain ones are absent in the myb28 mutant. The MYB29 gene plays an additional function in the methyl jasmonate-mediated induction of aliphatic glucosinolate biosynthetic genes (Hirai et al., 2007; Sonderby et al., 2007; Beekwilder et al., 2008; Gigolashvili et al., 2009).
The role of glucosinolates in plant defense against herbivorous insects is well established. After tissue damage, myrosinase activity hydrolyses glucosinolates, providing reactive compounds active against insects and pathogens (Beekwilder et al., 2008; Kim et al., 2008; Schlaeppi and Mauch, 2010). For instance, aliphatic glucosinolates play an important role in plant-herbivore interactions (Beekwilder et al., 2008; Redovnikovic et al., 2008) and in non-host resistance in the Arabidopsis-Pseudomonas system (Fan et al., 2011). However, in Arabidopsis, the suppression of aliphatic glucosinolates by RNA interference (RNAi) was also found to affect proteins and metabolites involved in photosynthesis, oxidative stress and hormone metabolism (Chen et al., 2012) as well as the circadian rhythms influences the expression of glucosinolates biosynthetic genes and other abiotic stress response-related genes (Rosa, 1997), highlighting the complexity of glucosinolate function in plants.
Thus, many abiotic factors have been reported to affect the glucosinolate profile and content (Schonhof et al., 2007; Haugen et al., 2008; Kuhlmann and Muller, 2009; Schreiner et al., 2009; Martínez-Ballesta et al., 2013), including salt stress—whose effects on glucosinolate accumulation have been investigated in Brassica species and Raphanus sativus (Qasim et al., 2003; Ashraf and Haris, 2004; Lopez-Berenguer et al., 2008; Yuan et al., 2010). However, little is known about the role of different glucosinolates - as well as their hydrolysis products, isothiocyanates—in abiotic stress responses. In this context, exogenous application of allyl-isothiocyanate induced stomatal closure in Arabidopsis through the inhibition of inward K+ channels present in the guard cells, allowing water conservation and preventing pathogen attack under water stress (Khokon et al., 2011; Li et al., 2014). Similarly, isothiocyanates induced heat tolerance in Arabidopsis plants by increasing the expression levels of heat shock proteins (HSP) (Hara et al., 2013). Administration of, sinigrin, an aliphatic glucosinolate, regulated water transport by increasing both movement through the symplastic water pathway and aquaporin abundance (Martínez-Ballesta et al., 2013).
The fact that moderate salt stress increases glucosinolate levels (Qasim et al., 2003; López-Berenguer et al., 2008; Keling and Zhujun, 2010) suggests that, under low water potential, these secondary metabolites could be an adaptive component of salt tolerance in members of the Brassicaceae family (Stroeher et al., 1995).
On the other hand, there are contrasting results concerning the relationship between glucosinolates biosynthesis and hormone-mediated plant growth and development. For instance, a mutation in the CYP83B1 (SUR2) and C-S lyase (SUR1) genes in A. thaliana, which are involved in the glucosinolates pathway, resulted in adventitious roots development—mediated by an increase in the levels of auxin (indole-3-acetic acid, IAA) (Smolen and Bender, 2002). Similarly, overexpression of the ATR1/MYB34 and MYB122 transcription factors led to increased levels of glucosinolates and high-IAA phenotypes. However, overexpression of HIG1/MYB51, another MYB family member involved in the regulation of the indole glucosinolate biosynthesis, did not produce any aberrant growth phenotypes (Gigolashvili et al., 2008).
We have explored the role of endogenous glucosinolate accumulation in the plant response to salinity, in relation to water uptake and transport, as well as plant morphological changes to cope with this stress. To this end we examined the phenotypic changes and glucosinolate profiles of the different genotypes as well as their ability to take up and transport water, the aquaporin abundance and modifications of root architecture under salt stress.
Materials and Methods
Plant Material and Growth Conditions
Plants of A. thaliana ecotype Col-0 wild type (WT), the myb28 single mutant and the myb28myb29 double mutant, previously described (Beekwilder et al., 2008), were used throughout this work. The single mutant myb28 line had reduced levels of short-chain aliphatic glucosinolates whereas long-chain aliphatic glucosinolates are absent. The single mutant myb29 line showed reduced accumulation of short-chain glucosinolates while the levels of long-chain are similar to the the wild type; however, it has been observed that there was no modification of the expression of glucosinolates biosynthetic genes by suppression of this transcription factor (Hirai et al., 2007), indicating that—rather than being essential for glucosinolates synthesis—myb29 participates in glucosinolates induction. The myb28myb29 double mutant knockouts decrease the accumulation of both short- and long-chain aliphatic glucosinolates to undetectable levels. In order to distinguish the effects of short- and long-chain glucosinolates on water relations under salinity, both the myb28 single and the double myb28myb29 mutants were employed in this work.
Seeds were surface-sterilized for 30 s in 70% ethanol, followed by 10% (v/v) commercial bleach for 10 min. They were then washed with sterilized water four times and suspended in sterile water at 4°C for 72 h. Plants (64) of each genotype and treatment were grown in hydroponic culture (Gibeaut et al., 1997) in a controlled-environment chamber (8h/16h day/night cycle, 150 mmol m−2 s−1 light, 22°C). The plants were grown in a modified, one-fifth-strength Hoagland solution with the following macronutrients (mM): 1.4 Ca(NO3)2, 0.35 MgSO4 and 0.1 Ca(H2PO4)2 and the following micronutrients (μM): 50 CaCl2, 12.5 H3BO3, 1 MnSO4, 1 ZnSO4, 0.5 CuSO4, 0.1 H2MoO4, 0.1 NiSO4, and 10 Fe-EDDHA. For each experiment, as indicated, K+ was added as KCl. The pH was adjusted daily to 5.5 and the solutions were renewed weekly.
The plants were allowed to grow for 4 weeks and then NaCl was added to the nutrient solution of half of the plants of each genotype, to give a final concentration of 100 mM. After 10 d, the plants were collected for analysis.
Analysis of Primary and Lateral Root Length and Leaf Area Determination
The primary root length and lateral roots (LRs) length were determined using an optical microscope system with a computer attachment (MPS 60, Leica, Wetzlar, Germany). Root lengths were measured on digital images using ImageJ 1.40 software (http://rsb.info.nih.gov/ij/), from the analysis of 20 plants. The experiments were performed at least twice, independently. Leaf area was determined using an area meter (model LI-3100C; Li-Cor, Lincoln, NE, USA).
Measurement of Fresh and Dry Weights and Relative Water Content
Twenty plants per treatment were chosen for the determination of fresh weight (FW), which was directly recorded with a portable balance (Scout Pro 400 g, Ohaus Corporation, NJ, USA). The plants were dried in an oven at 70°C until constant weight, to determine the dry weight (DW). In order to determine the leaf relative water content (RWC), five discs of 1-cm diameter were sampled. The fresh mass (Mf) of the discs was measured immediately; then, they were hydrated for 24 h at 4°C, in the dark, to determine the fully-turgid mass (Mt). After this, the discs were dried at 70°C, to obtain their dry mass (Md). The RWC was calculated as: (Mf - Md)/(Mt - Md).
Water Relations
The root hydraulic conductance (L0) of the Arabidopsis plants was measured by pressurizing the roots in a Scholander pressure chamber, as described in Javot et al. (2003). The aerial parts of the plant were removed and the freshly-excised roots were inserted into the pressure chamber, in a plastic tube with the same nutrient solution used for their growth. A gradual increase of pressure (from 0.1 to 0.4 MPa) was applied to the detached roots. The sap that accumulated in this pressure range during a certain time, according to the treatment, was collected in a graduated glass micropipette. The sap flow (Jv) was expressed in mg g−1 (root dry weight) h−1 and plotted against pressure (MPa), the slope being the L0 value in mg g−1 (root dry weight) h−1 MPa−1. The measurements were made in the middle of the photoperiod, 10 d after applying NaCl. Data were obtained from 12 measurements for each genotype.
Whole plant transpiration was determined by weight loss of each pot (sealed in a plastic tube at the base of the stem) in a 5-h daytime period, divided by the total leaf area at harvest, expressed in units of mg m−2 (leaf area) h−1.
Mineral Elements
The macronutrients (calcium, Ca; potassium, K; magnesium, Mg; sodium, Na; phosphorus, P; and sulfur, S) were analyzed in oven-dried leaf tissue (ca. 100 mg DW). The samples were digested, after HNO3-H2O2 (2:1) addition, in a microwave oven (CEM Mars Xpress, North Carolina, USA) and analyzed by ICP spectrometry (Iris Intrepid II, Thermo Electron Corporation, Franklin, USA). Also, the concentrations of C and N were determined in samples (ca. 2–3 mg DW) of leaves, using a CN analyzer (Thermo-Finnigan 1112 EA elemental analyzer; Thermo-Finnigan, Milan, Italy).
Extraction and Determination of Intact Glucosinolates and Phenolic Compounds
A standard procedure (Bennett et al., 2004) was used for the extraction of intact glucosinolates with modifications. For this, freeze-dried leaf powder (150 mg) was extracted with 1.5 mL of 70% methanol (MeOH); heated at 70°C for 30 min, shaking every 5 min with a vortex stirrer, followed by centrifugation (30 min, 13,000 g, 4°C) to pellet insoluble material. The supernatants were collected and the methanol was removed using a rotary evaporator; the dry material obtained was re-dissolved in the same volume of ultrapure water, as the original supernatant, and filtered through a 0.45-μm polyethersulphone filter (ANOTOP 10 plus; Whatman, Maidstone, UK). For glucosinolate analysis, each sample (20 μL) was analyzed by a Waters HPLC system (Waters Cromatografía S.A., Barcelona, Spain) consisting of a W600E multi-solvent delivery system, in-line degasser, W717plus Autosampler and W2996 Photodiode. The compounds were separated in a Luna C18 column (25 × 0.46 cm, 5 μm particle size; Phenomenex, Macclesfield, UK) with a security guard C18-ODS (4 × 30 mm) cartridge system (Phenomenex). The mobile phase was a mixture of water/formic acid (99:1 v/v) (A) and acetonitrile (B). Glucosinolates were eluted off the column in 35 min. The flow rate was 1 mL min−1 in a linear gradient, starting with 1% B and reaching 20% B in 30 min and 1% B in 40 min. Glucosinolates present in the samples were then identified using a previously-described LC-MS method (Martínez-Sánchez et al., 2006) and quantified by HPLC-DAD, using sinigrin (sinigrin monohydrate from Sinapis nigra, Phytoplan Diehm & Neuberger GmbH, Heidelberg, Germany) as standard. The glucosinolate concentration was expressed as mg per 100 g of fresh weight.
The chromatograms of phenolic compounds were recorded at 330 nm and quantified using external standards: caffeoyl quinic acid derivates using chlorogenic acid (Sigma-Aldrich, St. Louis, MO., U.S.A.), flavonoids with quercetin-3-rutinoside (Sigma-Aldrich) and sinapic acid derivatives using sinapinic acid (Sigma-Aldrich).
Glucosinolates Analysis by UPLC-MS/MS
The freeze-dried powder of the total leaves of all the genotypes was processed to determine their glucosinolates contents by UPLC-TripleQuad-MS/MS. Freeze-dried powder of leaf tissue (150 mg) was extracted as deswcribe above up to the centrifugation step. Extraction was performed using SPE Strata-X cartridges (33u Polymeric Strong Cation), following the instructions of the manufacturer (Phenomenex, Torrance, CA, USA). The cartridges were conditioned with 2 mL of MeOH and equilibrated with 2 mL of ultrapure water/formic acid (98:2, v/v). After this step, the samples were diluted in 2 mL of water/formic acid (98:2, v/v) and applied to the column, then washed with water/formic acid (98:2, v/v) and aspirated until dryness. The target analytes were eluted with 1 mL of MeOH/formic acid (98:2, v/v) and dried to completion using a SpeedVac concentrator (Savant SPD121P, Thermo Scientific, Massachusetts, USA). The extracts were reconstituted with 200 μL of solvents A/B (90:10, v:v) [solvent A: 13 mM ammonium acetate (pH 4 with acetic acid) and solvent B: acetonitrile:acetic acid (99.9:0.1, v:v)] and filtered through a 0.45-μm polyethersulphone filter (Millipore). Samples (20 μL) were analyzed by UPLC-MS/MS (Agilent Technologies, Waldbronn, Germany).
Chromatographic separation of sinigrin and allyl-isothiocyanate was carried out on a ZORBAX Eclipse Plus C-18 Rapid Resolution HD column (2.1 × 50 mm, 1.8 μm) (Agilent Technologies, Waldrom, Germany). The column temperatures were held at 10°C (left and right). The Multiple Reaction Monitoring (MRM) dynamic mode was used in the positive mode, assigning ±0.650 min as the™ interval time for each retention time and preferential transitions of the corresponding analytes. The dwell time was set at 30 ms. The flow-rate was 0.3 mL min−1, using the linear gradient scheme (t; %B): (0.0; 12), (0.2; 20), (1.0; 52), (2.5; 95), and (2.5; 12). The optimal ESI conditions for maximal detection of the analytes were: gas temperature, 225°C; sheath gas temperature, 350°C; capillary voltage, 3500 V; nozzle voltage, 1250 V; sheath gas flow, 12%; gas flow, 10; nebuliser, 40. The acquisition time was 2.5 min for each sample, with a post-run of 1.5 min for the column equilibration. The MS parameter fragmentor (ion optics capillary exit voltage) and collision energy were optimized for each compound, to generate the most-abundant product ions for the MRM mode. Data acquisition was performed using MassHunter software version B.04.00 (Agilent, Waldrom, Germany). The concentrations of glucosinolates were calculated from the area ratio of the ion peaks of the compounds to those of the corresponding standard curves (prepared fresh each day).
Thus, the results were obtained by using a sensitive, reproducible and robust UPLC-MS/MS method which improved the resolution of the peaks, with high reproducibility of the retention times and shorter analysis times (ca. 1/10) than regular HPLC-DAD-ESI-MSn methods.
Plasma Membrane Protein Purification from Roots
Root plasma membranes were purified using the two-phase, aqueous-polymer technique described previously (Muries et al., 2011). The protein concentration of the PIP-enriched fraction was determined with the RC DC Protein Assay kit (BioRad), using BSA as standard.
Gel Electrophoresis and Immunoblotting
Plasma membrane from the roots of Arabidopsis plants was isolated as previously described. Protein samples (10 μg per lane) were analyzed by 12% sodium dodecyl sulfate-polyacrylamide gel electrophoresis (SDS-PAGE) after denaturation at 56°C for 20 min in the presence of 2% (w/v) SDS and 100 mM DTT, in order to separate aquaporin dimers (Borgnia et al., 1999). The proteins were transferred to a PVDF membrane for 20 min at 15 V, in an electrophoretic transfer cell (Trans-Blot SD cell, BioRad), using Towbin transfer buffer (Towbin et al., 1979) with the addition of 0.05% SDS. After this, the membrane was blocked for 1 h at room temperature; in Tris-Buffered Saline (TBS) containing 2% (w/v) skimmed dry milk. Then, the membrane was incubated for 1 h at room temperature in a buffer containing TBS with 0.05% Tween 20, in the presence of an antibody (dilution 1:3000) raised against the first 42 N-terminus residues of Arabidopsis PIP1;1 (Kammerloher et al., 1994) (kindly provided by Prof. Dr. Schäffner) or against the 11 C-terminus residues of Brassica oleracea var. Italica PIP2 (Boursiac et al., 2005) (kindly provided by Prof. Dr. Santoni) (dilution 1:3000). Goat anti-rabbit IgG coupled to horseradish peroxidase was used as the secondary antibody (dilution 1:20000). A chemiluminescent signal was developed using the West-Pico, Super Signal substrate (Pierce). Immunoblots were performed on samples from three independent experiments. The intensity of each band was determined by a GS-800™ calibrated densitometer (Bio Rad, Barcelona, Spain). Background correction was performed by sampling membrane regions without spots and averaging their signal. The intensity of each band is the difference between the raw intensity of the protein of interest and the background. The scanned bands were normalized by calculating the ratio relative to the corresponding Coomassie-stained bands, thus ensuring that different intensities were not due to differences in the amount of protein loaded.
Statistical Analysis
The data were statistically analyzed, using the SPSS 17.0 software package (LEAD Technologies, Inc., Chicago, USA), by Tukey's test. Significant differences were determined at P < 0.05.
Results
Growth Parameters and Relative Water Content
Shoot and root biomass, length of primary and lateral roots, leaf area and percentage of seed germination were determined for the different genotypes with or without salt (Table 1). Under non-saline conditions, there were no statistically significant differences in shoot fresh (FW) and dry weights (DW) among genotypes. However, root FW and DW of the myb28myb29 mutant were roughly halved in respect to wild type (WT) or the myb28 mutant, in accordance with a shorter primary root length, as previously reported (Beekwilder et al., 2008). By contrast, salinity reduced shoot DW in all genotypes, as expected for plants with a diminished leaf area, but it did not affect, in the double mutant, either root DW or primary and lateral root lengths; the single mutant showed an increase in total lateral root length. In the WT, salt stress reduced the primary and lateral root lengths, while DW was maintained due to the increase in the number of lateral roots.
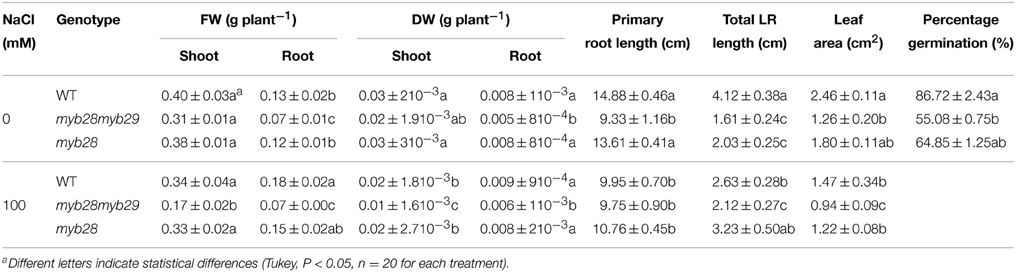
Table 1. Shoot and root fresh weight (FW, g plant−1) and dry weight (DW, g plant−1), primary root length (cm), total lateral root (LR, cm) length, leaf area (cm2), and percentage germination of the Arabidopsis thaliana ecotype Col-0 wild type (WT), single (myb28) and double (myb28myb29) knockout mutants under non-saline (0 mM NaCl) and saline (100 mM NaCl) conditions.
Total lateral root length in the myb28myb29 mutant was lower than in WT or the myb28 mutant but it was not affected by the presence of salt. Seed germination was reduced in the myb28myb29 mutant compared to WT and the single myb28 mutant.
Leaf area was greatest in WT followed by the myb28 mutant and smallest in the myb28myb29 mutant. Salinity decreased leaf area in all genotypes, with the exception of the myb28 mutant where it remained stable.
Hydraulic Conductance and Whole Plant Transpiration
The root hydraulic conductance (L0) was determined for all three genotypes by root pressurization (Figure 1A). In the absence of the salt treatment, the L0 of the myb28myb29 mutant was comparable to that of the WT and myb28 mutant. Salinity reduced L0 by 49.1 and 46.3% in the WT and single mutant, respectively, and by 63.0% in the double mutant.
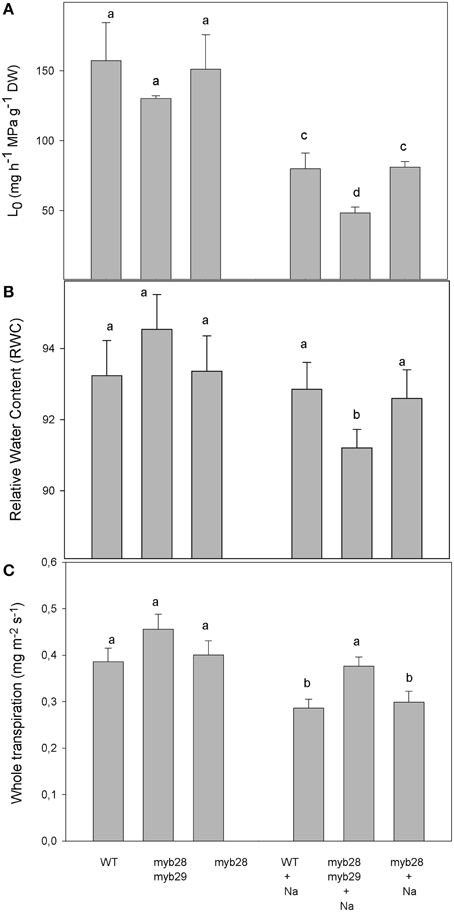
Figure 1. Root hydraulic conductance, L0, (mg h−1 MPa−1 g−1 DW) (A), relative water content (RWC) (B) and whole plant transpiration (mg m2 s−1) (C) of Arabidopsis thaliana ecotype Col-0 wild type (WT), single (myb28) and double (myb28myb29) knockout mutants under non-saline (0 mM NaCl) and saline (100 mM NaCl) conditions. Mean values ± standard errors are shown (n = 10). Mean values with different letters represent significant (P < 0.05) differences according to the Tukey test.
The relative water content (RWC) of the myb28myb29 mutant was significantly reduced by NaCl addition, whereas salinity had no effect on the RWC in the WT and myb28 mutant (Figure 1B).
Under salt stress, reductions in whole plant transpiration were higher in the WT and myb28 mutant, which had similar values, compared to the myb28myb29 mutant. However, under non-saline conditions, the whole plant transpiration was similar in all three genotypes (Figure 1C).
PIP Aquaporin Abundance in the Roots of the Arabidopsis thaliana Genotypes
For quantification of the A. thaliana PIP1 and PIP2 protein abundance in roots, Western Blotting was performed. Two specific antibodies developed against the PIP1 and PIP2 homologs of A. thaliana were used for protein immunodetection and two bands—at 29 kDa (monomeric form) and 58 kDa (dimeric form)—were detected in all samples (Figure 2). Under non-saline conditions, no significant differences were observed in the abundance of either PIP subfamily among the genotypes. However, the immunostain intensity differed among the genotypes when exposed to salinity. Thus, in general, the NaCl treatment decreased PIP1 (Figure 2A) and PIP2 (Figure 2B) abundance in the three genotypes, the reductions being 14.0, 56.4, and 21.2% in the PIP1 subfamily and 24.0, 50.3, and 41.2% in the PIP2 subfamily of the WT, myb28myb29 and myb28 plants, respectively.
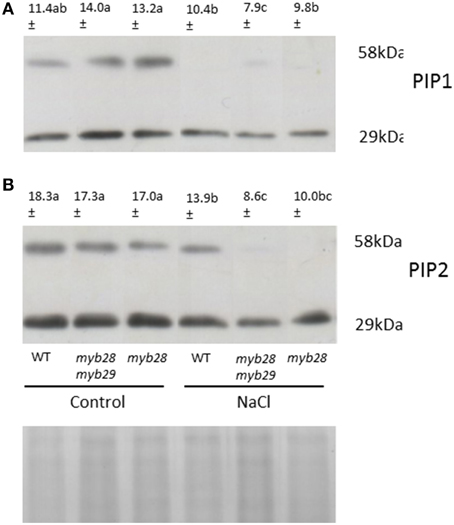
Figure 2. Immunodetection of PIP1 (A) and PIP2 (B) homologs in the root plasma membrane (PM) of Arabidopsis thaliana ecotype Col-0 wild type (WT), single (myb28) and double (myb28myb29) knockout mutants under non-saline (0 mM NaCl) and saline (100 mM NaCl) conditions. Total PM was separated by SDS-PAGE and probed with antibodies against AtPIP1 and AtPIP2. The PIP1 and PIP2 protein amounts (monomers and dimers) were quantified using the “Quantity one” program from Bio-Rad Laboratories. Mean values are shown (n = 3). Mean values with different letters represent significant (P < 0.05) differences according to the Tukey test. The scanned bands were normalized by calculating the ratio to the corresponding Coomassie-stained aquaporin band (29 KDa) ensuring that different intensities were not due to different loaded protein amounts.
Correlation between L0 and signal intensity of PIP2 and PIP2 was expressed in terms of the coefficient of determination (R2). R2 indicated the fraction of the variability in L0 that can be explained by the variability in aquaporin abundance through their linear relationship. High values for both PIP1 (R2 = 0.795) and PIP2 (R2 = 0.877) were observed.
Mineral Content
The ion concentrations in the leaves of the three genotypes were similar, under both non-saline and saline conditions (Table 2). Thus, salinity decreased the Ca, K, and S levels with regard to the control and increased the Na concentration in the three genotypes similarly, whereas the Mg, P, and N levels remained unchanged. The C level was increased by salinity in WT and myb28 plants, but was unmodified in the myb28myb29 mutant.
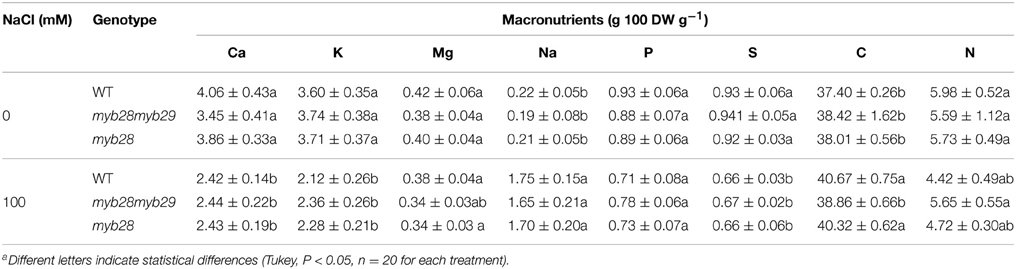
Table 2. Calcium (Ca), potassium (K), magnesium (Mg), sodium (Na), phosphorus (P), sulfur (S), carbon (C), and nitrogen (N) (g 100 DW g−1) in the leaves of Arabidopsis thaliana ecotype Col-0 wild type (WT), single (myb28) and double (myb28myb29) knockout mutants under non-saline (0 mM NaCl) and saline (100 mM NaCl) conditions.
Glucosinolates and Phenolic Compounds
The Arabidopsis leaves contained mainly glucoiberin (3MSOP), glucoraphanin (4MSOB), glucoalyssin (5MSOP), glucohesperin (6MSOH), glucosibarin (7MSOH) and glucohirsutin (8MSOO) as aliphatic glucosinolates (Table 3), while the major indole glucosinolates were glucobrassicin (I3M) and 4-methoxy-glucobrassicin (4MI3M), depending on the genotype. Thus, short-chain aliphatic glucosinolates were detected in the WT and myb28 mutant, whereas long-chain aliphatic glucosinolates were found exclusively in the WT. The indole glucosinolates occurred in all three genotypes (Table 3, Figure 3).
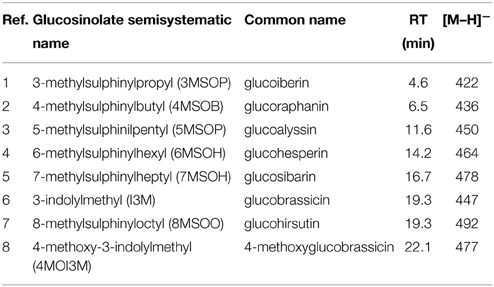
Table 3. ESI-MS data for glucosinolates detection in the different genotypes, abbreviation used in Figure 3 and retention time (RT).
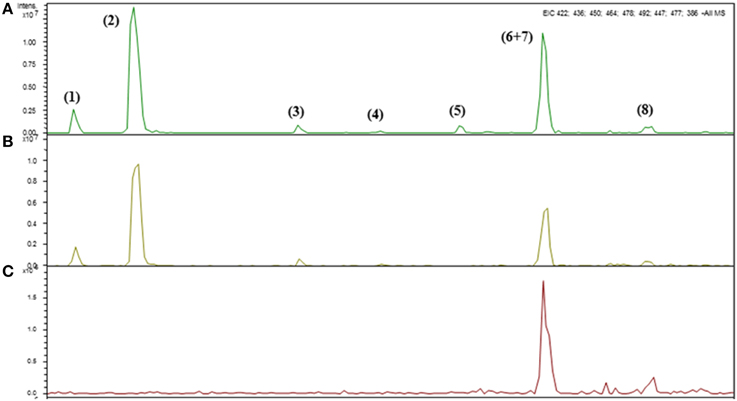
Figure 3. Identification of intact glucosinolates by HPLC-DAD-ESI-MSn in the leaves of Arabidopsis thaliana ecotype Col-0 wild type (WT) (A), single (myb28) (B) and double (myb28myb29) (C) knockout mutants under non-saline (0 mM NaCl) conditions.
In general, aliphatic glucosinolates were increased by salinity in WT (~2-fold) and myb28 mutant (~2.5-fold), reaching similar values (Figure 4). The level of the indolic glucosinolates was significantly higher in the myb28myb29 mutant (~1.8-fold), compared to the WT and myb28 mutant, but salinity decreased their content in the double mutant and left it unchanged in the WT and single mutant. Total glucosinolates were lower in the myb28myb29 mutant and salinity had no effect on their content. By contrast, salinity caused similar increments (~2-fold) of total glucosinolates in the WT and myb28 mutant.
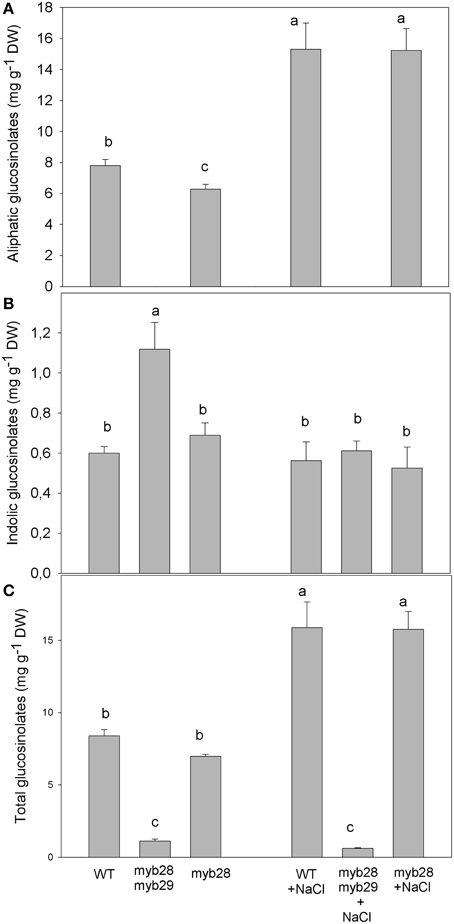
Figure 4. Total aliphatic (A), total indolic (B) and total glucosinolates (C) (mg g−1 DW) in the leaves of Arabidopsis thaliana ecotype Col-0 wild type (WT), single (myb28) and double (myb28myb29) knockout mutants under non-saline (0 mM NaCl) and saline (100 mM NaCl) conditions. Mean values ± standard errors are shown (n = 20). Mean values with different letters represent significant (P < 0.05) differences according to the Tukey test.
Regarding individual glucosinolates, salinity increased 3MSOP (~1.4-fold) and decreased 4MSOB (~1.2-fold) in the myb28 mutant, whereas 5MSOP increased in the WT (~2.1-fold) and myb28 mutant (~2.5-fold) (Table 4). The long-chain aliphatic glucosinolates 6MSOH, 7MSOH, and 8MSOO, detected only in the WT, were decreased by salinity, with 6MSOH becoming undetectable. The level of I3M was higher in the myb28myb29 mutant, where it was significantly reduced by salinity (~11.5-fold). Similarly, in the myb28 mutant, I3M was diminished by salt treatment (~1.3-fold), but it remained constant in WT. Under non-saline conditions, the 4MI3M content was similar in the three genotypes but salt stress decreased the level of this indolic glucosinolate in the myb28myb29 (~2.5-fold) and myb28 (~2.8-fold) mutants.
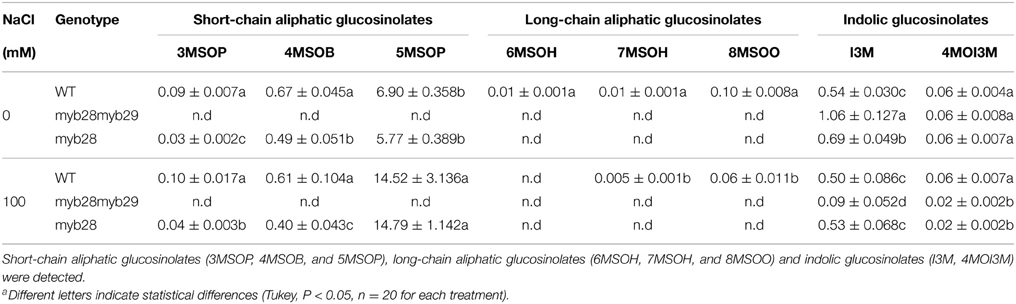
Table 4. Individual glucosinolates in the leaves of Arabidopsis thaliana ecotype Col-0 wild type (WT), single (myb28) and double (myb28myb29) knockout mutants under non-saline (0 mM NaCl) and saline (100 mM NaCl) conditions.
The total phenolic compounds, (defined as the sum of chlorogenic acid, flavonoids, and sinapic acid derivates), in the different genotypes are presented in Figure 5: under non-saline conditions, they were highest in the myb28myb29 mutant, followed by myb28 and the WT. Salinity increased the levels of phenolic compounds in the WT and myb28 mutant.
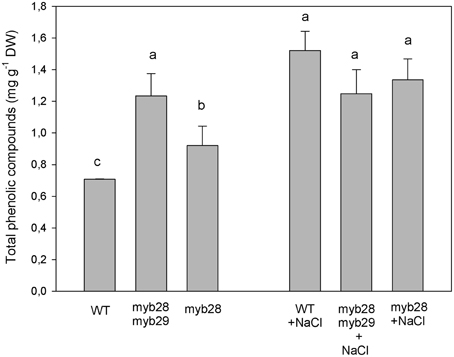
Figure 5. Total phenolic compounds (mg g−1 DW), as the sum of chlorogenic acid, flavonoids and sinapic acid derivates, in the leaves of Arabidopsis thaliana ecotype Col-0 wild type (WT), single (myb28) and double (myb28myb29) knockout mutants under non-saline (0 mM NaCl) and saline (100 mM NaCl) conditions. Mean values ± standard errors are shown (n = 20). Mean values with different letters represent significant (P < 0.05) differences according to the Tukey test.
Discussion
Previous analysis of the myb28 and myb29 single and myb28myb29 double knockout-mutant lines demonstrated that these two Myb genes cooperate to regulate aliphatic glucosinolates biosynthesis and that the presence of at least one functional gene is necessary for gene expression and metabolite accumulation. Not surprisingly modulation of the glucosinolate content influenced the extent of plant damage caused by the larvae of the lepidopteran insect Mamestra brassicae (Beekwilder et al., 2008). We explore in this study the effect of varying aliphatic and indolic glucosinolate level by genetic means on the response of these plants to abiotic stress. In particular, the connection between glucosinolates and water uptake and transport under salinity has been explored. As reported previously by Beekwilder et al. (2008), the initial growth of the double knockout line was delayed, and we provide some interpretation of this effect. We found that the myb28myb29 mutant had lower leaf area and root biomass than the WT under non-saline conditions, due to its reduced primary and lateral root (LR) lengths at the developmental stage investigated. In previous work, we observed an increase of glucosinolates at the highest salt concentration in relation to stress resistance and growth (Lopez-Berenguer et al., 2008) indicating that glucosinolates could be an adaptive component of salt tolerance (Qasim et al., 2003). These results have been related to the aquaporins function due to their role in root water uptake. Thus, aquaporins and hydraulic conductivity seem to be associated to changes in the anatomical and morphological features of the roots affecting nutritional status (Martínez-Ballesta et al., 2011). Therefore, the most parsimonious interpretation of our data is that the decrease in aliphatic glucosinolates expression and/or the increase in indolic glucosinolates, modify aquaporin abundance. This is consonant with a previous report where the lack of long-chain aliphatic glucosinolates in the cyp79F2 mutants of A. thaliana reduced LRs growth (Tantikanjana et al., 2004).
Leaf area and root hydraulic conductance under salt stress would then be affected primarily by root morphology. A decrease in the root hydraulic conductance (L0) may lead to leaf area reductions as a mechanism of plant response to salt stress. In this situation, the control of L0 mediated by root aquaporins may be crucial - as in the myb28myb29 mutant, where L0 was not synchronized with stomatal behavior and transpiration was unmodified.
Also, salt stress may induce morphological changes in the roots of A. thaliana; such as inhibition of primary root elongation and simultaneous increases in the LR number and branching under moderate salt stress (Potters et al., 2007; Zolla et al., 2010). These results agree with the morphological root changes observed in the WT, whereas increased LRs length in the single mutant may compensate the lack of more LRs. In Arabidopsis, salt stress can induce a strong reduction in the number of lateral root primordia (LRP) (Burssens et al., 2000) or, by contrast, an increase in LR number; for example, in Arabidopsis, Cicer arietinum and Oryza sativa cultivars (He et al., 2005; Shukla et al., 2006; Nibau et al., 2008; Krishnamurthy et al., 2011). A clear genotypic effect on the response of the root system to salinity is shown in this work. Salinity had no effect on the primary and lateral root lengths in the double mutant. The intrinsic reduced root system in this genotype, where there was a complete absence of aliphatic glucosinolates could originate that salinity did not modify root architecture. By contrast, the highest increase in the aliphatic glucosinolates content under salt stress was in the myb28 genotype, relative to the WT, and this coincided with stimulation of LR growth.
The relationship between aliphatic/indolic glucosinolates and root proliferation under salt stress needs further research. In addition, the percentage seed germination declined as the aliphatic glucosinolate levels decreased, both being lower in the myb28myb29 mutant, and as the levels of indolic glucosinolates increased. The effect could be again mediated by alterations in auxin content or sensing or by breakdown products of glucosinolates which contribute to broccoli seed germination, for instance as sulfur donors during germination (Gao et al., 2014).
The mineral content was unaffected by the mutations, except for carbon. The absence of aliphatic glucosinolates resulted in unmodified, steady-state transpiration in the myb28myb29 genotype under salt stress; this may have maintained the uptake of ions and could explain the lack of difference in their tissue accumulation in relation to the WT. Moreover, a lower RWC may have concentrated the foliar ions in salt treated myb28myb29 plants. The modest carbon increase in WT and myb28 mutant under salinity was probably related to increased accumulation of carbohydrates or other carbon rich compounds, a strategy to cope with salt stress. In fact, sugars, which accumulate in response to environmental stresses, may act as osmolytes to maintain cell turgor and they have been previously shown to increase in Arabidopsis plants under salt stress (Krasensky and Jonak, 2012). This response may not occur in the double mutant.
The good correlation between L0 and the protein levels of both PIP1 (R2 = 0.795) and PIP2 (R2 = 0.877) suggest a symplastic regulation of water flow under both non-saline and saline conditions. Aquaporin abundance in root plasma membrane may have contributed to the differences in L0- among genotypes. It has been reported previously that perturbation of glucosinolate biosynthesis by suppression of the CYP79F1 and CYP79F2 genes, (by RNAi), modified the expression of cell environment-interacting proteins (by ~12%) among others, including PIP2a (Chen et al., 2012). Similar correlation between L0 and the PIP1 content was observed in broccoli plants after exogenous sinigrin addition and the subsequent allyl-isothiocyanate formation. However, the PIP2 content was not related to the L0 values under the above conditions, although under salt stress the greater sinigrin effect on osmotic water permeability (Pf)—rather than L0—provided evidence for the contribution of PIP2 aquaporins to the cell-to-cell pathway (Martínez-Ballesta et al., 2014). Differences among exogenous and endogenous glucosinolates, genotypes and stress conditions may influence the contribution of aquaporins to this route.
Under non-saline conditions, in spite of the phenotypic alteration shown by myb28myb29 plants (reduced leaf and root growth), plant water relations were comparable with L0 contributions in all genotypes. However, under salinity, the greater reduction in L0 in the double mutant plants, together with the maintenance of whole plant transpiration, reduced their RWC. These results show that the contribution of short-chain aliphatic glucosinolates to water saving are more important under natural conditions—whereas variations in the environment, such as salt stress, may require an adjustment of stomatal opening. It has been shown previously that addition of glucosinolate breakdown product, for instance allyl-isothiocyanate, under salt stress increased the abundance of the PIP2 subfamily and led to the recovery of L0, with respect to the addition of NaCl alone (Martínez-Ballesta et al., 2014). This positive effect of glucosinolate byproducts in the face of salinity could help to explain the fact that the lack of aliphatic glucosinolates in the myb28myb29 genotype and the related PIP2 abundance resulted in a lower L0 under salt stress. Furthermore, under salt stress, axial resistance to water transport may contribute to total root resistance (Passioura, 1977; Rewald et al., 2011c) and root branching could influence L0 (Joly, 1989). The increased LRs number in the WT and LRs length in the myb28 mutant under salt stress may account for their similar L0 values, in addition to the lesser reduction in PIPs abundance, compared to the myb28myb29 mutant.
The transcription factors MYB28 and MYB29, together with MYB76, are the main regulators of aliphatic glucosinolate biosynthesis (Gigolashvili et al., 2007a,b; Hirai et al., 2007). In the double mutant, all aliphatic glucosinolates (long- or short-chain) were below the detection level, even in sensitive LC-MS analyses. Coordinated control of different glucosinolate biosynthetic pathways has been observed and the inhibition of one class of glucosinolates resulted in a compensatory increase in another class (Hemm et al., 2003; Grubb and Abel, 2006; Yan and Chen, 2007; Beekwilder et al., 2008; Malitsky et al., 2008). Thus, in our experiments indolic glucosinolates increased in the myb2829 mutants, compensating the lack of aliphatic glucosinolates—as also found in previous studies (Hirai et al., 2007; Beekwilder et al., 2008). Under salt stress, aliphatic glucosinolates increased in WT and myb28. This is related to the aquaporins expression as it has been suggested by their involvement on osmoregulation pathways (Martínez-Ballesta et al., 2014): this may have led to the improved water status of WT and myb28 plants under stress. Previous findings indicated that salt-induced increases in the glucosinolate content may be involved in the salt-stress response of the halophyte Thellungiella salsuginea (Pang et al., 2009, 2012). However, variations in the individual glucosinolates and particular developmental stages were considered. Thus, the link between PIP abundance, root hydraulic conductance and the presence of a particular aliphatic glucosinolate in glycophytes is now well stated and deserves further attention, especially in crops and where the induction of an individual glucosinolate is involved in increasing resistance to salt stress.
Conclusion
The results presented suggest that the pathways involved in the physiological responses to salt stress are connected to glucosinolate metabolism (i.e., the production of isothiocyanates and indoles) which is influenced by abiotic stress factors. In this sense, the root and leaf morphological changes provoked by salinity showed genotypic dependence and aliphatic, rather than indole, glucosinolates may condition these responses. Given the link between the L0 and PIP aquaporins, one would expect their involvement in the observed change in L0, indicating the contribution of these proteins to water flow through the root. The simplest interpretation for the differences in growth and responses to salt stress among the three genotypes could invoke differences in auxin content or sensing, however the lack of short-chain aliphatic glucosinolates or their hydrolysis products may affect more directly plant water relations and aquaporins response under salt stress. Future, research will need to address how internal and external factors that regulate glucosinolate metabolism affect planta and to identify which signaling molecules are involved in the response to salt stress and the mechanisms of the interconnected glucosinolate-aquaporin relation. A first indication of water balance adaptation to salinity influenced by the availability of aliphatic glucosinolates as elements involved in plant osmoregulation is given. In addition, whether the perturbation of aliphatic glucosinolates is sufficient to affect auxin synthesis in the shoot (and therefore root morphology) could be obtained by crossing the double knockout line to the DR5:GUS reporter in order to visualize auxin concentration in the mutant.
Conflict of Interest Statement
The authors declare that the research was conducted in the absence of any commercial or financial relationships that could be construed as a potential conflict of interest.
Acknowledgments
The authors thank Dr. David J. Walker, for correction of the written English in the manuscript. This work was supported by the following Spanish Ministry of Science and Innovation R&D and innovation programs AGL2009-12720 and Ramón y Cajal' (Ref RYC-2009-04574).
Abbreviations
BSA, bovine serum albumin; DAD, Diode Array Detector; ESI, Electrospray interface; SDS-PAGE, sodium dodecyl sulfate-polyacrylamide gel electrophoresis.
References
Ashraf, M., and Haris, P. J. C. (2004). Potential biochemical indicators of salinity tolerance in plants. Plant Sci. 166, 3–16. doi: 10.1016/j.plantsci.2003.10.024
Beekwilder, J., Van Leeuwen, W., Van Dam, N. M., Bertossi, M., Grandi, V., and Mizzi, L., et al. (2008). The impact of the absence of aliphatic glucosinolates on insect herbivory in Arabidopsis. PLoS ONE 3:e2068. doi: 10.1371/journal.pone.0002068
Bennett, R. N., Mellon, F. A., Rosa, E. A., Perkins, L., and Kroon, P. A. (2004). Profiling glucosinolates, flavonoids, alkaloids, and other secondary metabolites in tissues of Azima tetracantha L. (Salvadoraceae). J. Agric. Food Chem. 52, 5856–5862. doi: 10.1021/jf040091+
Borgnia, M. J., Kozono, D., Maloney, P., and Agre, P. (1999). Purification and functional reconstitution of bacterial aquaporins. Biophys. J. 76, A436–A436.
Boursiac, Y., Chen, S., Luu, D. T., Sorieul, M., van den Dries, N., and Maurel, C. (2005). Early effects of salinity on water transport in Arabidopsis roots. Molecular and cellular features of aquaporin expression. Plant Physiol. 139, 790–805. doi: 10.1104/pp.105.065029
Brown, P. D., Tokuhisa, J. G., Reichelt, M., and Gershenzon, J. (2003). Variation of glucosinolate accumulation among different organs and developmental stages of Arabidopsis thaliana. Phytochemistry 62, 471–481. doi: 10.1016/S0031-9422(02)00549-6
Burssens, S., Himanen, K., van de Cotte, B., Beeckman, T., Van Montagu, M., Inzé, D., et al. (2000). Expression of cell cycle regulatory genes and morphological alterations in response to salt stress in Arabidopsis thaliana. Planta 211, 632–640. doi: 10.1007/s004250000334
Chen, Y. Z., Pang, Q. Y., Hea, Y., Zhua, N., Branstroma, I., Yanb, X. F., et al. (2012). Proteomics and metabolomics of Arabidopsis responses to perturbation of glucosinolate biosynthesis. Mol. Plant 5, 1138–1150. doi: 10.1093/mp/sss034
Fahey, J. W., Zalcmann, A. T., and Talalay, P. (2001). The chemical diversity and distribution of glucosinolates and isothiocyanates among plants. Phytochemistry 56, 5–51. doi: 10.1016/S0031-9422(00)00316-2
Fan, J., Crooks, C., Creissen, G., Hill, L., Fairhurst, S., Doerner, P., et al. (2011). Pseudomonas sax genes overcome aliphatic isothiocyanate-mediated non-host resistance in Arabidopsis. Science 331, 1185–1188. doi: 10.1126/science.1199707
Finley, J. W. (2005). Proposed criteria for assessing the efficacy of cancer reduction by plant foods enriched in carotenoids, glucosinolates, polyphenols and selenocompounds. Ann. Bot. 95, 1075–1096. doi: 10.1093/aob/mci123
Gao, J., Yu, X., Ma, F., and Li, J. (2014). RNA-Seq analysis of transcriptome and glucosinolate metabolism in seeds and sprouts of broccoli (Brassica oleracea var. italic). Plos ONE 9:e88804. doi: 10.1371/journal.pone.0088804
Gibeaut, D. M., Hulen, J., Cramer, G. R., and Seemann, J. R. (1997). Maximal biomass of Arabidopsis thaliana using a simple, low-maintenance hydroponic method and favorable environmental conditions. Plant Physiol. 115, 317–319. doi: 10.1104/pp.115.2.317
Gigolashvili, T., Berger, B., and Flügge, U. I. (2009). Specific and coordinated control of indolic and aliphatic glucosinolate biosynthesis by R2R3- MYB transcription factors in Arabidopsis thaliana. Phytochem. Rev. 8, 3–13. doi: 10.1007/s11101-008-9112-6
Gigolashvili, T., Berger, B., Mock, H. S., Muller, C., Weisshaar, B., and Flugge, U. I. (2007a). The transcription factor HIG1/MYB51 regulates indolic glucosinolate biosynthesis in Arabidopsis thaliana. Plant J. 50, 886–901. doi: 10.1111/j.1365-313X.2007.03099.x
Gigolashvili, T., Engqvist, M., Yatusevich, R., Muller, C., and Flugge, U. I. (2008). HAG2/MYB76 and HAG3/MYB29 exert a specific and coordinated control on the regulation of aliphatic glucosinolate biosynthesis in Arabidopsis thaliana. New Phytol. 177, 627–642. doi: 10.1111/j.1469-8137.2007.02295.x
Gigolashvili, T., Yatusevich, R., Berger, B., Muller, C., and Flugge, U. I. (2007b). The R2R3-MYB transcription factor HAG1/MYB28 is a regulator of methionine-derived glucosinolate biosynthesis in Arabidopsis thaliana. Plant J. 51, 247–261. doi: 10.1111/j.1365-313X.2007.03133.x
Grubb, C., and Abel, S. (2006). Glucosinolate metabolism and its control. Trends Plant Sci. 11, 89–100. doi: 10.1016/j.tplants.2005.12.006
Hara, M., Harazaki, A., and Tabata, K. (2013). Administration of isothiocyanates enhances heat tolerance in Arabidopsis thaliana. Plant Growth Regul. 69, 71–77. doi: 10.1007/s10725-012-9748-5
Haugen, R., Steffes, L., Wolf, J., Brown, P., Matzner, S., and Siemens, D. H. (2008). Evolution of drought tolerance and defense: dependence of tradeoffs on mechanism, environment and defense switching. Oikos 117, 231–244. doi: 10.1111/j.2007.0030-1299.16111.x
He, X. J., Mu, R. L., Cao, W. H., Zhang, Z. G., Zhang, J. S., and Chen, S. Y. (2005). AtNAC2, a transcription factor downstream of ethylene and auxin signaling pathways, is involved in salt stress response and lateral root development. Plant J. 44, 903–916. doi: 10.1111/j.1365-313X.2005.02575.x
Hemm, M. R., Ruegger, M. O., and Chapple, C. (2003). The Arabidopsis ref2 mutant is defective in the gene encoding CYP83A1 and shows both phenylpropanoid and glucosinolate phenotypes. Plant Cell 15, 179–194. doi: 10.1105/tpc.006544
Hirai, M. Y., Sugiyama, K., Sawada, Y., Tohge, T., Obayashi, T., Suzuki, A., et al. (2007). Omics-based identification of Arabidopsis Myb transcription factors regulating aliphatic glucosinolate biosynthesis. Proc. Natl. Acad. Sci. U.S.A. 104, 6478–6483. doi: 10.1073/pnas.0611629104
Javot, H., Lauvergeat, V., Santoni, V., Martin-Laurent, F., Güclü, J., Vinh, J., et al. (2003). Role of a single aquaporin isoform in root water uptake. Plant Cell 15, 509–522. doi: 10.1105/tpc.008888
Joly, R. J. (1989). Effects of sodium chloride on the hydraulic conductivity of soybean root systems. Plant Physiol. 91, 1262–1265. doi: 10.1104/pp.91.4.1262
Kammerloher, W., Fischer, U., Piechottka, G. P., and Schaffner, A. R. (1994). Water channels in the plant plasma membrane cloned by immunoselection from a mammalian expression system. Plant J. 6, 187–199. doi: 10.1046/j.1365-313X.1994.6020187.x
Keling, H., and Zhujun, Z. (2010). Effects of different concentrations of sodium chloride on plant growth and glucosinolate content and composition in pakchoi. Afr. J. Biotechnol. 9, 4428–4433.
Khokon, M. A., Jahan, M. S., Rahman, T., Hossain, M. A., Muroyama, D., Minami, I., et al. (2011). Allylisothiocyanate (AITC) induces stomatal closure in Arabidopsis. Plant Cell Environ. 34, 1900–1906. doi: 10.1111/j.1365-3040.2011.02385.x
Kim, J. H., Lee, B. W., Schroeder, F. C., and Jander, G. (2008). Identification of indole glucosinolate breakdown products with antifeedant effects on Myzus persicae (green peach aphid). Plant J. 54, 1015–1026. doi: 10.1111/j.1365-313X.2008.03476.x
Krasensky, J., and Jonak, C. (2012). Drought, salt, and temperature stress-induced metabolic rearrangements and regulatory networks. J. Exp. Bot. 1–16. doi: 10.1093/jxb/err460
Krishnamurthy, P., Ranathunge, K., Nayak, S., Schreiber, L., and Mathew, M. K. (2011). Root apoplastic barriers block Na+ transport to shoots in rice (Oryza sativa L.). J. Exp. Bot. 62, 4215–4228. doi: 10.1093/jxb/err135
Kuhlmann, F., and Muller, C. (2009). Independent responses to ultraviolet radiation and herbivore attack in broccoli. J. Exp. Bot. 60, 3467–3475. doi: 10.1093/jxb/erp182
Li, S., Liu, J., Liu, Z., Li, X., Wu, F., and He, Y. (2014). Heat- induced task target mediates thermotolerance via heat stress transcription factor A1a-directed pathway in Arabidopsis. Plant Cell 26, 1764–1780. doi: 10.1105/tpc.114.124883
Lopez-Berenguer, C., Martinez-Ballesta, M. C., Garcia-Viguera, C., and Carvajal, M. (2008). Leaf water balance mediated by aquaporins under salt stress and associated glucosinolate synthesis in broccoli. Plant Sci. 174, 321–328. doi: 10.1016/j.plantsci.2007.11.012
López-Berenguer, C., Martínez-Ballesta, M. C., García-Viguera, C., and Carvajal, M. (2008). Leaf water balance mediated by aquaporins under salt stress and associated glucosinolate synthesis in broccoli. Plant Sci. 174, 321–328. doi: 10.1016/j.plantsci.2007.11.012
Malitsky, S., Blum, E., Less, H., Venger, I., Elbaz, M., Morin, S., et al. (2008). The transcript and metabolite networks affected by the two clades of Arabidopsis glucosinolate biosynthesis regulators. Plant Physiol. 148, 2021–2049. doi: 10.1104/pp.108.124784
Martínez-Ballesta, M. C., Moreno, D. A., and Carvajal, M. (2013). The physiological importance of glucosinolates on plant response to abiotic stress in Brassica. Int. J. Mol. Sci. 14, 11607–11625. doi: 10.3390/ijms140611607
Martínez-Ballesta, M. C., Muries, B., Moreno, D. A., Domínguez-Perles, R., García-Viguera, C., and Carvajal, M. (2014). Involvement of a glucosinolate (sinigrin) in the regulation of water transport in Brassica oleracea grown under salt stress. Physiol. Plant. 150, 145–160. doi: 10.1111/ppl.12082
Martínez-Ballesta, M. C., Rodríguez-Hernández, M. C., Alcaraz-López, C., Mota-Cadenas, C., Muries, B., and Carvajal, M. (2011). “Plant hydraulic conductivity: the aquaporins contribution.” in Hydraulic Conductivity - Issues, Determination and Applications, ed L. Elango (Murcia: In Tech), 103–121.
Martínez-Sánchez, A., Allende, A., Bennett, R. N., Ferreres, F., and Gil, M. I. (2006). Microbial, nutritional and sensory quality of rocket leaves as affected by different sanitizers. Postharvest Biol. Tech. 42, 86–97. doi: 10.1016/j.postharvbio.2006.05.010
Muries, B., Faize, M., Carvajal, M., and Martínez-Ballesta, M. C. (2011). Differential aquaporin expression induced by salinity in broccoli plants. Mol. Biosyst. 7, 1322–1335. doi: 10.1039/c0mb00285b
Nibau, C., Gibbs, D. J., and Coates, J. C. (2008). Branching out in new directions: the control of root architecture by lateral root formation. New Phytol. 179, 595–614. doi: 10.1111/j.1469-8137.2008.02472.x
Pang, Q., Guo, J., Chen, S., Chen, Y, Zhang, L, Fei, M., et al. (2012). Effect of salt treatment on the glucosinolate-myrosinase system in Thellungiella salsuginea. Plant Soil 355, 363–374. doi: 10.1007/s11104-011-1108-0
Pang, Q. Y., Chen, S. X., Li, L. X., and Yan, X. F. (2009). Characterization of glucosinolate-myrosinase system in developing salt cress Thellungiella halophila. Physiol. Plant. 136, 1–9. doi: 10.1111/j.1399-3054.2009.01211.x
Passioura, J. B. (1977). Determining soil-water diffusivities from 1-Step outflow experiments. Aust. J. Soil Res. 15, 1–8. doi: 10.1071/SR9770001
Potters, G., Pasternak, T. P., Guisez, Y., and Jansen, M. A. K. (2007). Stress induced morphogenic responses: growing out of trouble? Trends Plant Sci. 12, 98–105. doi: 10.1016/j.tplants.2007.01.004
Qasim, M., Ashraf, M., Ashraf, M. Y., Rehman, S. U., and Rha, E. S. (2003). Salt induced changes in two canola cultivars differing in salt tolerance. Biol. Plant. 46, 629–632. doi: 10.1023/A:1024844402000
Redovnikovic, I. R., Glivetic, T., Delonga, K., and Vorkapic-Furac, J. (2008). Glucosinolates and their portential role in plant. Period. Biol. 110, 297–309.
Reichelt, M., Brown, P. D., Scheneider, B., Oldham, N. J., Stauber, E., Tokuhisa, J., et al. (2002). Benzoic acid glucosinolate esters and other glucosinolates from Arabidopsis thaliana. Phytochem. 59, 663–671. doi: 10.1016/S0031-9422(02)00014-6
Rewald, B., Leuschner, C., Wiesman, Z., and Ephrath, J. E. (2011c). Influence of salinity on root hydraulic properties of three olive varieties. Plant Biosyst. 145, 12–22. doi: 10.1080/11263504.2010.514130
Rosa, E. A. S. (1997). Daily variation in glucosinolate concentrations in the leaves and roots of cabbage seedlings in two constant temperature regimes. J. Sci. Food Agric. 73, 364–368.
Schlaeppi, K., and Mauch, F. (2010). Indolic secondary metabolites protect Arabidopsis from the oomycete pathogen Phytophthora brassicae. Plant Signal. Behav. 5, 1099–1101. doi: 10.4161/psb.5.9.12410
Schonhof, I., Blankenburg, D., Müller, S., and Krumbein, A. (2007). Sulfur and nitrogen supply influence growth, product appearance, and glucosinolate concentration of broccoli. J. Plant Nutr. Soil Sci. 170, 65–72. doi: 10.1002/jpln.200620639
Schreiner, M., Beyene, B., Krumbein, A., and Stutzel, H. (2009). Ontogenetic changes of 2-propenyl and 3-indolylmethyl glucosinolates in Brassica carinata leaves as affected by water supply. J. Agric. Food Chem. 57, 7259–7263. doi: 10.1021/jf901076h
Shukla, R. K., Raha, S., Tripathi, V., and Chattopadhyay, D. (2006). Expression of CAP2, an APETALA2-family transcription factor from chickpea, enhances Growth and tolerance to dehydration and salt stress in transgenic tobacco. Plant Physiol. 142, 113–123. doi: 10.1104/pp.106.081752
Smolen, G., and Bender, I. (2002). Arabidopsis cytochrome P450 cyp83B1 mutations activate the tryptophan biosynthetic pathway. Genetics 160, 323–332.
Sonderby, I. E, Hansen, B. G., Bjarnholt, N., Ticconi, C., Halkier, B. A., et al. (2007). A systems biology approach Identifies a R2R3 MYB gene subfamily with distinct and overlapping functions in regulation of aliphatic glucosinolates. PLoS ONE 2:e1322. doi: 10.1371/journal.pone.0001322
Stroeher, V. L., Boothe, J. G., and Good, A. G. (1995). Molecular cloning and expression of a turgor-responsive gene in Brassica napus. Plant Mol. Biol. 27, 541–551. doi: 10.1007/BF00019320
Tantikanjana, T., Mikkelsen, M. D., Hussain, M., Halkier, B. A., and Sundaresan, V. (2004). Functional analysis of the tandem- duplicated P450 genes SPS/BUS/CYP79F1 and CYP79F2 in glucosinolate biosynthesis and plant development by Ds transposition- generated double mutants. Plant Physiol. 135, 840–848. doi: 10.1104/pp.104.040113
Towbin, H., Staehelin, T., and Gordon, J. (1979). Electrophoretic transfer of proteins from polyacrylamide gels to nitrocellulose sheets: procedure and some applications. Proc. Natl. Acad. Sci. U.S.A. 76, 4350–4354. doi: 10.1073/pnas.76.9.4350
Yan, X. F., and Chen, S. X. (2007). Regulation of plant glucosinolate metabolism. Planta 226, 1343–1352. doi: 10.1007/s00425-007-0627-7
Yuan, G. F., Wang, X. P., Guo, R. F., and Wang, Q. M. (2010). Effect of salt stress on phenolic compounds, glucosinolates, myrosinase and antioxidant activity in radish sprouts. Food Chem. 121, 1014–1019. doi: 10.1016/j.foodchem.2010.01.040
Zareba, G., and Serradelf, N. (2004). Chemoprotective effects of broccoli and other brassica vegetables. Drugs Fut. 29, 1097–1104. doi: 10.1358/dof.2004.029.11.868839
Keywords: Arabidopsis thaliana, Brassicaceae, glucosinolates, hydraulic conductance, plasma membrane intrinsic protein
Citation: Martínez-Ballesta M, Moreno-Fernández DA, Castejón D, Ochando C, Morandini PA and Carvajal M (2015) The impact of the absence of aliphatic glucosinolates on water transport under salt stress in Arabidopsis thaliana. Front. Plant Sci. 6:524. doi: 10.3389/fpls.2015.00524
Received: 12 February 2015; Accepted: 29 June 2015;
Published: 15 July 2015.
Edited by:
Masakazu Hara, Shizuoka University, JapanReviewed by:
Omar Pantoja, Universidad Nacional Autonoma de Mexico, MexicoTamara Gigolashvili, University of Cologne, Germany
Copyright © 2015 Martínez-Ballesta, Moreno-Fernández, Castejón, Ochando, Morandini and Carvajal. This is an open-access article distributed under the terms of the Creative Commons Attribution License (CC BY). The use, distribution or reproduction in other forums is permitted, provided the original author(s) or licensor are credited and that the original publication in this journal is cited, in accordance with accepted academic practice. No use, distribution or reproduction is permitted which does not comply with these terms.
*Correspondence: Mcarmen Martínez-Ballesta, Plant Nutrition Department, Centro de Edafología y Biología Aplicada del Segura (CEBAS-CSIC), PO Box 164, 30100 Espinardo, Murcia, Spain, mballesta@cebas.csic.es