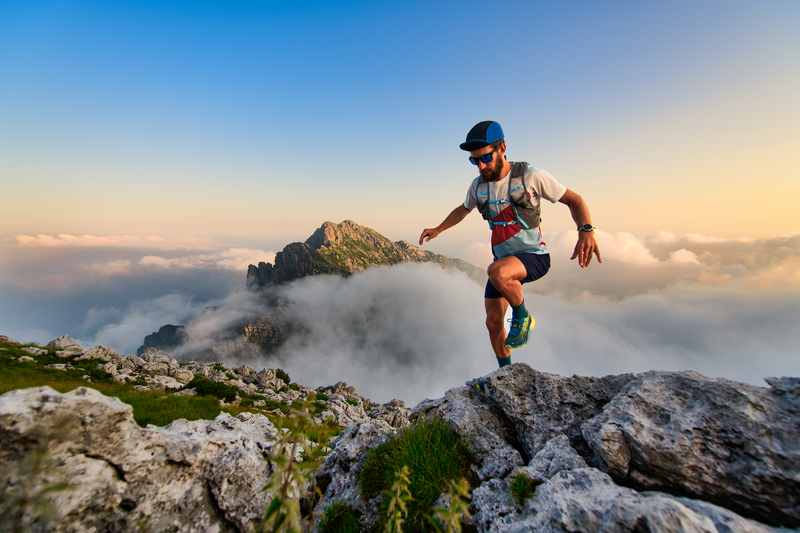
95% of researchers rate our articles as excellent or good
Learn more about the work of our research integrity team to safeguard the quality of each article we publish.
Find out more
REVIEW article
Front. Plant Sci. , 16 June 2015
Sec. Plant Physiology
Volume 6 - 2015 | https://doi.org/10.3389/fpls.2015.00420
This article is part of the Research Topic Recent insights into the double role of hydrogen peroxide in plants View all 21 articles
Plants are constantly challenged by various abiotic stresses that negatively affect growth and productivity worldwide. During the course of their evolution, plants have developed sophisticated mechanisms to recognize external signals allowing them to respond appropriately to environmental conditions, although the degree of adjustability or tolerance to specific stresses differs from species to species. Overproduction of reactive oxygen species (ROS; hydrogen peroxide, H2O2; superoxide, ; hydroxyl radical, OH⋅ and singlet oxygen, 1O2) is enhanced under abiotic and/or biotic stresses, which can cause oxidative damage to plant macromolecules and cell structures, leading to inhibition of plant growth and development, or to death. Among the various ROS, freely diffusible and relatively long-lived H2O2 acts as a central player in stress signal transduction pathways. These pathways can then activate multiple acclamatory responses that reinforce resistance to various abiotic and biotic stressors. To utilize H2O2 as a signaling molecule, non-toxic levels must be maintained in a delicate balancing act between H2O2 production and scavenging. Several recent studies have demonstrated that the H2O2-priming can enhance abiotic stress tolerance by modulating ROS detoxification and by regulating multiple stress-responsive pathways and gene expression. Despite the importance of the H2O2-priming, little is known about how this process improves the tolerance of plants to stress. Understanding the mechanisms of H2O2-priming-induced abiotic stress tolerance will be valuable for identifying biotechnological strategies to improve abiotic stress tolerance in crop plants. This review is an overview of our current knowledge of the possible mechanisms associated with H2O2-induced abiotic oxidative stress tolerance in plants, with special reference to antioxidant metabolism.
In plants the production of reactive oxygen species (ROS) is a common outcome of various metabolic reactions that occur in multiple sites within a plant cell. ROS like hydrogen peroxide (H2O2), superoxide (), the hydroxyl radical (OH⋅) and singlet oxygen (1) are also produced as one of the earliest responses of plant cells to environmental stresses, and these ROS molecules can cause damage to a variety of biological processes (Halliwell, 2006; Gill and Tuteja, 2010; Das and Roychoudhury, 2014). In plants subjected to various abiotic stresses, such as salt, drought, chilling, heat and metal or metalloid stresses, ROS levels can rise significantly, leading to redox imbalance and oxidative stress (Hossain et al., 2010; Hasanuzzaman et al., 2011a,b; Hossain and Fujita, 2013; Mostofa and Fujita, 2013; Das and Roychoudhury, 2014; Mostofa et al., 2014a,b,c; Nahar et al., 2014). High ROS levels can result in extensive damage to proteins, DNA, and lipids, thereby affecting normal cellular functions, which can lead to permanent metabolic dysfunction and plant death (Anjum et al., 2015). To combat oxidative stress, plants have developed an elaborate system to control cellular ROS titer (Mittler et al., 2011). Surprisingly, plants have also evolved a way to exploit lower titer of ROS as signaling component to regulate wide variety of plant processes, including cell elongation, differentiation, morphogenesis and responses to environmental stress (Dat et al., 2000; Foreman et al., 2003; Tsukagoshi et al., 2010; Bhattacharjee, 2012).
In the last decade, H2O2 received considerable interest among the ROS and other oxygen-derived free radicals. H2O2, the result of two electron reduction via (the first step one electron reduction component), possesses the highest half-life (1 ms) of the ROS. A comparatively long life span and the small size of H2O2 molecules permit them to traverse through cellular membranes to different cellular compartments, facilitating signaling functions, including retrograde signaling (Apel and Hirt, 2004; Bienert et al., 2006; Maruta et al., 2012; Noctor et al., 2014). The signaling role of H2O2 is well established, particularly with reference to plant processes like stress acclimation, antioxidative defense, cell wall cross-linking, stomatal behavior, phytoalexin production, regulation of the cell cycle, and photosynthesis. So, the toxicity or danger associated with H2O2 on one hand and signaling cascades on other make it a versatile molecule whose concentration needs to be tightly controlled within plant cells (Petrov and Van Breusegem, 2012).
There are multiple sources of H2O2 in plant cells, including over-energization of electron transport chains (ETC) or redox reactions in chloroplasts or mitochondria, fatty acid oxidation, and photorespiration (Figure 1). Of these sources, the most significant is oxidation of glycolate in the peroxisome during the photosynthetic carbon oxidation cycle. In addition, the oxidative burst associated with part of hypersensitive response to pathogens also cause rapid increase in the concentration of H2O2 (Miller et al., 2010). One of the main sources of H2O2 is a class of cell membrane-bound NADPH-dependent oxidases that are similar to the respiratory burst oxidase homologs (RBOH). In plants, RBOH are in fact enzymes regulated by a class of Rho-like proteins called ‘ROP’s (Rho-related GTPases; Agrawal et al., 2003). Another class of enzymes, associated with the formation of H2O2, is the cell wall-associated peroxidases (Bolwell et al., 2002). Rates of H2O2 accumulation in peroxisomes and chloroplasts may be 30–100 times higher compared with H2O2 formation in mitochondria. Importantly, ROS formation in mitochondria does not vary significantly in presence or absence of light, since the total O2 consumption is less affected by light than TCA cycle activity. However, the formation of by electron transport systems can be influenced by light, if exposure to light affects alternative oxidase activity (Dutilleul et al., 2003). Alternative oxidases have been found to influence ROS generation and to be involved in determining cell survival under stressful conditions (Maxwell et al., 1999; Robson and Vanlerberghe, 2002).
FIGURE 1. Schematic representation of H2O2 generation in different intra- and extra-cellular sites and the subsequent signaling associated with the regulation of defense gene expression in plant cells.
The antioxidant systems that regulate H2O2 levels consist of both non-enzymatic and enzymatic H2O2 scavengers. Enzymes, such as catalase (CAT), ascorbate peroxidase (APX), glutathione peroxidase (GPX), glutathione S-transferases (GSTs), glutathione reductase (GR), and peroxyredoxin (Prx), and non-enzymatic compounds, like ascorbate (AsA), glutathione (GSH), α-tocopherol and flavonoids, are constantly involved in regulating the concentration of ROS, including H2O2 (Miller et al., 2010; Kapoor et al., 2015). In fact, both the production and scavenging of H2O2 in plant cells seem to be integrated in a network and are responsible for the ‘biological effect.’ The paradox of H2O2 physiology lies with its opposing activities; at higher concentrations it causes oxidative damage to important cellular metabolites, whereas at lower concentrations it initiates cell signaling (Gechev and Hille, 2005; Bhattacharjee, 2012). The redox imbalance associated with environmental stresses, such as salinity and extremes of temperature, increases the overall rate of metabolism and eventually up-regulates H2O2 production in plant cells (Bhattacharjee, 2012, 2013).
Priming (pre-treatment of seeds or plants by exposure to stressor or chemical compounds, making them more tolerant to later stress events) is potentially an important mechanism of induced resistance in plants against biotic stresses (Beckers and Conrath, 2007; Tanou et al., 2012; Borges et al., 2014). Recent studies have shown that priming can also modulate abiotic stress tolerance (Filippou et al., 2012; Hossain and Fujita, 2013; Mostofa and Fujita, 2013; Borges et al., 2014; Mostofa et al., 2014a,b,c; Nahar et al., 2014; Wang et al., 2014a). Despite the agronomic and ecological importance of priming, in-depth molecular mechanisms associated with priming in plants are still unknown (Conrath, 2011). Mounting evidence suggests that the initial exposure to chemical priming agents (such as H2O2, ABA, NO, SA etc.) renders plants more tolerant to abiotic stresses (Wang et al., 2010a; Hasanuzzaman et al., 2011a; Mostofa and Fujita, 2013; Mostofa et al., 2014a; Sathiyaraj et al., 2014; Teng et al., 2014). A number of studies on plants have demonstrated that the pre-treatment with an appropriate level of H2O2 can enhance abiotic stress tolerance through the modulation of multiple physiological processes, such as photosynthesis, and by modulating multiple stress-responsive pathways, such as the ROS and methylglyoxal (MG) detoxification pathways (Azevedo-Neto et al., 2005; Chao et al., 2009; Liu et al., 2010a; Xu et al., 2010; Wang et al., 2010a, 2014a; Ishibashi et al., 2011; Gondim et al., 2012, 2013; Hossain and Fujita, 2013). Although H2O2 is known to act as a signaling molecule, activating multiple defense responses that reinforce resistance to various environmental stresses in plants (Petrov and Van Breusegem, 2012), very little is known about the mechanisms by which plants perceive/sense H2O2 and how this sensing mechanism is coordinated within the developmental program of a plant. In this review, we summarize our current understanding of the possible mechanisms associated with H2O2-induced enhanced abiotic stress tolerance with special reference to ROS detoxifying/scavenging proteins and gene expression.
Many recent studies on plants have demonstrated that H2O2 is a key player in the signal transduction process associated with tolerance to abiotic and biotic stresses, and the induction of the stress cross-tolerance phenomena often observed in plants. A number of reports, discussed in more detail below, have shown that exogenous application of H2O2 can induce tolerance to salinity, drought, chilling, and high temperatures, and heavy metal stress, all of which cause elevated H2O2 production (Gong et al., 2001; Uchida et al., 2002; Azevedo-Neto et al., 2005; Chao et al., 2009; Liu et al., 2010a; Wang et al., 2010a, 2014a; Ishibashi et al., 2011; Gondim et al., 2012, 2013; Hossain and Fujita, 2013).
The salt stress-induced oxidative burst due to uncontrolled ROS accumulation has been well documented in plants. However, several recent studies on plants have demonstrated that pre-treatment with exogenous H2O2 can induce salt tolerance. Uchida et al. (2002) studied the effects of H2O2 and nitric oxide (NO) pre-treatments on oxidative stress in rice (Oryza sativa) plants under salt or heat stress. Their results showed that seedlings treated with low concentrations (<10 μM) of H2O2 or NO resulted in greener leaves and a higher photosynthetic activity than that of the control plants under conditions of salt or heat stress. It was also shown that pre-treatment induced increases in ROS scavenging enzyme activities and increased expression of genes encoding Δ′-pyrroline-5-carboxylate synthase, sucrose-phosphate synthase, and the small heat shock protein 26. Their findings indicate that NO and H2O2 act as signaling molecules that modulate heat and salt stress tolerance by regulating the expression of stress-related genes. In addition, Azevedo-Neto et al. (2005) found that supplementation of the nutrient solution with H2O2 induced salt tolerance in maize plants, by enhancing antioxidant metabolism and reducing lipid peroxidation in both leaves and roots. Wahid et al. (2007) reported that exogenous H2O2 improved salinity tolerance in Triticum aestivum when seeds were soaked in H2O2 (1–120 μM, 8 h) and subsequently grown in saline conditions (150 mM NaCl). H2O2 levels in the seedlings, arising from H2O2-treated seeds, were markedly lower when grown under saline conditions than control seedlings from seeds not treated with H2O2, and also exhibited better photosynthetic capacity. These results suggest that seedlings from H2O2-treated seeds had more effective antioxidant systems than found in untreated controls. Moreover, the H2O2 treatment appeared to improve leaf water relations, helped to maintain turgor, and improved the K+:Na+ ratio of salt stressed seedlings. H2O2 treatment also enhanced membrane properties, with greatly reduced relative membrane permeability (RMP) and lower ion leakage. Surprisingly, the expression of two heat-stable proteins (32 and 52 kDa) was also observed in H2O2 pre-treated seedlings. Fedina et al. (2009) reported that Hordeum vulgare seedlings pre-treated with H2O2 (1 and 5 mM) had higher rates of CO2 fixation and lower malondialdehyde (MDA) and H2O2 contents, following exposure to 150 mM NaCl for 4 and 7 days, when compared with seedlings subjected to NaCl stress only. In addition, the leaf Cl- content of NaCl treated plants was considerably less in H2O2 pre-treated plants. The above findings indicate that H2O2 metabolism might be important for the induction of salt tolerance.
Gondim et al. (2010) evaluated the roles of H2O2 on the growth and acclimation of maize (Zea mays) triple hybrid (BRS3003) seedlings exposed to salinity stress, with three consecutive studies. In the first studies, H2O2 accelerated the percentage germination of seeds at 100 mM, but not at 500 mM H2O2. In second study, pre-treatment of seeds with H2O2 caused an up-regulation of APX and CAT activities after 30 h. In contrast, GPX activity was lower in seeds primed with H2O2 for 12, 24, 30, 36, and 42 h as compared with the seeds primed with water only. The activity of SOD was not affected by pre-treatment of seeds with H2O2, except for the 24 h pre-treatment. In the third experiment, seeds were pre-treated by soaking in 100 mM H2O2 for 36 h, or in distilled water (DW), and then grown in a culture solution with or without salt stress (80 mM NaCl). Their findings showed that priming of seeds with H2O2 increased seedling tolerance to salinity, with seedlings demonstrating improved growth rates. The differences in the levels of antioxidant enzyme activities detailed above may explain the higher salinity tolerance of seedlings from seeds pre-treated with H2O2. In addition, Li et al. (2011) reported that exogenously applied H2O2 (0.05 μM) reduced the MDA content, enhanced the GSH content and increased the activities of APX, CAT, SOD, and POD in wheat seedlings under salt stress. A similar response in Suaeda fruticosa (a halophyte) was also found, indicating that cellular defense antioxidant mechanisms are enhanced by the exogenous application of H2O2 (Hameed et al., 2012). Up-regulation of the activities of CAT and SOD following the exogenous application of H2O2 (0.5 mM) was also observed in oat (Avena sativa) plants under salt stress (Xu et al., 2008). Similarly, Gondim et al. (2012) found that foliar H2O2 priming was effective in minimizing salt stress in maize and analysis of the antioxidant enzymes CAT, GPOX, APX, and SOD revealed that the H2O2 foliar spray increased the activities of all of these enzymes. CAT was found to be the most highly responsive of the above enzymes to H2O2, with high activities observed (48 h) after treatment, while GPX and APX responded much later (240 h after treatment). Lower MDA levels were also detected in maize plants with higher CAT activities, which may have resulted from the H2O2 detoxifying function of this enzyme. In addition, Gondim et al. (2013) studied the influence of exogenous H2O2 application on AsA and GSH metabolism, relative chlorophyll content, relative water content (RWC), and gas exchange, in Zea mays grown under salinity. Photosynthesis and transpiration, stomatal conductance, and intercellular CO2 concentrations all declined in plants under salt stress; however, the negative impact of salt stress was not as great in plants sprayed with H2O2. In addition, H2O2-sprayed plants had higher RWCs, relative chlorophyll contents and lower leaf H2O2 accumulation, which correlated positively with improved gas exchange, compared with control plants under conditions of NaCl stress. The non-enzymatic antioxidants AsA and GSH did not appear to play any obvious roles as ROS scavengers in this study. The authors of the above study concluded that salt tolerance of maize plants, brought by pretreatment of leaves with H2O2, was due to less H2O2 accumulation and to maintenance of the leaf RWC and chlorophyll contents. These characteristics allowed higher photosynthesis and improved growth of maize plants under salt stress. In addition to these findings, Ashfaque et al. (2014) conducted an experiment to study the role H2O2 played in mitigating salt stress in wheat (Triticum aestivum L.) plants. Treatment of plants with H2O2 positively influenced plant growth under saline and non-saline conditions. The application of 50 or 100 μM H2O2 reduced the severity of salt stress, with reductions in both Na+ and Cl- ion levels and an increase in proline content and in N assimilation. Improved water relations, increased levels of photosynthetic pigments and greater growth rates were also observed in H2O2 under salt stress when compared with untreated plants. Under non-saline conditions application of H2O2 also improved all the parameters detailed above. Treatment with 100 μM H2O2 provided maximal protection for wheat plants grown under non-saline conditions and also alleviated the effects of salt stress in plants grown under saline conditions. Recently, Sathiyaraj et al. (2014) found that Panax ginseng seedlings treated with 100 μM H2O2 for 2 days showed enhanced salinity tolerance and increased activities of APX, CAT, and guaiacol peroxidase. Other oxidative parameters such as MDA levels and endogenous H2O2 and levels were lower in H2O2 treated salt-stressed seedlings. Seedling dry weight, and chlorophyll and carotenoid contents were also greater in H2O2 treated seedlings than in untreated controls, when seedlings were subjected to salt stress. The above findings demonstrate that H2O2 priming can induce tolerance to salinity in plants by modulating physiological and metabolic processes such as photosynthesis, proline accumulation and ROS detoxification, and that this ultimately leads to better growth and development. Importantly, ROS metabolism also plays a pivotal role in the development of stress and cross stress tolerance.
Drought stress is widely thought to induce oxidative stress by increasing the levels of H2O2 and singlet oxygen (de Carvalho, 2013). However, Jing et al. (2009) investigated the capacity of H2O2 priming to promote drought tolerance in Cucumber plants. Drought stress resulted in cucumber plants with round chloroplasts, and indistinct chloroplast membranes and thylakoids. While H2O2 priming did not change chloroplast ultrastructure, priming did increase the activities of the antioxidant enzymes SOD, CAT, GPOX, APX, DHAR, DHAR, GR, and the levels of AsA and GSH, resulting in lower levels of MDA, H2O2 and . The authors of this study concluded that by increasing antioxidant capacity H2O2 priming reduced the accumulation of ROS in treated plants, and alleviated some of the membrane damage found in the chloroplasts of plants under drought stress. In a similar study Ishibashi et al. (2011) showed that spraying plants with H2O2 could alleviate the symptoms of drought stress in soybean. The RWC content, photosynthetic rate and stomatal conductance of drought-stressed leaves in plants sprayed with H2O2 were all higher than in leaves sprayed with DW. In contrast to spraying with DW, spraying with H2O2 caused an increase in the expression of galactinol synthase (GolS) and d-myo-inositol 3-phosphate synthase 2 (GmMIPS2) genes, which are responsible for the synthesis of oligosaccharides. These findings indicated that H2O2 spraying enabled soybean plants to avoid drought stress by helping to maintain leaf water levels, and that leaf water retention was probably due to increased oligosaccharide biosynthesis rather than rapid stomatal closure. Abass and Mohamed (2011) also studied the effects of priming seeds with H2O2 on the drought tolerance of common bean seedlings (Phaseolus vulgaris L.). A significant decrease in plant growth parameters, photosynthetic pigments, and the total carbohydrate content was observed in response to drought stress. In contrast, a significant increase in compatible solutes, polyamine and antioxidant levels, and abscisic acid (ABA) contents were observed in plants in response to drought stress. H2O2-priming of seeds enhanced all of the above parameters in seedlings grown under drought conditions when compared with the seedlings of water-treated seeds. The above findings suggest that H2O2 could trigger the activation of defense mechanisms, including increased levels of antioxidants, which then persist in developing seedlings and help to alleviate damage and improve plant growth and performance under drought. Liao et al. (2012) studied the beneficial roles of exogenous NO and H2O2 in marigold (Tagetes erecta L.) adventitious root formation in response to drought. NO or H2O2 treatment reduced the damage to mesophyll cell ultrastructure caused by drought stress. NO or H2O2 treatment also increased leaf chlorophyll contents, chlorophyll fluorescence parameters (Fv/Fm, ΦPS II, and qP), and hypocotyl soluble carbohydrate and protein contents, while reducing starch contents. These findings demonstrate that NO or H2O2 can protect mesophyll cell ultrastructure from damage, improve the photosynthetic performance of leaves and mitigate the negative effects of drought stress, by enhancing nitrogen and carbohydrate accumulation. Recently, Hossain and Fujita (2013) examined the potential biochemical mechanisms of H2O2 priming-induced drought tolerance in mustard (Brassica juncea L.) seedlings by investigating ROS scavenging and MG metabolism. Eight-day-old seedlings were pre-treated with a low concentration (50 μM) of H2O2 for 24 h prior to the imposition of drought stress for 48 h. H2O2 priming enhanced cell membrane stability in leaf tissues under drought stress, by reducing tissue MDA contents. The levels of endogenous H2O2, in H2O2 pre-treated, drought stressed-seedlings were markedly lower than that of seedlings subjected to drought stress without H2O2 pre-treatment. Lower activities of APX, CAT, and Gly II were observed in response to drought stress, whereas DHAR, GPX, and Gly I activities significantly increased. AsA, GSH, and GSSG levels increased significantly, whereas the GSH/GSSG ratio decreased in drought-stressed seedlings. Surprisingly, H2O2 pre-treated drought-stressed seedlings maintained significantly higher APX, GR, CAT, GST, and Gly II activities, as well as a higher GSH/GSSG ratio compared with seedlings under drought only. These results show that H2O2 priming can activate both ROS and MG detoxification pathways and modulate the tolerance of seedlings to water deficit (Hossain and Fujita, 2013). Ashraf et al. (2014) investigated the beneficial roles of exogenous H2O2 on drought stress tolerance in maize. Maize seedlings were pre-treated with different concentrations of H2O2 and grown under conditions of water stress. Higher germination percentages were found in seeds soaked in 140 mM H2O2. Drought led to a sharp decrease in photosynthetic pigments, whereas the levels of H2O2, lipid peroxidation and AsA increased. The activities of CAT, SOD, and POX rapidly increased. Importantly, the 140 mM H2O2 treatment reduced photosynthetic pigment degradation and lipid peroxidation and increased the activities of antioxidant enzymes and AsA levels. The beneficial influence of exogenous H2O2 treatments have also been observed in plants under osmotic stress. Liu et al. (2010a) studied the effects of exogenous H2O2 on osmotic stress-induced alterations in the ultra-structures of chloroplasts and mitochondria in two cucumber (Cucumis sativus L.) varieties. Osmotic stress caused the degradation of chloroplast and mitochondrial membranes in both cucumber genotypes and increased MDA levels. Osmotic stress and exogenous H2O2 both increased MnSOD, GPX, CAT, GPOX, APX, GR, MDHAR, DAHR activities and levels of the antioxidants AsA and GSH. The combined effects of osmotic stress and exogenous H2O2 resulted in the highest antioxidant levels in both cucumber ecotypes. Liu et al. (2010a) proposed that pre-treatment with H2O2 increased antioxidant levels in the leaves of cucumbers, thereby decreasing MDA levels, and protecting the ultrastructure of most chloroplasts and mitochondria in plants under osmotic stress. Terzi et al. (2014) also found that exogenous H2O2 (10 mM) pre-treatment induced osmotic stress tolerance in maize (Zea mays L.) seedlings. H2O2 treatment caused a decrease in MDA levels and stomatal conductance, whereas an increase in endogenous H2O2, leaf water potential, ABA concentration, and metabolite levels, including soluble sugars, proline, and polyamines, were observed. Osmotic stress caused a decline in leaf water potential and stomatal conductance, but the levels of MDA, H2O2, metabolite levels and the ABA content increased. Importantly, H2O2 pretreated osmotical stressed seedlings showed improved water status and stomatal conductance, as well as accumulation of MDA, H2O2, ABA, and metabolites. These results demonstrate that H2O2 pre-treatment induces osmotic stress tolerance by increasing soluble sugar, proline, and polyamine levels.
The positive role of exogenous H2O2 in modulating low temperature stress tolerance has been well documented. Prasad et al. (1994a,b) reported that addition of H2O2 modulated chilling tolerance, due to a transient increase in H2O2-activated acclimation mechanisms. The authors suggested that H2O2 has dual effects on maize plants during acclimation to chilling; it serves as a signal to induce the synthesis of ROS-scavenging enzymes, and in non-acclimated seedlings it accumulates to higher levels and acts as a destructive agent. Additionally, it was reported that both H2O2 and SA could mediate the induction of protective mechanisms against abiotic stresses. SA pre-treatment induced an increase in H2O2 concentrations that in turn triggers an increase in antioxidant enzyme activities and eventually leads to higher tolerance to chilling stress in maize seedlings (Janda et al., 1999). Likewise, H2O2 and SA were involved in the signal transduction pathway leading to acclimation during heat stress in mustard (Dat et al., 1998). Yu et al. (2003) showed that a transient oxidative shock, induced by exogenous H2O2, effectively increased chilling tolerance in mung bean (Vigna radiata L. cv. V3327) seedlings. Seedlings pre-treated with 200 mM H2O2 had increased survival rates (from 30 to 70%) and lowered EL (86 to 21%). Importantly, the endogenous level of H2O2 was not affected by exogenous application of H2O2. Surprisingly, exogenous H2O2 repressed the stimulation of ROS detoxifying enzymes APX and CAT; however, GSH levels increased significantly under both chilling and control conditions. Pre-treatment of mung bean plants with both ABA and H2O2 showed no synergistic effect on GSH content. The authors concluded that H2O2-mediated chilling tolerance in mung bean plants might be mediated by an increase in GSH content that is independent of ABA. Supporting this finding, Hung et al. (2007) showed that H2O2 pre-treatment induced chilling tolerance in chilling sensitive mung bean seedlings (V. radiata L. Cv Tainan Number 5). Seedlings pre-treated with 200 mM H2O2 or cold-acclimated (10°C for 48 h in the light) showed lower electrolyte leakage (EL) compared to seedlings subjected to chilling stress (4°C for 36 h) without H2O2 treatment or cold-acclimation. Chilling tolerance induced by H2O2 appeared to depend on the accumulation of GSH, as tolerance could be reversed by pretreatment with buthionine sulfoximine (BSO). In contrast, tolerance induced by cold-acclimation was neither accompanied by the accumulation of GSH nor reversed by BSO, suggesting that there are at least two independent mechanisms for developing chilling tolerance. Wang et al. (2010a) studied the effects of foliar pre-treatment with H2O2 in modulating chilling stress tolerance of mascarene grass (Zoysia tenuifolia) and manilagrass (Zoysia matrella). Pre-treatment with H2O2 (10 mM) was found to modulate chilling (7°C/2°C, day/night) stress tolerance as indicated by lower MDA and EL levels and higher protein contents. Pre-treatment significantly increased the activities of APX, GPX, and CAT in Zoysia matrella and APX, GR, and POD activities in Zoysia tenuifolia, indicating that H2O2 acts as a signaling molecule and modulates the metabolic responses associated with ROS-induced damage caused by chilling. Importantly, optimal pre-treatments reduced any increases in H2O2 levels, improved chilling tolerance, and increased CAT, POD, APX, GR, and GPX activities. Therefore, antioxidative enzymes are likely to be important factors for the acquisition of chilling tolerance in both Zoysia cultivars. Moussa and Mohamed (2011) showed that priming of pea seeds with H2O2 or NO significantly enhanced drought induced oxidative stress tolerance. Seeds were pre-treated with 70 mM H2O2 or 10 μM sodium nitroprusside (a NO donor). Seeds pre-treated pea seedlings have less ROS-induced damage, accelerated proline synthesis and enhanced total chlorophyll and carotenoid contents, increased photosynthetic activity, and increased growth when subjected to osmotic stress. Drought stress reduced the activities of APX, GPX, and CAT, and caused an overproduction of in the leaves of pea plants, which in turn increased MDA levels and reduced photosynthetic performance. Pre-treatment with SNP or H2O2 modulated the activities of antioxidant enzymes, limited production, and inhibited membrane peroxidation under drought stress, which indicated an enhanced operation of antioxidant systems. Moreover, after H2O2 or SNP pre-treatment seedlings had enhanced membrane stability as revealed by a lower MDA contents. The increased production of antioxidants in seedlings from seeds pre-treated with H2O2 or SNP persisted for some time, alleviating ROS-induced impairment and modulating the physiological characters associated with drought tolerance of seedlings. Recently, İşeri et al. (2013) investigated whether exogenous H2O2 application could influence the short-term cold responses of tomato plants and induce acclimation. Pre-treatments were performed by immersing roots into 1 mM H2O2 solution for 1 h and then transferring the seedlings to the soil (acclimated group). Cold stress (3°C for 16 h) caused a significant reduction in the RWC of control and non-acclimated groups when compared with unstressed plants. H2O2 promoted the maintenance of a higher RWC under stress. Anthocyanin levels in the leaves of acclimated plants under cold stress were significantly higher than those of unstressed control and non-acclimated plants. High MDA levels demonstrated low temperature induced oxidative damage in control and non-acclimated plants. MDA levels in acclimated plants remained similar to those of unstressed plants, which demonstrated that the H2O2 acclimation process protected the cells against cold induced lipid peroxidation. In addition, H2O2 acclimation caused proline accumulation in roots under cold stress and APX activity in the roots of cold-stressed and -unstressed H2O2-acclimated plants increased when compared with control and non-acclimated plants, with the highest increase in the roots of acclimated plants under cold stress. CAT levels in the roots of acclimated plants also increased, whereas levels remained unchanged in unstressed plants. Endogenous H2O2 levels increased significantly in the roots of control and non-acclimated plants under cold stress. In contrast, the H2O2 content of the roots of acclimated plants was significantly lower than that of control and non-acclimated plants under cold stress. These results demonstrate that H2O2 significantly enhances oxidative stress responses by elevating the antioxidant status of tomato plants.
Like other abiotic stresses endogenous levels of H2O2 increase in heat stressed plants (Hossain et al., 2013a,b; Mostofa et al., 2014b) and exogenous pre-treatments haves been found to increase the heat tolerance of plants. Kang et al. (2009) reported that H2O2 pre-treatments increased the activities of APX and glucose-6-phosphated dehydrogenase (G6PDH) in cucumber and tomato seedlings, and induced tolerance to heat stress. Bhattacharjee (2012) reported that H2O2 enhanced tolerance of two rice cultivars differing in salt tolerance (SR 26B, salt-tolerant; Ratna, salt-sensitive cultivar) to heat- or chilling-induced oxidative stress. Salt or drought stress results in significant increases in lipid peroxidation and protein oxidation, along with concomitant increases in the accumulation of ROS (and H2O2) and a reduction in antioxidant defenses (assessed in terms of total thiol content and the activities of SOD, CAT, APX, and GR) in both the seedlings of salt-sensitive Ratna and salt-tolerant SR 26B cultivars. Imbibitional treatment with low concentrations of H2O2 reduced oxidative damage to newly assembled membrane systems caused by heat and chilling stress in the seedlings of both cultivars of rice (Ratna and SR 26B). Imbibitional H2O2 treatment also caused an increase in antioxidant defenses (activities of SOD, CAT, APX, GR, and total thiol content) in the heat and chilling stressed seedlings and caused a significant improvement in the early growth performances of both cultivars. Better responses to H2O2-mediated acclamatory performances and restoration of redox-homeostasis under extremes of temperature were noted for the salt-sensitive rice cultivar Ratna compared with the salt-tolerant SR 26B. In general, these results suggest a significant role for an ‘inductive pulse’ of H2O2 in acclimatizing plants to adverse temperatures, by helping to maintain redox-homeostasis and mitigating oxidative membrane, protein and lipid damage during the recovery phase of the post-germination event. Wang et al. (2014a) studied the beneficial roles of exogenous H2O2 in modulating heat stress tolerance in turfgrass species. Ryegrass (Lolium perenne cv. Accent) and tall fescue (Festuca arundinacea cv. Barlexas) were sprayed with 10 mM H2O2 before they were exposed to heat stress (38/30°C, day/night) and compared with plants maintained at control temperatures (26/15°C, day/night). Before being subjected to heat stress seedlings treated with H2O2 were found to have increased activities of POD, CAT, APX, GR, and GPX, as well as larger AsA and GSH pools. Importantly the ratio of GSH/GSSG was also lower. Under heat stress H2O2 pre-treated seedlings showed lower oxidative damage and H2O2 levels, and increased activities of APX, GR, GST, and GPX. These results indicated that H2O2 could up-regulate the antioxidant defense systems that ultimately lead to improved thermotolerance in turfgrass species.
Excessive production of ROS, especially H2O2, in response to heavy metal exposure has been widely observed in plants (Hossain et al., 2010; Mostofa and Fujita, 2013; Mostofa et al., 2014a). H2O2 priming has also been found to increase tolerance of plants to heavy metals. Hu et al. (2009) showed H2O2 pre-treatment induced Cd tolerance in rice (O. sativa). Cd stress led to a significant decrease in both the length and biomass of roots and shoots. However, pre-treatment with 100 μM H2O2 for 1 day mitigated Cd stress and increased the levels of the antioxidant enzymes (SOD, CAT, GPX, APX, and GST), as well as elevated the levels of GSH and AsA. Consequently, the levels of MDA and H2O2 declined and the growth rates of the seedlings improved. H2O2 pre-treatment also decreased the Cd concentration found in the shoots, and lowered the shoot:root Cd ratio, indicating that H2O2 may affect Cd distribution in rice seedlings. Improved Cd tolerance was thought to be partly due to enhanced antioxidant metabolism that effectively prevented the increase in ROS levels under Cd stress. Higher Cd sequestration in root tissue may also contribute to the decline in Cd translocation. Chao et al. (2009) investigated the role of GSH in modulating heavy metal (Cd) stress tolerance of rice seedlings. Seedlings treated with either a heat shock or H2O2 showed a significant increase in leaf GSH levels. Treatment with exogenous GSH under non-heat stress conditions, which also resulted in an increase in GSH levels in leaves, also enhanced the Cd tolerance of rice seedlings. Pre-treatment of seedlings with an inhibitor of GSH synthesis inhibited the increase in GSH levels caused by heat shock or H2O2 treatment and caused a reduction Cd tolerance. Importantly, the negative effects of BSO could be reversed by the addition of GSH. A time-course analyses of heat stress in rice seedlings demonstrated that the accumulation of H2O2 preceded the increase in GSH. This finding suggests that early augmentation of H2O2 levels during heat shock treatments acts as a signal to modulate GSH biosynthesis and to protect rice plants from Cd-induced damage. Xu et al. (2010) reported the H2O2-induced up-regulation of AsA and GSH metabolism-induced Al tolerance in wheat seedlings. Al stress resulted in higher and H2O2 contents, greater MDA levels, programmed cell death, and inhibited root growth in both rice genotypes. The activities of CAT, POD, SOD, GPX, GR, MDHAR, and DHAR and the levels of GSH and AsA increased in response to Al stress. However, H2O2-primed Al-stressed seedlings showed higher activities of GPX, CAT, POD, MDHAR, DHAR, and GR, and higher AsA and GSH contents as well as a more favorable redox state than seedlings subjected to Al-stress only. Notably, a large increase in ROS detoxifying enzyme activities was observed in the Al-susceptible genotype as compared with the resistant genotype. H2O2 pre-treatment increased the tolerance of plants to Al-induced oxidative stress by increasing level of GSH and AsA and the activities of enzymes involved in their metabolism. Bai et al. (2011) studied the effects of H2O2 pretreatment on Cd tolerance and translocation by utilizing two rice genotypes (N07-6 and N07-63) with contrasting Cd tolerance. Cd stress (50 μmol/L) led a sharp decline in seedling growth and increased production of MDA, GSH, NPT, and phytochelatins (PCs), as well as GST activity. H2O2 pre-treatment further improved Cd stress tolerance by increasing the levels of NPT, GSH, and PCs, and the activity of GST in roots. The increase was greater in N07-63 as compared with the N07-6.
Chou et al. (2012) investigated the involvement of H2O2 in heat-shock induced Cd tolerance, in relation to the activities of ROS detoxifying enzymes (APX and GR) in rice plants. Heat-shock treatments increased the content of H2O2 before increases in the activities of APX and GR were observed in rice leaves. Importantly, heat-shock induced OsAPX2 gene expression was associated with heat shock induced increases in APX activity. Upon imposition of Cd stress the H2O2 content and the activities of APX and GR increased, but the increase was less than that observed in seedlings subjected to Cd-stress without heat pre-treatment. The authors concluded that H2O2 is involved in the regulation of heat shock and Cd-induced increases in the activities of GR and APX in rice leaves, and thus cross-tolerance in rice plants. Yildiz et al. (2013) studied the ameliorating effects of H2O2 on chromium (Cr) toxicity in canola (B. napus L.) plants in relation to thiol content, lipid peroxidation, antioxidant enzyme activities and the growth and chlorophyll content, as well as the levels of a metallothionein protein (BnMP1). Cr stress (50 μM) significantly reduced plant growth, which was accompanied by increased lipid peroxidation and a decrease in the chlorophyll content of the leaves. H2O2 pre-treatment, enhanced plant growth reduced the MDA levels and promoted higher levels of pigments. Additionally, the accumulation of Cr was higher in the aerial parts of the H2O2-pretreated seedlings. Increased thiol levels were observed under Cr stress and were further enhanced by H2O2 pre-treatment. POD and SOD activities increased in response to Cr stress, whereas the activities of CAT and APX decreased. H2O2 pre-treatment caused an increase in the activities of APX and POD in response to Cr stress, but CAT and SOD activities remained unchanged. BnMP1 expression analysis showed enhanced expression after 1 day of treatment, and then a decrease after 7 days exposure to Cr. In contrast, after 7 days exposure to Cr, H2O2-pre-treated seedlings showed a less decrease in BnMP1 expression as compared with the seedlings subjected to Cr stress only. These findings indicate that H2O2 may act as a signal that triggers defense mechanisms that in turn protect plants from ROS-induced damage caused by exposure to Cr. In addition, the roles of exogenous H2O2 on plant growth, water status, mineral ion content, proline content, and total sugar and soluble protein contents were evaluated in maize leaves exposed to copper (Cu) stress (0.5 mM Cu) by Guzel and Terzi (2013). Cu stress resulted in a decrease in leaf water potential, ionic concentration (Na+, K+, Ca+, Mg2+) and protein levels, but increases in the content of proline content and total soluble sugars, when compared with controls. Importantly H2O2-pre-treated seedlings showed an increase in growth, water content, mineral concentration, proline content, total soluble sugar and soluble protein contents when compared with control plants. A greater increase was also observed in the proline and total sugar contents of the Cu+H2O2 group of plants compared with the Cu stress alone group. These findings suggest that exogenous H2O2 can increase dry matter production and mineral ion distribution in maize seedlings. Additionally, osmotic regulation might be involved in the alleviation of Cu toxicity of maize leaves caused by pre-treatment of H2O2.
The possible involvement of H2O2 in heat-induced cross-adaptation to salinity, drought, chilling and heat stress was studied by Gong et al. (2001) in two cultivars of maize differing in their stress tolerance. A heat-shock pre-treatment (42°C, 4 h) following 4-h recovery at a temperature of 28°C considerably enhanced the survival of seedlings, reduced the leakage of electrolyte from the roots and the loss of coleoptile vitality of seedlings after stress was imposed. Importantly, the heat-shock pre-treatment produced an H2O2 peak in the maize seedlings. The accumulation of H2O2 lead to the generation of cross-tolerance, indicating that an early short-lived increase in endogenous H2O2 is essential for the induction of cross-adaptation, by triggering increased expression of genes encoding ROS detoxification and therefore increasing the activities of antioxidant enzymes. Hence H2O2 could have a signaling role in inducing cross-tolerance in maize seedlings.
Recent studies have shown that H2O2 originating in the same cell can induce two dissimilar kinds of responses: one that depends on the subcellular sites of H2O2 production and the other integrating H2O2 signals independently of the subcellular site of production. Several studies have shown that H2O2 is a part of the retrogate signaling mechanism, from mitochondria or chloroplasts that activate the expression of nuclear-encoded stress-responsive genes. The aforementioned evidence clearly demonstrates that H2O2 priming modulates abiotic stress tolerance in plants by modulating ROS and MG detoxification and scavenging (Gong et al., 2001; Uchida et al., 2002; Yu et al., 2003; Xu et al., 2008, 2010; Hu et al., 2009; Jing et al., 2009; Gondim et al., 2010, 2012; Liu et al., 2010a; Wang et al., 2010a; Li et al., 2011; Bhattacharjee, 2012; Chou et al., 2012; Zhang et al., 2012; Hossain and Fujita, 2013; Ashraf et al., 2014; Sathiyaraj et al., 2014; Wang et al., 2014a), by enhancing the expression of heat shock proteins (Uchida et al., 2002; Wahid et al., 2007), by enhancing GSH and AsA biosynthesis (Yu et al., 2003; Hung et al., 2007; Chao et al., 2009; Xu et al., 2010; Bai et al., 2011; Wang et al., 2014a), by enhancing proline biosynthesis (Uchida et al., 2002; Guzel and Terzi, 2013; İşeri et al., 2013; Ashfaque et al., 2014; Sathiyaraj et al., 2014; Terzi et al., 2014), by enhancing photosynthesis (Wahid et al., 2007; Ishibashi et al., 2011; Moussa and Mohamed, 2011; Liao et al., 2012; Gondim et al., 2013), by enhancing ABA biosynthesis (Abass and Mohamed, 2011; Terzi et al., 2014) and by regulating multiple stress responsive pathways and genes. Based on the above findings we propose a hypothetical model that summarizes the possible mode of action of H2O2 in planta (Figure 2). It is speculated that H2O2 priming induces a mild oxidative stress by disruption of cellular ROS homeostasis, from which develops a ROS-dependent signaling network and induces the accumulation of latent defense proteins such as ROS-scavenging enzymes and transcription factors (TFs), among others, resulting in a primed state and enhanced stress responses. Another notable effect of H2O2 is its capacity to induce the expression of TFs and genes responsible for osmolyte synthesis, e.g., proline and betaine, and activate phosphorylation cascades using mitogen-activated protein kinases (MAPKs). In the following section we will critically discuss the perception of extracellular and intracellular H2O2 by plants and how this signal transduces and modulates the abiotic stress tolerance of plants.
FIGURE 2. A hypothetical model of the influence of H2O2 on plant defense mechanisms associated with abiotic stresses. H2O2 treatment is capable of inducing abiotic stress tolerance through the development of a small oxidative burst. This burst subsequently activates a ROS-dependent signaling network, thereby enhancing the accumulation of latent defense proteins, such as ROS-scavenging enzymes and transcription factors (TFs), resulting in a primed state and an enhanced stress response (modified from Borges et al., 2014).
H2O2 can act as a signaling molecule via chemical reactions with targeted amino acids that can lead to peptide/protein modifications. It is now well established that GSH and protein cysteine (Cys) residues are particularly well suited for reactions with oxidants such as H2O2 (D’Autréaux and Toledano, 2007; Munné-Bosch et al., 2013). Cys residues in proteins are one of the most sensitive targets for ROS-mediated posttranslational modifications, and have become the focus of many ROS signaling studies. The electron-rich sulfur atom makes Cys residues the major sites of oxidative modifications within proteins (Akter et al., 2015). It is a well-recognized concept that profiling of ROS/RNS-modified proteins containing Cys can be used to help identify key redox sensors involved in signal transduction pathways (Couturier et al., 2013). Heat stress transcription factors (Hsfs) may also function as ROS-dependent redox-sensors. Hsf proteins contain a DNA binding domain, control the transcription of heat stress associated genes (Baniwal et al., 2004) and are distributed both in the cytosol (mainly in the inactive form) and in the nucleus. Once Hsfs enter the nucleus, they bind to the heat shock elements of the promoters of ROS-sensitive genes, such as the gene encoding APX. There is evidence that certain Hsfs directly sense ROS and control gene expression during oxidative stress (Miller and Mittler, 2006). For example, the transcription of genes encoding the cytosolic peroxidases APX1 and APX2 can be regulated by Hsfs, which in turn can be modulated by ROS (Mazars et al., 2009). Increased expression of Hsfs caused by exogenous H2O2 was also found to modulate salinity tolerance (Uchida et al., 2002; Wahid et al., 2007). Transgenic B. napus plants over-expressing the Arabidopsis HEAT SHOCK TRANSCRIPTION FACTORA1b showed enhanced resistance to drought (Bechtold et al., 2013).
H2O2 signaling appears to be integrated with many different signaling networks in plant cells, like Ca2+-signaling, protein kinase networks, cellular metabolic networks etc. In some cases, H2O2 and ROS accumulation was found to precede the activation of signaling, whereas in other cases H2O2 accumulation was found to be a consequence of signaling. Signal transduction components including protein kinases, such as calcium-dependent protein kinases (CDPKs) and mitogen-activated protein (MAP) kinases have been implicated in stress tolerance as well as cross-tolerance between biotic and abiotic stress responses (Wurzinger et al., 2011). MAPK cascades are important pathways in abiotic stress responses and enable extracellular stimuli to be transduced into intracellular changes (Zhou et al., 2014). A number of cellular stimuli that induce ROS (H2O2) production can also activate MAPK pathways in multiple cell types (Torres and Forman, 2003; McCubrey et al., 2006). H2O2- and ROS-responsive MAPKKK, MAPK1, MAPK4, and MAPK6 usually remain highly active under oxidative stress and redox regulation of environmental stress responses. Direct exposure of cells to exogenous H2O2 leads to activation of MAPK pathways (Dabrowski et al., 2000; Ruffels et al., 2004). The MEKK1 pathway, which was found to be highly active under oxidative stress and induced by unfavorable environmental conditions seems to be the activator of two MAPKKs (MKK1 and MKK2), that in turn activate other MAP kinases (Mittler et al., 2011). MEKK1 is thought to be needed for the activation of MAPK4 by H2O2 (Mittler et al., 2011). Similarly, MAPK12 was found to be up regulated in response to ABA and H2O2 application (Mittler et al., 2011). MKP2 is a key regulator of the MPK3 and MPK6 networks that are involved in controlling both abiotic and biotic stress responses (Jammes et al., 2009; Mittler et al., 2011). Zhou et al. (2012) reported the regulatory role of H2O2 in cold acclimation-induced chilling tolerance in tomato. Cold acclimation induces a modest increase in H2O2, RBOH1 gene expression and NADPH oxidase activity that modulates the expression and activity of ROS detoxifying enzymes and ensures stress cross-tolerance. Zhou et al. (2014) further proved the involvement of apoplastic H2O2 in modulating stress cross-tolerance. Tomato plants pre-treated with mild cold, paraquat (PQ), or drought pre-treatment modulated abiotic stress tolerance by increasing the endogenous level of H2O2 in the apoplast, which is well correlated with RBOH1 transcription. An enhanced H2O2 level was found to modulate the expression of stress and defense-related genes, increase the activities of SOD, APX, CAT, and GR, maintain higher GSH/GSSG ratio and activate MPK1/2. Their findings support the involvement of H2O2 and MPK1/2 in cross-tolerance in plants. The exogenous application of H2O2 to A. thaliana can activate the MAPK cascades that regulate ROS production and detoxification (Pan et al., 2012). In some cases, MPK3/6 responses to cadmium (Cd) treatment are mediated by the H2O2-signaling pathway, where MPK3/6 is upregulated after an accumulation of H2O2 (Liu et al., 2010b). H2O2 may also be involved in the MAP kinase 8 (MPK8) pathway, since expression of RBOHD rapidly decreases via MPK8, resulting in negative regulation of H2O2 synthesis (Takahashi et al., 2011).
Ca2+ one of the most important second messenger in the sophisticated network of plant abiotic stress signaling (Dodd et al., 2010; Wei et al., 2014) and regulation of Ca2+ homeostasis is one of the main targets of H2O2 signaling (Petrov and Van Breusegem, 2012). Regulation of guard cells during stomatal opening is one of the most widely studied processes involving H2O2-mediated Ca2+ signaling and Ca2+ is a central signaling component in guard cell responses to stimuli like ABA, ROS and NO (Allen et al., 2000; Young et al., 2006). Ca2+ homeostasis also regulates antioxidative defenses in plants. An increase in the concentration of intracellular Ca2+ causes efficient detoxification of H2O2 and involves increased levels of detoxification enzymes, including Ca2+-sensitive CAT3. Application of the Ca2+ channel blocker LaCl3, Ca2+ chelator (EGTA) or the calmodulin (CaM) inhibitor (trifluroperazine) to germinating Amaranthus seeds, causes a significant reduction in the levels of H2O2-scavenging enzymes (Bhattacharjee, 2008), strongly supporting the role of Ca2+ as a regulator of H2O2 titer in plant tissues. A rapid increase in cellular Ca2+ is considered as one of the earliest responses associated with H2O2 signaling. Wu et al. (2010) showed that spermidine oxidase-derived H2O2 regulates pollen membrane hyper-polarization-activated Ca2+ channels in order to induce pollen tube growth. In contrast, cytoplasmic Ca2+ is able to trigger changes in H2O2 levels. It is also evident that H2O2 synthesis requires a continuous cytoplasmic influx of Ca2+, which activates NADPH oxidases located at the plasma membrane (Lamb and Dixon, 1997). The Ca–CaM signaling pathway also regulates a number of different target proteins in signaling cascades, including MAP kinases. MAP kinase pathways in turn negatively regulate H2O2 synthesis by up-regulating the expression of RbohD. Thus, CDPKs are involved in tolerance to abiotic stresses (Wei et al., 2014). Treatment of tomato and wheat leaves with H2O2 increased the expression of CDPKs (Chico et al., 2002; Li et al., 2008). Costa et al. (2010) showed that CAT scavenging of H2O2 is regulated through a Ca2+-dependent pathway in the peroxisomes of Arabidopsis guard cells. Recent evidence suggests that RBOH-dependent H2O2 production might be mediated by Ca2+ homeostasis in Arabidopsis (Suzuki et al., 2011). In this case, cytoplasmic Ca+2 was shown to bind to the EF-hands of the N-terminal region of RBOH and thus promote the activation of RBOH and the production H2O2 (Takeda et al., 2008). The Ca2+ channels and transporters activated by these stimuli form specific Ca2+ signatures and changes in these Ca2+ signatures are transmitted by protein sensors that preferentially bind Ca2+. The binding of Ca2+ results in conformational changes in these protein sensors that modulate their activities or their ability to interact with other proteins, and activate the expression of downstream salt-responsive genes through a Ca2+ signaling cascade (Rentel and Knight, 2004; Dodd et al., 2010; Kudla et al., 2010; Batistic and Kudla, 2012).
WRKY and zinc finger TFs are both widely involved in the regulation of ROS-related defense genes. It was observed that the ZAT7 and ZAT12 zinc finger proteins of Arabidopsis are strongly up regulated by oxidative stress in apx knockout mutants in response to H2O2 and methyl viologen (MV) treatment (Rizhsky, 2004). ZAT10 has a dual role both as an inducer and as a repressor of ROS-responsive genes under salt, drought and osmotic stresses (Sakamoto et al., 2004; Mittler, 2006). ZAT6 positively regulates tolerance to drought, salt, and chilling stress, as well as resistance to bacterial infection, by modulating ROS levels and SA-related gene expression (Shi et al., 2014). Accumulation of NO under stressful conditions was found to initiate defense responses similar to those seen following H2O2 production (Wang et al., 2014b). NO and H2O2 are also involved in the stimulation of stomatal closure in Arabidopsis in response to ultraviolet-B exposure (He et al., 2005). Removal of H2O2 with antioxidants or inhibition of its synthesis by inhibiting NADPH oxidase activity prevents NO generation and stomatal closure. Recent evidence supports the idea that H2O2-induced synthesis of NO might be mediated by MPK6 in Arabidopsis (Wang et al., 2010b).
The links between H2O2 signaling and other signaling pathways are summarized in Figure 3. These demonstrate a complex interaction between H2O2 signaling, environmental and metabolic cues, their down-stream effects and plant development. It appears that H2O2 may be involved, in one way or another, in every aspect of plant cell signaling, development, stress responses, and stress tolerance.
FIGURE 3. A schematic representation of major signaling components and their interactions in the H2O2 signaling network and the possible outcome in plant cells (Detail in text).
Adaptation, the important responses of plants to environmental stresses, is related to environmental stimulation, signal transduction, gene expression, and cellular metabolic adjustment (Pastori and Foyer, 2002). Plants, like other living organisms, sense increased levels of ROS and react with antioxidant mechanisms, finely coordinated, and expressed, to effectively protect the organism from oxidative stress. Modulation of gene expression by H2O2 has received much attention as H2O2 is generated in response to a variety of stress stimuli and it is likely to mediate crosstalk between different signaling pathways (Bowler and Fluhr, 2000). H2O2 modulates the expression of genes involved in ROS control, signal transduction, transcriptional regulation, and protein, carbohydrate, and lipid metabolism, demonstrating the complexity of the transcriptional responses to H2O2 (Li et al., 2011). A large number of genes involved in stress perception, signal transduction, transcription, defense, and general metabolism have been identified, revealing a highly dynamic and redundant network of genes associated with enzymes involved in ROS-production and ROS-scavenging. In most cases, H2O2 seems to be positively used by plants to activate multiple stress-responsive genes that help cope with environmental changes. Genes encoding antioxidant enzymes are central players in this network and their function has profound effects in controlling excessive ROS accumulation and cellular redox balance. In contrast, ROS can also negatively regulate the expression of genes encoding antioxidant enzymes, providing a feedback mechanism that can in turn regulate ROS levels, which is a critical component in the modulation of signaling networks (Mylona and Polidoros, 2010). A number of studies have shown that manipulation of plant antioxidant defenses results in cross-tolerance to subsequent exposure of plants to conditions that cause oxidative stress (Neill et al., 2002). Short-term exposure of CAT-deficient mutants to H2O2 can trigger an increase in tolerance to subsequent severe oxidative stress, indicating that cross-tolerance is mediated by H2O2 (Vanderauwera et al., 2005). The pivotal role of peroxisomal CAT in decomposing photorespiratory H2O2 and modulating the signaling role of H2O2 was recently shown in Arabidopsis CAT2-deficient plants (Vandenabeele et al., 2004; Queval et al., 2007). Specifically, photorespiration-generated H2O2 modulates nuclear transcriptional programs influencing the expression of cytosolic, chloroplastic, and mitochondrial genes, providing additional evidence for the importance of interorganelle communication as part of a plant’s defense response.
Polidoros and Scandalios (1999) showed that high concentrations of H2O2 rapidly induced CAT and GST1 expression, indicating that oxidative stress directly induces antioxidant responses. They also showed that H2O2 induced expression of a GST gene in the leaves of maize seedlings (Polidoros et al., 2005). GST comprises of a family of nuclear-encoded enzymes involved in cellular detoxification processes following various abiotic stresses, including exposure to xenobiotics and metals (Mylona et al., 2007). Recently, Wang et al. (2013) showed that H2O2 is involved in the regulation of rice (O. sativa L.) tolerance to salt stress where exogenous H2O2 significantly enhances the activities of APX, CAT, POD, SOD, and G6PDH in a concentration-dependent manner in rice roots. GPX1 promoter analysis showed that salt induction is mediated via ROS (predominantly H2O2) in an intracellular process, whereas induction by exogenous H2O2 involves a different signaling pathway that involves NADPH oxidase. Surprisingly, the promoter of GPX1 did not respond to exogenous ABA, although GPX1 transcripts increased in response to ABA in citrus (Citrus sinensis) plants. In Arabidopsis, it was demonstrated that GPX relays the H2O2 signal to other signaling molecules, such as ABA (Dietz, 2008). Accumulation of H2O2 opens Ca2+ channels, and induces the generation of Ca2+/CaM complexes and the activation of MAPK cascades. TF encoding genes, such as ZAT10, ZAT12, and ABI4, are induced in response to various abiotic and biotic stresses. Transcriptome analysis has shown that some nuclear-encoded TF encoding genes can be induced specifically by the accumulation of a particular ROS (H2O2), while others are induced by all ROS (Scarpeci et al., 2008). Also a number of TFs have been shown to modulate other antioxidant gene responses. H2O2 also functions as a major stress signal molecule in plants and the expression of at least 1–2% of Arabidopsis genes is now known to be H2O2-dependent (Desikan et al., 2001). These gene expression studies clearly show that increased cellular H2O2 levels have a considerable impact on the transcriptome of all plant species, by changing the expression of 100s of genes. These genes are generally involved in cell wall protection, desiccation tolerance, production of ROS scavenging enzymes and DNA damage repair.
Disorganization and/or damage to chloroplast and mitochondrial ultrastructures have been reported in plants in response to excessive accumulation of ROS induced by abiotic stresses (Yamane et al., 2003; Xu et al., 2008; Liu et al., 2010a; Huang et al., 2015). The alteration of chloroplast or mitochondrial ultrastructures was correlated with higher ROS (H2O2) and MDA accumulation, as well as lower antioxidant enzyme activities under stressful conditions (Xu et al., 2008; Liu et al., 2010a; Huang et al., 2015). H2O2 pre-treatment induced stress tolerance was associated with higher antioxidant enzyme activities and lower levels of endogenous ROS accumulation (Liu et al., 2010a; Huang et al., 2015). These findings indicate that effective ROS metabolism is essential for maintaining the structural integrity of cellular organelles and keeping them fully functional in plants under abiotic stresses. H2O2-primed plants can overcome the adverse stress conditions through better ROS metabolism, as well as through the protection of cellular organelles indirectly.
Although the precise mechanisms associated with abiotic stress tolerance remain to be fully elucidated, recent studies have found that many abiotic stress responses are coordinated by complex signaling networks, involving both phytohormones and ROS, especially H2O2 (Bartoli et al., 2013; Figure 4). Here we discuss examples demonstrating that major plant hormones interact with H2O2 and other signaling pathways in modulating plant growth and abiotic stress tolerance.
FIGURE 4. A simplified schematic model showing interactions between the H2O2-transduced network and different hormonal signaling pathways in the plant cell (Adapted from Habibi, 2014).
Abscisic acid is not only an important plant hormone that regulates plant growth and development but also a global regulator that mediates abiotic stress responses. Abiotic stresses can induce an accumulation of ABA (Jia et al., 2002), moreover ABA can induce the production of ROS in plant cells and H2O2 production in guard cells plays an important role in ABA-mediated stomatal closure (Kwak et al., 2003), while NO production acts downstream of H2O2 in ABA-induced stomatal closure (Bright et al., 2006). In addition to its function in stomatal closure, ABA also plays crucial role in ROS-mediated stress tolerance. In maize, water stress or ABA treatment influences the accumulation of ROS and increases antioxidant enzyme activities (Jiang and Zhang, 2002), whereas ROS scavengers completely block the ABA-mediated increases in antioxidant activities (Zhang et al., 2006). The expression of NADPH oxidase encoding genes and H2O2 accumulation in Arabidopsis is induced immediately after exposure to salt stress or ABA treatment. H2O2 accumulation can be suppressed by an inhibitor of NADPH oxidases (DPI) and DPI-treated plants show reduced salt stress tolerance (Zhang et al., 2001; Kwak et al., 2003; Leshem et al., 2007). During ABA-mediated stress responses, MAPK cascades may act both upstream and downstream of ROS production (Zhang et al., 2006; Zhou et al., 2014). MPK9 and MPK12 were identified as downstream factors that integrate ABA-ROS signaling, leading to anion channel activation (Jammes et al., 2009). It was reported that H2O2 is an essential signal in mediating stomatal closure induced by ABA via activation of Ca-permeable channels in the plasma membrane under a variety of abiotic stresses, such as drought and salinity (Pei et al., 2000; Neill et al., 2002; Kim et al., 2010). In addition, cytokinin-deficient mutants have been found to be tolerant to drought and salinity and have ABA hypersensitivity and reduced levels of ABA (Nishiyama et al., 2011).
Jasmonic acid (JA) and methyl jasmonate (MeJA, a biologically active derivative of JA) are well-established signal molecules involved in a plant’s defense responses and are effective inducers of H2O2 accumulation in plant cell cultures (Wang and Wu, 2004, 2013). Pre-treatment of Arabidopsis and tobacco plants with JA abrogated O3-induced H2O2 accumulation, SA production, and defense gene activation (Overmyer et al., 2000; Rao et al., 2000, 2002). MeJA might also play an important role in signal transduction in grape cells, regulating the levels of NO and H2O2 and enhancing the activities of enzymes involved in phytoalexin synthesis (Wang et al., 2012). Like ABA, MeJA can induce stomatal closure mediated by ROS-dependent signaling pathways (Suhita et al., 2004). JA can also interact with ABA, ethylene (ET) and SA in oxidative stress triggered by water deficiency (Brossa et al., 2011). Increased JA accumulation under drought was found to increase the transcript abundance and activities of APX, GR, MDHAR, DHAR, and L-galactono-1,4-lactone dehydrogenase (GalLDH), as well as enhancing the contents of AsA and GSH (Shan and Liang, 2010). During water stress, transient accumulation of JA is needed for ABA increases in citrus roots (de Ollas et al., 2013).
Salicylic acid (SA) plays an important role in plant responses to various types of environmental stresses, including drought, low temperature and high salinity (Kang et al., 2014; Miura and Tada, 2014). Under stress, the levels of endogenous SA and H2O2 increase in plant cells. However, the levels H2O2 degrading enzymes, such as CAT and APX, can be suppressed by SA treatment (Yuan and Lin, 2008). Previous studies have shown that H2O2 alone is not sufficient to trigger antioxidant defense responses and cell death in cell cultures (Chen et al., 1993). However, a SA-deficient transgenic Arabidopsis line overexpressing salicylate hydroxylase (NahG) gene exposed to O3 accumulated more H2O2 compared with non-transgenic plants, yet failed to efficiently induce defense genes, such as GST1 and GPX, and perturb the redox state of glutathione (Rao et al., 2002). This indicates that H2O2 alone is not sufficient to trigger defense responses, and SA is required to maintain the cellular redox state and potentiate defense responses in O3-exposed plants (Rao and Davis, 1999). Pre-treatment with SA causes ROS accumulation (Mittler, 2002; Harfouche et al., 2008). ROS production mediated by salicylhydroxamic acid (SHAM)-sensitive guaiacol peroxidases was induced by SA in guard cells, which may be independent of the ABA pathway (Mori et al., 2001; Khokon et al., 2011). Other enzymes inhibited by SA, such as APX, CAT, and carbonic anhydrase, are also involved in scavenging ROS (Chen et al., 1993; Conrath et al., 1995; Durner and Klessig, 1995; Slaymaker et al., 2002). SA and ROS, mainly H2O2, have been proposed to form a self-amplifying feedback loop in response to abiotic and biotic stresses (Vlot et al., 2009).
Auxins have been shown to play an important role in stress-related hormonal networks. They can indirectly modulate ROS homeostasis by affecting the stability of DELLA proteins (Fu and Harberd, 2003; Weiss and Ori, 2007; Paponov et al., 2008) or directly by inducing ROS detoxification enzymes, such as GSTs and quinine reductases (Laskowski et al., 2002). Analysis of the TRANSPORT INHIBITOR RESPONSE 1 (TIR1)/AUXIN SIGNALLING F-BOX PROTEINS (AFB) auxin receptors shows that these genes are involved in tolerance to oxidative stress by regulating H2O2, antioxidant enzymes, antioxidant levels and the chlorophyll content of plants (Iglesias et al., 2010). In addition, auxin-induced H2O2 production acts as a signal for the stomatal opening response (Song et al., 2006). H2O2 is also involved in auxin-induced root geotropism (Joo et al., 2001). Any exogenous or endogenous stimulus that perturbs cellular redox balance can activate auxin homeostasis because NADPH oxidase-dependent ROS production influences polar auxin transport (Joo et al., 2001, 2005).
Gibberellic acids (GAs) mediate growth in response to environmental signals by relieving the constraints on gene expression imposed by a family of growth-repressing regulators, the DELLA proteins (Peng et al., 1999; Harberd et al., 2009). Moreover, the Arabidopsis GA-deficient ga1-3 mutants, that have higher levels of DELLA proteins, are much less susceptible to ROS-dependent cell death (Achard et al., 2008). Stress (drought or high salt) conditions enhance ABA/GA ratios favoring DELLA protein accumulation and lower ROS levels (Finkelstein et al., 2008; Considine and Foyer, 2014). ABA can decrease ROS production in rice seeds, leading to a repression of ascorbate and GA accumulation (Ye et al., 2012). Recently, it was reported that ROS are involved in GA/ABA signaling in barley aleurone cells (Ishibashi et al., 2012). In addition, GA-stimulated Arabidopsis gene GASA14 regulates leaf expansion and abiotic stress tolerance by modulating ROS accumulation (Sun et al., 2013). The overexpression of GASA4 suppressed ROS accumulation in transgenic plants and enhanced their tolerance to NO (Rubinovich and Weiss, 2010).
Ethylene can induce ROS generation and H2O2 can stimulate the expression of ET biosynthesis and responsive genes (Vandenabeele et al., 2003). The PCD pathways occurring in leaf abscission depends on NADPH oxidase-dependent H2O2 generation triggered by ET (Sakamoto et al., 2008). Moreover, stomatal closure also requires the integrated cooperation of H2O2 and ET. In Arabidopsis, the ETHYLENE RESPONSE FACTOR1 (ERF1) is greatly induced by high salinity and drought stress (Cheng et al., 2013). Induction of the salt stress response in Arabidopsis required both JA and ET signaling, but was inhibited by ABA. Transgenic plants overexpressing ERF1 showed higher salt and drought tolerance and less water loss due to transpiration (Cheng et al., 2013).
From the above discussion, it is evident that ROS production and associated redox processing are an integral part of hormone regulation and functioning in the control of plant abiotic stress responses and tolerance. Further understanding of H2O2-hormone-antioxidant interactions will help to facilitate the development of plants tolerant to various abiotic stresses.
H2O2 priming represents a fruitful area of future research, which should help plant scientists explore the molecular mechanisms associated with abiotic stress tolerance and promote a more environmental friendly sustainable agriculture. Plants have mechanisms, particularly under environmental stress, to utilize ROS, especially H2O2, for signaling purposes that confer acclamatory stress tolerance through the modulation of osmotic adjustment, ROS detoxification, photosynthetic C fixation and hormonal regulation. A large number of studies have suggested that pre-treatment of seeds or seedlings with H2O2, or the combined application of H2O2 and abiotic stress, induces an inductive pulse that helps to protect plants under abiotic stresses by restoration of redox-homeostasis and mitigation of oxidative damage to membranes, proteins and lipids and by modulating stress signaling pathways (Figures 2–4), however, the mechanisms for this are not well established. Many researchers have suggested a central role for H2O2 in intracellular and systemic signaling routes that increase tolerance and acclimation to abiotic stresses bur how H2O2 is sensed by plant cells is still a mystery, and numerous factors are still being considered with respect to their roles in H2O2-induced signal transduction and development of responses to oxidative stress. Recent findings have shown that effective ROS signaling may require an increased flux of antioxidants, notably those that are thiol-dependent. With respect to signal transduction, ROS can interact with other signaling pathways, such as the activation of NADPH oxidase dependent or monomeric G protein; lipid-derived signals; induction of MAPK; redox sensitive TFs; regulation of Ca2+; and plant hormone signal transduction. An understanding of the H2O2 physiology of plants, particularly H2O2 sensing and the identification of the components of H2O2 signaling network and H2O2 cross-talk with other growth factors, is necessary and is of great importance if we are to improve the performance of crop plants growing under conditions that cause abiotic stress.
Identification of the genetic network and of the downstream reactions modulated by H2O2 originating from specific organelles remains a future challenge. Additionally, one of challenges in H2O2 research is to identify specific H2O2 receptors and to establish how the cell is able to decode endogenous H2O2 signals and discriminate between different stimuli giving rise to a very specific defense response. Utilization of genetically engineered plants that suppress H2O2 generation or increase H2O2 production and the isolation of H2O2-signaling mutants will be invaluable in elucidating further the biological roles of H2O2 in specific cells and in the response to various abiotic stressors that cause oxidative damage. Further proteomic, metabolomic and transcriptomic studies will provide further insights into the responses of cells to H2O2. Future research is likely to find new signaling roles for H2O2 in regulating ROS scavenging systems with respect to modulating abiotic stress tolerance. A better understanding of H2O2 and its role in regulating ROS scavenging systems will be valuable in helping produce crop plants with greater levels of tolerance to oxidative stress using traditional plant breeding or biotechnological approaches. Understanding the subtle and sensitive mechanisms plants use to fine-tune H2O2 titer and the associated signaling cascades may hold the key to improving agriculture in the future.
The authors declare that the research was conducted in the absence of any commercial or financial relationships that could be construed as a potential conflict of interest.
Authors apologize if some references related to the main theme of the current review could not be cited due to space constraints.
Abass, S. M., and Mohamed, H. I. (2011). Alleviation of adverse effects of drought stress on common bean (Phaseolus vulgaris L.) by exogenous application of hydrogen peroxide. Bangladesh J. Bot. 41, 75–83.
Achard, P., Renou, J. P., Berthomé, R., Harberd, N. P., and Genschik, P. (2008). Plant DELLAs restrain growth and promote survival of adversity by reducing the levels of reactive oxygen species. Curr. Biol. 18, 656–660. doi: 10.1016/j.cub.2008.04.034
Agrawal, G. K., Iwahashi, H., and Rakwal, R. (2003). Small GTPase ‘Rop’: molecular switch for plant defense responses. FEBS Lett. 546, 173–180. doi: 10.1016/S0014-5793(03)00646-X
Akter, S., Huang, J.,Waszczak, C., Jacques, S., Gevaert, K., Van Breusegem, F., et al. (2015). Cysteines under ROS attack in plants: a proteomics view. J. Exp. Bot. 66, 2935–2944. doi: 10.1093/jxb/erv044
Allen, G. J., Chu, S. P., Schumacher, K., Shimazaki, C. T., Vafeados, D., Kemper, A., et al. (2000). Alteration of stimulus-specific guard cell calcium oscillations and stomatal closing in Arabidopsis det3 mutant. Science 289, 2338–2342. doi: 10.1126/science.289.5488.2338
Anjum, N. A., Sofo, A., Scopa, A., Roychoudhury, A., Gill, S. S., Iqbal, M., et al. (2015). Lipids and proteins-major targets of oxidative modifications in abiotic stressed plants. Environ. Sci. Pollut. Res. 22, 4099–4121. doi: 10.1007/s11356-014-3917-1
Apel, K., and Hirt, H. (2004). Reactive oxygen species: metabolism, oxidative stress, and signal transduction. Annu. Rev. Plant Biol. 55, 373–399. doi: 10.1146/annurev.arplant.55.031903.141701
Ashfaque, F., Khan, M. I. R., and Khan, N. A. (2014). Exogenously applied H2O2 promotesproline accumulation, water relations, photosynthetic efficiency and growth of wheat (Triticum aestivum L.) under salt stress. Annu. Res. Rev. Biol. 4, 105–120. doi: 10.9734/ARRB/2014/5629
Ashraf, M. A., Rasheed, R., Hussain, I., Iqbal, M., Haider, M. Z., Parveen, S., et al. (2014). Hydrogen peroxide modulates antioxidant system and nutrient relation in maize (Zea mays L.) under water-deficit conditions. Arch. Agron. Soil Sci. 61, 507–523. doi: 10.1080/03650340.2014.938644
Azevedo-Neto, A. D., Prisco, J. T., Eneas-Filho, J., Medeiros, J. V. R., and Gomes-Filho, E. (2005). Hydrogen peroxide pre-treatment induces salt stress acclimation in maize plants. J. Plant Physiol. 162, 1114–1122. doi: 10.1016/j.jplph.2005.01.007
Bai, X. J., Liu, L. J., Zhang, C. H., Ge, Y., and Cheng, W. D. (2011). Effect of H2O2 pretreatment on Cd tolerance of different rice cultivars. Rice Sci. 18, 29–35. doi: 10.1016/S1672-6308(11)60005-X
Baniwal, S. K., Bharti, K., Chan, K. Y., Fauth, M., Ganguli, A., Kotak, S., et al. (2004). Heat stress response in plants: a complex game with chaperones and more than twenty heat stress transcription factors. J. Biosci. 29, 471–487. doi: 10.1007/BF02712120
Bartoli, C. G., Casalongue, C. A., Simontacchi, M., Marquex-Garcia, B., and Foyer, C. H. (2013). Interactions between hormone and redox signalling pathways in the control of growth and cross tolerance to stress. Environ. Exp. Bot. 94, 73–88. doi: 10.1016/j.envexpbot.2012.05.003
Batistic, O., and Kudla, J. (2012). Analysis of calcium signaling pathways in plants. Biochim. Biophys. Acta 1820, 1283–1293. doi: 10.1016/j.bbagen.2011.10.012
Bechtold, U., Albihlal, W. S., Lawson, T., Fryer, M. J., Sparrow, P. A. C., Richard, F., et al. (2013). Arabidopsis HEAT SHOCK TRANSCRIPTION FACTORA1b overexpression enhances water productivity, resistance to drought, and infection. J. Exp. Bot. 64, 3467–3481. doi: 10.1093/jxb/ert185
Beckers, G. J. M., and Conrath, U. (2007). Priming for stress resistance: from the lab to the field. Curr. Opin. Plant Biol. 10, 1–7. doi: 10.1016/j.pbi.2007.06.002
Bhattacharjee, S. (2008). Calcium-dependent signaling pathway in heat-induced oxidative injury in Amaranthus lividus. Biol. Plant. 52, 1137–1140. doi: 10.1007/s10535-008-0028-1
Bhattacharjee, S. (2012). An inductive pulse of hydrogen peroxide pretreatment restores redox- homeostasis and mitigates oxidative membrane damage under extremes of temperature in two rice cultivars (Oryza sativa L., Cultivars Ratna and SR 26B). Plant Growth Regul. 68, 395–410. doi: 10.1007/s10725-012-9728-9
Bhattacharjee, S. (2013). Heat and chilling induced disruption of redox homeostasis and its regulation by hydrogen peroxide in rice (Oryza sativa L., Cultivar Ratna). Physiol. Mol. Biol. Plants 19, 199–207. doi: 10.1007/s12298-012-0159-x
Bienert, G. P., Schjoerring, J. K., and Jahn, T. P. (2006). Membrane transport of hydrogen peroxide. Biochim. Biophys. Acta 1758, 994–1003. doi: 10.1016/j.bbamem.2006.02.015
Bolwell, G. P., Bindschedler, L. V., Blee, K. A., Butt, V. S., Davies, D. R., Gardner, S. L., et al. (2002). The apoplastic oxidative burst in response to biotic stress in plants: a tree component system. J. Exp. Bot. 53, 1367–1376. doi: 10.1093/jexbot/53.372.1367
Borges, A. A., Jiménez-Arias, D., Expósito-Rodríguez, M., Sandalio, L. M., and Pérez, J. A. (2014). Priming crops against biotic and abiotic stresses: MSB as a tool for studying mechanisms. Front. Plant Sci. 5:642. doi: 10.3389/fpls.2014.00642
Bowler, C., and Fluhr, R. (2000). The role of calcium and activated oxygen as signals for controlling cross-tolerance. Trends Plant Sci. 5, 241–245. doi: 10.1016/S1360-1385(00)01628-9
Bright, J., Desikan, R., Hancock, J. T., Weir, I. S., and Neill, S. J. (2006). ABA-induced NO generation and stomatal closure in Arabidopsis are dependent on H2O2 synthesis. Plant J. 45, 113–122. doi: 10.1111/j.1365-313X.2005.02615.x
Brossa, R., Lopez-Carbonell, M., Jubany-Mari, T., and Alegre, L. (2011). Interplay between abscisic acid and jasmonic acid and its role in water-oxidative stress in wild-type, ABA-deficient, JA-deficient, and ascorbate deficient Arabidopsis thaliana plants. J. Plant Growth Regul. 30, 322–333. doi: 10.1007/s00344-011-9194-z
Chao, Y. Y., Hsu, Y. T., and Kao, C. H. (2009). Involvement of glutathione in heat shock-and hydrogen peroxide-induced cadmium tolerance of rice (Oryza sativa L.) seedlings. Plant Soil 318, 37–45. doi: 10.1007/s11104-008-9815-x
Chen, Z., Silver, H., and Klessig, D. F. (1993). Active oxygen species in the induction of plant systemic acquired resistance by salicylic acid. Science 262, 1883–1886. doi: 10.1126/science.8266079
Cheng, M. C., Liao, P. M., Kuo, W. W., and Lin, T. P. (2013). The Arabidopsis ETHYLENE RESPONSE FACTOR1 regulates abiotic stress-responsive gene expression by binding to different cis-acting elements in response to different stress signals. Plant Physiol. 162, 1566–1582. doi: 10.1104/pp.113.221911
Chico, J. M., Raíces, M., Téllez-Iñón, M. T., and Ulloa, R. M. (2002). A calcium-dependent protein kinase is systemically induced upon wounding in tomato plants. Plant Physiol. 128, 256–270. doi: 10.1104/pp.010649
Chou, T. S., Chao, Y. Y., and Kao, C. H. (2012). Involvement of hydrogen peroxide in heat shock- and cadmium-induced expression of ascorbate peroxidase and glutathione reductase in leaves of rice seedlings. J. Plant Physiol. 169, 478–486. doi: 10.1016/j.jplph.2011.11.012
Conrath, U. (2011). Molecular aspects of defence priming. Trends Plant Sci. 16, 524–531. doi: 10.1016/j.tplants.2011.06.004
Conrath, U., Chen, Z., Ricigliano, J. R., and Klessig, D. F. (1995). Two inducers of plant defense responses, 2,6-dichloroisonicotinec acid and salicylic acid, inhibit catalase activity in tobacco. Proc. Natl. Acad. Sci. U.S.A. 92, 7143–7147. doi: 10.1073/pnas.92.16.7143
Considine, M. J., and Foyer, C. H. (2014). Redox regulation of plant development. Antioxid. Redox Signal. 21, 1305–1326. doi: 10.1089/ars.2013.5665
Costa, A., Drago, I., Behera, S., Zottini, M., Pizzo, P., Schroeder, J. I., et al. (2010). H2O2 in plant peroxisomes: an in vivo analysis uncovers a Ca2+-dependent scavenging system. Plant J. 62, 760–772. doi: 10.1111/j.1365-313X.2010.04190.x
Couturier, J., Chibani, K., Jacquot, J.-P., and Rouhier, N. (2013). Cysteine-based redox regulation and signaling in plants. Front. Plant Sci. 4:105. doi: 10.3389/fpls.2013.00105
Dabrowski, A., Boguslowicz, C., Dabrowska, M., Tribillo, I., and Gabryelewicz, A. (2000). Reactive oxygen species activate mitogen activated protein kinases in pancreatic acinar cells. Pancreas 21, 376–384. doi: 10.1097/00006676-200011000-00008
Das, K., and Roychoudhury, A. (2014). Reactive oxygen species (ROS) and response of antioxidants as ROS-scavengers during environmental stress in plants. Front. Environ. Sci. 2:53. doi: 10.3389/fenvs.2014.00053
Dat, J. F., Foyer, C. H., and Scott, I. M. (1998). Changes in salicylic acid and antioxidants during induced thermotolerance in mustard seedlings. Plant Physiol. 118, 1455–1461. doi: 10.1104/pp.118.4.1455
Dat, J., Vandenabeele, S., Vranová, E., Van Montagu, M., Inzé, D., and Van Breusegem, F. (2000). Dual action of the active oxygen species during plant stress responses. Cell Mol. Life Sci. 57, 779–795. doi: 10.1007/s000180050041
D’Autréaux, B., and Toledano, M. B. (2007). ROS as signaling molecules: mechanisms that generate specificity in ROS homeostasis. Nat. Rev. Mol. Cell Biol. 8, 813–824. doi: 10.1038/nrm2256
de Carvalho, M. H. C. (2013). Drought stress and reactive oxygen species. Production, scavenging and signalling. Plant Signal. Behav. 3, 156–165. doi: 10.4161/psb.3.3.5536
de Ollas, C., Hernando, B., Arbona, V., and Gomez-Cadenas, A. (2013). Jasmonic acid transient accumulation is needed for abscisic acid increase in citrus roots under drought stress conditions. Physiol. Plant. 147, 296–306. doi: 10.1111/j.1399-3054.2012.01659.x
Desikan, R., Mackerness, S. A. H., Hancock, J. T., and Neill, S. J. (2001). Regulation of the Arabidopsis transcriptome by oxidative stress. Plant Physiol. 127, 159–172. doi: 10.1104/pp.127.1.159
Dietz, K. J. (2008). Redox signal integration: from stimulus to networks and genes. Physiol. Plant. 133, 459–468. doi: 10.1111/j.1399-3054.2008.01120.x
Dodd, A. N., Kudla, J., and Sanders, D. (2010). The language of calcium signaling. Annu. Rev. Plant Biol. 61, 593–620. doi: 10.1146/annurev-arplant-070109-104628
Durner, J., and Klessig, D. F. (1995). Inhibition of ascorbate peroxidase by salicylic acid and 2,6-dichloroisonicotinic acid, two inducers of plant defense responses. Proc. Natl. Acad. Sci. U.S.A. 92, 11312–11316. doi: 10.1073/pnas.92.24.11312
Dutilleul, C., Garmier, M., Noctor, G., Mathieu, C. D., Chétrit, P., Foyer, C. H., et al. (2003). Leaf mitochondria modulate whole cell redox homeostasis, set antioxidant capacity and determine stress resistance through altered signaling and diurnal regulation. Plant Cell 15, 1212–1226. doi: 10.1105/tpc.009464
Fedina, I. S., Nedeva, D., and Çiçek, N. (2009). Pre-treatment with H2O2 induces salt tolerance in barley seedlings. Biol. Plant. 53, 321–324. doi: 10.1007/s10535-009-0058-3
Filippou, P., Tanou, G., Molassiotis, A., and Fotopoulos, V. (2012). “Plant acclimation to environmental stress using priming agents,” in: Plant Acclimation to Environmental Stress, eds N. Tuteja and S. S. Gill (Berlin, NY: Springer Science & Business Media), 1–28.
Finkelstein, R., Reeves, W., Ariizumi, T., and Steber, C. (2008). Molecular aspects of seed dormancy. Annu. Rev. Plant Biol. 59, 387–415. doi: 10.1146/annurev.arplant.59.032607.092740
Foreman, J., Demidchik, V., Bothwell, J. H., Mylona, P., Miedema, H., Torres, M. A., et al. (2003). Reactive oxygen species produced by NADPH oxidase regulate plant cell growth. Nature 422, 442–446. doi: 10.1038/nature01485
Fu, X., and Harberd, N. P. (2003). Auxin promotes Arabidopsis root growth by modulating gibberellin response. Nature 421, 740–743. doi: 10.1038/nature01387
Gechev, T. S., and Hille, J. (2005). Hydrogen peroxide as a signal controlling plant programmed cell death. J. Cell Biol. 8, 17–20.
Gill, S. S., and Tuteja, N. (2010). Reactive oxygen species and antioxidant machinery in abiotic stress tolerance in crop plants. Plant Physiol. Biochem. 48, 909–930. doi: 10.1016/j.plaphy.2010.08.016
Gondim, F. A., Gomes-Filho, E., Costa, J. H., Alencar, N. L. M., and Priso, J. T. (2012). Catalase plays a key role in salt stress acclimation induced by hydrogen peroxide pretreatment in maize. J. Plant Physiol. Biochem. 56, 62–71. doi: 10.1016/j.plaphy.2012.04.012
Gondim, F. A., Gomes-Filho, E, Lacerda, C. F., Prisco, J. T., Neto, A. D. A., and Marques, E. C. (2010). Pretreatment with H2O2 in maize seeds: effects on germination and seedling acclimation to salt stress. Braz. J. Plant Physiol. 22, 103–112. doi: 10.1590/s1677-04202010000200004
Gondim, F. A., Miranda, R. S., Gomes-Filho, E., and Prisco, J. T. (2013). Enhanced salt tolerance in maize plants induced by H2O2 leaf spraying is associated with improved gas exchange rather than with non-enzymatic antioxidant system. Theor. Exp. Plant Physiol. 25, 251–260. doi: 10.1590/S2197-00252013000400003
Gong, M., Chen, B., Li, Z. G., and Guo, L. H. (2001). Heat-shock-induced cross adaptation to heat, chilling, drought and salt stress in maize seedlings and involvement of H2O2. J. Plant Physiol. 158, 112–1130. doi: 10.1078/0176-1617-00327
Guzel, S., and Terzi, R. (2013). Exogenous hydrogen peroxide increases dry matter production, mineral content and level of osmotic solutes in young maize leaves and alleviates deleterious effects of copper stress. Bot. Stud. 54, 26. doi: 10.1186/1999-3110-54-26
Habibi, G. (2014). “Hydrogen peroxide (H2O2) generation, scavenging and signaling in plants,” in Oxidative Damage to Plants, ed. P. Ahmad (Amsterdam: Elsevier), 557–574. doi: 10.1016/b978-0-12-799963-0.00019-8
Halliwell, B. (2006). Reactive species and antioxidants. Redox biology is a fundamental theme of aerobic life. Plant Physiol. 141, 312–322. doi: 10.1104/pp.106.077073
Hameed, A., Hussain, T., Gulzar, S., Aziz, F., Gul, B., and Khan, M. A. (2012). Salt tolerance of a cash crop halophyte Suaeda fruticosa: biochemical responses to salt and exogenous chemical treatments. Acta Physiol. Plant 34, 2331–2340. doi: 10.1007/s11738-012-1035-6
Harberd, N. P., Belfield, E., and Yasumura, Y. (2009). The angiosperm gibberellin-GID1- DELLA growth regulatory mechanism: how an “inhibitor of an inhibitor” enables flexible response to fluctuating environments. Plant Cell 21, 1328–1339. doi: 10.1105/tpc.109.066969
Harfouche, A. L., Rugini, E., Mencarelli, F., Botondi, R., and Muleo, R. (2008). Salicylic acid induces H2O2 production and endochitinase gene expression but not ethylene biosynthesis in Castanea sativa in vitro model system. J. Plant Physiol. 165, 734–744. doi: 10.1016/j.jplph.2007.03.010
Hasanuzzaman, M., Hossain, M. A., and Fujita, M. (2011a). Nitric oxide modulates antioxidant defense and the methylglyoxal detoxification system and reduces salinity-induced damage of wheat seedlings. Plant Biotechnol. Rep. 5, 353–365. doi: 10.1007/s11816-011-0189-9
Hasanuzzaman, M., Hossain, M. A., and Fujita, M. (2011b). Selenium-induced up-regulation of the antioxidant defense and methylglyoxal detoxification system reduces salinity-induced damage in rapeseed seedlings. Biol. Trace Elem. Res. 143, 1704–1721. doi: 10.1007/s12011-011-8958-4
He, J. M., Xu, H., She, X. P., Song, X. G., and Zhao, W. M. (2005). The role and the interrelationship of hydrogen peroxide and nitric oxide in the UV-B-induced stomatal closure in broad bean. Funct. Plant Biol. 32, 237–247. doi: 10.1071/fp04185
Hossain, M. A., and Fujita, M. (2013). Hydrogen peroxide priming stimulates drought tolerance in mustard (Brassica juncea L.). Plant Gene Trait. 4, 109–123.
Hossain, M. A., Hasanuzzaman, M., and Fujita, M. (2010). Up-regulation of antioxidant and glyoxalase systems by exogenous glycinebetaine and proline in mung bean confer tolerance to cadmium stress. Physiol. Mol. Biol. Plant. 16, 259–272. doi: 10.1007/s12298-010-0028-4
Hossain, M. A., Mostofa, M. G., and Fujita, M. (2013a). Cross protection by cold-shock to salinity and drought stress-induced oxidative stress in mustard (Brassica campestris L.) seedlings. Mol. Plant Breed. 4, 50–70.
Hossain, M. A., Mostofa, M. G., and Fujita, M. (2013b). Heat-shock positively modulates oxidative protection of salt and drought-stressed mustard (Brassica campestris L.) seedlings. J. Plant Sci. Mol. Breed. 2, 1–14.
Hu, Y., Ge, Y., Zhang, C., Ju, T., and Cheng, W. (2009). Cadmium toxicity and translocation in rice seedlings are reduced by hydrogen peroxide pretreatment. Plant Growth Regul. 59, 51–61. doi: 10.1007/s10725-009-9387-7
Huang, Y. W., Zhou, Z. Q., Yang, H. X., Wei, C. X., Wan, Y. Y., Wang, X. J., et al. (2015). Glucose application protects chloroplast ultrastructure in heat-stressed cucumber leaves through modifying antioxidant enzyme activity. Biol. Plant. 59, 131–138. doi: 10.1007/s10535-014-0470-1
Hung, S. H., Wang, C. C., Ivanov, S. V., Alexieva, V., and Yu, C. W. (2007). Repetition of hydrogen peroxide treatment induces a chilling tolerance comparable to cold acclimation in mung bean. J. Am. Soc. Hort. Sci. 132, 770–776.
Iglesias, M. J., Terrile, M. C., Bartoli, C. G., DIppólito, S., and Casalongué, C. A. (2010). Auxin signaling participates in the adaptative response against oxidative stress and salinity by interacting with redox metabolism in Arabidopsis. Plant Mol. Biol. 74, 215–222. doi: 10.1007/s11103-010-9667-7
İşeri, O. D., Körpe, D. A., Sahin, F. I., and Haberal, M. (2013). Hydrogen peroxide pretreatment of roots enhanced oxidative stress response of tomato under cold stress. Acta Physiol. Plant. 35, 1905–1913. doi: 10.1007/s11738-013-1228-7
Ishibashi, Y., Tawaratsumida, T., Kondo, K., Kasa, S., Sakamoto, M., Aoki, N., et al. (2012). Reactive oxygen species are involved in gibberellin/abscisic acid signaling in barley aleurone cells. Plant Physiol. 158, 1705–1714. doi: 10.1104/pp.111.192740
Ishibashi, Y., Yamaguchi, H., Yuasa, T., Inwaya-Inoue, M., Arima, S., and Zheng, S. (2011). Hydrogen peroxide spraying alleviates drought stress in soybean plants. J. Plant Physiol. 168, 1562–1567. doi: 10.1016/j.jplph.2011.02.003
Jammes, F., Song, C., Shin, D., Muncmasa, S., and Takeda, K. (2009). MAP kinases MPK9 and MPK12 are preferentially expressed in guard cells and positively regulate ROS-mediated ABA signaling. Proc. Natl. Acad. Sci. U.S.A. 106, 20520–20525. doi: 10.1073/pnas.0907205106
Janda, T., Szalai, G., and Paldi, E. (1999). Hydroponic treatment with salicylic acid decreases the effects of chilling injury in maize (Zea mays L.) plants. Planta 208, 175–180. doi: 10.1007/s004250050547
Jia, W., Wang, Y., Zhang, S., and Zhang, J. (2002). Salt-stress-induced ABA accumulation is more sensitively triggered in roots than in shoots. J. Exp. Bot. 53, 2201–2206. doi: 10.1093/jxb/erf079
Jiang, M., and Zhang, J. (2002). Involvement of plasma membrane NADPH oxidase in abscisic acid- and water stress-induced antioxidant defense in leaves of maize seedlings. Planta 215, 1022–1030. doi: 10.1007/s00425-002-0829-y
Jing, L. Z., Kui, G. Y., Hang, L. S., and Gang, B. J. (2009). Effects of exogenous hydrogen peroxide on ultrastructure of chloroplasts and activities of antioxidant enzymes in greenhouse-ecotype cucumber under drought stress. Acta Hort. Sinica. 36, 1140–1146.
Joo, J. H., Bae, Y. S., and Lee, J. S. (2001). Role of auxin-induced reactive oxygen species in root gracitropism. Plant Physiol. 126, 1055–1060. doi: 10.1104/pp.126.3.1055
Joo, J. H., Yoo, H. J., Hwang, I., Lee, J. S., Nam, K. H., and Bae, Y. S. (2005). Auxin-induced reactive oxygen species production requires the activation of phosphatidylinositol 3-kinase. FEBS Lett. 579, 1243–1248. doi: 10.1016/j.febslet.2005.01.018
Kang, G., Li, G., and Guo, T. (2014). Molecular mechanism of salicylic acid-induced abiotic stress tolerance in higher plants. Acta Physiol. Plant. 36, 2287–2297. doi: 10.1007/s11738-014-1603-z
Kang, N. J., Kang, Y. I., Kang, K. H., and Jeong, B. R. (2009). Induction of thermotolerance and activation of antioxidant enzymes in H2O2 pre-applied leaves of cucumber and tomato seedlings. J. Jpn. Soc. Hort. Sci. 78, 320–329. doi: 10.2503/jjshs1.78.320
Kapoor, D., Sharma, R., Handa, N., Kaur, H., Rattan, A., Yadav, P., et al. (2015). Redox homeostasis in plants under abiotic stress: role of electron carriers, energy metabolism mediators and proteinaceous thiols. Front. Environ. Sci. 3:13. doi: 10.3389/fenvs.2015.00013
Khokon, A. R., Okuma, E., Hossain, M. A., Munemasa, S., Uraji, M., Nakamura, Y., et al. (2011). Involvement of extracellular oxidative burst in salicylic acid- induced stomatal closure in Arabidopsis. Plant Cell Environ. 34, 434–443. doi: 10.1111/j.1365-3040.2010.02253.x
Kim, T. H., Maik, B., Hu, H. H., Noriyuki, N., and Julian, I. S. (2010) Guard cell signal transduction network advances in Understanding abscisic acid, CO2, and Ca2+ signaling. Annu. Rev. Plant Biol. 61, 56–91. doi: 10.1146/annurev-arplant-042809-112226
Kudla, J., Batistic, O., and Hashimoto, K. (2010). Calcium signals: the lead currency of plant information processing. Plant Cell 22, 541–563. doi: 10.1105/tpc.109.072686
Kwak, J. M., Mori, I. C., Pei, Z. M., Leonhardt, N., Torres, M. A., Dangl, J. L., et al. (2003). NADPH oxidase AtrbohD and AtrbohF genes function in ROS-dependent ABA signaling in Arabidopsis. EMBO J. 22, 2623–2633. doi: 10.1093/emboj/cdg277
Lamb, C., and Dixon, R. A. (1997). The oxidative burst in plant disease resistance. Annu. Rev. Plant Physiol. Plant Mol. Biol. 48, 251–275. doi: 10.1146/annurev.arplant.48.1.251
Laskowski, M. J., Dreher, K. A., Gehring, M. A., Abel, S., Gensler, A. L., and Sussex, I. M. (2002). FQR1, a novel primary auxin-response gene, encodes a flavin mononucleotide-binding quinone reductase. Plant Physiol. 128, 578–590. doi: 10.1104/pp.010581
Leshem, Y., Seri, L., and Levine, A. (2007). Induction of phosphatidylinositol 3-kinase-mediated endocytosis by salt stress leads to intracellular production of reactive oxygen species and salt tolerance. Plant J. 51, 185–197. doi: 10.1111/j.1365-313X.2007.03134.x
Li, A., Wang, X., Leseberg, C. H., Jia, J., and Mao, L. (2008). Biotic and abiotic stress responses through calcium-dependent protein kinase (CDPK) signalling in wheat (Triticum aestivum L.). Plant Signal. Behav. 3, 654–656. doi: 10.4161/psb.3.9.5757
Li, J. T., Qiu, Z. B., Zhang, X. W., and Wang, L. S. (2011). Exogenous hydrogen peroxide can enhance tolerance of wheat seedlings to salt stress. Acta Physiol. Plant. 33, 835–842. doi: 10.1007/s11738-010-0608-5
Liao, W. B., Huang, G. B., Yu, J. H., and Zhang, M. L. (2012). Nitric oxide and hydrogen peroxide alleviate drought stress in marigold explants and promote its adventitious root development. Plant Physiol. Biochem. 58, 6–15. doi: 10.1016/j.plaphy.2012.06.012
Liu, Z. J., Guo, Y. K., and Bai, J. G. (2010a). Exogenous hydrogen peroxide changes antioxidant enzyme activity and protects ultrastructure in leaves of two cucumber ecotypes under osmotic stress. J. Plant Growth Regul. 29, 171–183. doi: 10.1007/s00344-009-9121-8
Liu, X. M., Kim, K. E., Kim, K. C., Nguyen, X. C., Han, H. J., Jung, M. S., et al. (2010b). Cadmium activates Arabidopsis MPK3 and MPK6 via accumulation of reactive oxygen species. Photochemistry 71, 614–618. doi: 10.1016/j.phytochem.2010.01.005
Maruta, T., Noshi, M., Tanouchi, A., Tamoi, M., Yabuta, Y., Yoshimura, K., et al. (2012). H2O2-triggered retrograde signalling from chloroplasts to nucleus plays specific role in response to stress. J. Biol. Chem. 287, 11717–11729. doi: 10.1074/jbc.M111.292847
Maxwell, D. P., Wang, Y., and McIntosh, L. (1999). Alternative oxidase lowers mitochondrial ROS production in plant cells. Proc. Natl. Acad. Sci. U.S.A. 96, 8271–8276. doi: 10.1073/pnas.96.14.8271
Mazars, C., Bourque, S., Mithöfer, A., Pugin, A., and Ranjeva, R. (2009). Calcium homeostasis in plant cell nuclei. New Phytol. 181, 261–274. doi: 10.1111/j.1469-8137.2008.02680.x
McCubrey, J. A., Lahair, M. M., and Franklin, R. A. (2006). Reactive oxygen species-induced activation of the MAP kinase signaling pathways. Antioxid. Redox Signal. 8, 1775–1789. doi: 10.1089/ars.2006.8.1775
Miller, G., and Mittler, R. (2006). Could heat shock transcription factors function as hydrogen peroxide sensors in plants? Ann. Bot. 98, 279–288. doi: 10.1093/aob/mcl107
Miller, G., Suzuki, N., Ciftci-Yilmaz, S., and Mittler, R. (2010). Reactive oxygen species homeostasis and signalling during drought and salinity stresses. Plant Cell Environ. 33, 453–467. doi: 10.1111/j.1365-3040.2009.02041.x
Mittler, R. (2002). Oxidative stress, antioxidants and stress tolerance. Trends Plant Sci. 7, 405–410. doi: 10.1016/S1360-1385(02)02312-9
Mittler, R. (2006). Abiotic stress, the field environment and stress combination. Trends Plant Sci. 1, 15–19. doi: 10.1016/j.tplants.2005.11.002
Mittler, R., Vanderauwera, S., Suzuki, N., Miller, G., Tognetti, V. B., Vandepoele, K., et al. (2011). ROS signaling: the new wave? Trends Plant Sci. 16, 300–309. doi: 10.1016/j.tplants.2011.03.007
Miura, K., and Tada, Y. (2014). Regulation of water, salinity, and cold stress responses by salicylic acid. Front. Plant Sci. 5:4. doi: 10.3389/fpls.2014.00004
Mori, I. C., Pinontoan, R., Kawano, T., and Muto, S. (2001). Involvement of super-oxide generation in salicylic acid-induced stomatal closure in Vicia faba. Plant Cell Physiol. 42, 1383–1388. doi: 10.1093/pcp/pce176
Mostofa, M. G., Seraj, Z. I., and Fujita, M. (2014a). Exogenous sodium nitroprusside and glutathione alleviate copper toxicity by reducing copper uptake and oxidative damage in rice (Oryza sativa L.) seedlings. Protoplasma 251, 1373–1386. doi: 10.1007/s00709-014-0639-7
Mostofa, M. G., Yoshida, N., and Fujita, M. (2014b). Spermidine pretreatment enhances heat tolerance in rice seedlings through modulating antioxidative and glyoxalase systems. Plant Growth Regul. 73, 31–44. doi: 10.1007/s10725-013-9865-9
Mostofa, M. G., Hossain, M. A., and Fujita, M. (2014c). Trehalose pretreatment induces salt tolerance in rice seedlings: oxidative damage and co-induction of antioxidant defense and glyoxalase systems. Protoplsasma 252, 461–475. doi: 10.1007/s00709-014-0691-3
Mostofa, M. G., and Fujita, M. (2013). Salicylic acid alleviates copper toxicity in rice (Oryza sativa L.) seedlings by up-regulating antioxidative and glyoxalase systems. Ecotoxicology 22, 959–973. doi: 10.1007/s10646-013-1073-x
Moussa, H. R., and Mohamed, M. A. E. F. H. (2011). Role of nitric acid or H2O2 in antioxidant defense system of Pisum sativum L. under drought stress. Nat. Sci. 9, 211–216.
Munné-Bosch, S., Queval, G., and Foyer, C. H. (2013). The impact of global change factors on redox signaling underpinning stress tolerance. Plant Physiol. 161, 5–19. doi: 10.1104/pp.112.205690
Mylona, P. V., and Polidoros, A. N. (2010). “Regulation of antioxidant genes,” in: Reactive Oxygen Species and Antioxidants in Higher Plants, ed. S. Dutta Gupta (London: Science Publishers), 101–127. doi: 10.1201/9781439854082-7
Mylona, P. V., Polidoros, A. N., and Scandalios, J. G. (2007). Antioxidant gene responses to ROS-generating xenobiotics in developing and germinated scutella of maize. J. Exp. Bot. 58, 1301–1312. doi: 10.1093/jxb/erl292
Nahar, K., Hasanuzzaman, M., Alam, M. M., and Fujita, M. (2014). Exogenous glutathione confers high temperature stress tolerance in mung bean (Vigna radiata L.) by modulating antioxidant defense and methylglyoxal detoxification system. Environ. Exp. Bot. 112, 44–54. doi: 10.1016/j.envexpbot.2014.12.001
Neill, S. J., Desikan, R., Clarke, A., Hurst, R. D., and Hancock, J. T. (2002). Hydrogen peroxide and nitric oxide as signaling molecules in plants. J. Exp. Bot. 53, 1237–1247. doi: 10.1093/jexbot/53.372.1237
Nishiyama, R., Watanabe, Y., Fujita, Y., Le, D. T., and Kojima, M. (2011). Analysis of cytokinin mutants and regulation of cytokinin metabolic genes reveals important regulatory roles of cytokinins in drought, salt and abscisic acid responses, and abscisic acid biosynthesis. Plant Cell 23, 2169–2183. doi: 10.1105/tpc.111.087395
Noctor, G., Mhamdi, A., and Foyer, C. H. (2014). The roles of reactive oxygen metabolism in drought: not so cut and dried. Plant Physiol. 164, 1636–1648. doi: 10.1104/pp.113.233478
Overmyer, K., Tuominen, H., Kettunen, R., Betz, C., Langebartels, C., Sandermann, H., et al. (2000). The ozone-sensitive Arabidopsis rcd1 mutant reveals opposite roles for ethylene and jasmonate signalling pathways in regulating superoxide-dependent cell death. Plant Cell 2, 1849–1862. doi: 10.1105/tpc.12.10.1849
Pan, J., Zhang, M., Kong, X., Xing, X., Liu, Y., Zhou, Y., et al. (2012). ZmMPK17, a novel maize group D MAP kinase gene, is involved in multiple stress responses. Planta 235, 661–676. doi: 10.1007/s00425-011-1510-0
Paponov, I. A., Paponov, M., Teale, W., Menges, M., Chakrabortee, S., Murray, J. A., et al. (2008). Comprehensive transcriptome analysis of auxin responses in Arabidopsis. Mol. Plant 1, 321–337. doi: 10.1093/mp/ssm021
Pastori, G. M., and Foyer, C. H. (2002). Common components, networks, and pathways of cross-tolerance to stress. The central role of ‘redox’ and abscisic acid-mediated controls. Plant Physiol. 129, 460–468. doi: 10.1104/pp.011021
Pei, Z. M., Murata, Y., Benning, G., Thomine, S., Klusener, B., Allen, G., et al. (2000). Calcium channels activated by hydrogen peroxide mediate abscisic acid signaling in guard cells. Nature 406, 731–734. doi: 10.1038/35021067
Peng, J., Richards, D. E., Hartley, N. M., Murphy, G. P., Devos, K. M., Flintham, J. E., et al. (1999). Green revolution genes encode mutant gibberellin response modulators. Nature 400, 256–261. doi: 10.1038/22307
Petrov, V. D., and Van Breusegem, F. (2012). Hydrogen peroxide-a central hub for information flow in plant cells. AoB Plants 2012:pls014. doi: 10.1093/aobpla/pls014
Polidoros, A. N., Mylona, P. V., Pasentsis, K., Scandalios, J. G., and Tsaftaris, A. S. (2005). The maize alternative oxidase 1a (Aox1a) gene is regulated by signals related to oxidative stress. Redox Rep. 10, 71–78. doi: 10.1179/135100005X21688
Polidoros, A. N., and Scandalios, J. G. (1999), Role of hydrogen peroxide and different classes of antioxidants in the regulation of catalase and glutathione S-transferase gene expression in maize (Zea mays L.). Physiol. Plant. 106, 112–120. doi: 10.1034/j.1399-3054.1999.106116.x
Prasad, T. K., Anderson, M. D., Martin, B. A., and Stewart, C. R. (1994a). Evidence for chilling-induced oxidative stress in maize seedlings and a regulatory role for hydrogen-peroxide. Plant Cell 6, 65–74. doi: 10.1105/tpc.6.1.65
Prasad, T. K., Anderson, M. D., and Stewart, C. R. (1994b). Acclimation, hydrogen-peroxide, and abscisic-acid protect mitochondria against irreversible chilling injury in maize seedlings. Plant Physiol. 105, 619–627.
Queval, G., Issakidis-Bourguet, E., Hoeberichts, F. A., Vandorpe, M., Gakire, B., Vanacker, H., et al. (2007). Conditional oxidative stress responses in the Arabidopsis photorespiratory mutant CAT2 demonstrate that redox state is a key modulator of daylength-dependent gene expression, and define photoperiod as a crucial factor in the regulation of H2O2 -induced cell death. Plant J. 52, 640–657. doi: 10.1111/j.1365-313X.2007.03263.x
Rao, M. V., and Davis, K. R. (1999). Ozone-induced cell death occurs via two distinct mechanisms in Arabidopsis: the role of salicylic acid. Plant J. 17, 603–614. doi: 10.1046/j.1365-313X.1999.00400.x
Rao, M. V., Lee, H., Creelman, R. A., Mullet, J. E., and Davis, K. R. (2000). Jasmonic acid signalling modulates ozone-induced hypersensitive cell death. Plant Cell 12, 1633–1646. doi: 10.1105/tpc.12.9.1633
Rao, M. V., Lee, H. I., and Davis, K. R. (2002). Ozone-induced ethylene production is dependent on salicylic acid, and both salicylic acid and ethylene act in concert to regulate ozone-induced cell death. Plant J. 32, 447–456. doi: 10.1046/j.1365-313X.2002.01434.x
Rentel, M. C., and Knight, M. R. (2004). Oxidative stress-induced calcium signalling in Arabidopsis. Plant Physiol. 135, 1471–1479. doi: 10.1104/pp.104.042663
Rizhsky, L. (2004). The zinc finger protein Zat12 is required for cytosolic ascorbate peroxidase 1 expression during oxidative stress in Arabidopsis. J. Biol. Chem. 279, 11736–11743. doi: 10.1074/jbc.m313350200
Robson, C. A., and Vanlerberghe, G. C. (2002). Transgenic plant cells lacking mitochondrial alternative oxidase have increased susceptibility to mitochondria-dependent and independent pathways of cell death. Plant Physiol. 129, 1908–1920. doi: 10.1104/pp.004853
Rubinovich, R., and Weiss, D. (2010). The Arabidopsis cysteine-rich protein GASA4 promotes GA responses and exhibits redox activity in bacteria and in planta. Plant J. 64, 1018–1027. doi: 10.1111/j.1365-313X.2010.04390.x
Ruffels, J., Griffin M., and Dickenson J. M. (2004). Activation of ERK1/2, JNK and PKB by hydrogen peroxide in human SHSY5Y neuroblastoma cells: role of ERK1/2 in H2O2-induced cell death. Eur. J. Pharmacol. 483, 163–173. doi: 10.1016/j.ejphar.2003.10.032
Sakamoto, H., Maruyama, K., Sakuma, Y., Meshi, T., Iwabuchi, M., Shinozaki, K., et al. (2004). Arabidopsis Cys2/His2-type zinc-finger proteins function as transcription repressors under drought, cold, and high-salinity stress conditions. Plant Physiol. 136, 2734–2746. doi: 10.1104/pp.104.046599
Sakamoto, M., Munemura, I., Tomita, R., and Kobayashi, K. (2008). Involvement of hydrogen peroxide in leaf abscission signaling, revealed by analysis with an in vitro abscission system in Capsicum plants. Plant J. 56, 13–27. doi: 10.1111/j.1365-313X.2008.03577.x
Sathiyaraj, G., Srinivasan, S., Kim, Y. J., Lee, O. R., Balusamy, S. D. R., Khorolaragchaa, A., et al. (2014). Acclimation of hydrogen peroxide enhances salt tolerance by activating defense-related proteins in Panax ginseng CA. Meyer. Mol. Biol. Rep. 41, 3761–3771. doi: 10.1007/s11033-014-3241-3
Scarpeci, T. E., Zanor, M. I., Carrillo, N., Mueller-Roeber, B., and Valle, E. M. (2008). Generation of superoxide anion in chloroplasts of Arabidopsis thaliana during active photosynthesis: a focus on rapidly induced genes. Plant Mol. Biol. 66, 361–378. doi: 10.1007/s11103-007-9274-4
Shan, C., and Liang, Z. (2010). Jasmonic acid regulates ascorbate and glutathionemetabolism in Agropyron cristatum leaves under water stress. Plant Sci. 178, 130–139. doi: 10.1016/j.plantsci.2009.11.002
Shi, H., Wang, X., Ye, T., Cheng, F., Deng, J., Yang, P., et al. (2014). The Cysteine2/Histidine2-type transcription factor ZINC FINGER OF Arabidopsis thaliana 6 modulates biotic and abiotic stress responses by activating salicylic acid-related genes and C-REPEAT-BINDING FACTOR genes in Arabidopsis. Plant Physiol. 165, 1367–1379. doi: 10.1104/pp.114.242404
Slaymaker, D. H., Navarre, D. A., Clark, D., Del Pozo, O., Martin, G. B., and Klessig, D. F. (2002). The tobacco salicylic acid-binding protein 3 (SABP3) is the chloroplast carbonic anhydrase, which exhibits antioxidant activity and plays a role in the hypersensitive defense response. Proc. Natl. Acad. Sci. U.S.A. 99, 11640–11645. doi: 10.1073/pnas.182427699
Song, X. G., She, X. P., He, J. M., Huang, C., and Song, T. S. (2006). Cytokinin- and auxin-induced stomatal opening involves a decrease in levels of hydrogen peroxide in guard cells of Vicia faba. Funct. Plant Biol. 33, 573–583. doi: 10.1071/FP05232
Suhita, D., Agepati, S., Raghavendra, J., Kwack, J. M., and Vavasseur, A. (2004). Cytoplasmic alkalinization precedes reactive oxygen species production during methyl jasmonate- and abscisic acid-induced stomatal closure. Plant Physiol. 134, 1536–1545. doi: 10.1104/pp.103.032250
Sun, S., Wang, H., Yu, H., Zhong, C., Zhang, X., Peng, J., et al. (2013). GASA14 regulates leaf expansion and abiotic stress resistance by modulating reactive oxygen species accumulation. J. Exp. Bot. 64, 1637–1647. doi: 10.1093/jxb/ert021
Suzuki, N., Miller, G., Morales, J., Shulaev, V., Torres, M. A., and Mittler, R. (2011). Respiratory burst oxidases: the engines of ROS signaling. Curr. Opin. Plant Biol. 14, 691–699. doi: 10.1016/j.pbi.2011.07.014
Takahashi, F., Mizoguchi, T., Yoshida, R., Ichimura, K., and Shinozaki, K. (2011). Calmodulin dependent activation of MAP kinase for ROS homeostasis in Arabidopsis. Mol. Cell 41, 649–660. doi: 10.1016/j.molcel.2011.02.029
Takeda, S., Gapper, C., Kaya, H., Bell, E., Kuchitsu, K., and Dolan, L. (2008). Local positive feedback regulation determines cell shape in root hair cells. Science 319, 1241–1244. doi: 10.1126/science.1152505
Tanou, G., Filippou, P., Belghazi, M., Diamantidis, G., Fotopoulos, V., and Molassiotis, A. (2012). Oxidative and nitrosative-based signaling and associated post-translational modifications orchestrate the acclimation of citrus plants to salinity stress. Plant J. 72, 585–599. doi: 10.1111/j.1365-313X.2012.05100.x
Teng K., Li, J., Liu, L., Han, Y., Dum, Y., Zhang, J., et al. (2014). Exogenous ABA induces drought tolerance in upland rice: the role of chloroplast and ABA biosynthesis-related gene expression on photosystem II during PEG stress. Acta Physiol. Plant. 36, 2219–2227. doi: 10.1007/s11738-014-1599-4
Terzi, R., Kadioglu, A., Kalaycioglu, E., and Saglam, A. (2014). Hydrogen peroxide pretreatment induces osmotic stress tolerance by influencing osmolyte and abscisic acid levels in maize leaves. J. Plant Interact. 9, 559–565. doi: 10.1080/17429145.2013.871077
Torres, M., and Forman, H. J. (2003). Redox signaling and the MAP kinase pathways. BioFactors 17, 287–296. doi: 10.1002/biof.5520170128
Tsukagoshi, H., Busch, W., and Benfey, P. N. (2010). Transcriptional regulation of ROS controls transition from proliferation to differentiation in the root. Cell 143, 606–616. doi: 10.1016/j.cell.2010.10.020
Uchida, A., Jagendorf, A. T., Hibino, T., Takabe, T., and Takabe, T. (2002). Effects of hydrogen peroxide and nitric oxide on both salt and heat stress tolerance in rice. Plant Sci. 163, 515–523. doi: 10.1016/S0168-9452(02)00159-0
Vandenabeele, S., Van Der Kelen, K., Dat, J., Gadjev, I., Boonefaes, T., Morsa, S., et al. (2003). A comprehensive analysis of hydrogen peroxide-induced gene expression in tobacco. Proc. Natl. Acad. Sci. U.S.A. 100, 16113–16118. doi: 10.1073/pnas.2136610100
Vandenabeele, S., Vanderauwera, S., Vuylsteke, M., Rombauts, S., Langebartels, C., Seidlitz, H. K., et al. (2004). Catalase deficiency drastically affects gene expression induced by high light in Arabidopsis thaliana. Plant J. 39, 45–58. doi: 10.1111/j.1365-313X.2004.02105.x
Vanderauwera, S., Zimmermann, P., Rombauts, S., Vandenabeele, S., Langebartels, C., Gruissem, W., et al. (2005). Genome-wide analysis of hydrogen peroxide-regulated gene expression in Arabidopsis reveals a high light-induced transcriptional cluster involved in anthocyanin biosynthesis. Plant Physiol. 139, 806–821. doi: 10.1104/pp.105.065896
Vlot, A. C., Dempsey, D. M. A., and Klessig, D. F. (2009). Salicylic acid, a multi- faceted hormone to combat disease. Annu. Rev. Phytopathol. 47, 177–206. doi: 10.1146/annurev.phyto.050908.135202
Wahid, A., Perveen, M., Gelani, S., and Basra, S. M. A. (2007). Pretreatment of seed with H2O2 improves salt tolerance of wheat seedlings by alleviation of oxidative damage and expression of stress proteins. J. Plant Physiol. 164, 283–294. doi: 10.1016/j.jplph.2006.01.005
Wang, J. W., and Wu, J. Y. (2004). Involvement of nitric oxide in elicitor-induced defense responses and secondary metabolism of Taxus chinensis cells. Nitric Oxide 11, 298–306. doi: 10.1016/j.niox.2004.10.003
Wang, K. T., Zheng, Y. H., Tang, W. C., Li, T. J., Zhang, Q., and Shang, H. T. (2012). Effects of methyl jasmonate treatment on levels of nitric oxide and hydrogen peroxide and phytoalexin synthesis in postharvest grape berries. Acta Hort. Sinica. 39, 1559–1566.
Wang, L., and Wu, J. Q. (2013). The essential role of jasmonic acid in plant-herbivores interactions-using the wild tobacco nicotiana attenuate as a model. J. Genet. Genomics 40, 597–606. doi: 10.1016/j.jgg.2013.10.001
Wang, X., Hou, C., Liu, J., He, W., Nan, W., Gong, H., et al. (2013). Hydrogen peroxide is involved in the regulation of rice (Oryza sativa L.) tolerance to salt stress. Acta Physiol. Plant. 35, 891–900. doi: 10.1007/s11738-012-1132-6
Wang, Y., Li, J., Wang, J., and Li, Z. (2010a). Exogenous H2O2 improves the chilling tolerance of manilagrass and mascarenegrass by activating the antioxidative system. Plant Growth Regul. 61, 195–204. doi: 10.1007/s10725-010-9470-0
Wang, P., Du, Y., Li, Y., Ren, D., and Song, C. P. (2010b). Hydrogen peroxide-mediated activation of map kinase 6 modulates nitric oxide biosynthesis and signal transduction in Arabidopsis. Plant Cell 22, 2981–2998. doi: 10.1105/tpc.109.072959
Wang, Y., Zhang, J., Li, J. L., and Ma, X. R. (2014a). Exogenous hydrogen peroxide enhanced the thermotolerance of Festuca arundinacea and Lolium perenne by increasing the antioxidative capacity. Acta Physiol. Plant 36, 2915–2924. doi: 10.1007/s11738-014-1661-2
Wang, L., Guo, Y., Jia, L., Chu, H., Zhou, S., Chen, K., et al. (2014b). Hydrogen peroxide acts upstream of nitric oxide in the heat shock pathway in Arabidopsis seedlings. Plant Physiol. 164, 2184–2196. doi: 10.1104/pp.113.229369
Wei, S., Hu, W., Deng, X., Zhang, Y., Liu, X., Zhao, X., et al. (2014). A rice calcium-dependent protein kinase OsCPK9 positively regulates drought stress tolerance and spikelet fertility. BMC Plant Biol. 14:133. doi: 10.1186/1471-2229-14-133
Weiss, D., and Ori, N. (2007). Mechanisms of cross talk between gibberellin and other hormones. Plant Physiol. 144, 1240–1246. doi: 10.1104/pp.107.100370
Wu, J., Shang, Z., Wu, J., Jiang, X., Moschou, P. N., Sun, W., et al. (2010). Spermidine oxidase-derived H2O2 regulates pollen plasma membrane hyperpolarization-activated Ca2+-permeable channels and pollen tube growth. Plant J. 63, 1042–1053. doi: 10.1111/j.1365-313X.2010.04301.x
Wurzinger, B., Mair, A., Pfister, B., and Teige, M. (2011). Cross-talk of calcium-dependent protein kinase and MAP kinase signaling. Plant Signal. Behav. 6, 8–12. doi: 10.4161/psb.6.1.14012
Xu, F. J., Jin, C. W., Liu, W. J., Zhang, Y. S., and Lin, X. Y. (2010). Pretreatment with H2O2 alleviates aluminum-induced oxidative stress in wheat seedlings. J. Integr. Plant Biol. 54, 44–53.
Xu, P. L., Guo, Y. K., Bai, J. G., Shang, L., and Wang, X. J. (2008). Effects of longterm chilling on ultrastructure and antioxidant activity in leaves of two cucumber cultivars under low light. Physiol. Plant. 132, 467–478. doi: 10.1111/j.1399-3054.2007.01036.x
Yamane, K., Kawasaki, M., Taniguchi, M., and ans Miyake, H. (2003). Differential effect of NaCl and polyethylene glycol on the ultrastructure of chloroplasts in rice seedlings. J. Plant Physiol. 160, 573–575. doi: 10.1078/0176-1617-00948
Ye, N., Jia, L., and Zhang, J. (2012). ABA signal in rice under stress conditions. Rice 5, 1–9. doi: 10.1186/1939-8433-5-1
Yildiz, M., Terzi, H., and Bingül, N. (2013). Protective role of hydrogen peroxide pretreatment on defense systems and BnMP1 gene expression in Cr(VI)-stressed canola seedlings. Ecotoxicology 22, 1303–1312. doi: 10.1007/s10646-013-1117-2
Young, J. J., Mehta, S., Israelsson, M., Godoski, J., Grill, E., and Schroeder, J. I. (2006). CO2 signaling in guard cells: calcium sensitivity response modulation, a Ca2+-independent phase, and CO2 insensitivity of the gca2 mutant. Proc. Natl. Acad. Sci. U.S.A. 103, 7506–7511. doi: 10.1073/pnas.0602225103
Yu, C. W., Murphy, T. M., and Lin, C. H. (2003). Hydrogen peroxide-induces chilling tolerance in mung beans mediated through ABA-independent glutathione accumulation. Funct. Plant Biol. 30, 955–963. doi: 10.1071/FP03091
Yuan,S., and Lin, H. H. (2008). Role of salicylic acid in plant abiotic stress. Z. Naturforsch C 63, 313–320. doi: 10.1515/znc-2008-5-601
Zhang, A., Jiang, M., Zhang, J., Tan, M., and Hu, X. (2006). Mitogen-activated protein kinase is involved in abscisic acid-induced antioxidant defense and acts downstream of reactive oxygen species production in leaves of maize plants. Plant Physiol. 141, 475–487. doi: 10.1104/pp.105.075416
Zhang, J., Wang, Y., Yang, H. F., and Li, J. L. (2012). The improvement of thermotolerance in tall fescue and perennial ryegrass by activating the antioxidative system. Adv. Mat. Res. 610–613, 249–253. doi: 10.4028/www.scientific.net/AMR.610-613.249
Zhang, X., Zhang, L., Dong, F., Gao, J., Galbraith, D. W., and Song, C.-P. (2001). Hydrogen peroxide is involved in abscisic acid-induced stomatal closure in Vicia faba. Plant Physiol. 126, 1438–1448. doi: 10.1104/pp.126.4.1438
Zhou, J., Wang, J., Shi, K., Xia, X. J., Zhou, Y. H., and Yu, J. Q. (2012). Hydrogen peroxide is involved in the cold acclimation-induced chilling tolerance of tomato plants. Plant Physiol. Biochem. 60, 141–149. doi: 10.1016/j.plaphy.2012.07.010
Keywords: hydrogen peroxide, abiotic stress, oxidative stress, priming, stress tolerance
Citation: Hossain MA, Bhattacharjee S, Armin S-M, Qian P, Xin W, Li H-Y, Burritt DJ, Fujita M and Tran L-SP (2015) Hydrogen peroxide priming modulates abiotic oxidative stress tolerance: insights from ROS detoxification and scavenging. Front. Plant Sci. 6:420. doi: 10.3389/fpls.2015.00420
Received: 12 March 2015; Accepted: 25 May 2015;
Published online: 16 June 2015
Edited by:
Zuhua He, Shanghai Institutes for Biological Sciences – Chinese Academy of Sciences, ChinaReviewed by:
Vasileios Fotopoulos, Cyprus University of Technology, CyprusCopyright © 2015 Hossain, Bhattacharjee, Armin, Qian, Xin, Li, Burritt, Fujita and Tran. This is an open-access article distributed under the terms of the Creative Commons Attribution License (CC BY). The use, distribution or reproduction in other forums is permitted, provided the original author(s) or licensor are credited and that the original publication in this journal is cited, in accordance with accepted academic practice. No use, distribution or reproduction is permitted which does not comply with these terms.
*Correspondence: Mohammad A. Hossain, Department of Genetics and Plant Breeding, Bangladesh Agricultural University, Mymensingh-2202, Bangladesh,YW53YXJncGJAYmF1LmVkdS5iZA==; Lam-Son P. Tran, Signaling Pathway Research Unit, RIKEN Center for Sustainable Resource Science, 1-7-22 Suehiro-cho, Yokohama 230-0045, Japan,c29uLnRyYW5AcmlrZW4uanA=
Disclaimer: All claims expressed in this article are solely those of the authors and do not necessarily represent those of their affiliated organizations, or those of the publisher, the editors and the reviewers. Any product that may be evaluated in this article or claim that may be made by its manufacturer is not guaranteed or endorsed by the publisher.
Research integrity at Frontiers
Learn more about the work of our research integrity team to safeguard the quality of each article we publish.