- 1Department of Biology, Genetics Institute, Plant Molecular and Cellular Biology Program, University of Florida, Gainesville, FL, USA
- 2Department of Biology, Pennsylvania State University, PA, USA
- 3Department of Vegetable Research, Institute of Plant Sciences, Agricultural Research Organization, Bet-Dagan, Israel
- 4Interdisciplinary Center for Biotechnology Research, University of Florida, Gainesville, FL, USA
Guard cells represent a unique single cell-type system for the study of cellular responses to abiotic and biotic perturbations that affect stomatal movement. Decades of effort through both classical physiological and functional genomics approaches have generated an enormous amount of information on the roles of individual metabolites in stomatal guard cell function and physiology. Recent application of metabolomics methods has produced a substantial amount of new information on metabolome control of stomatal movement. In conjunction with other “omics” approaches, the knowledge-base is growing to reach a systems-level description of this single cell-type. Here we summarize current knowledge of the guard cell metabolome and highlight critical metabolites that bear significant impact on future engineering and breeding efforts to generate plants/crops that are resistant to environmental challenges and produce high yield and quality products for food and energy security.
Introduction
Guard cells as a unique plant single cell-type perform many functions essential to plant growth and survival. Each pair of guard cells and the regulated pore they enclose, known as a stoma or stomate, provides a conduit for atmospheric photosynthetic gas exchange (CO2 uptake and O2 release) and transpirational release of water (H2O) in terrestrial plants, in addition to defense against pathogenic invasion. Stomatal opening and closing, in which the guard cells actively increase and decrease their volume via turgor changes to regulate the pore size in response to environmental stimuli, are vital processes in maintaining the balance of H2O loss and CO2 fixation. While drought stress induces stomatal closure, pathogens exploit stomatal opening to facilitate entry into the leaf (Zeng and He, 2010). Abscisic acid (ABA), CO2 and blue light mediated stomatal movements have generated tremendous interest in their signaling mechanisms. Each pathway/network has unique components such as distinct receptors and early signaling elements. They also have common components, for example, actual stomatal movement is caused by water influx/efflux mostly driven by solute fluxes through plasma membrane anion channels and K+ channels. When the concentrations of solutes decrease in guard cells in the cases of ABA and elevated CO2, water potential increases in the cells and water flows out, causing a decrease of turgor pressure and closure of the pores. Blue light activates H+-ATPases and resultant membrane hyperpolarization drives K+ influx, leading to decreased water potential, increased turgor pressure, and stomatal opening. Please refer to excellent articles published over the years on these signaling mechanisms (e.g., Zeiger and Zhu, 1998; Schroeder et al., 2001; Yu and Assmann, 2014; Zhang et al., 2014; Tian et al., 2015). Although stomatal movements in response to ABA, CO2, and blue light are well studied, the metabolome of the guard cell is far from catalogued. Endeavors in metabolomic approaches have led to deeper understanding of the biology inherent to several specialized important single-cell types including guard cells (Misra et al., 2014). Recent efforts to comprehend the guard cell metabolome (Jin et al., 2013) and systems biology approaches to identify the critical regulators in stomatal movement (Sun et al., 2014) have provided interesting leads into the intricate regulation of stomatal movement in response to environmental stimuli. Ongoing systems biology approaches, combining modeling and high-throughput experiments, will help to elucidate the mechanisms underlying stomatal control and unravel targets for modulation of stomatal responses to environment (Medeiros et al., 2015).
Many factors pose immense challenges to global food and bioenergy security, including population growth, climate, and environmental changes coupled to land degradation and changes in hydrological resources, essential ecosystem services, and agricultural production systems. Urgent efforts are needed to enhance the resilience of crops to the adverse effects of climate change. Stomata are highly responsive to hormonal and environmental cues, including those associated with climate change: water availability, temperature, and CO2 concentrations. Thus, understanding the basic biology, the concealed information content, and the connection to functional output of guard cells through multiple -omics approaches such as transcriptomics (Wang et al., 2011), proteomics (Zhao et al., 2008; Zhu et al., 2014) and metabolomics (Jin et al., 2013) is highly relevant to the goal of improving crop productivity and yield in ever changing climatic regimens. Here we briefly review collective efforts to unravel the functional guard cell metabolome (Figure 1), to discover metabolites of convergence and divergence among various environmental cues, to examine the molecular mechanisms of guard cell metabolic regulation, and ultimately to highlight the potential of guard cell biology in harnessing possible solutions for global food and bioenergy security.
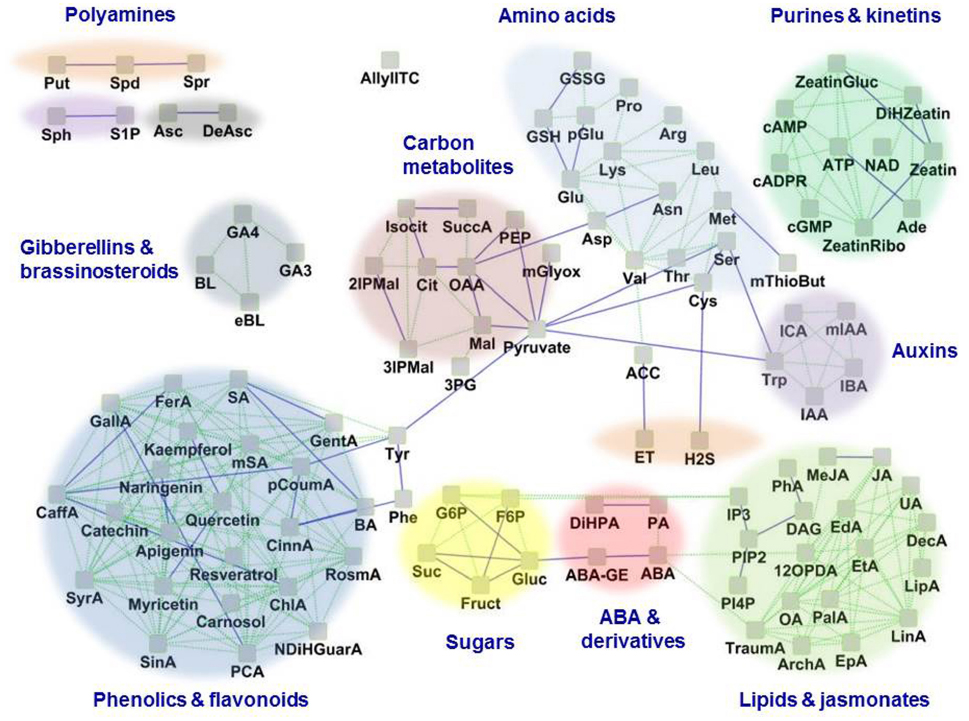
Figure 1. The guard cell metabolome. Based on available literature, 109 known metabolites in guard cells are represented as a network based on their structural and biochemical relationships. Solid blue lines represent KEGG pathway-based biochemical relatedness and green dotted lines represent the Tanimoto structural-index based relatedness, which were inferred using the MetaMapR tool (http//dgrapov.github.io/MetaMapR/) and were visualized using Cytoscape (http://www.cytoscape.org/). The clusters of metabolites are highlighted based on metabolic categorization. Abbreviations of metabolites are as follows: ABA, Abscisic acid; ABA-GE, Abscisic acid glucose ester; ACC, 1-Amino cyclopropane-1-carboxylic acid; Ade, Adenine; ArchA, Arachidonic acid; Arg, L-Arginine; Asc, Ascorbic acid; Asn, L-Asparagine; Asp, L-Aspartic acid; ATP, Adenosine triphosphate; AllylITC, Allylisothiocyanate; BA, Benzoic acid; BL, Brassinolide; cADPR, Cyclic adenosine diphosphate ribose; CaffA, Caffeic acid; cAMP, Cyclic adenosine monophosphate; ChlA, Chlorogenic acid; CinnA, trans-Cinnamic acid; Cit, Citric acid; Cys, L-Cysteine; DAG, 1,2-Diacylglycerol; DAsc, Dehydroascorbate; DecA, Decanoic acid; DiHPA, Dihydrophaseic acid; eBL, Epibrassinolide; EdA, Eicosadienoic acid; EpA, Eicosapentaenoic acid; ET, Ethylene; EtA, Eicosatrienoic acid; FerA, Ferulic acid; F6P, Fructose-6-phosphate; Fruct, Fructose; GallA, Gallic acid; GA3, Gibberellic acid A3; GA4, Gibberellic acid A4; GentA, Gentisic acid; Glu, L-Glutamic acid; Gluc, Glucose; G6P, Glucose-6-phosphate; GSH, Glutathione (reduced); GSSG, Glutathione (oxidized); His, L-Histidine; H2S, Hydrogen sulfide; IAA, Indole-3-acetic acid; IBA, Indole-3-butyric acid; ICA, Indole-3-carboxylic acid; I3P, Inositol-1, 4, 5-trisphosphate; 2IPMal, 2-Isopropylmalic acid; 3IPMal, 3-Isopropylmalic acid; Isocit, DL-Isocitric acid; JA, Jasmonic acid; Leu, L-Leucine; LinA, Linoleic acid; LipA, α-Lipoic acid; Lys, L-Lysine; Mal, L-Malic acid; MeJA, Methyl jasmonate; Met, L-Methionine; mGlyox, Methylglyoxal; mIAA, Methyl indole-3-acetate; mSA, Methyl salicylate; mThioBut, α-keto-γ-(methyl-thio) Butyric acid; mSA, Methyl salicylic acid; NAD, Nicotinamide adenine dinucleotide; NDiHGuarA, Nor dihydro guaiaretic acid; OA, Oleic acid; OAA, Oxalo acetic acid; OlAEE, Oleic acid ethyl ester; 12OPDA, 12-oxophytodienoic acid; cGMP, Guanosine-3′,5′-cyclic monophosphate; PA, Phaseic acid; PalA, Palmitic acid; PCA, Protocatechuic acid; pCoumA, p-Coumaric acid; PEP, Phosphoenolpyruvate; 3PG, 3-Phosphoglycerate; PhA, Phosphatidic acid; pGlu, L-Pyroglutamic acid; Phe, L-Phenylalanine; Pinitol, D-Pinitol; PI3P, Phosphatidylinositol-3-phosphate; PI4P, Phosphatidylinositol-4-phosphate; PIP2, Phosphatidylinositol-4, 5-bisphosphate; Pro, L-Proline; Put, Putrescine; RosmA, Rosmarinic acid; SA, Salicylic acid; Ser, L-Serine; SinA, Sinapinic acid; S1P, Sphingosine-1-phosphate; Sph, D-erythro-Sphingosine; Spd, Spermidine; Spr, Spermine; Suc, Sucrose; SuccA, Succinic acid; SyrA, Syringic acid; Thr, L-Threonine; TraumA, Traumatic acid; Trp, L-Tryptophan; Tyr, L-Tyrosine; UA, Undecanoic acid; Val, L-Valine; Zeatin, trans-Zeatin; ZeatinGluc, trans-Zeatin glucoside; ZeatinRibo, trans-Zeatin riboside.
Primary Metabolites of Carbon Metabolism in Regulatory Roles of Stomatal Function
Early hypotheses regarding guard cell osmoregulation suggested that sugar generated from starch degradation at dawn is the primary osmolyte that opens stomata (Lloyd, 1908). Upon the discovery that potassium (K+) ions, with chloride (Cl–) and malate2– as counter anions, are osmolytes that open stomata, a role for sugar in guard cell osmoregulation and stomatal movement was abandoned for several decades (Imamura, 1943; Yamashita, 1952; Fischer, 1968; Humble and Raschke, 1971; Allaway, 1973; Outlaw and Lowry, 1977; Asai et al., 2000). A later study reporting that blue and red (photosynthetic) light can open stomata and are followed by sucrose accumulation in guard cells revived the hypothesis of sucrose as an osmolyte that opens stomata (Talbott and Zeiger, 1993). A correlation between the decline of K+ content in guard cells in the middle of the day concomitantly with an increase in sucrose content further suggested that sucrose is an osmolyte that replaces K+ and maintains stomatal opening (Amodeo et al., 1996; Talbott and Zeiger, 1996).
The origin of sucrose in guard cells is not yet clear. Potentially, sucrose could be obtained from guard cell starch degradation, guard cell photosynthesis, or import from mesophyll cells (Gotow et al., 1988; Talbott and Zeiger, 1988; Lawson et al., 2014). It is generally accepted though, that the contribution of sucrose produced from guard cell photosynthesis to the osmotic requirement for stomatal opening is minimal and that most of the sugar or the organic compounds from which sugar can be synthesized is obtained from the mesophyll cells (Outlaw, 1989; Reckmann et al., 1990). When exported out of the mesophyll cells for phloem loading, some sucrose accumulates in the guard cell apoplast (Lu et al., 1995, 1997; Outlaw and De Vlieghere-He, 2001). As a result, the concentration of sucrose in the guard cell apoplast increases as photosynthesis proceeds. This sucrose may be imported by guard cells and contribute to guard cell osmolarity and stomatal opening. But it also has been proposed that as sucrose accumulates in the apoplast, its osmotic effect drives water efflux from guard cells, resulting in a decrease in stomatal apertures in a mechanism that thus inversely coordinates photosynthesis and transpiration rates (Lu et al., 1997; Outlaw and De Vlieghere-He, 2001).
Apoplastic sucrose may enter the guard cells either via sucrose transporters, or via guard cell hexose transporters following sucrose cleavage by apoplastic invertase to yield the hexoses glucose and fructose (Stadler et al., 2003; Weise et al., 2008; Bates et al., 2012). Regardless of its origin, mesophyll or guard cells, sucrose must be cleaved to be metabolized, and the hexoses obtained from sucrose cleavage, glucose and fructose, must be phosphorylated by intracellular hexose phosphorylating enzymes, hexokinases (HXK) and fructokinases (FRK; Dennis and Blakeley, 2000). Glucose can be phosphorylated only by HXK, an enzyme demonstrated to exist in guard cells and to participate in sugar sensing (Moore et al., 2003; Rolland et al., 2006; Granot, 2008). A recent study has shown that sugars such as sucrose, glucose, and fructose, do not exert an apoplastic osmotic effect on guard cells, but rather are sensed within guard cells by HXK to stimulate stomatal closure, thus coordinating photosynthesis and sugar levels with transpiration (Kelly et al., 2013).
The phosphorylated hexoses (hexose-P) within guard cells may be converted to starch or enter glycolysis and the tricarboxylic acid (TCA) cycle to yield energy (ATP) and various metabolites including pyruvate and malate that regulate stomatal movement (Allaway, 1973; Pearson, 1973; Outlaw and Lowry, 1977). Glycolysis is a central metabolic pathway for cellular respiration and generation of energy in the form of ATP. In the glycolytic pathway, 2,3-biphosphoglycerate-independent phosphoglycerate mutase (iPGAM) catalyzes the interconversion of 3-phosphoglycerate to 2-phosphoglycerate. Arabidopsis thaliana double mutants of iPGAM genes show hyposensitivity in blue light, ABA, and low CO2 regulated stomatal movements, confirming a role of glycolysis in guard cell function (Zhao and Assmann, 2011). ABA inhibition of stomatal opening in Commelina benghalensis is reversed by exogenous ATP and pyruvate (Raghavendra et al., 1976), suggesting a role of pyruvate in negative regulation of ABA signaling (Yu and Assmann, 2014). Recently, it was established that a putative mitochondrial pyruvate importer, NRGA1, negatively regulates ABA inhibition of K+ inward channels, ABA activation of slow anion channels and drought tolerance in A. thaliana (Li et al., 2014). Altogether, these findings suggest that accumulation of pyruvate in mitochondria would oppose stomatal closure.
Malate, an osmolyte that contributes to stomatal opening, can be generated from hexoses and phosphorylated hexoses obtained from guard cell starch degradation or from triose-phosphates produced in guard cell chloroplasts and exported to the cytoplasm where triose-P metabolism yields malate among other metabolites. ABA-stimulated stomatal closure is accompanied by malate disposal through release, gluconeogenesis, or consumption in the TCA cycle, supporting the role of malate as an osmolyte that opens stomata (Dittrich and Raschke, 1977). In the guard cell cytosol, malate can be metabolized into oxaloacetate (OAA) by malate dehydrogenase. Subsequently, phosphoenolpyruvate carboxykinase (PEPCK) can catalyze the production of PEP from OAA that in turn would enter into gluconeogenesis. An isoform of PEPCK, PCK1, is expressed in A. thaliana guard cells according to three experimental approaches: PCK1 gene promoter analysis and analyses of the proteome, and transcriptome of guard cell protoplasts (Leonhardt et al., 2004; Penfield et al., 2012; and Zhao et al., 2008). Loss-of-function PCK1 plants (pck1-2) show hyposensitivity in response to dark-induced (but not ABA-induced) stomatal closure, indicating the importance of malate metabolism for some stomatal responses (Penfield et al., 2012). Malate produced in photosynthetic tissues may also arrive at and enter the guard cells through malate transporters (Lee et al., 2008). Mesophyll-produced malate also coordinates stomatal behavior with mesophyll photosynthesis, as increasing apoplastic malate activates anion channels that reduce stomatal aperture (Hedrich and Marten, 1993; Fernie and Martinoia, 2009; Araujo et al., 2011). In addition, methylglyoxal, an oxygenated short aldehydic glycolytic intermediate, can induce stomatal closure in A. thaliana accompanied by extracellular reactive oxygen species (ROS) production mediated by SHAM-sensitive peroxidases, intracellular ROS accumulation, and suppression of free cytosolic (Ca2+) oscillations (Hoque et al., 2012). These results indicate a strong interconnectivity between central carbon metabolism and ABA signaling in guard cells.
Reactive Oxygen Species Related Metabolites in Guard Cell Signaling
Reactive oxygen species and nitric oxide (NO) are central components of the signaling network regulating stomatal movement in response to ABA, jasmonic acid (JA), darkness, UV, pathogen, and high CO2 concentrations (Zhang et al., 2001; Desikan et al., 2004, 2006; Zhu et al., 2012; Akter et al., 2013; He et al., 2013; Joudoi et al., 2013; Ou et al., 2014). Upon application of NO-releasing compounds, NO induces dose-dependent stomatal closure. In contrast, NO has also been implicated as a key component in negative feedback regulation of ABA guard cell signaling through S-nitrosylation of OST1 at cysteine 137 and subsequent inactivation of kinase activity that in turn blocks the positive regulatory role of OST1 in ABA signaling (Wang et al., 2015). NO-mediated negative feedback regulation may prevent complete stomatal closure, allowing some basal level of CO2 uptake and photosynthesis. Hydrogen peroxide (H2O2) may also elicit stomatal movement in a similar manner through redox modification of guard cell signaling components. However, experimental data are lacking for this hypothesis. In addition, ascorbic acid (Asc) and glutathione (GSH) are critical in maintaining cellular ROS levels and redox homeostasis (Noctor and Foyer, 1998). Asc is a key antioxidant that scavenges ROS including H2O2. Dehydroascorbate reductase (DHAR) is the key regulatory enzyme that catalyzes the generation of Asc (reduced form) from dehydroascorbate (DAsc, oxidized form) in a reaction that requires GSH. Tobacco DHAR overexpression lines that have elevated levels of reduced Asc in guard cells show hyposensitivity in stomatal response to ABA and H2O2 and these plants are drought susceptible. In contrast, DHAR antisense tobacco lines show drought tolerance (Chen and Gallie, 2004). These findings indicate that Asc redox state plays an important regulatory role in ABA and H2O2 mediated stomatal responses. Altered redox state and stomatal aperture in mutants defective in GSH synthesis are well established (Okuma et al., 2011; Munemasa et al., 2013). Negative regulation of methyl jasmonate (MeJA)-induced stomatal closure by GSH in A. thaliana has been demonstrated (Akter et al., 2013). In addition, GSH peroxidases are known to function as redox transducers as well as scavengers in ABA-mediated stress responses (Miao et al., 2006). Thus, understanding redox changes and their regulation and coordination with stomatal functions would provide new insights into guard cell signaling networks.
Stomatal guard cells have a thick cuticular layer containing high concentrations of wax-bound phenolics that provide protection against UV radiation (Karabourniotis et al., 2001; He et al., 2013) and form a constitutive defense barrier against pathogens and insects. Intracellular phenolics and flavonoids synthesized from the phenylpropanoid pathway are also responsible for cellular defense and pigmentation among other functions. Flavonoids protect plants from UV-B irradiation (Li et al., 1993) and also function as stress-induced antioxidants (Dixon and Paiva, 1995). Flavonols accumulate in guard cells of A. thaliana, but not in the surrounding pavement cells (Watkins et al., 2014). Enhanced flavonol content and decreased ROS levels upon ethylene (ET) treatment in guard cells were correlated with a reduction in the rate of stomatal closure in response to ABA. The results suggest that flavonols may quench the ABA-dependent ROS burst (Watkins et al., 2014). Moreover, some flavonoids, such as quercetin, apigenin, and kaempferol, have functions similar to synthetic auxin transport inhibitors, so changes in the synthesis or deposition of specific flavonoids within cells may act to change the rate or direction of auxin transport (Winkel-Shirley, 2002). Given that ABA reduces guard cell auxin concentrations (Jin et al., 2013), it would be interesting to further investigate the interrelationships between flavonoids, ROS, ABA, and guard cell auxin transport.
Role of Lipid Signaling in Stomatal Movement and Development
Lipids are essential for membrane formation and energy storage. In addition, lipids and their metabolites are also important cellular signaling molecules, including in stomatal regulation. For instance, lipid-based secondary messengers that positively regulate guard cell ABA signaling and stomatal closure include phosphatidic acid (PhA), phosphatidyl-inositol-3-phosphate (PI3P), inositol-1,4,5-trisphosphate (IP3), inositol-6-phosphate (IP6), and sphingolipids (Kim et al., 2010; Figure 1).
Phosphoinositides play important roles in guard cell signaling. Phospholipase C (PLC) hydrolyses phosphatidylinositol 4,5-bisphosphate (PIP2) to produce 1,2-diacylglycerol (DAG) and IP3. ABA induced the production of IP3 in Vicia faba guard cells (Lee et al., 1996), and cytosolic Ca2+ elevation and subsequent stomatal closure occurred upon experimental elevation of cytosolic IP3 in Commelina communis guard cells (Gilroy et al., 1990). Increases in guard cell PI3P and PI 4-phosphate (PI4P; the products of PI 3-kinase (PI3K) and PI 4-kinase (PI4K) activities, respectively) induce stomatal closure mediated by ABA-induced ROS generation (Jung et al., 2002; Park et al., 2003). IP6 is generated in guard cells in response to ABA. IP6 is an endomembrane-acting Ca2+-release signal that inhibits the inwardly rectifying K+ channel, which would then inhibit stomatal opening (Lemtiri-Chlieh et al., 2003).
PhA, a product of phospholipase Dα1 (PLDα1) activity, is a positive regulator in ABA-induced ROS and NO production that promotes stomatal closure (Zhang et al., 2009). In A. thaliana guard cells, NO synthesis is positively regulated by both ABA and ROS, and interaction of PhA with the two NADPH oxidases, AtrbohD and AtrbohF (Kwak et al., 2003), is necessary for ABA-induced ROS production (Zhang et al., 2009). The NADPH oxidase-deficient double mutant atrbohD/F shows impaired ABA induction of NO production and stomatal closure, indicating that ROS production is necessary for NO production. Application of NO scavengers can inhibit ROS-mediated stomatal closure, indicating that NO is required for ROS-promoted stomatal closure. In contrast, application of NO cannot induce ROS production in A. thaliana guard cells (Bright et al., 2006). These findings indicate that PhA functions upstream of ROS production and ROS function upstream of NO production.
The lipid metabolite sphingosine-1-phosphate (S1P) is a product of sphingosine kinase (SPHK) activity, which uses the long-chain amine alcohol sphingosine as a substrate. S1P induced increases of cytosolic (Ca2+) (Ng et al., 2001) and stimulated stomatal closure in C. communis and A. thaliana (Ng et al., 2001, Coursol et al., 2003). The A. thaliana genome encodes two functional SPHK genes, SPHK1 and SPHK2 (Worrall et al., 2008; Guo et al., 2011). Both SPHKs can use sphingosine and phyto-sphingosine as substrates to produce S1P and phyto-S1P, respectively. Both S1P and phyto-S1P induce stomatal closure in A. thaliana (Coursol et al., 2005). S1P inhibits inward K+ channels and promotes slow anion channel activity in A. thaliana guard cell protoplasts, which in turn cause inhibition of stomatal opening and promotion of stomatal closure, respectively (Coursol et al., 2003). In A. thaliana, a functional G-protein α-subunit (GPA1) is required for S1P regulation of ion channels (Coursol et al., 2003). In A. thaliana, PhA interacts with SPHKs, promoting substrate binding, which in turn increases SPHK activity. Phyto-S1P induces PhA production in wild type (WT) A. thaliana, but not in the pldα mutant, indicating a positive regulatory role of phyto-S1P in PLDα-mediated PhA production. It has been suggested that phyto-S1P promotes PLDα activity by increasing cytoplasmic Ca2+ concentration (Guo and Wang, 2012). These findings indicate that phyto-S1P and PhA are dependent on each other via positive feedback regulation.
A guard cell-specific and ABA-independent oxylipin pathway was recently reported (Montillet and Hirt, 2013). Derived from complex membrane lipids, unesterified fatty acids are catalyzed by lipoxygenase (LOX) into various oxylipin products, such as JA, fatty acid hydroperoxides, and reactive electrophile species (RES) oxylipins, and these can induce stomatal closure at nanomolar concentrations (Montillet et al., 2013). A. thaliana lox1 mutants were as sensitive to exogenously applied ABA as WT plants, suggesting that LOX1 activity is not involved in ABA-induced stomatal closure. In addition, a transgenic SA-deficient NahG line, and the two SA biosynthesis mutant lines, sid1-1 and sid2-1, responded normally to ABA, but were non-responsive to RES oxylipins. In addition, lox1 mutant lines were as sensitive to SA (100 μM) as WT, demonstrating that exogenously applied SA compensated for the LOX1 deficiency. The results indicate that SA is required to convey the RES oxylipin signal, but not the ABA-mediated signal, leading to stomatal closure.
Naturally occurring saturated short, straight chain fatty acids, such as decanoic and undecanoic acids, can inhibit stomatal opening and cause stomatal closure in epidermal strips of C. communis (Willmer et al., 1978). In contrast, some polyunsaturated fatty acids, such as linolenic and arachidonic acid enhance stomatal opening and inhibit stomatal closing, consistent with their promotion of inward K+ channel activity and inhibition of outward K+ channel activity (Lee et al., 1994). Very-long-chain polyunsaturated fatty acids (VLCPUFAs), such as eicosapentaenoic acid (20:5 δ5,8,11,14,17) are abundant lipids in several key plant pathogens (Sun et al., 2013), and may elicit plant defense responses, including stomatal closure. Interestingly, it was shown that exogenous application of eicosadienoic and eicosatrienoic acids to WT plants or endogenous production in the transgenic plants could reduce water loss from excised leaves and confer ABA hypersensitivity to stomatal responses (Yuan et al., 2014). Some fatty acids have been shown to regulate stomatal development, thus affecting the overall plant response to the environment. The A. thaliana gene HIC (high carbon dioxide) encodes a putative 3-keto acyl coenzyme A synthase (KCS), an enzyme involved in the synthesis of very-long-chain fatty acids (VLCFA) and is a negative regulator of stomatal development in response to CO2 (Gray et al., 2000). Mutant hic plants exhibit up to a 42% increase in stomatal density in response to a doubling of CO2, possibly by preventing the synthesis of component(s) of the extracellular matrix found at the guard cell surface, such as waxes, glycerolipids, sphingolipids, and cutin (Gray et al., 2000). FATTY-ACID DESATURASE4 (FAD4) is required to desaturate palmitic acid (16:0), and the fad4 mutant is unable to change stomatal index (defined as the percentage of stomata as compared to all the epidermal cells (including stomata) in a unit area of leaf) in response to elevated CO2 (Lake et al., 2002). Metabolic profiling of sdd1 (STOMATAL DENSITY AND DISTRIBUTION1) plants, which have three to fourfold higher stomatal density than WT plants, showed a fivefold reduction of unsaturated C16 fatty acids compared to WT, and a concomitant rise in saturated fatty acid 16:0 species (i.e., palmitic acid; Fiehn et al., 2000). The fates of these fatty acids are scarcely known, although it is assumed that some are incorporated into the cutin layers. In A. thaliana, mutations of the VLCFA-producing enzymes CER6, CER1, and HIC that are involved in cuticle biosynthesis result in increased stomatal index (Gray et al., 2000). Whether stomatal index/density affects stomatal movement is not clear. Nevertheless, the aforementioned roles of fatty acid metabolites and their metabolic enzymes offer new avenues to elucidate lipid signaling networks in guard cells, which will facilitate engineering of fatty acid metabolism in crops for enhanced stress tolerance and productivity.
Phytohormone Cross-talk in Stomatal Function
The phytohormone ABA, first reported in plants in the 1960s (Eagles and Wareing, 1963; Ohkuma et al., 1963), is the single most studied metabolite in guard cell physiology owing to its distinct stress (e.g., drought) responsiveness and strong effect on stomatal closure. ABA causes stomatal closure, prevents opening of closed stomata, and reduces transpiration in the leaves of a wide range of species. Stomata accumulate (Cornish and Zeevaart, 1986), catabolize (Grantz et al., 1985), and conjugate exogenously supplied ABA (Grantz et al., 1985; Lee et al., 2006), but to date it is unclear if stomatal opening initially includes or requires depletion of endogenous guard cell ABA (Tallman, 2004). The biosynthesis of ABA from carotenoids in plastids and its catabolism and storage in the cytosol and endoplasmic reticulum in plant cells is well characterized (Nambara and Marion-Poll, 2005). The regulatory network of ABA sensing involve three major components, PYRABACTIN RESISTANCE1 (PYR1)/PYR1-LIKE (PYL)/REGULATORY COMPONENTS OF ABA RECEPTORS (RCAR; i.e., PYR/PYL/RCAR; an ABA receptor; Ma et al., 2009; Park et al., 2009; Joshi-Saha et al., 2011), type 2C protein phosphatase (PP2C; a negative regulator) and SNF1-related protein kinase 2 (SnRK2; a positive regulator), and they offer a double negative regulatory system, (PYR/PYL/RCAR—| PP2C—| SnRK2), which has been well studied (Klingler et al., 2010; Umezawa et al., 2010). PP2Cs inactivate SnRK2s kinases by physical interaction and direct dephosphorylation. Upon ABA binding, PYLs change their conformations and then physically interact and inhibit PP2Cs. However, PYLs inhibit PP2Cs in both the presence and absence of ABA and activate SnRK2s (Zhang et al., 2015). Several natural and artificial compounds interacting with the ABA receptor PYR/PYL/RCAR family are now known (Hitomi et al., 2013). Evolutionary insights obtained from studies on components of the ABA signaling network indicate that PYR/RCAR ABA receptor and ABF-type (ABA-responsive element binding factors) transcription factor families arose during land colonization by plants, while the ABA biosynthesis enzymes have evolved in different plant and fungal specific pathways (Hauser et al., 2011). The structural insights provided from the three-dimensional structures of module PYR/PYL/RCAR-ABA-PP2C pave the way to the design of ABA agonists able to modulate the plant stress response (Santiago et al., 2012).
ABA is transported over short and long distances in plants. Plasma membrane-localized ABA transporters belonging to ATP-binding cassette (ABC; Kang et al., 2010) and nitrate transporter 1/peptide transporter (NRT1/PTR) families are established (Boursiac et al., 2013) and ABA-perception sites were visualized on the plasma membrane of stomatal guard cells (Yamazaki et al., 2003), in addition to internal sites of perception. For instance, application of ABA into the cytosol of V. faba guard-cell protoplasts via patch-clamp techniques inhibited inward K+ currents thus inhibiting stomatal opening (Schwartz et al., 1994). Although ABA synthesis in guard cells and vascular tissues has been shown (Seo and Koshiba, 2011; Bauer et al., 2013; Boursiac et al., 2013), the relative extent to which guard cells and vascular tissues contribute to the ABA dynamics in guard cells is a topic of ongoing interest. For instance, the recent design, engineering and use of ABAleons with ABA affinities in the range of 100–600 nM to map ABA concentration changes in plant tissues with spatial and temporal resolution in distinct cell types, and in response to low humidity and NaCl in guard cells (Waadt et al., 2014) has promising future applications.
ABA causes alkalization of the guard cell cytosol (Blatt and Armstrong, 1993), which directly enhances outward K+ channel activity (Blatt and Armstrong, 1993; Ilan et al., 1994; Miedema and Assmann, 1996), and a sustained efflux of both anions and K+ from guard cells contributes to loss of guard cell turgor, thus facilitating stomatal closing. In addition, ABA-induced stomatal closing can be Ca2+-dependent or -independent (Schroeder et al., 2001). ABA mediated inhibition of stomatal opening is a process distinct from ABA-induced stomatal closure, and it is unclear if H2O2 and NO are involved in the ABA inhibition of stomatal opening (Desikan et al., 2004). Even after half a century of research, the role of ABA in guard cell signaling continues to be elucidated (Kim et al., 2010; Yu and Assmann, 2014). ABA content can be decreased via catabolism to phaseic acid (PA), sequestration in the form of an ABA-glucose ester (ABA-GE), which is thought to be physiologically inactive, or deposition in vacuoles (Nambara and Marion-Poll, 2005). Studies on sugar-response mutants indicate that ABA and sugar-response pathways overlap extensively (León and Sheen, 2003). It is known that the sugar sensing effects mediated by HXK are dependent on production of and signaling by ABA (Rolland et al., 2006; Rognoni et al., 2007; Ramon et al., 2008); for example, these interactions take place in mesophyll cells where sugar and HXK inhibit expression of photosynthesis genes (Rolland et al., 2006). Recently, it has been shown that sugar and HXK stimulate the ABA signaling pathway within guard cells, promoting stomatal closure (Kelly et al., 2013). These effects were also observed in epidermal peels, suggesting that sugar and HXK stimulate production of ABA, release of biologically active ABA from inactive ABA pools, and/or inhibition of ABA degradation within guard cells (Koiwai et al., 2004; Christmann et al., 2005; Melhorn et al., 2008; Wasilewska et al., 2008; Zhu et al., 2011). These observations also imply that ABA is probably essential for daily regulation of stomatal aperture even in the absence of water stress (Kelly et al., 2013).
A comprehensive and comparative metabolomics study undertaken in guard and mesophyll cells of A. thaliana revealed that following ABA treatment, metabolites are clustered into different temporal modules in guard cells and mesophyll cells (Jin et al., 2013). Guard cell modules differ in WT plants as compared to the modules in the heterotrimeric G-protein α subunit null mutant (gpa1), with fewer metabolites showing ABA-altered profiles in gpa1, consistent with hyposensitivity of gpa1 K+, anion, and Ca2+ channels to ABA (Wang et al., 2001; Fan et al., 2008; Zhang et al., 2011). For instance, the Ca2+-mobilizing metabolites S1P and cyclic adenosine 5′-diphosphoribose (cADPR) exhibited weaker ABA-stimulated increases in gpa1 than in WT guard cells. Phytohormones such as ABA catabolites, i.e., ABA glucose-ester, PA, and dihydrophaseic acid (DiHPA), and indole-3-acetic acid (IAA), JA, MeJA, and methyl salicylate were responsive to ABA, with greater responsiveness in WT than in the gpa1 guard cells. In particular, IAA concentrations in guard cells declined following ABA treatment in WT guard cells but not in gpa1 guard cells. These findings are consistent with the observation that exogenous application of IAA activates the guard cell H+-ATPase and impairs ABA-inhibition of stomatal opening, and suggest that endogenous ABA in guard cells functions upstream to regulate other endogenous hormones, particularly IAA, consistent with G proteins modulating multiple hormonal signaling pathways. Most phytohormones also showed differential ABA responses in guard cells as compared to mesophyll cells (Jin et al., 2013). In support of the idea that multiple hormones regulate guard cell responses, in V. faba, cytokinin and auxin induced stomatal opening (Levitt et al., 1987; Song et al., 2006) in conjunction with decreased H2O2 production (Song et al., 2006). Salicylic acid (SA) is a ubiquitous phenolic phytohormone involved in stomatal movement. Addition of 1 mM SA to fully opened stomata resulted in a significant reduction (75%) in stomatal aperture (Lee, 1998) in C. communis. SA is known to induce stomatal closure accompanied by extracellular ROS production, intracellular ROS accumulation and inward K+ channel inactivation (Khokon et al., 2011a). Although both ABA and SA were reported to be needed for stomatal closure in response to pathogens, with SA action upstream of ABA (Melotto et al., 2006), a recent study using the ABA biosynthesis mutant aba2 and a mutant of JA biosynthesis reported no differences in SA induced stomatal closure in the mutants as compared to WT. The authors concluded that neither ABA nor JA is involved in SA, yeast elicitor, or chitosan-induced stomatal closure in A. thaliana (Issak et al., 2013). These results appear to indicate the presence of an ABA independent SA signaling pathway in guard cells, but more research is need to fully resolve the contradictory conclusions in the literature.
In V. faba (Zhang et al., 2001) and Pisum sativum (Suhita et al., 2004), ABA-mediated stomatal closure is preceded by cytoplasmic alkalization and H2O2 production, events that also occur during MeJA-mediated stomatal closure. In fact, ABA and MeJA-mediated stomatal closure share several characteristic signaling components, such as Ca2+ involvement, protein phosphorylation, cytoplasmic alkalization, ROS production, and modulation of plasma membrane K+ channels in the guard cells (Suhita et al., 2004). Extremely low levels of the phytotoxin coronatine (COR), secreted by virulent strains of Pseudomonas syringae p.v. tomato (Pst) act as a JA mimic, activate the JA signaling pathway, and enable the strains to reopen stomata, thereby circumventing host stomatal defense (Montillet and Hirt, 2013). However, unlike COR, exogenous MeJA does not appear to antagonize ABA-induced stomatal closure (Melotto et al., 2006). In fact, the ability of MeJA to regulate stomatal apertures remains controversial (Montillet et al., 2013). Allene oxide synthase (AOS) is a key enzyme in the oxylipin pathway and plays a vital role in production of 12-oxo-phytodienoic acid (12-OPDA, a JA precursor) and JA. Recently, it has been proposed that 12-OPDA, rather than MeJA, acts in promotion of stomatal closure (Savchenko et al., 2014).
The role of brassinosteroids (BRs) in stomatal movements is less established. Brassinolide (BL), the most bioactive BR form, has been shown to promote stomatal closure in V. faba (Haubrick et al., 2006), where BL-induced stomatal opening was not observed. Interestingly, low concentrations of epibrassinolide (eBL) promoted stomatal opening in epidermal peels of Solanum lycopersicum in the dark, whereas high concentrations of eBL promoted stomatal closure in the light (Xia et al., 2014). Exogenous (apoplastic) and endogenous (cytosolic) BR may act differently, and guard cells of different species may respond differently to BL application. In S. lycopersicum, transient H2O2 production was deemed essential for poising the cellular redox status, which played an important role in BR-induced stomatal opening (Xia et al., 2014). BR promoted stomatal closure through apparent biosynthesis of ABA, while stomatal opening was dependent on the GSH redox status of the guard cells. It was proposed that GSH regeneration and/or biosynthesis, leading to a reduced redox status, strictly controls the ROS level and negatively regulates the ABA response pathway, and that BR can directly induce ROS production independently of ABA via NADPH oxidase.
ET and its precursor 1-aminocyclopropane-1-carboxylic acid (ACC) activate the production of H2O2 in guard cells and induce stomatal closure in V. faba (Song et al., 2014), and the closure was preceded by elevated ROS generated by NADPH oxidases (Desikan et al., 2006). However, the ET effect varies depending on species and conditions. For example, ET promotes stomatal closure in Arachis hypogaea (Pallas and Kays, 1982) and A. thaliana (using intact leaves; Desikan et al., 2006), but evokes stomatal opening in Dianthus caryophyllus and S. lycopersicum (Madhavan et al., 1983), V. faba (Levitt et al., 1987), and A. thaliana (using epidermal peels; Tanaka et al., 2005). The ET effect on stomatal opening was attributed to its impairment of ABA regulation of stomatal closure (Tanaka et al., 2005), but recently, it was shown in A. thaliana that ET mediated BR-induced stomatal closure via Gα protein-activated AtrbohF-dependent H2O2 production and subsequent Nia1-catalyzed NO production (Ge et al., 2015; Shi et al., 2015). Nonetheless, the exact mechanisms underlying the different ET effects are unknown.
Nitrogen and Sulfur Rich Metabolites in Guard Cell Signaling
Nitrogenous bases in the form of purines and pyrimidines form an essential pool of nitrogen in plant cells. Nitrogenous metabolites have been extensively studied as metabolic intermediates and signaling molecules in stomatal movement and guard cell function. Important nitrogenous signaling molecules, such as cADPR, a metabolite derived from nicotinamide adenine dinucleotide (NAD; Wu et al., 1997), play important roles in guard cell ABA signaling. Injection of cADPR into guard cells resulted in [(Ca2+)cyt] increases and turgor reduction. When guard cells were preloaded with the cADPR antagonist 8NH2-cADPR, a slowing of stomatal closure was observed in response to ABA (Leckie et al., 1998). Recently, it was established that inhibition of the poly (ADP-R) polymerase activity correlated with increased number of stomata in A. thaliana leaves (Schulz et al., 2014), highlighting the role of poly (ADP-R) metabolism in stomatal development. Another nucleotide-related metabolite, cyclic guanosine monophosphate (cGMP), has been implicated in ABA-induced stomatal closure by acting downstream of H2O2 and NO in the signaling pathway by which ABA induces stomatal closure (Dubovskaya et al., 2011). H2O2 and NO-induced cytosolic calcium increases [(Ca2+)cyt] were cGMP-dependent, positioning cGMP upstream of (Ca2+)cyt, and involved the action of the type 2C protein phosphatase, ABI1. Increases in cGMP were mediated through the stimulation of guanylyl cyclase by H2O2 and NO (Dubovskaya et al., 2011). The nitrated form of cGMP (8-nitro-cGMP) is a positive regulator in promotion of stomatal closure (Joudoi et al., 2013). NO and cGMP induce the synthesis of 8-nitro-cGMP in guard cells in the presence of ROS leading to the hypothesis that NO-dependent guanine nitration of cGMP may occur in plants and the resulting 8-nitro-cGMP acts as a signaling molecule that activates cADPR production in guard cells. By contrast, a positive role for cGMP in kinetin- and natriuretic peptide–induced stomatal opening in Tradescantia albiflora (Pharmawati et al., 1998) and in auxin-induced stomatal opening in C. communis and A. thaliana (Cousson and Vavasseur, 1998; Cousson, 2003) also has been recognized. Furthermore, application of 8-bromo-cGMP, a membrane-permeant cGMP analog, causes stomata to open in the dark (Cousson, 2003; Joudoi et al., 2013), but 8-nitro-cGMP does not. These results lead to the conclusion that cGMP and its nitrated derivative play different roles in guard cell signaling, wherein cGMP promotes stomatal opening in the dark, while 8-nitro-cGMP promotes stomatal closure in the light (Joudoi et al., 2013).
Another important group of N-containing specialized metabolites in plants are polyamines. Exogenous application of polyamines, such as 1 mM spermine, inhibit stomatal opening by inhibiting inwardly rectifying K+ channels (Liu et al., 2000). Application of spermidine also promotes stomatal closure but the mechanism is unknown as outward K+ channels and anion channels are not affected (Liu et al., 2000). On the other hand, (acetyl-)1,3-diaminopropane (DAP), a product of oxidative deamination of spermidine and spermine, suppresses anionic currents, and increases those of inwardly rectifying K+ channels, and may induce membrane hyperpolarization and extracellular acidification by activating the H+ ATPase, thus restraining stomatal closing (Jammes et al., 2014). These mechanisms act antagonistically to ABA. It is thought that during acclimation to low soil-water availability, acetyl-DAP prevents complete stomatal closure (Jammes et al., 2014). Moreover, DAP and such amine oxidase reaction products are precursors of γ-amino butyric acid, alkaloids, β-alanine, and other uncommon polyamines that play significant roles in stress tolerance and defense (Bouchereau et al., 1999). In fact, based on proteome analysis in Brassica napus guard cells, ABA-responsive proteins that decrease in abundance include those involved in spermidine synthesis, purine metabolism, and alkaloid biosynthesis pathways (Zhu et al., 2010).
Glucosinolates are N- and S-containing specialized metabolites in plants that have been shown to be present in guard cells. Glucosinolate-myrosinase systems in Brassicales, especially A. thaliana, are well understood in plant-herbivore interactions and defense against pathogens (Yan and Chen, 2007; Andersson et al., 2015). However, recent evidence from proteomic investigations has indicated that glucosinolates are required for ABA responses of guard cells (Zhao et al., 2008, Zhu et al., 2010, 2014). THIOGLUCOSIDE GLUCOHYDROLASE1 (TGG1), encoding a myrosinase that catalyzes the production of isothiocyanates (ITC) from glucosinolates, is highly abundant in guard cell proteomes. In fact, myrosinases are proposed to redundantly function downstream of ROS production and upstream of cytosolic Ca2+ elevation in ABA and MeJA signaling in guard cells (Islam et al., 2009). A. thaliana tgg1 mutants are hyposensitive to ABA inhibition of guard cell inward K+ channels and stomatal opening. In addition, thiol-reagents such as ITCs have been shown to be potent inducers of stomatal closure, possibly via covalent reactions with RES oxylipin targets (Montillet et al., 2013). Some of the glucosinolate-producing plant species, such as Brassica juncea, produce 2-propenylglucosinlate, which can be hydrolyzed to allylisothiocyanate (allylITC). Exogenous application of allyl-ITC was found to induce stomatal closure (Khokon et al., 2011b). The stomatal closure by allylITC was induced via production of ROS and NO, and elevation of cytosolic Ca2+. In addition, other ITCs, nitriles, and thiocyanates (e.g., 3-butenenitrile and ethyl thiocyanate) have also been shown to induce foliar ROS generation and stomatal closure (Hossain et al., 2013). Manipulation of glucosinolate metabolic pathways by plant metabolic engineering and breeding approaches may lead to development of crop varieties with combined disease and drought resistance. Recently, another sulfur containing compound, hydrogen sulfide (H2S), generated by L-cysteine desulfhydrase was shown to act upstream of NO to modulate ABA-dependent stomatal closure (Scuffi et al., 2014).
Conclusion
The plant leaf metabolome can boast as many as 5,000 different metabolites (Bino et al., 2004). Considering the roles of established metabolites in guard cell functions, we have begun the heydays of functional genomics, fluxomics, and systems biology toward understanding of this highly sophisticated single cell type model system. Although studies on guard cell metabolism are highly biased toward ABA and osmolytes owing to their primary importance in stomatal movement, the identification of additional critical metabolites (as shown in Figure 1) underlying or correlated with stomatal movement will form a solid foundation toward a broader understanding of optimal plant adaptation to environmental changes. For example, although progress in the study of stomatal movement in plant immunity has been made (Zhang et al., 2008), a deeper mechanistic understanding is required to harness the potential for generation of disease resistant crops. Information currently available has revealed universal and diverse metabolites and pathways leading to stomatal responses.
Many years of traditional breeding has unknowingly selected varieties with cool leaf temperature in some species, i.e., larger stomatal opening for higher yield. For instance, in Pima cotton (Gossypium barbadense L.) and bread wheat, increased stomatal conductance led to lint and grain yield increases respectively (Lu et al., 1998). Furthermore, in cotton, stomatal conductance and leaf cooling were significantly correlated with fruiting prolificacy and yield during the hottest period of the year (Radin et al., 1994). Understanding the functions and molecular networks of the regulatory metabolites of stomatal functions would open avenues for development of “smart” crops, providing a unique platform for endeavors at the genetic level to favor food security and human nutrition. Although we did not focus on the roles of stomatal ontogeny, shape, size, and distribution, which can also significantly affect plant water balance, growth and biomass, the engineering of stomatal development and response as a means to improve water use efficiency is an attractive approach to improve drought tolerance in crops (Schroeder et al., 2001). Guard cell metabolomics and systems biology hold the potential to unravel key molecular networks that control plant productivity and defense in a changing climate.
Conflict of Interest Statement
The authors declare that the research was conducted in the absence of any commercial or financial relationships that could be construed as a potential conflict of interest.
Acknowledgments
Work on guard cell signaling in the Assmann laboratory is supported by BARD grant IS-4541-12 and by NSF grants MCB-1121612, MCB-1157921, and MCB-1412644 to SA. Research on guard cell signaling in the Chen laboratory is supported by NSF grants MCB- 0818051, MCB-1158000, and MCB-1412547 to SC. Work on guard cell signaling in the Granot laboratory is supported by BARD grant IS-4541-12.
References
Akter, N., Okuma, E., Sobahan, M. A., Uraji, M., Munemasa, S., Nakamura, Y., et al. (2013). Negative regulation of methyl jasmonate-induced stomatal closure by glutathione in Arabidopsis. J. Plant Growth Regul. 32, 208–215. doi: 10.1007/s00344-012-9291-7
Allaway, W. G. (1973). Accumulation of malate in guard cells of Vicia faba during stomatal opening. Planta 110, 63–70. doi: 10.1007/BF00386923
Amodeo, G., Talbott, L. D., and Zeiger, E. (1996). Use of potassium and sucrose by onion guard cells during a daily cycle of osmoregulation. Plant Cell Physiol. 37, 575–579. doi: 10.1093/oxfordjournals.pcp.a028983
Andersson, M. X., Nilsson, A. K., Johansson, O. N., Boztaş, G., Adolfsson, L. E., Pinosa, F., et al. (2015). Involvement of the electrophilic isothiocyanate sulforaphane in Arabidopsis local defense responses. Plant Physiol. 167, 251–261. doi: 10.1104/pp.114.251892
Araujo, W. L., Nunes-Nesi, A., Osorio, S., Usadel, B., Fuentes, D., Nagy, R., et al. (2011). Antisense inhibition of the iron-sulphur subunit of succinate dehydrogenase enhances photosynthesis and growth in tomato via an organic acid-mediated effect on stomatal aperture. Plant Cell 23, 600–627. doi: 10.1105/tpc.110.081224
Asai, N., Nakajima, N., Tamaoki, M., Kamada, H., and Kondo, N. (2000). Role of malate synthesis mediated by phosphoenolpyruvate carboxylase in guard cells in the regulation of stomatal movement. Plant Cell Physiol. 41, 10–15. doi: 10.1093/pcp/41.1.10
Bates, G. W., Rosenthal, D. M., Sun, J., Chattopadhyay, M., Peffer, E., Yang, J., et al. (2012). A comparative study of the Arabidopsis thaliana guard-cell transcriptome and its modulation by sucrose. PLoS ONE 7:e49641. doi: 10.1371/journal.pone.0049641
Bauer, H., Ache, P., Lautner, S., Fromm, J., Hartung, W., Al-Rasheid, K. A., et al. (2013). The stomatal response to reduced relative humidity requires guard cell-autonomous ABA synthesis. Curr. Biol. 23, 53–57. doi: 10.1016/j.cub.2012.11.022
Bino, R. J., Hall, R. D., Fiehn, O., Kopka, J., Saito, K., Draper, J., et al. (2004). Potential of metabolomics as a functional genomics tool. Trends Plant Sci. 9, 418–425. doi: 10.1016/j.tplants.2004.07.004
Blatt, M. R., and Armstrong, F. (1993). K+ channels of stomatal guard cells: abscisic-acid-evoked control of the outward-rectifier mediated by cytoplasmic pH. Planta 191, 330–341. doi: 10.1007/BF00195690
Bouchereau, A., Aziz, A., Larher, F., and Martin-Tanguy, J. (1999). Polyamines and environmental challenges: recent development. Plant Sci. 140, 103–125. doi: 10.1016/S0168-9452(98)00218-0
Boursiac, Y., Léran, S., Corratgé-Faillie, C., Gojon, A., Krouk, G., and Lacombe, B. (2013). ABA transport and transporters. Trends Plant Sci. 18, 325–333. doi: 10.1016/j.tplants.2013.01.007
Bright, J., Desikan, R., Hancock, J. T., Weir, I. S., and Neill, S. J. (2006). ABA-induced NO generation and stomatal closure in Arabidopsis are dependent on H2O2 synthesis. Plant J. 45, 113–122. doi: 10.1111/j.1365-313X.2005.02615.x
Chen, Z., and Gallie, D. R. (2004). The ascorbic acid redox state controls guard cell signaling and stomatal movement. Plant Cell 16, 1143–1162. doi: 10.1105/tpc.021584
Christmann, A., Hoffmann, T., Teplova, I., Grill, E., and Muller, A. (2005). Generation of active pools of abscisic acid revealed by in vivo imaging of water-stressed Arabidopsis. Plant Physiol. 137, 209–219. doi: 10.1104/pp.104.053082
Cornish, K., and Zeevaart, J. A. (1986). Abscisic acid accumulation by in situ and isolated guard cells of Pisum sativum L. and Vicia faba L. in relation to water stress. Plant Physiol. 81, 1017–1021. doi: 10.1104/pp.81.4.1017
Coursol, S., Fan, L. M., Le Stunff, H., Spiegel, S., Gilroy, S., and Assmann, S. M. (2003). Sphingolipid signaling in Arabidopsis guard cells involves heterotrimeric G proteins. Nature 423, 651–654. doi: 10.1038/nature01643
Coursol, S., Le Stunff, H., Lynch, D. V., Gilroy, S., Assmann, S. M., and Spiegel, S. (2005). Arabidopsis sphingosine kinase and the effects of phytosphingosine-1-phosphate on stomatal aperture. Plant Physiol. 137, 724–737. doi: 10.1104/pp.104.055806
Cousson, A. (2003). Pharmacological evidence for a positive influence of the cyclic GMP-independent transduction on the cyclic GMP-mediated Ca2+-dependent pathway within the Arabidopsis stomatal opening in response to auxin. Plant Sci. 164, 759–767. doi: 10.1016/S0168-9452(03)00062-1
Cousson, A., and Vavasseur, A. (1998). Putative involvement of cytosolic Ca2+ and GTP-binding proteins in cyclic-GMP-mediated induction of stomatal opening by auxin in Commelina communis L. Planta 206, 308–314. doi: 10.1007/s004250050405
Dennis, D. T., and Blakeley, S. D. (2000). “Carbohydrate metabolism,” in Biochemistry and Molecular Biology of Plants, eds B. B. Buchanan, W. Gruissem, and R. L. Jones (Rockville, MD: American Society of Plant Physiologists), 676–728.
Desikan, R., Cheung, M. K., Bright, J., Henson, D., Hancock, J. T., and Neill, S. J. (2004). ABA, hydrogen peroxide and nitric oxide signalling in stomatal guard cells. J. Exp. Bot. 55, 205–212. doi: 10.1093/jxb/erh033
Desikan, R., Last, K., Harrett-Williams, R., Tagliavia, C., Harter, K., Hooley, R., et al. (2006). Ethylene-induced stomatal closure in Arabidopsis occurs via AtrbohF-mediated hydrogen peroxide synthesis. Plant J. 47, 907–916. doi: 10.1111/j.1365-313X.2006.02842.x
Dittrich, P., and K. Raschke, K. (1977). Malate metabolism in isolated epidermis of Commelina communis L. in relation to stomatal functioning. Planta 134, 77–81. doi: 10.1007/BF00390098
Dixon, R. A., and Paiva, N. L. (1995). Stress-induced phenylpropanoid metabolism. Plant Cell 7, 1085–1097. doi: 10.1105/tpc.7.7.1085
Dubovskaya, L. V., Bakakina, Y. S., Kolesneva, E. V., Sodel, D. L., McAinsh, M. R., and Hetherington, A. M. (2011). cGMP-dependent ABA-induced stomatal closure in the ABA-insensitive Arabidopsis mutant abi1-1. New Phytol. 191, 57–69. doi: 10.1111/j.1469-8137.2011.03661.x
Eagles, C. F., and Wareing, P. F. (1963). Dormancy regulators in woody plants: experimental induction of dormancy in Betula pubescens. Nature 199, 874–875. doi: 10.1038/199874a0
Fan, L. M., Zhang, W., Chen, J. G., Taylor, J. P., Jones, A. M., and Assmann, S. M. (2008). Abscisic acid regulation of guard-cell K+ and anion channels in Gβ-and RGS-deficient Arabidopsis lines. Proc. Natl. Acad. Sci. U.S.A. 105, 8476–8481. doi: 10.1073/pnas.0800980105
Fernie, A. R., and Martinoia, E. (2009). Malate. Jack of all trades or master of a few? Phytochemistry 70, 828–832. doi: 10.1016/j.phytochem.2009.04.023
Fiehn, O., Kopka, J., Dörmann, P., Altmann, T., Trethewey, R. N., and Willmitzer, L. (2000). Metabolite profiling for plant functional genomics. Nat. Biotechnol. 18, 1157–1161. doi: 10.1038/81137
Fischer, R. A. (1968). Stomatal opening: role of potassium uptake by guard cells. Science 160, 784–785. doi: 10.1126/science.160.3829.784
Ge, X. M., Cai, H. L., Lei, X., Zhou, X., Yue, M., and He, J. M. (2015). Heterotrimeric G protein mediates ethylene-induced stomatal closure via hydrogen peroxide synthesis in Arabidopsis. Plant J. 82, 138–150. doi: 10.1111/tpj.12799
Gilroy, S., Read, N., and Trewavas, A. J. (1990). Elevation of cytoplasmic calcium by caged calcium or caged inositol trisphosphate initiates stomatal closure. Nature 346, 769–771. doi: 10.1038/346769a0
Gotow, K., Taylor, S., and Zeiger, E. (1988). Photosynthetic carbon fixation in guard cell protoplasts of Vicia faba L. : evidence from radiolabel experiments. Plant Physiol. 86, 700–705. doi: 10.1104/pp.86.3.700
Granot, D. (2008). Putting plant hexokinases in their proper place. Phytochemistry 69, 2649–2654. doi: 10.1016/j.phytochem.2008.08.026
Grantz, D. A., Ho, T. H. D., Uknes, S. J., Cheeseman, J. M., and Boyer, J. S. (1985). Metabolism of abscisic acid in guard cells of Vicia faba L. and Commelina communis L. Plant Physiol. 78, 51–56. doi: 10.1104/pp.78.1.51
Gray, J. E., Holroyd, G. H., van der Lee, F. M., Bahrami, A. R., Sijmons, P. C., Woodward, F. I., et al. (2000). The HIC signaling pathway links CO2 perception to stomatal development. Nature 408, 713–716. doi: 10.1038/35047071
Guo, L., and Wang, X. (2012). Crosstalk between phospholipase D and sphingosine kinase in plant stress signaling. Front. Plant Sci. 3:51. doi: 10.3389/fpls.2012.00051
Guo, L., Mishra, G., Taylor, K., and Wang, X. (2011). Phosphatidic acid binds and stimulates Arabidopsis sphingosine kinases. J. Biol. Chem. 286, 13336–13345. doi: 10.1074/jbc.M110.190892
Haubrick, L. L., Torsethaugen, G., and Assmann, S. M. (2006). Effect of brassinolide, alone and in concert with abscisic acid, on control of stomatal aperture and potassium currents of Vicia faba guard cell protoplasts. Physiol. Plant. 128, 134–143. doi: 10.1111/j.1399-3054.2006.00708.x
Hauser, F., Waadt, R., and Schroeder, J. I. (2011). Evolution of abscisic acid synthesis and signaling mechanisms. Curr. Biol. 21, R346–R355. doi: 10.1016/j.cub.2011.03.015
He, J. M., Ma, X. G., Zhang, Y., Sun, T. F., Xu, F. F., Chen, Y. P., et al. (2013). Role and interrelationship of Gα protein, hydrogen peroxide, and nitric oxide in ultraviolet B-induced stomatal closure in Arabidopsis leaves. Plant Physiol. 161, 1570–1583. doi: 10.1104/pp.112.211623
Hedrich, R., and Marten, I. (1993). Malate-induced feedback regulation of plasma membrane anion channels could provide a CO2 sensor to guard cells. EMBO J. 12, 897–901.
Hitomi, K., Getzoff, E. D., and Schroeder, J. I. (2013). Going green: phytohormone mimetics for drought rescue. Plant Physiol. 163, 1087–1088. doi: 10.1104/pp.113.227660
Hoque, T. S., Uraji, M., Ye, W., Hossain, M. A., Nakamura, Y., and Murata, Y. (2012). Methylglyoxal-induced stomatal closure accompanied by peroxidase-mediated ROS production in Arabidopsis. J. Plant Physiol. 169, 979–986. doi: 10.1016/j.jplph.2012.02.007
Hossain, M. S., Ye, W., Hossain, M. A., Okuma, E., Uraji, M., Nakamura, Y., et al. (2013). Glucosinolate degradation products, isothiocyanates, nitriles, and thiocyanates, induce stomatal closure accompanied by peroxidase-mediated reactive oxygen species production in Arabidopsis thaliana. Biosci. Biotechnol. Biochem. 77, 977–983. doi: 10.1271/bbb.120928
Humble, G. D., and Raschke, K. (1971). Stomatal opening quantitatively related to potassium transport: evidence from electron probe analysis. Plant Physiol. 48, 447–453. doi: 10.1104/pp.48.4.447
Ilan, N., Schwartz, A., and Moran, N. (1994). External pH effects on the depolarization-activated K channels in guard cell protoplast of Vicia faba. J. Gen. Physiol. 103, 807–831. doi: 10.1085/jgp.103.5.807
Imamura, S. (1943). Untersuchungen uber den mechanismus der turgorschwandung der spaltoffnungesschliesszellen. Jpn. J. Bot. 12, 82–88.
Islam, M. M., Tani, C., Watanabe-Sugimoto, M., Uraji, M., Jahan, M. S., Masuda, C., et al. (2009). Myrosinases, TGG1 and TGG2, redundantly function in ABA and MeJA signaling in Arabidopsis guard cells. Plant Cell Physiol. 50, 1171–1175. doi: 10.1093/pcp/pcp066
Issak, M., Okuma, E., Munemasa, S., Nakamura, Y., Mori, I. C., and Murata, Y. (2013). Neither endogenous abscisic acid nor endogenous jasmonate is involved in salicylic acid-, yeast elicitor-, or chitosan-induced stomatal closure in Arabidopsis thaliana. Biosci. Biotechnol. Biochem. 77, 1111–1113. doi: 10.1271/bbb.120980
Jammes, F., Leonhardt, N., Tran, D., Bousserouel, H., Véry, A.-A., Renou, J.-P., et al. (2014). Acetylated 1,3-diaminopropane antagonizes abscisic acid-mediated stomatal closing in Arabidopsis. Plant J. 79, 322–333. doi: 10.1111/tpj.12564
Jin, X., Wang, R. S., Zhu, M., Jeon, B. W., Albert, R., Chen, S., et al. (2013). Abscisic acid–responsive guard cell metabolomes of Arabidopsis wild-type and gpa1 G-protein mutants. Plant Cell 25, 4789–4811. doi: 10.1105/tpc.113.119800
Joshi-Saha, A., Valon, C., and Leung, J. (2011). A brand new START: abscisic acid perception and transduction in the guard cell. Sci. Signal. 4, re4–re4. doi: 10.1126/scisignal.2002164
Joudoi, T., Shichiri, Y., Kamizono, N., Akaike, T., Sawa, T., Yoshitake, J., et al. (2013). Nitrated cyclic GMP modulates guard cell signaling in Arabidopsis. Plant Cell 25, 558–571. doi: 10.1105/tpc.112.105049
Jung, J. Y., Kim, Y. W., Kwak, J. M., Hwang, J. U., Young, J., Schroeder, J. I., et al. (2002). Phosphatidylinositol 3-and 4-phosphate are required for normal stomatal movements. Plant Cell 14, 2399–2412. doi: 10.1105/tpc.004143
Kang, J., Hwang, J. U., Lee, M., Kim, Y. Y., Assmann, S. M., Martinoia, E., et al. (2010). PDR-type ABC transporter mediates cellular uptake of the phytohormone abscisic acid. Proc. Natl. Acad. Sci. U.S.A. 107, 2355–2360. doi: 10.1073/pnas.0909222107
Karabourniotis, G., Tzobanoglou, D., Nikolopoulos, D., and Liakopoulos, G. (2001). Epicuticular phenolics over guard cells: exploitation for in situ stomatal counting by fluorescence microscopy and combined image analysis. Ann. Bot. 87, 631–639. doi: 10.1006/anbo.2001.1386
Kelly, G., Moshelion, M., David-Schwartz, R., Halperin, O., Wallach, R., Attia, Z., et al. (2013). Hexokinase mediates stomatal closure. Plant J. 75, 977–988. doi: 10.1111/tpj.12258
Khokon, A. R., Okuma, E., Hossain, M. A., Munemasa, S., Uraji, M., Nakamura, Y., et al. (2011a). Involvement of extracellular oxidative burst in salicylic acid-induced stomatal closure in Arabidopsis. Plant Cell Environ. 34, 434–443. doi: 10.1111/j.1365-3040.2010.02253.x
Khokon, M., Jahan, M. S., Rahman, T., Hossain, M. A., Muroyama, D., Minami, I., et al. (2011b). Allyl isothiocyanate (AITC) induces stomatal closure in Arabidopsis. Plant Cell Environ. 34, 1900–1906. doi: 10.1111/j.1365-3040.2011.02385.x
Kim, T. H., Böhmer, B., Hu, H., Nishimura, N., and Schroeder, J. I. (2010). Guard cell signal transduction network: advances in understanding abscisic acid, CO2, and Ca2+ signaling. Annu. Rev. Plant Biol. 61, 561–591. doi: 10.1146/annurev-arplant-042809-112226
Klingler, J. P., Batelli, G., and Zhu, J. K. (2010). ABA receptors: the START of a new paradigm in phytohormone signaling. J. Exp. Bot. 61, 3199–3210. doi: 10.1093/jxb/erq151
Koiwai, H., Nakaminami, K., Seo, M., Mitsuhashi, W., Toyomasu, T., and Koshiba, T. (2004). Tissue-specific localization of an abscisic acid biosynthetic enzyme, AAO3, in Arabidopsis. Plant Physiol. 134, 1697–1707. doi: 10.1104/pp.103.036970
Kwak, J. M., Mori, I. C., Pei, Z. M., Leonhardt, N., Torres, M. A., Dangl, J. L., et al. (2003). NADPH oxidase AtrbohD and AtrbohF genes function in ROS-dependent ABA signaling in Arabidopsis. EMBO J. 22, 2623–2633. doi: 10.1093/emboj/cdg277
Lake, J. A., Woodward, F. I., and Quick, W. P. (2002). Long-distance CO2 signaling in plants. J. Esp. Bot. 53, 183–193. doi: 10.1093/jexbot/53.367.183
Lawson, T., Simkin, A. J., Kelly, G., and Granot, D. (2014). Mesophyll photosynthesis and guard cell metabolism impacts on stomatal behavior. New Phytol. 203, 1064–1081. doi: 10.1111/nph.12945
Leckie, C. P., McAinsh, M. R., Allen, G. J., Sanders, D., and Hetherington, A. M. (1998). Abscisic acid-induced stomatal closure mediated by cyclic ADP-ribose. Proc. Natl. Acad. Sci. U.S.A. 95, 15837–15842. doi: 10.1073/pnas.95.26.15837
Lee, J.-S. (1998). The mechanism of stomatal closing by salicylic acid in Commelina communis L. J. Plant Biol. 41, 97–102. doi: 10.1007/BF03030395
Lee, K. H., Piao, H. L., Kim, H. Y., Choi, S. M., Jiang, F., Hartung, W., et al. (2006). Activation of glucosidase via stress-induced polymerization rapidly increases active pools of abscisic acid. Cell 126, 1109–1120. doi: 10.1016/j.cell.2006.07.034
Lee, M., Choi, Y., Burla, B., Kim, Y. Y., Jeon, B., Maeshima, M., et al. (2008). The ABC transporter AtABCB14 is a malate importer and modulates stomatal response to CO2. Nat. Cell Biol. 10, 1217–1223. doi: 10.1038/ncb1782
Lee, Y., Choi, Y. B., Suh, S., Lee, J., Assmann, S. M., Joe, C. O., et al. (1996). Abscisic acid-induced phosphoinositide turnover in guard cell protoplasts of Vicia faba. Plant Physiol. 110, 987–996.
Lee, Y., Lee, H. J., Crain, R. C., Lee, A., and Korn, S. J. (1994). Polyunsaturated fatty acids modulates stomatal aperture and two distinct K+ channel currents in guard cells. Cell. Signal. 6, 181–186. doi: 10.1016/0898-6568(94)90075-2
Lemtiri-Chlieh, F., MacRobbie, E. A., Webb, A. A., Manison, N. F., Brownlee, C., Skepper, J. N., et al. (2003). Inositol hexakisphosphate mobilizes an endomembrane store of calcium in guard cells. Proc. Natl. Acad. Sci. U.S.A. 100, 10091–10095. doi: 10.1073/pnas.1133289100
León, P., and Sheen, J. (2003). Sugar and hormone connections. Trends Plant Sci. 8, 110–116. doi: 10.1016/s1360-1385(03)00011-6
Leonhardt, N., Kwak, J. M., Robert, N., Waner, D., Leonhardt, G., and Schroeder, J. I. (2004). Microarray expression analyses of Arabidopsis guard cells and isolation of a recessive abscisic acid hypersensitive protein phosphatase 2C mutant. Plant Cell 16, 596–615. doi: 10.1105/tpc.019000
Levitt, L. K., Stein, D. B., and Rubinstein, B. (1987). Promotion of stomatal opening by indole acetic acid and ethrel in epidermal strips of Vicia faba L. Plant Physiol. 85, 318–323. doi: 10.1104/pp.85.2.318
Li, C. L., Wang, M., Ma, X. Y., and Zhang, W. (2014). NRGA1, a putative mitochondrial pyruvate carrier, mediates aba regulation of guard cell ion channels and drought stress responses in Arabidopsis. Mol. Plant 7, 1508–1521. doi: 10.1093/mp/ssu061
Li, J., Ou-Lee, T. M., Raba, R., Amundson, R. G., and Last, R. L. (1993). Arabidopsis flavonoid mutants are hypersensitive to UV-B irradiation. Plant Cell 5, 171–179. doi: 10.1105/tpc.5.2.171
Liu, K., Fu, H., Bei, Q., and Luan, S. (2000). Inward potassium channel in guard cells as a target for polyamine regulation of stomatal movements. Plant Physiol. 124, 1315–1326. doi: 10.1104/pp.124.3.1315
Lloyd, F. E. (1908). The Physiology of Stomata. Washington: Carnegie Institution for Science 82, 1–142.
Lu, P., Outlaw, W. H. Jr, Smith, B. G., and Freed, G. A. (1997). A new mechanism for the regulation of stomatal aperture size in intact leaves. Accumulation of mesophyll-derived sucrose in the guard-cell wall of Vicia faba. Plant Physiol. 114, 109–118.
Lu, P., Zhang, S. Q., Outlaw, W. H. Jr, and Riddle, K. A. (1995). Sucrose: a solute that accumulates in the guard-cell apoplast and guard-cell symplast of open stomata. FEBS Lett. 362, 180–184. doi: 10.1016/0014-5793(95)00239-6
Lu, Z., Percy, R. G., Qualset, C. O., and Zeiger, E. (1998). Stomatal conductance predicts yields in irrigated Pima cotton and bread wheat grown at high temperatures. J. Exp. Bot. 49, 453–460. doi: 10.1093/jxb/49.Special_Issue.453
Ma, Y., Szostkiewicz, I., Korte, A., Moes, D., Yang, Y., Christmann, A., et al. (2009). Regulators of PP2C phosphatase activity function as abscisic acid sensors. Science 324, 1064–1068. doi: 10.1126/science.1172408
Madhavan, S., Chrominiski, A., and Smith, B. N. (1983). Effect of ethylene on stomatal opening in tomato and carnation leaves. Plant Cell Physiol. 24, 569–572.
Medeiros, D. B., Daloso, D. M., Fernie, A. R., Nikoloski, Z., and Araújo, W. L. (2015). Utilizing systems biology to unravel stomatal function and the hierarchies underpinning its control. Plant Cell Environ. doi: 10.1111/pce.12517 [Epub ahead of print].
Melhorn, V., Matsumi, K., Koiwai, H., Ikegami, K., Okamoto, M., and Nambara, E. (2008). Transient expression of AtNCED3 and AAO3 genes in guard cells causes stomatal closure in Vicia faba. J. Plant Res. 121, 125–131. doi: 10.1007/s10265-007-0127-7
Melotto, M., Underwood, W., Koczan, J., Nomura, K., and He, S. Y. (2006). Plant stomata function in innate immunity against bacterial invasion. Cell 126, 969–980. doi: 10.1016/j.cell.2006.06.054
Miao, Y., Lv, D., Wang, P., Wang, X.-C., Chen, J., Miao, C., et al. (2006). An Arabidopsis glutathione peroxidase functions as both a redox transducer and a scavenger in abscisic acid and drought stress responses. Plant Cell 18, 2749–2766. doi: 10.1105/tpc.106.044230
Miedema, H., and Assmann, S. M. (1996). A membrane-delimited effect of internal pH on the K+ outward rectifier of Vicia faba guard cells. J. Mem. Biol. 154, 227–237. doi: 10.1007/s002329900147
Misra, B. B., Assmann, S. M., and Chen, S. (2014). Plant single-cell and single-cell-type metabolomics. Trends Plant Sci. 19, 637–646. doi: 10.1016/j.tplants.2014.05.005
Montillet, J. L., and Hirt, H. (2013). New checkpoints in stomatal defense. Trends Plant Sci. 18, 295–297. doi: 10.1016/j.tplants.2013.03.007
Montillet, J. L., Leonhardt, N., Mondy, S., Tranchimand, S., Rumeau, D., Boudsocq, M., et al. (2013). An abscisic acid-independent oxylipin pathway controls stomatal closure and immune defense in Arabidopsis. PLoS Biol. 11:e1001513. doi: 10.3410/f.717991704.793474995
Moore, B., Zhou, L., Rolland, F., Hall, Q., Cheng, W. H., Liu, Y. X., et al. (2003). Role of the Arabidopsis glucose sensor HXK1 in nutrient, light, and hormonal signaling. Science 300, 332–336. doi: 10.1126/science.1080585
Munemasa, S., Muroyama, D., Nagahashi, H., Nakamura, Y., Mori, I. C., and Murata, Y. (2013). Regulation of reactive oxygen species-mediated abscisic acid signaling in guard cells and drought tolerance by glutathione. Front. Plant Sci. 4:472. doi: 10.3389/fpls.2013.00472
Nambara, E., and Marion-Poll, A. (2005). Abscisic acid biosynthesis and catabolism. Annu. Rev. Plant Biol. 56, 165–185. doi: 10.1146/annurev.arplant.56.032604.144046
Ng, C. K. Y., Carr, K., McAinsh, M. R., Powell, B., and Hetherington, A. M. (2001). Drought-induced guard cell signal transduction involves sphingosine-1-phosphate. Nature 410, 596–599. doi: 10.1038/35069092
Noctor, G., and Foyer, C. H. (1998). Ascorbate and glutathione: keeping active oxygen under control. Annu. Rev. Plant Biol. 49, 249–279. doi: 10.1146/annurev.arplant.49.1.249
Ohkuma, K., Lyon, J. L., Addicott, F. T., and Smith, O. E. (1963). Abscisin II, an absc-ission-accelerating substance from young cotton fruit. Science 142, 1592–1593.
Okuma, E., Jahan, M. S., Munemasa, S., Hossain, M. A., Muroyama, D., Islam, M. M., et al. (2011). Negative regulation of abscisic acid-induced stomatal closure by glutathione in Arabidopsis. J. Plant Physiol. 168, 2048–2055. doi: 10.1016/j.jplph.2011.06.002
Ou, X., Gan, Y., Chen, P., Qiu, M., Jiang, K., and Wang, G. (2014). Stomata prioritize their responses to multiple biotic and abiotic signal inputs. PLoS ONE 9:e101587. doi: 10.1371/journal.pone.0101587
Outlaw, W. H. (1989). Critical examination of the quantitative evidence for and against photosynthetic CO2 fixation by guard cells. Physiol. Plant. 77, 275–281. doi: 10.1111/j.1399-3054.1989.tb04981.x
Outlaw, W. H., and Lowry, O. H. (1977). Organic acid and potassium accumulation in guard cells during stomatal opening. Proc. Natl. Acad. Sci. U.S.A. 74, 4434–4438.
Outlaw, W. H. Jr, and De Vlieghere-He, X. (2001). Transpiration rate. An important factor controlling the sucrose content of the guard cell apoplast of broad bean. Plant Physiol. 126, 1716–1724. doi: 10.1104/pp.126.4.1716
Pallas, J. E., and Kays, S. J. (1982). Inhibition of photosynthesis by ethylene-a stomatal effect. Plant Physiol. 70, 598–601. doi: 10.1104/pp.70.2.598
Park, K. Y., Jung, J. Y., Park, J., Hwang, J. U., Kim, Y. W., and Hwang, I. (2003). A role for phosphatidylinositol 3-phosphate in abscisic acid-induced reactive oxygen species generation in guard cells. Plant Physiol. 132, 92–98. doi: 10.1104/pp.102.016964
Park, S. Y., Fung, P., Nishimura, N., Jensen, D. R., Fujii, H., Zhao, Y., et al. (2009). Abscisic acid inhibits type 2C protein phosphatases via the PYR/PYL family of START proteins. Science 324, 1068–1071. doi: 10.1126/science.1173041
Pearson, C. J. (1973). Daily changes in stomatal aperture and in carbohydrates and malate within epidermis and mesophyll of leaves of Commelina cyanea and Vicia faba. Aust. J. Biol. Sci. 26, 1035–1044.
Penfield, S., Clements, S., Bailey, K. J., Gilday, A. D., Leegood, R. C., Gray, J. E., et al. (2012). Expression and manipulation of PHOSPHOENOLPYRUVATE CARBOXYKINASE 1 identifies a role for malate metabolism in stomatal closure. Plant J. 69, 679–688. doi: 10.1111/j.1365-313X.2011.04822.x
Pharmawati, M., Billington, T., and Gehring, C. A. (1998). Stomatal guard cell responses to kinetin and natriuretic peptides are cGMP-dependent. Cell. Mol. Life Sci. 54, 272–276. doi: 10.1007/s000180050149
Radin, J. W., Lu, Z., Percy, R. G., and Zeiger, E. (1994). Genetic variability for stomatal conductance in Pima cotton and its relation to improvements of heat adaptation. Proc. Natl. Acad. Sci. U.S.A. 91, 7217–7221.
Raghavendra, A. S., Rao, I. M., and Das, V. S. R. (1976). Characterization of abscisic acid inhibition of stomatal opening in isolated epidermal strips. Plant Sci. Lett. 6, 111–115. doi: 10.1016/0304-4211(76)90144-9
Ramon, M., Rolland, F., and Sheen, J. (2008). Sugar sensing and signaling. Arabidopsis Book 6:e0117. doi: 10.1199/tab.0117
Reckmann, U., Scheibe, R., and Raschke, K. (1990). Rubisco activity in guard cells compared with the solute requirement for stomatal opening. Plant Physiol. 92, 246–253. doi: 10.1104/pp.92.1.246
Rognoni, S., Teng, S., Arru, L., Smeekens, S. C. M., and Perata, P. (2007). Sugar effects on early seedling development in Arabidopsis. Plant Growth Regul. 52, 217–228. doi: 10.1007/s10725-007-9193-z
Rolland, F., Baena-Gonzalez, E., and Sheen, J. (2006). Sugar sensing and signaling in plants: conserved and novel mechanisms. Annu Rev. Plant Biol. 57, 675–709. doi: 10.1146/annurev.arplant.57.032905.105441
Santiago, J., Dupeux, F., Betz, K., Antoni, R., Gonzalez-Guzman, M., Rodriguez, L., et al. (2012). Structural insights into PYR/PYL/RCAR ABA receptors and PP2Cs. Plant Sci. 182, 3–11. doi: 10.1016/j.plantsci.2010.11.014
Savchenko, T., Kolla, V. A., Wang, C. Q., Nasafi, Z., Hicks, D. R., Phadungchob, B., et al. (2014). Functional convergence of oxylipin and abscisic acid pathways controls stomatal closure in response to drought. Plant Physiol. 164, 1151–1160. doi: 10.1104/pp.113.234310
Schroeder, J. I., Kwak, J. M., and Allen, G. J. (2001). Guard cell abscisic acid signaling and engineering drought hardiness in plants. Nature 410, 327–330. doi: 10.1038/35066500
Schulz, P., Jansseune, K., Degenkolbe, T., Méret, M., Claeys, H., Skirycz, A., et al. (2014). Poly (ADP-Ribose) polymerase activity controls plant growth by promoting leaf cell number. PLoS ONE 9:e90322. doi: 10.1371/journal.pone.0090322
Schwartz, A., Wu, W. H., Tucker, E. B., and Assmann, S. M. (1994). Inhibition of inward K+ channels and stomatal response by abscisic acid: an intracellular locus of phytohormone action. Proc. Natl. Acad. Sci. U.S.A. 91, 4019–4023.
Scuffi, D., Núñez, Á., Laspina, N., Gotor, C., Lamattina, L., and Garcia-Mata, C. (2014). Hydrogen sulfide generated by L-cysteine desulfhydrase acts upstream of nitric oxide to modulate ABA-dependent stomatal closure. Plant Physiol. 245373, 114. doi: 10.1104/pp.114.245373
Seo, M., and Koshiba, T. (2011). Transport of ABA from the site of biosynthesis to the site of action. J. Plant Res. 124, 501–507. doi: 10.1007/s10265-011-0411-4
Shi, C., Qi, C., Ren, H., Huang, A., Hei, S., and She, X. (2015). Ethylene mediates brassinosteroid-induced stomatal closure via Gα protein-activated hydrogen peroxide and nitric oxide production in Arabidopsis. Plant J. 82, 280–301. doi: 10.1111/tpj.12815
Song, X., She, X., He, J., Huang, C., and Song, T. (2006). Cytokinin- and auxin-induced stomatal opening involves a decrease in levels of hydrogen peroxide in guard cells of Vicia faba. Funct. Plant Biol. 33, 573–583. doi: 10.1071/FP05232
Song, X. G., She, X. P., Yue, M., Liu, Y. E., Wang, Y. X., Zhu, X., et al. (2014). Involvement of copper amine oxidase (CuAO)-dependent hydrogen peroxide synthesis in ethylene-induced stomatal closure in Vicia faba. Russ. J. Plant Physiol. 61, 390–396. doi: 10.1134/S1021443714020150
Stadler, R., Buttner, M., Ache, P., Hedrich, R., Ivashikina, N., Melzer, M., et al. (2003). Diurnal and light-regulated expression of AtSTP1 in guard cells of Arabidopsis. Plant Physiol. 133, 528–537. doi: 10.1104/pp.103.024240
Suhita, D., Raghavendra, A. S., Kwak, J. M., and Vavasseur, A. (2004). Cytoplasmic alkalization precedes reactive oxygen species production during methyl jasmonate- and abscisic acid-induced stomatal closure. Plant Physiol. 134, 1536–1545. doi: 10.1104/pp.103.032250
Sun, Q., Liu, J., Zhang, Q., Qing, X., Dobson, G., Li, X., et al. (2013). Characterization of three novel desaturases involved in the delta-6 desaturation pathways for polyunsaturated fatty acid biosynthesis from Phytophthora infestans. Appl. Microbiol. Biotechnol. 97, 7689–7697. doi: 10.1007/s00253-012-4613-z
Sun, Z., Jin, X., Albert, R., and Assmann, S. M. (2014). Multi-level modeling of light-induced stomatal opening offers new insights into its regulation by drought. PLoS Comp. Biol. 10:e1003930. doi: 10.1371/journal.pcbi.1003930
Talbott, L. D., and Zeiger, E. (1988). Light quality and osmoregulation in Vicia guard cells: evidence for involvement of three metabolic pathways. Plant Physiol. 88, 887–895. doi: 10.1104/pp.88.3.887
Talbott, L. D., and Zeiger, E. (1993). Sugar and organic acid accumulation in guard cells of Vicia faba in response to red and blue light. Plant Physiol. 102, 1163–1169.
Talbott, L. D., and Zeiger, E. (1996). Central roles for potassium and sucrose in guard-cell osmoregulation. Plant Physiol. 111, 1051–1057.
Tallman, G. (2004). Are diurnal patterns of stomatal movement the result of alternating metabolism of endogenous guard cell ABA and accumulation of ABA delivered to the apoplast around guard cells by transpiration? J. Exp. Bot. 55, 1963–1976. doi: 10.1093/jxb/erh212
Tanaka, Y., Sano, T., Tamaoki, M., Nakajima, N., Kondo, N., and Hasezawa, S. (2005). Ethylene inhibits abscisic acid-induced stomatal closure in Arabidopsis. Plant Physiol. 138, 2337–2343. doi: 10.1104/pp.105.063503
Tian, W., Hou, C., Ren, Z., Pan, Y., Jia, J., Zhang, H., et al. (2015). A molecular pathway for CO2 response in Arabidopsis guard cells. Nat. Commun. 6:6057. doi: 10.1038/ncomms7057
Umezawa, T., Nakashima, K., Miyakawa, T., Kuromori, T., Tanokura, M., Shinozaki, K., et al. (2010). Molecular basis of the core regulatory network in ABA responses: sensing, signaling and transport. Plant Cell Physiol. 51, 1821–1839. doi: 10.1093/pcp/pcq156
Waadt, R., Hitomi, K., Nishimura, N., Hitomi, C., Adams, S. R., Getzoff, E. D., et al. (2014). FRET-based reporters for the direct visualization of abscisic acid concentration changes and distribution in Arabidopsis. eLife 3:e01739. doi: 10.7554/eLife.01739
Wang, P., Du, Y., Hou, Y. J., Zhao, Y., Hsu, C. C., Yuan, F., et al. (2015). Nitric oxide negatively regulates abscisic acid signaling in guard cells by S-nitrosylation of OST1. Proc. Natl. Acad. Sci. U.S.A. 112, 613–618. doi: 10.1073/pnas.1423481112
Wang, R. S., Pandey, S., Li, S., Gookin, T. E., Zhao, Z., Albert, R., et al. (2011). Common and unique elements of the ABA-regulated transcriptome of Arabidopsis guard cells. BMC Genomics 12:216. doi: 10.1186/1471-2164-12-216
Wang, X. Q., Ullah, H., Jones, A. M., and Assmann, S. M. (2001). G protein regulation of ion channels and abscisic acid signaling in Arabidopsis guard cells. Science 292, 2070–2072. doi: 10.1126/science.1059046
Wasilewska, A., Vlad, F., Sirichandra, C., Redko, Y., Jammes, F., Valon, C., et al. (2008). An update on abscisic acid signaling in plants and more. Mol. Plant 1, 198–217. doi: 10.1093/mp/ssm022
Watkins, J. M., Hechler, P. J., and Muday, G. K. (2014). Ethylene-induced flavonol accumulation in guard cells suppresses reactive oxygen species and moderates stomatal aperture. Plant Physiol. 164, 1707–1717. doi: 10.1104/pp.113.233528
Weise, A., Lalonde, S., Kuhn, C., Frommer, W. B., and Ward, J. M. (2008). Introns control expression of sucrose transporter LeSUT1 in trichomes, companion cells and in guard cells. Plant Mol. Biol. 68, 251–262. doi: 10.1007/s11103-008-9366-9
Willmer, C. M., Don, R., and Parker, W. (1978). Levels of short-chain fatty acids and of abscisic acid in water-stressed and non-stressed leaves and their effects on stomata in epidermal strips and excised leaves. Planta 139, 281–287. doi: 10.1007/BF00388642
Winkel-Shirley, B. (2002). Biosynthesis of flavonoids and effects of stress. Curr. Opin. Plant Biol. 5, 218–223. doi: 10.1016/S1369-5266(02)00256-X
Worrall, D., Liang, Y. K., Alvarez, S., Holroyd, G. H., Spiegel, S., Panagopulos, et al. (2008). Involvement of sphingosine kinase in plant cell signalling. Plant J. 56, 64–72. doi: 10.1111/j.1365-313X.2008.03579.x
Wu, Y., Kuzma, J., Maréchal, E., Graeff, R., Lee, H. C., Foster, R., et al. (1997). Abscisic acid signaling through cyclic ADP-ribose in plants. Science 278, 2126–2130. doi: 10.1126/science.278.5346.2126
Xia, X. J., Gao, C. J., Song, L. X., Zhou, Y. H., Shi, K., and Yu, J. Q. (2014). Role of H2O2 dynamics in brassinosteroid-induced stomatal closure and opening in Solanum lycopersicum. Plant Cell Environ. 37, 2036–2050. doi: 10.1111/pce.12275
Yamashita, T. (1952). Influences of potassium supply upon various properties and movement of guard cell. Sielboldia Acta Biollogy 1, 51–70.
Yamazaki, D., Yoshida, S., Asami, T., and Kuchitsu, K. (2003). Visualization of abscisic acid-perception sites on the plasma membrane of stomatal guard cells. Plant J. 35, 129–139. doi: 10.1046/j.1365-313X.2003.01782.x
Yan, X. F., and Chen, S. (2007). Regulation of plant glucosinolate metabolism. Planta 226, 1343–1352. doi: 10.1007/s00425-007-0627-7
Yu, Y., and Assmann, S. M. (2014). Metabolite transporter regulation of ABA function and guard cell response. Mol. Plant 7, 1505–1507. doi: 10.1093/mp/ssu093
Yuan, X., Li, Y., Liu, S., Xia, F., Li, X., and Qi, B. (2014). Accumulation of eicosapolyenoic acids enhances sensitivity to abscisic acid and mitigates the effects of drought in transgenic Arabidopsis thaliana. J. Exp. Bot. 65, 1637–1649. doi: 10.1093/jxb/eru031
Zeiger, E., and Zhu, J. (1998). Role of zeaxanthin in blue light photoreception and the modulation of light-CO2 interactions in guard cells. J. Exp. Bot. 49, 433–442. doi: 10.1093/jxb/49.Special_Issue.433
Zeng, W., and He, S. Y. (2010). A prominent role of the flagellin receptor FLAGELLIN-SENSING2 in mediating stomatal response to Pseudomonas syringae pv tomato DC3000 in Arabidopsis. Plant Physiol. 153, 1188–1198. doi: 10.1104/pp.110.157016
Zhang, Y., Zhu, H., Zhang, Q., Li, M., Yan, M., Wang, R., et al. (2009). Phospholipase Dα1 and phosphatidic acid regulate NADPH oxidase activity and production of reactive oxygen species in ABA-mediated stomatal closure in Arabidopsis. Plant Cell 21, 2357–2377. doi: 10.1105/tpc.108.062992
Zhang, W., He, S. Y., and Assmann, S. M. (2008). The plant innate immunity response in stomatal guard cells invokes G-protein-dependent ion channel regulation. Plant J. 56, 984–996. doi: 10.1111/j.1365-313X.2008.03657.x
Zhang, W., Jeon, B. W., and Assmann, S. M. (2011). Heterotrimeric G-protein regulation of ROS signalling and calcium currents in Arabidopsis guard cells. J. Exp. Bot. 62, 2371–2379. doi: 10.1093/jxb/erq424
Zhang, T., Chen, S., and Harmon, A. (2014). Protein phosphorylation in stomatal movement. Plant Signal. Behav. 9:e972845. doi: 10.4161/15592316.2014.972845
Zhang, X. L., Jiang, L., Xin, Q., Liu, Y., Tan, J. X., and Chen, Z. Z. (2015). Structural basis and functions of abscisic acid receptors PYLs. Front. Plant Sci. 6:88. doi: 10.3389/fpls.2015.00088
Zhang, X., Dong, F. C., Gao, J. F., and Song, C. P. (2001). Hydrogen peroxide-induced changes in intracellular pH of guard cells precede stomatal closure. Cell Res. 11, 37–43. doi: 10.1038/sj.cr.7290064
Zhao, Z., and Assmann, S. M. (2011). The glycolytic enzyme, phosphoglycerate mutase, has critical roles in stomatal movement, vegetative growth, and pollen production in Arabidopsis thaliana. J. Exp. Bot. 62, 5179–5189. doi: 10.1093/jxb/err223
Zhao, Z., Zhang, W., Stanley, B. A., and Assmann, S. M. (2008). Functional proteomics of Arabidopsis thaliana guard cells uncovers new stomatal signaling pathways. Plant Cell 20, 3210–3226. doi: 10.1105/tpc.108.063263
Zhu, G. H., Liu, Y. G., Ye, N. H., Liu, R., and Zhang, J. H. (2011). Involvement of the abscisic acid catabolic gene CYP707A2 in the glucose-induced delay in seed germination and post-germination growth of Arabidopsis. Physiol. Plant. 143, 375–384. doi: 10.1111/j.1399-3054.2011.01510.x
Zhu, M., Dai, S., Zhu, N., Booy, A., Simons, B., Yi, S., et al. (2012). Methyl jasmonate responsive proteins in Brassica napus guard cells revealed by iTRAQ-based quantitative proteomics. J. Proteome Res. 11, 3728–3742. doi: 10.1021/pr300213k
Zhu, M., Simons, B., Zhu, N., Oppenheimer, D. G., and Chen, S. (2010). Analysis of abscisic acid responsive proteins in Brassica napus guard cells by multiplexed isobaric tagging. J. Proteomics 73, 790–805. doi: 10.1016/j.jprot.2009.11.002
Keywords: stomata, primary metabolites, abscisic acid, phytohormones, lipids, specialized metabolites, food security
Citation: Misra BB, Acharya BR, Granot D, Assmann SM and Chen S (2015) The guard cell metabolome: functions in stomatal movement and global food security. Front. Plant Sci. 6:334. doi: 10.3389/fpls.2015.00334
Received: 21 March 2015; Accepted: 28 April 2015;
Published: 19 May 2015.
Edited by:
Yariv Brotman, Max Planck Institute of Molecular Plant Physiology, GermanyReviewed by:
Norihito Nakamichi, Nagoya University, JapanMaria F. Drincovich, Rosario National University, Argentina
Copyright © 2015 Misra, Acharya, Granot, Assmann and Chen. This is an open-access article distributed under the terms of the Creative Commons Attribution License (CC BY). The use, distribution or reproduction in other forums is permitted, provided the original author(s) or licensor are credited and that the original publication in this journal is cited, in accordance with accepted academic practice. No use, distribution or reproduction is permitted which does not comply with these terms.
*Correspondence: Sixue Chen, Department of Biology, Genetics Institute, Plant Molecular and Cellular Biology Program, University of Florida, Cancer and Genetics Research Complex, Room 438, 2033 Mowry Road, Gainesville, FL 32610, USA, schen@ufl.edu