- 1Department of Agronomy, Food, Natural Resources, Animals and the Environment, University of Padova, Padova, Italy
- 2Department of Pharmaceutical and Pharmacological Sciences, University of Padova, Padova, Italy
- 3Department of Biology, Colorado State University, Fort Collins, CO, USA
Selenium (Se) at very low doses has crucial functions in humans and animals. Since plants represent the main dietary source of this element, Se-containing crops may be used as a means to deliver Se to consumers (biofortification). Several strategies have been exploited to increase plant Se content. Selenium assimilation in plants affects both sulfur (S) and nitrogen (N) metabolic pathways, which is why recent research has also focused on the effect of Se fertilization on the production of S- and N- secondary metabolites with putative health benefits. In this review we discuss the function of Se in plant and human nutrition and the progress in the genetic engineering of Se metabolism to increase the levels and bioavailability of this element in food crops. Particular attention is paid to Se biofortification and the synthesis of compounds with beneficial effects on health.
The Importance of Selenium to Human and Animal Health
Selenium is an essential trace element for humans and animals, and some organic forms like methyl-selenocysteine (MeSeCys) appear to be particularly effective sources of dietary Se. Selenium is incorporated as selenocysteine (SeCys) at the active site of a wide range of selenoproteins involved in major metabolic pathways, such as thyroid hormone metabolism, antioxidant defense and immune function (Rayman, 2012). Low intake of Se in the diet may cause a number of diseases, including heart diseases, hypothyroidism, reduced male fertility, weakened immune system and enhanced susceptibility to infections and cancer (Hatfield et al., 2014; Roman et al., 2014). Selenium deficiency is thought to affect 800 million people worldwide. In livestock, Se deficiency is also responsible for the white muscle disease, with clinical signs that include lesions in skeletal and/or heart muscle. Selenium supplementation of grazing livestock is mandatory in USA and Canada, because there is a marked seasonal and soil-dependent variation in their Se nutrition. For most of the world human and livestock population, vegetables are an important source of Se intake. Thus, increasing Se content in food crops offers an effective approach to reduce the Se deficiency problem in humans and animals.
Selenium Transport and Assimilation in Plants
While there is no proof of essentiality for Se in plants (Pilon-Smits et al., 2009), Se is readily taken up by plants in the form of selenate through the sulfate transporters (Figure 1). Due to their chemical similarities (Shibagaki et al., 2002; El Kassis et al., 2007), Se and sulfur (S) compete for the same transporters, and Se uptake is generally limited by high S levels. After uptake, selenate can access the sulfate assimilation pathway and be reduced via selenite to selenide (Figure 1). Selenide can be incorporated into the S-analog amino acid selenocysteine (SeCys), which may further be converted in three enzymatic steps to selenomethionine (SeMet; for a review, see Sors et al., 2005). The mistaken insertion of these Se-amino acids into proteins instead of cysteine and methionine may cause metabolic dysfunction (Sabbagh and Van Hoewyk, 2012). Incorporation of Se into proteins may be avoided by diverting Se to other, less toxic forms. Some plants accumulate the non-protein organic Se-compounds methylselenocysteine (MeSeCys), γ-glutamyl-MeSeCys and/or selenocystationine, sometimes to very high tissue levels without ill effects (Terry et al., 2000). Selenium can also be volatilized from plants in the forms of dimethylselenide or dimethyldiselenide, which are produced from SeMet and methyl-SeCys, respectively (Figure 1). The different selenocompounds found in plants have different toxicity levels and different nutritional value, with organic forms generally being more efficient in Se biofortification. Therefore, it is important to know which forms of Se are present in plant material used for nutritional supplementation. If we know which enzymes control the various metabolic steps it is also possible to genetically engineer more nutritious forms of Se in crop plants by enhancing the levels of critical enzymes.
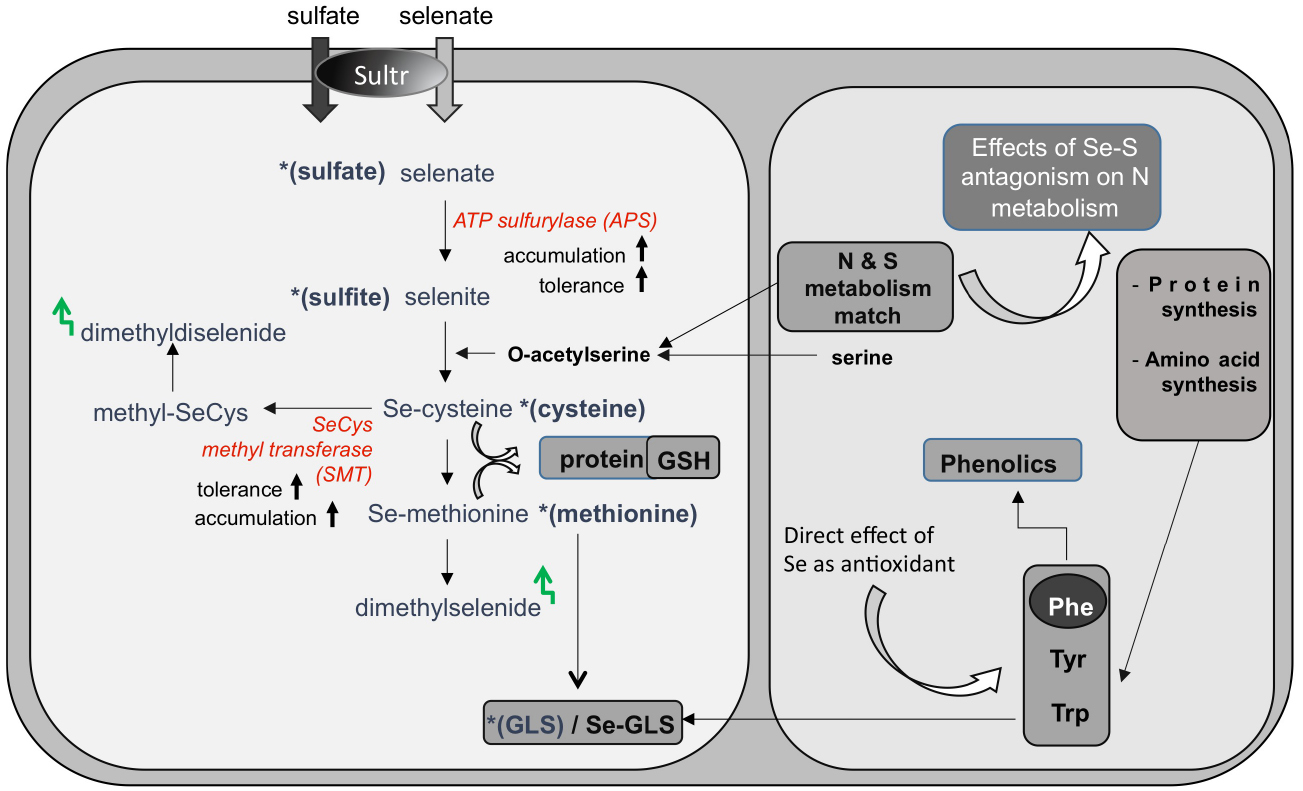
Figure 1. Selenate (and sulfate) uptake and assimilation in plants. Selenate is taken up by sulfate transporters (Sultr), and activated by ATP sulfurylase for further assimilation to selenocysteine (SeCys). SeCys can be further metabolized to selenomethionine and to volatile dimethylselenide. Non-hyperaccumulators often store selenate, because APS is a rate-limiting enzyme. Its overexpression resulted in enhanced Se accumulation and tolerance. Selenium hyperaccumulators methylate SeCys via the enzyme SeCys methyltransferase (SMT) and accumulate methyl-SeCys, a non-protein aminoacid. Methyl-SeCys may also be converted to volatile dimethyldiselenide. Expression of SMT in non-hyperaccumulators resulted in enhanced Se accumulation (as methylSeCys) and tolerance. Sulfur and nitrogen metabolic pathways interact at the level of -acetylserine. Changes in S assimilation induced by Se can in turn affect N metabolism, with respect to protein and amino acid synthesis. Amino acids methionine, phenylalanine (Phe), tyrosine (Tyr), and tryptophan (Trp) are precursors of glucosinolates (GLS) and Phe is a precursor for phenolics. Variation in the synthesis of these amino acids influence the production of nutraceutical compounds [glucosinolates (GLS) and phenolics]. In addition, Se can directly induce production of phenolics in plants.
Selenium Biofortification Efforts
Selenium is chemically analogous to S and therefore accumulated by all plants to some extent, in all plant parts. The plant Se levels found in nature and in crops depends on soil Se abundance and the levels of competing S compounds (Figure 2). In addition, plant Se concentrations at a given seleniferous site, i.e., a site containing more than 1 (and up to 100) mg Se kg–1 soil, may vary over 100-fold between plant species (Galeas et al., 2007). Different plant species differ with respect to their capacity to accumulate Se, which likely correlates with their expression levels of sulfate transporters. Plant species also vary with respect to which forms of Se they accumulate due to the presence and activity of various S/Se metabolic enzymes. Selenium biofortification efforts may make use of this natural variation between plant species, and choose crop species that naturally tend to contain higher Se (and S) levels, such as Brassica and Allium species (Terry et al., 2000). Since Se biofortification is most effective when organic Se is supplied, plant species known to accumulate organic forms of Se may be preferred, including broccoli and garlic (Lyi et al., 2005; Hsu et al., 2011). Care has to be taken to not supply unnecessary S in Se-fortified crop production, since S will reduce Se uptake. In soils where Se levels are very low, as e.g., in Finland, the United Kingdom, parts of China, and New Zealand (Chen et al., 2002; Broadley et al., 2006; Alfthan et al., 2014), it is not enough to just plant Se-accumulating crop species, but also necessary to provide inorganic Se as fertilizer for the crop. This practice is in effect in Finland since the 1980s, and has led to significantly enhanced blood Se levels in the general population (Alfthan et al., 2014). Whether this is concomitant with positive health effects remains to be investigated; a complicating factor is that there is no reference population. In Se-deficient areas of China, too, Se biofortification of crops is practiced to prevent the devastating Keshan disease still prevalent in vast areas, which is characterized by cardiomyopathy caused by Se deficiency (Bañuelos et al., 2013).
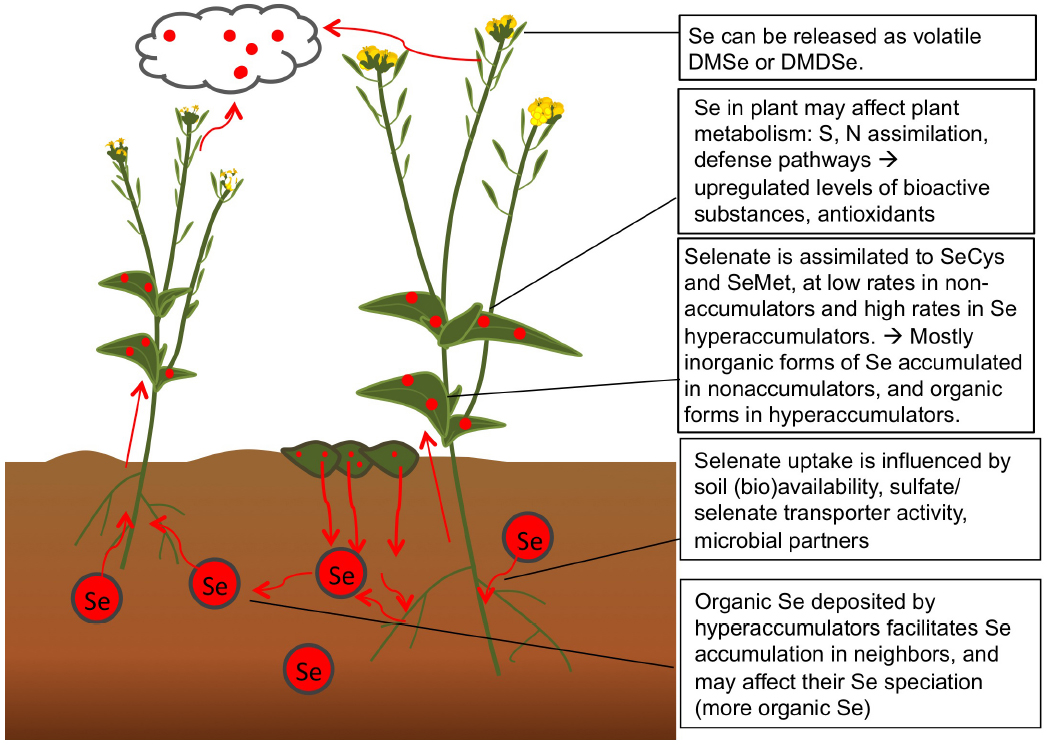
Figure 2. Processes related to Se in the soil-plant system, relevant for Se biofortification. Selenate is taken up from soil and assimilated (particularly by Se hyperaccumulators) to organic forms of Se. Some Se is accumulated and some volatiled as nontoxic dimethyl(di)selenide.
Genetic Engineering of Plant Se Metabolism and its Potential for Biofortification
Genetic engineering, which has been shown to enhance Se accumulation, tolerance, and volatilization by plants, has focused on S-related enzymes. First, overexpression in Brassica juncea of ATP sulfurylase (APS), a key enzyme for selenate-to-selenite transition, resulted in enhanced selenate reduction: the transgenic APS plants accumulated organic Se (likely methyl-SeCys) when supplied with selenate, while wildtype controls accumulated selenate (Pilon-Smits et al., 1999). The APS transgenics accumulated and tolerated more Se as well (Figure 1). In another approach, SeCys methyltransferase (SMT) was overexpressed in A. thaliana and B. juncea (Ellis et al., 2004; LeDuc et al., 2004). The SMT transgenics showed enhanced Se accumulation, and the form was methyl-SeCys (Figure 1). In both APS and SMT transgenics more Se is accumulated, and their form of Se is more suitable for biofortification (Figure 2).
When APS and SMT B. juncea transgenics were crossed to create double-transgenic plants, these accumulated up to 9 times higher Se levels than wild type (LeDuc et al., 2006). Most of the Se in the double transgenics was in the form of methyl-SeCys: the APSxSMT plants accumulated up to eightfold more methyl-SeCys than wild type and nearly twice as much as the SMT transgenics.
When grown on naturally seleniferous soil in a greenhouse pot experiment, the APS transgenics accumulated Se to threefold higher levels than wildtype B. juncea (Van Huysen et al., 2004). In two field experiments carried out on selenate-contaminated soil in central California, the APS transgenics accumulated fourfold higher Se levels than wildtype B. juncea, and SMT transgenics showed twofold higher Se levels (Bañuelos et al., 2005, 2007). Biomass production was comparable for the different plant types. Thus, genetic engineering has produced new genotypes of B. juncea with enhanced Se accumulation and higher levels of nutritious organic Se, all promising for use as Se-fortified foods.
In addition to the S assimilation enzymes, sulfate transporters may be potential targets of genetic engineering; selenate transporters from Se hyperaccumulators will be particularly interesting in this respect.
Effects of Se Biofortification on Secondary Plant Compounds
Variations in plant S uptake and assimilation induced by Se may cause changes in the synthesis of S-secondary compounds with nutritional value, such as glucosinolates (GLS), which function in plant defense against insects and herbivores (Figure 1). The hydrolysis of GLS within cells produces isothiocyanates, which act as cancer-preventing agents in mammals (Dinkova-Kostova, 2013).
Because S nutrition is strictly associated with N metabolism, Se can exert an additional effect on the synthesis of proteins and amino acids, as well as on N-secondary compounds with free radical scavenging activities, like phenolics (Figure 1). Amino acids such as methionine, phenylalanine (Phe), tyrosine (Tyr) and tryptophan (Trp) are precursors of GLS. Furthermore, Phe is the substrate for phenolics biosynthesis. Variation in the synthesis of these amino acids influence the production of both types of beneficial compounds.
Several studies examined how Se enrichment of plants affects their content in these phytochemicals (Robbins et al., 2005; Barickman et al., 2013; Schiavon et al., 2013). Tomato (Solanum lycopersicon L.) plants and Brassica species in particular, contain high levels of phenolic compounds. Additionally, Brassica spp. are rich in glucosinolates (GLSs).
In broccoli (Brassica oleracea L.), Se fertilization was shown to reduce the amount of total phenolic acids, without altering the profile distribution of specific compounds (Robbins et al., 2005). In contrast, Se at low dosages (5 and 10 μM) increased the leaf phenolic content of hydroponically grown tomato plants (Schiavon et al., 2013). Furthermore, the supply of selenate via foliar spray at 2 and 20 mg Se plant–1 resulted in Se-biofortified tomato fruits, with enhanced levels of the antioxidant flavonoids naringenin, chalcone and kaempferol (Schiavon et al., 2013).
Selenium fertilization may also affect the levels of GLS, a class of secondary plant S compounds. GLS may have anticarcinogenic properties, based on studies using experimental in vitro and in vivo models, but can also cause toxicity at elevated levels (Assayed and Abd El-Aty, 2009). The presence of GLS and GLS-metabolites at high level in animal feed can cause the decrease in growth and production, affecting organs such as liver, kidney, lungs and inducing morphological and physiological changes of thyroid (Tripathi and Mishra, 2007). Robbins et al. (2005) reported a weak reduction of indole, aliphatic, total glucosinolates, and glucoraphanin after Se fertilization, and a strong fall of sulforaphane production. A Se-related decrease of these compounds in broccoli was also observed by Barickman et al. (2013), but high levels of GLSs could be maintained with Se concentration lower than 0.8 mg L–1 or by increasing S concentration in the medium. Exposing plants to low Se concentrations can promote S uptake and assimilation in some species, including B. juncea (Harris et al., 2014), thus potentially increasing the level of S-organic compounds. However, while upregulating S uptake and assimilation, Se treatment was also found to upregulate genes involved in GLSs breakdown in A. thaliana (Van Hoewyk et al., 2008).
Recently, Ávila et al. (2013, 2014) showed the reduction of GLSs in the florets of broccoli treated with selenate, whereas in the sprouts GLS levels were not affected. Moreover, sprouts contained nearly sixfold higher content of the potent anticancer glucoraphanin than florets. Se-enriched sprouts were expected to exhibit greater potential anticancer activity because of high accumulation of SeMCys with similar glucosinolate production. Brassica crops supplied with selenate were able to form selenoglucosinolates, with a methylselenoalkyl group that was likely derived from selenomethionine (Matich et al., 2012). Selenoglucosinolates accounted for 60% of the concentrations of their S analogs (Matich et al., 2012). The production of selenoglucosinolates following Se-fertilization has implications for human health, as the synthetic Se-containing isothiocyanates are reported to be more potent anticancer compounds than their S counterparts (Emmert et al., 2010). As mentioned, the Se-GLS and/or GLS content must be monitored when plants or their residues are used for human or animal consumption, to avoid potential toxicity effects.
Future Prospects
Studies so far indicate that it is possible to maximize multiple bioactive components in a single plant. However, because in some cases the accumulation of Se may interfere with the production of some classes of phytochemicals, the Se biofortification programs must consider the interactions between Se and the main metabolic pathways of the plant. Particular attention should be paid to the reciprocal effects of Se and S on their accumulation and assimilation into organic compounds. In this context, managing S concentration during Se fertilization to vary S:Se ratios could be envisioned as a strategy to increase Se to beneficial dietary levels in plants without compromising GLS and other health-promoting compound contents.
An interesting new area of research involves the use of plant-microbe interactions to enhance Se biofortification. Another avenue to explore is the cultivation of Se fortified crops on seleniferous soil, thereby improving the amenity of that soil for further agriculture, and using the produced biomass to fortify the diets of people (and their livestock) in Se-deficient areas. Finally, since different plant species appear to be able to influence their neighboring plants’ Se accumulation and perhaps speciation (El Mehdawi et al., 2012), it will be interesting to further explore the potential of various co-cropping techniques to optimize crop Se biofortification and nutritional quality.
Conflict of Interest Statement
The authors declare that the research was conducted in the absence of any commercial or financial relationships that could be construed as a potential conflict of interest.
References
Alfthan, G., Eurola, M., Ekholm, P., Venäläinen, E. R., Root, T., Korkalainen, K., et al. (2014). Effects of nationwide addition of selenium to fertilizers on foods, and animal and human health in Finland: From deficiency to optimal selenium status of the population. J. Trace Elem. Med. Biol. doi: 10.1016/j.jtemb.2014.04.009 [Epub ahead of print].
PubMed Abstract | Full Text | CrossRef Full Text | Google Scholar
Assayed, M. E., and Abd El-Aty, A. M. (2009). Cruciferous plants: phytochemical toxicity versus cancer chemoprotection. Mini Rev. Med. Chem. 9, 1470–1478. doi: 10.2174/138955709790361511
PubMed Abstract | Full Text | CrossRef Full Text | Google Scholar
Ávila, F. W., Faquin, V., Yang, Y., Ramos, S. J., Guilherme, L. R., Thannhauser, T. W., et al. (2013). Assessment of the anticancer compounds Se-methylselenocysteine and glucosinolates in Se-biofortified broccoli (Brassica oleracea L. var. italica) sprouts and florets. J. Agric. Food Chem. 61, 6216–6223. doi: 10.1021/jf4016834
PubMed Abstract | Full Text | CrossRef Full Text | Google Scholar
Ávila, F. W., Yang, Y., Faquin, V., Ramos, S. J., Guilherme, L. R., Thannhauser, T. W., et al. (2014). Impact of selenium supply on Se-methylselenocysteine and glucosinolate accumulation in selenium-biofortified Brassica sprouts. Food Chem. 15, 578–586. doi: 10.1016/j.foodchem.2014.05.134
PubMed Abstract | Full Text | CrossRef Full Text | Google Scholar
Bañuelos, G., LeDuc, D. L., Pilon-Smits, E. A. H., Tagmount, A., and Terry, N. (2007). Transgenic Indian mustard overexpressing selenocysteine lyase or selenocysteine methyltransferase exhibit enhanced potential for selenium phytoremediation under field conditions. Environ. Sci. Technol. 41, 599–605. doi: 10.1021/es061152i
PubMed Abstract | Full Text | CrossRef Full Text | Google Scholar
Bañuelos, G. S., Lin, Z.-Q., and Yin, X. (2013). Selenium in the Environment and Human Health. Leiden: CRC Press. doi: 10.1201/b15960
Bañuelos, G., Terry, N., LeDuc, D. L., Pilon-Smits, E. A. H., and Mackey, B. (2005). Field trial of transgenic Indian mustard plants shows enhanced phytoremediation of selenium-contaminated sediment. Environ. Sci. Technol. 39, 1771–1777. doi: 10.1021/es049035f
PubMed Abstract | Full Text | CrossRef Full Text | Google Scholar
Barickman, T. C., Kopsell, D. A., and Sams, C. E. (2013). Selenium influences glucosinolate and isothiocyanates and increases sulfur uptake in Arabidopsis thaliana and rapid-cycling Brassica oleracea. J. Agric. Food Chem. 61, 202–209. doi: 10.1021/jf3037227
PubMed Abstract | Full Text | CrossRef Full Text | Google Scholar
Broadley, M. R., White, P. J., Bryson, R. J., Meacham, M. C., Bowen, H. C., Johnson, S. E., et al. (2006). Biofortification of UK food crops with selenium (Se). Proc. Nutr. Soc. 65, 169–181. doi: 10.1079/PNS2006490
PubMed Abstract | Full Text | CrossRef Full Text | Google Scholar
Chen, L., Yang, F., Xu, J., Hu, Y., Hu, Q., and Zhang, Y., et al. (2002). Determination of selenium concentration of rice in china and effect of fertilization of selenite and selenate on selenium content of rice. J. Agric. Food Chem. 50, 5128–5130. doi: 10.1021/jf0201374
PubMed Abstract | Full Text | CrossRef Full Text | Google Scholar
Dinkova-Kostova, A. T. (2013). Chemoprotection against cancer by isothiocyanates: a focus on the animal models and the protective mechanisms. Top. Curr. Chem. 329, 179–201. doi: 10.1007/128_2012_337
PubMed Abstract | Full Text | CrossRef Full Text | Google Scholar
El Kassis, E., Cathala, N., Rouached, H., Fourcroy, P., Berthomieu, P., Terry, N., et al. (2007). Characterization of a selenate-resistant Arabidopsis mutant. Root growth as a potential target for selenate toxicity. Plant Physiol. 143, 1231–1241. doi: 10.1104/pp.106.091462
PubMed Abstract | Full Text | CrossRef Full Text | Google Scholar
El Mehdawi, A. F., Cappa, J. J., Fakra, S. C., Self, J., and Pilon-Smits, E. A. H. (2012). Interactions of selenium and non-accumulators during co-cultivation on seleniferous or non-seleniferous soil—the importance of having good neighbors. New Phytol. 194, 264–277. doi: 10.1111/j.1469-8137.2011.04043.x
PubMed Abstract | Full Text | CrossRef Full Text | Google Scholar
Ellis, D. R., Sors, T. G., Brunk, D. G., Albrecht, C., Orser, C., Lahner, B., et al. (2004). Production of Se-methylselenocysteine in transgenic plants expressing selenocysteine methyltransferase. BMC Plant Biol. 4:1–11. doi: 10.1186/1471-2229-4-1
PubMed Abstract | Full Text | CrossRef Full Text | Google Scholar
Emmert, S. W., Desai, D., Amin, S., and Richie, J. P. (2010). Enhanced Nrf2-dependent induction of glutathione in mouse embryonic fibroblasts by isoselenocyanate analog of sulforaphane. Bioorg. Med. Chem. Lett. 20, 2675–2679. doi: 10.1016/j.bmcl.2010.01.044
PubMed Abstract | Full Text | CrossRef Full Text | Google Scholar
Galeas, M. L., Zhang, L. H., Freeman, J. L., Wegner, M., and Pilon-Smits, E. A. H. (2007). Seasonal fluctuations of selenium and sulfur accumulation in selenium hyperaccumulators and related non-accumulators. New Phytol. 173, 517–525. doi: 10.1111/j.1469-8137.2006.01943.x
PubMed Abstract | Full Text | CrossRef Full Text | Google Scholar
Harris, J., Schneberg, K. A., and Pilon-Smits, E. A. H. (2014). Sulfur—selenium—molybdenum interactions distinguish selenium hyperaccumulator Stanleya pinnata from non-hyperaccumulator Brassica juncea (Brassicaceae). Planta 239, 479–491. doi: 10.1007/s00425-013-1996-8
PubMed Abstract | Full Text | CrossRef Full Text | Google Scholar
Hatfield, D. L., Tsuji, P. A., Carlson, B. A., and Gladyshev, V. N. (2014). Selenium and selenocysteine: roles in cancer, health, and development. Trends Biochem. Sci. 39, 112–120. doi: 10.1016/j.tibs.2013.12.007
PubMed Abstract | Full Text | CrossRef Full Text | Google Scholar
Hsu, F.-C., Wirtz, M., Heppel, S., Bogs, J. Kramer, U., Khan, M. S., Bub, A., et al. (2011). Generation of Se-fortified broccoli as functional food: impact of Se fertilization on S metabolism. Plant Cell Environ. 34, 192–207. doi: 10.1111/j.1365-3040.2010.02235.x
PubMed Abstract | Full Text | CrossRef Full Text | Google Scholar
LeDuc, D. L., AbdelSamie, M., Montes-Bayón, M., Wu, C. P., Reisinger, S. J., and Terry, N. (2006). Overexpressing both ATP sulfurylase and selenocysteine methyltransferase enhances selenium phytoremediation traits in Indian mustard. Environ. Pollut. 44, 70–76. doi: 10.1016/j.envpol.2006.01.008
PubMed Abstract | Full Text | CrossRef Full Text | Google Scholar
LeDuc, D. L., Tarun, A. S., Montes-Bayon, M., Meija, J., Malit, M. F., Wu, C. P., et al. (2004). Overexpression of selenocysteine methyltransferase in Arabidopsis and indian mustard increases selenium tolerance and accumulation. Plant Physiol. 135, 377–383. doi: 10.1104/pp.103.026989
PubMed Abstract | Full Text | CrossRef Full Text | Google Scholar
Lyi, S. M., Heller, L. I., Rutzke, M., Welch, R. M., Kochian, L. V., and Li, L. (2005). Molecular and biochemical characterization of the selenocysteine Se-methyltransferase gene and Se-methylselenocysteine synthesis in broccoli. Plant Physiol. 138, 409–420. doi: 10.1104/pp.104.056549
PubMed Abstract | Full Text | CrossRef Full Text | Google Scholar
Matich, A. J., McKenzie, M. J., Lill, R. E., Brummell, D. A., McGhie, T. K., Chen, R. K., et al. (2012). Selenoglucosinolates and their metabolites produced in Brassica spp. fertilised with sodium selenate. Phytochemistry 75, 140–152. doi: 10.1016/j.phytochem.2011.11.021
PubMed Abstract | Full Text | CrossRef Full Text | Google Scholar
Pilon-Smits, E. A. H., Hwang, S. B., Lytle, C. M., Zhu, Y. L., Tai, J. C., Bravo, R. C., et al. (1999). Overexpression of ATP sulfurylase in Brassica juncea leads to increased selenate uptake, reduction and tolerance. Plant Physiol. 119, 123–132. doi: 10.1104/pp.119.1.123
PubMed Abstract | Full Text | CrossRef Full Text | Google Scholar
Pilon-Smits, E. A. H., Quinn, C. F., Tapken, W., Malagoli, M., and Schiavon, M. (2009). Physiological functions of beneficial elements. Curr. Opin. Plant Biol. 12, 267–274. doi: 10.1016/j.pbi.2009.04.009
PubMed Abstract | Full Text | CrossRef Full Text | Google Scholar
Rayman, M. P. (2012). Selenium and human health. Lancet 379, 1256–1268. doi: 10.1016/S0140-6736(11)61452-9
Robbins, R. J., Keck, A.-S., Banuelos, G., and Finley, J. W. (2005). Cultivation conditions and selenium fertilization alter the phenolic profile, glucosinolate, and sulforaphane content of broccoli. J. Med. Food 8, 204–214. doi: 10.1089/jmf.2005.8.204
PubMed Abstract | Full Text | CrossRef Full Text | Google Scholar
Roman, M., Jitaru, P., and Barbante, C. (2014). Selenium biochemistry and its role for human health. Metallomics 6, 25–54. doi: 10.1039/C3MT00185G
PubMed Abstract | Full Text | CrossRef Full Text | Google Scholar
Sabbagh, M., and Van Hoewyk, D. (2012). Malformed selenoproteins are removed by the ubiquitin—proteasome pathway in Stanleya pinnata. Plant Cell Physiol. 53, 555–564. doi: 10.1093/pcp/pcs015
PubMed Abstract | Full Text | CrossRef Full Text | Google Scholar
Schiavon, M., Dall’acqua, S., Mietto, A., Pilon-Smits, E. A., Sambo, P., Masi, A., et al. (2013). Selenium fertilization alters the chemical composition and antioxidant constituents of tomato (Solanum lycopersicon L.). J. Agric. Food Chem. 61, 10542–10554. doi: 10.1021/jf4031822
PubMed Abstract | Full Text | CrossRef Full Text | Google Scholar
Shibagaki, N., Rose, A., McDermott, J. P., Fujiwara, T., Hayashi, H., Yoneyama, T., et al. (2002). Selenate-resistant mutants of Arabidopsis thaliana identify Sultr1;2, a sulfate transporter required for efficient transport of sulfate into roots. Plant J. 29, 475–486. doi: 10.1046/j.0960-7412.2001.01232.x
PubMed Abstract | Full Text | CrossRef Full Text | Google Scholar
Sors, T. G., Ellis, D. R., and Salt, D. E. (2005). Selenium uptake, translocation, assimilation and metabolic fate in plants. Photosynth. Res. 86, 373–389. doi: 10.1007/s11120-005-5222-9
PubMed Abstract | Full Text | CrossRef Full Text | Google Scholar
Terry, N., Zayed, A. M., de Souza, M. P., and Tarun, A. S. (2000). Selenium in higher plants. Annu. Rev. Plant Physiol. Mol. Biol. 51, 401–432. doi: 10.1146/annurev.arplant.51.1.401
PubMed Abstract | Full Text | CrossRef Full Text | Google Scholar
Tripathi, M. K., and Mishra, A. S. (2007). Glucosinolate in animal nutrition: a review. Anim. Feed Sci. Tech. 132, 1–27. doi: 10.1016/j.anifeedsci.2006.03.003
Van Hoewyk, D., Takahashi, H., Hess, A., Tamaoki, M., and Pilon-Smits, E. A. H. (2008). Transcriptome and biochemical analyses give insights into selenium-stress responses and selenium tolerance mechanisms in Arabidopsis. Physiol. Plant. 132, 236–253. doi: 10.1111/j.1399-3054.2007.01002.x
PubMed Abstract | Full Text | CrossRef Full Text | Google Scholar
Van Huysen, T., Terry, N., and Pilon-Smits, E. A. H. (2004). Exploring the selenium phytoremediation potential of transgenic Brassica juncea overexpressing ATP sulfurylase or cystathionine-γ-synthase. Int. J. Phytoremed. 6, 111–118. doi: 10.1080/16226510490454786
PubMed Abstract | Full Text | CrossRef Full Text | Google Scholar
Keywords: selenium, plant biofortification, food, nutritional quality, secondary metabolites
Citation: Malagoli M, Schiavon M, dall’Acqua S and Pilon-Smits EAH (2015) Effects of selenium biofortification on crop nutritional quality. Front. Plant Sci. 6:280. doi: 10.3389/fpls.2015.00280
Received: 31 October 2014; Paper pending published: 11 December 2014;
Accepted: 08 April 2015; Published: 21 April 2015.
Edited by:
David W. M. Leung, University of Canterbury, New ZealandReviewed by:
Ruediger Hell, University of Heidelberg, GermanyGijs A. Kleter, Wageningen University and Research Centre, Netherlands
Copyright © 2015 Malagoli, Schiavon, dall’Acqua and Pilon-Smits. This is an open-access article distributed under the terms of the Creative Commons Attribution License (CC BY). The use, distribution or reproduction in other forums is permitted, provided the original author(s) or licensor are credited and that the original publication in this journal is cited, in accordance with accepted academic practice. No use, distribution or reproduction is permitted which does not comply with these terms.
*Correspondence: Mario Malagoli, Department of Agronomy, Food, Natural Resources, Animals and the Environment, University of Padova, Agripolis, 35020 Legnaro Padova, ItalybWFyaW8ubWFsYWdvbGlAdW5pcGQuaXQ=