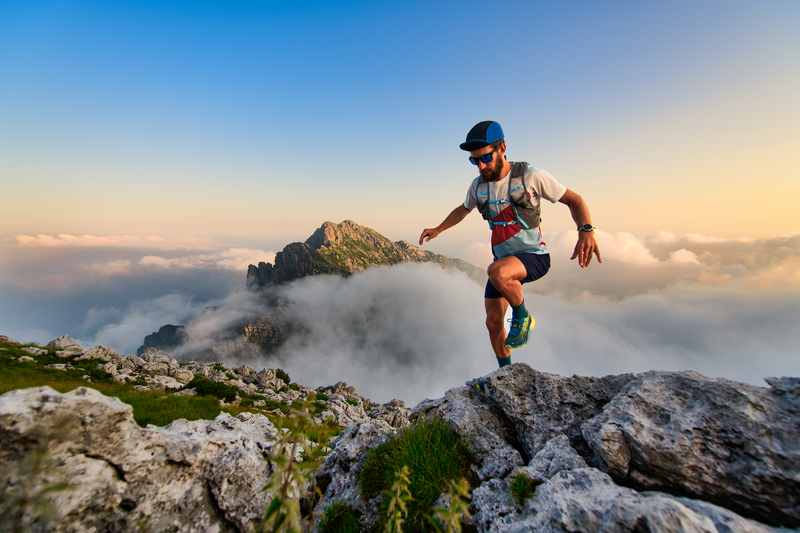
95% of researchers rate our articles as excellent or good
Learn more about the work of our research integrity team to safeguard the quality of each article we publish.
Find out more
REVIEW article
Front. Plant Sci. , 21 April 2015
Sec. Plant Physiology
Volume 6 - 2015 | https://doi.org/10.3389/fpls.2015.00223
This article is part of the Research Topic Plant cell wall integrity in plant development View all 12 articles
In the present review, we describe current knowledge about synthesis of borate crosslinked rhamnogalacturonan II (RG-II) and it physiological roles. RG-II is a portion of pectic polysaccharide with high complexity, present in primary cell wall. It is composed of homogalacturonan backbone and four distinct side chains (A–D). Borate forms ester bonds with the apiosyl residues of side chain A of two RG-II monomers to generate borate dimerized RG-II, contributing for the formation of networks of pectic polysaccharides. In plant cell walls, more than 90% of RG-II are dimerized by borate under boron (B) sufficient conditions. Borate crosslinking of RG-II in primary cell walls, to our knowledge, is the only experimentally proven molecular function of B, an essential trace-element. Although abundance of RG-II and B is quite small in cell wall polysaccharides, increasing evidence supports that RG-II and its borate crosslinking are critical for plant growth and development. Significant advancement was made recently on the location and the mechanisms of RG-II synthesis and borate cross-linking. Molecular genetic studies have successfully identified key enzymes for RG-II synthesis and regulators including B transporters required for efficient formation of RG-II crosslinking and consequent normal plant growth. The present article focuses recent advances on (i) RG-II polysaccharide synthesis, (ii) occurrence of borate crosslinking and (iii) B transport for borate supply to RG-II. Molecular mechanisms underlying formation of borate RG-II crosslinking and the physiological impacts are discussed.
Plant cell wall is composed of not only polysaccharides and proteins, but it also contains essential minerals including calcium (Ca) and boron (B). It has already been demonstrated that both elements are required for formation of networks of pectic polysaccharides in primary cell wall. Pectic networks are formed through crosslinking of pectic polysaccharide chains, and these crosslinkings are established in two different domains of pectin; homogalacturonan (HG) by Ca divalent cations and in rhamnogalacturonan II (RG-II) by borate.
B is one of essential micronutrient for plants and occurrence of B deficiency has been reported in both nature and agricultural practice (Shorrocks, 1997). B deficiency generally impairs cell elongation rather than cell division in growing tissues of plants. Typical B deficiency symptoms include inhibition of root cell elongation, leaf cell expansion and pollen tube elongation (Dell and Huang, 1997).
To our knowledge, borate crosslinking of RG-II in primary cell walls is only one proven function of B at the molecular level. Possible roles of B in membrane structure and in wall-membrane attachment have been also suggested (Blevins and Lukaszewski, 1998; Camacho-Cristóbal et al., 2008). The structure of RG-II-borate is well-characterized. RG-II is a pectic polysaccharide domain with high complexity, which is composed of HG backbone and four distinct side chains (A–D; Figure 1A). Borate forms ester bonds with the two apiosyl residues of side chain A of two RG-II monomers to generate borate dimerized RG-II, resulting in formation of networks of pectic polysaccharides (O’Neill et al., 2004). In plant cell walls, more than 90% of RG-II are dimerized by borate under B sufficient conditions. Abundance of RG-II is quite small in cell wall polysaccharides, however, increasing evidence supports that RG-II and its borate crosslinking are critical for plant growth and development by supporting cell adhesion and mechanical strength.
Figure 1. Schematic model of RG-II structure and its borate crosslinking. (A) A schematic model of RG-II structure. HG is a backbone of RG-II, and four distinct side chains (A–D) are linked to the backbone. Apiosyl residues in side chain A are covalently crosslinked by borate diester bond. Me Fuc: 2-O-methyl-L-fucose, Me Xyl: 2-O-methyl-D-xylose, Ace: aceric acid. (B) A schematic model of the reaction of borate dimerization of RG-II.
Then, how is such a complicated pectic polysaccharide RG-II synthesized and efficiently crosslinked by borate to be functional? The present article overviews recent advances on synthesis and physiological impacts of borate crosslinked RG-II in primary cell walls. Recent studies have provided novel clues to reveal molecular mechanisms of RG-II synthesis and subsequent borate crosslinking in vitro and in vivo. The article especially focuses on the processes in (i) RG-II polysaccharide synthesis, (ii) occurrence of borate crosslinking and (iii) B transport for borate supply to RG-II. Molecular genetic studies have uncovered glycosyl residues required for efficient borate dimerization in RG-II, through identification of enzymes involved in RG-II synthesis. Biochemical studies with the use of cultured cells suggest that borate RG-II dimerization occurs in the cytoplasm, possibly in Golgi or secretion pathways but not in apoplast, and also raise a new possibility that glycosylinositol phosphorylceramides could form a complex with RG-II to enhance borate dimerization of RG-II. Furthermore, studies on B transport also gave a new insight into preferential B distribution to cell walls and efficient dimerization of RG-II under limited B conditions for normal growth and development.
Pectin is composed of three major polysaccharides; (i) HG, (ii) rhamnogalacturonan I (RG-I), and (iii) RG-II (Atmodjo et al., 2013). In brief, pectic polysaccharides are synthesized through two steps, (1) synthesis of nucleotide monosaccharides as activated-sugar donor substrates in cytoplasm and (2) transfer of activated-sugar to poly- or monosaccharides acceptor in Golgi apparatus. The synthesized polysaccharides are subsequently incorporated into cell walls.
A fundamental mystery for RG-II domain synthesis is the order of polysaccharide synthesis and organization of the four side chains. One possible hypothesis could be that synthesized side chains or monosaccharides of side chains are transferred to preexisting HG backbone. Another possibility could be galacturonic acids (GalA) present at the end of each side chain are linked after full-length side chains are individually synthesized. It might also be possible that a RG-II specific sugar works as a reaction origin and monosaccharides are sequentially transferred. To date, overall picture of RG-II synthesis remains unclear. Recent studies in Arabidopsis thaliana successfully led to identification of several enzymes involved in synthesis of HG, a backbone of RG-II, and RG-II side chains.
HG, a major component of pectin, is a polymer of (1 → 4)-α-linked D-galacturonic acids (D-GalA) and is also a backbone of RG-II. HG is synthesized in Golgi apparatus by HG;galacturonosyltransferase (HG;GalAT) which catalyzes elongation of HG by transferring D-GalA from UDP-D-GalA to oligogalacturonan (Sterling et al., 2001; Akita et al., 2002; Ishii, 2002). HG polysaccharides are secreted to apoplast and incorporated into cell walls after methyl-esterification at O-6 position. In cell wall, some regions of methyl-esterified HG is de-esterified or acetylated at O-2 or 3 position. The de-esterified HGs are cross-linked by Ca2+ to form gel and this cross-linkage is essential for mechanical strength and plant growth (Hongo et al., 2012).
Over the last decade, molecular identity of HG;GalAT has been revealed. In A. thaliana, GAlactUronosylTransferase (GAUT) 1 belonging to glycosyltransferase (GT) 8 family was first shown to exhibit HG;GalAT activity (Sterling et al., 2006). GAUT1 is localized in the Golgi lumen as a complex with anchor protein GAUT7 and catalyzes HG biosynthesis (Atmodjo et al., 2011). In addition, homology search based on amino acid sequence of the protein has revealed that 15 GAUT and 10 GAUT-like (GATL) families are present in A. thaliana, respectively (Sterling et al., 2006). Fifteen AtGAUT genes excluding AtGAUT2 are ubiquitously expressed, and all 10 AtGATL genes are expressed with different tissues specificities (Caffall et al., 2009; Kong et al., 2011).
Until now, characterization of several mutants of GAUT and GATL genes has been conducted to depict their essential roles in cell morphology and physiological processes in A. thaliana. While either gaut13 or gaut14 single mutants did not show any morphological defects at whole plant level, inhibition of pollen tube elongation was found in the gaut13 gaut14 double mutants (Wang et al., 2013). In immunohistochemical analysis, HG amount was observed to be reduced in the cell wall of pollen tube in the double mutant. These results suggest that GAUT13 and GAUT14 redundantly function in HG synthesis of pollen tube walls and pollen tube elongation. Considering that the cell wall of pollen tube is relatively rich in pectin, defects of HG synthesis are considered to be more evident in these cells.
Increasing number of reports have suggested that GAUT genes are involved in not only HG synthesis but also in synthesis of other cell wall components. Single mutants of GAUT8 and GAUT12 exhibited dwarf phenotype with disruption of vascular bundle development, and reduction of cell adhesion was observed in gaut8 (Bouton et al., 2002; Orfila et al., 2005; Pena et al., 2007; Persson et al., 2007). Lower amounts of GalA and xylan, a component of hemicellulose in cell walls, were found in gaut8 and gaut12 than wild type. HG;GalAT activities were reduced in the microsome fraction isolated from these mutants, but HG;GalAT activity was not detected in GAUT12 in vitro. In addition, AtGATL5 and AtGAUT11 single mutants exhibited reduction of seed coat mucilage in which RG-I is contained (Caffall et al., 2009; Kong et al., 2013). In these mutants, amounts of GalA and rhamnose (Rha), monosaccharides composed of RG-I backbone, in extracted mucilage were reduced, showing that GATL5 and GAUT11 participate in both of HG and RG-I synthesis and mucilage synthesis. Since the amount and structure of RG-II were not examined in these studies, it is unknown that these GAUTs contribute to synthesis of RG-II backbone. Furthermore, as there is no direct evidence that these GAUTs possess enzymatic activities to transfer GalA to xylan or RG-I as acceptor polysaccharides, it is still possible that the disorders found in xylan and RG-I were just consequence of the secondary effect of reduced HG synthesis. However, assuming their diversity among the gene family, it is highly possible that some members of GAUTs and GATLs have roles in not only HG synthesis but also in synthesis of RG-I and hemicellulose.
To date, there are no reports indicating that mutations of GAUT and GATL genes affected RG-II synthesis. However, it is highly possible that some of GAUT and GATL genes are involved in synthesis of HG, corresponding to backbone of RG-II as a HG;GalAT. In addition, as GalA is also present in side chain A, some of them might play a specific role as a GalA transferase to synthesize side chain A.
As mentioned above, RG-II is highly complicated but conserved polysaccharides among plant species, which has HG as a backbone and four side chains (A–D) constituted of 12 different monosaccharides. RG-II is covalently cross-linked by borate diester bonds between the apiosyl residues in side chain A (O’Neill et al., 2004; Atmodjo et al., 2013). Through identification of monosaccharide synthases and transferases for RG-II synthesis (Table 1), it is further demonstrated that normal RG-II structure is key to efficient and stable crosslinking of RG-II by borate.
Side chain A is composed of seven different monosaccharides, L-fucose (Fuc), Api, glucuronic acid (GlcA), L-galactose (Gal), methyl-xylose (Xyl), GalA and Rha. It has been demonstrated that the normal structure of side chain A is essential for borate crosslinking. In A. thaliana, MURUS1 (MUR1) encodes GDP-D-mannose-4,6-dehydratase required for synthesis of L-Fuc, a component monosaccharide found in RG-II. In mur1 mutant plants, inhibition of leaf expansion and reduced RG-II crosslinking rate were observed (O’Neill et al., 2001) possibly because of truncated side chain A (Pabst et al., 2013). Application of B restored leaf expansion of mur1, accompanied with increased proportion of RG-II crosslinking (O’Neill et al., 2001). This suggested that RG-II crosslinking by borate and leaf expansion was positively correlated, and borate crosslinking of RG-II was essential for normal leaf expansion. This study with mur1 is the first example which shows that normal RG-II structure is essential for efficient RG-II crosslinking by borate and plant growth.
Furthermore, in this decade, several key monosaccharide synthases for RG-II side chains have been identified through genetic studies. Api is present in both side chains A and B and apiosyl residues in side chain A are the sites where borate diester bonds are formed. UDP-Api is synthesized by UDP-D-Api/UDP-D-Xyl synthase (AXS1; Molhoj et al., 2003). In Nicotiana benthamiana, silencing of AXS1 expression caused arrest of plant growth associated with decrease in amounts of side chains A and B (Ahn et al., 2006). UDP-glucuronic acid is a key precursor for production in Ara, Xyl, GlcA, and Api found in cell walls including RG-II. Synthesis of UDP-glucuronic acid is mediate by UDP-glucose dehydrogenase (UGD). The double mutant of UGD2 and UGD3 led to dwarfism and impaired fertility with remarkable reduction of side chains A and B (Reboul et al., 2011).
L-Gal is a monosaccharide present in side chain A. Silencing of GME, GDP-D-mannose 3,5-epimerase, required for L-Gal synthesis caused dwarfism associated with reduction of L-Gal in cell walls and RG-II crosslinking rate in tomato (Gilbert et al., 2009; Voxeur et al., 2011). Importantly, high level of B supply rescued growth defects of GME-silenced tomato plants, accompanied with recovery of RG-II crosslinking rate, similar to the case of A. thaliana mur1 mutants. In addition, RGXT1, RGXT2, and RGXT3 were found to encode (1,3)-α-D-xylosyltransferase capable of transferring Xyl to L-Fuc in vitro, probably for synthesis of side chain A (Egelund et al., 2006, 2008). Among the four RGXT members, mutations in RGXT4 were shown to be responsible for male gametophyte defective 4 (mgp4) mutant phenotype in A. thaliana (Liu et al., 2011). Mutations in RGXT4 inhibited elongation of roots and pollen tube, and reduced Xyl amount and RG-II dimerization by 20% in cell wall fraction compared to wild type plants (Liu et al., 2011). Growth disorders of rgxt4 mutant plants were also restored by high B application to increase proportion of RG-II crosslinking, suggesting that RGXT4 is also involved in synthesis of side chain A. Growth recovery in these mutants by high B supply further supports a view that reduced RG-II crosslinking impairs plant growth and that normal structure of side chain A is important for formation of borate crosslinked RG-II.
RG-II contains an eight carbon sugar, 3-deoxy-D-manno-2-octulosonic acid (Kdo) in side chain C, probably unique to RG-II in plants. Kdo is a component of lipid A in Gram-negative bacteria. Recent reports raise a possibility that lipid A-like molecules are synthesized even in plants, however, it is not yet confirmed that Kdo is included. Kdo is synthesized as Kdo-8-phosphate by KDSA encoding Kdo-8-phosphate synthase. After dephosphorylation, Kdo is converted to activated form, CMP-Kdo, by CMP-KDO synthetase (CKS) encoding CTP:KDO cytidylyltransferase. The double mutant of AtKDSA1 and AtKDSA2 was not obtained due to impaired pollen tube elongation (Delmas et al., 2008). Similarly, insertional mutations in CKS gene inhibited pollen tube elongation, resulting in absence of homozygous mutant plants (Kobayashi et al., 2011). It should be noted that recombinant CKS protein exhibited the enzymatic activity in vitro, and is localized in mitochondria but not Golgi lumen in planta (Kobayashi et al., 2011). It was found that a putative Kdo transferase KDTA was also localized in mitochondria, but the RG-II structure was not affected in the null-mutant of KDTA, unlikely involved in RG-II synthesis (Seveno et al., 2010). Recently, bioinformatics analysis has made a list of candidate genes involved in RG-II synthesis by co-expression analysis (Voxeur et al., 2012). Among them, two putative sialyltransferase-like proteins (SIA1, SIA2) were included and mutations of the corresponding genes were found to inhibit pollen tube elongation (Deng et al., 2010; Dumont et al., 2014). Although there is no experimental evidence for enzymatic activity, it is suggested that these genes, rather than the gene designated as KDTA, encode enzymes to transfer Kdo in side chain C and/or 3-deoxy-D-lyxo-2-heptulosaric acid (Dha) in side chain D, which has similar characteristics to Kdo.
As described above, enzymes required for RG-II synthesis has been increasingly identified but identification of most of the transferases is still missing in the pathway. Bioinformatic analysis is expected to become a powerful tool to identify novel monosaccharide synthases and transferases that specifically function in RG-II synthesis as mentioned (Voxeur et al., 2012). One interesting feature in some loss-of-function mutants is growth recovery by exogenous application of B associated with increased proportion of RG-II crosslinking possibly due to change of movement of chemical equilibrium (monomeric RG-II + borate ⇄ borate dimerized RG-II). This observation suggests that incomplete structure of RG-II reduces dimerization efficiency or stability of dimerized RG-II, and this highly complicated structure is required for RG-II dimerization and its function. It is possible that mutant plants with altered B nutrient properties might include mutants defective in RG-II synthesis. Further identification of the enzyme and its localization will reveal whole pathway of RG-II synthesis.
The timing, the location and the mechanisms that underlie dimerization of two RG-II polysaccharides by borate are still unknown, after RG-II is synthesized in Golgi. Two possible sites for the dimerization reaction are Golgi and apoplast, however, in either case, organization of pectic networks with dimerized RG-II seems puzzling. If RG-II is cross-linked inside Golgi prior to secretion to apoplast, it is unclear how cross-linked RG-II complex is precisely incorporated in two independent HG chains. Alternatively, if borate crosslinking is formed in apoplast, how can two RG-II polysaccharides efficiently find each other to make 90% of RG-II crosslinked in cell wall?
To date, the presence of enzymes catalyzing borate crosslinking of RG-II has not been revealed (Figure 1B). It has been demonstrated that borate crosslinking can occur only with isolated RG-II and boric acid in the presence of divalent cations in vitro (O’Neill et al., 1996), implying that any particular molecule/enzyme is not necessarily required for the reaction. However, these reconstitution experiments were conducted with isolated RG-II by endopolygalacturonase digestion and relatively higher B concentration at mM level. It is known that the B concentration in root cell sap of A. thaliana grown under normal B conditions is in the range of μM level (Takano et al., 2002). The reconstitution experiments conducted in the previous studies may not reflect physiological situations in plant cells. For the RG-II dimerization to occur under the physiological concentration of B, it is likely that molecules/enzymes are present that facilitate and/or stabilize borate crosslinking of RG-II in planta.
One recent study with Rosa cultured cells provides a significant implication for sites and timing of this reaction (Chormova et al., 2014). In this report, a novel method to detect dimeric and monomeric RG-II by gel electrophoresis was first established. As HPLC connected with refractive index detector has been widely used, this technique is quite useful to compare a series of samples in one gel. It was uncovered that application of boric acid in media increased dimeric RG-II but preexisting monomeric RG-II remained in Rosa cultured cells, suggesting that newly synthesized RG-II is capable to form borate dimerized RG-II. This idea was further supported by the observation that RG-II domain supplied in media did not form dimerized RG-II and was not incorporated to preexisting RG-II in cell walls. These results clearly show that borate crosslinking occurs during RG-II synthesis in Golgi and/or secretion into apoplast.
Another recent report from the same group raises a possibility that glycosylinositol phosphorylceramides (GIPCs) present in lipid raft are able to promote borate RG-II dimerization with the use of Rosa cultured cell (Voxeur and Fry, 2014). The experiment by paper electrophoresis pointed out the possible interaction between GIPC and RG-II in the presence of boric acid in vitro, implying the formation of complex of GIPC and RG-II linked by borate. More importantly, application of GIPCs was found to enhance borate dimerization of RG-II in vitro. Although these experiments were performed in vitro, this study provides a new hypothesis for the process of RG-II dimerization. In addition to borate dimerization of RG-II, degradation of borate crosslinking and RG-II turnover will be an intriguing point.
How is boric acid/borate supplied to RG-II to form dimerized RG-II? Boric acid utilized in the cell wall is derived from boric acid present in soil solution. B is mainly present as boric acid in soil solution and plants uptake boric acid from soil into root cells, and translocate into aerial portion of the plants through xylem. Since boric acid is a small non-charged molecule under physiological pH (pKa 9.24), it shows relatively high permeability to lipid membrane compared to the other minerals. Based on this, it had been long considered that boric acid was transported only by passive diffusion across membrane along with transpiration stream within plants. Recent studies have identified two types of B transport molecules required for efficient transport of boric acid under limited B conditions, directly affecting borate crosslinking of RG-II.
One type is NIPs. NIPs are boric acid channels belonging to major intrinsic protein (MIP) family which includes aquaporins. In A. thaliana, NIP5;1 was first identified as a boric acid channel which facilitates uptake of boric acid from external environments into root cells under low B conditions (Takano et al., 2006). NIP5;1 is localized to outer domain of plasma membrane in root epidermis of root tip and elongation zone (Takano et al., 2010). Under limited B conditions, A. thaliana mutant plants of NIP5;1 exhibited lower B concentrations in root and shoot tissues, and inhibition of root cell elongation and leaf expansion. These impaired growth is considered to be caused by reduction of borate cross-linked RG-II as high B supply in media increased B concentrations in these tissues and rescued the growth suppression. It suggests that initial uptake of boric acid is an essential process to satisfy the B demand in the whole tissues to establish borate crosslinked RG-II when B concentrations in media is limited.
A. thaliana NIP6;1, a paralogous gene to NIP5;1, is shown to participate in preferential distribution of boric acid to growing leaves (Tanaka et al., 2008). NIP6;1 is expressed in vascular bundles particularly in phloem regions including companion cells. Disruption of NIP6;1 restricts B distribution in young leaves, consequently causing inhibition of upper leaf expansion. Assuming that crosslinking of RG-II by borate is required when new cell walls are constructed in the developing cells, boric acid should be continuously supplied to the sink organs in order to maintain RG-II crosslinking in the newly emerging cells.
Recently, homologs in other plant species, OsNIP3;1 in Oryza sativa and ZmNIP3;1 in Zea mays, have been shown to play similar roles to AtNIP5;1 and AtNIP6;1 for efficient uptake and distribution of boric acid under limited B supply. In OsNIP3;1 RNAi rice plants, the whole plant size was reduced compared to the wild type plants under limited B availability and B distribution patterns in aerial portion of the rice plants was altered (Hanaoka et al., 2014). In maize, tassel-less1 (tls1) was previously isolated as a mutant which exhibited impaired vegetative growth and development of tassels and ears. It was revealed that these growth disorders in tls1 mutants were caused by mutations in ZmNIP3;1 encoding a boric acid channel (Durbak et al., 2014; Leonard et al., 2014). In tsl1 mutants, growth defects including reduced size of shoot apical meristem and impaired development of inflorescence were observed, and reduction of borate dimerized RG-II in immature tassels was experimentally detected. These phenotypes are obviously found in low-B environments whereas high B application rescued. These studies further confirm that overall uptake and subsequent distribution of boric acid is essential for formation of borate cross-linked RG-II and normal growth and development.
The other type is a boric acid /borate efflux-type transporter BOR1 which shows similarity to anion exchangers found in yeast and animals. A. thaliana BOR1 was first identified from bor1-1 mutant plants defective in xylem loading in roots and preferential distribution of B into young leaves in shoots (Takano et al., 2001, 2002). BOR1 is predominately expressed in inner domain of plasma membrane of endodermis in roots (Takano et al., 2010) and is crucial for efficient translocation of B from roots to shoots to maintain B levels in leaves under B starvation. In bor1-1 mutant plants, the B concentrations in shoots are dramatically decreased and expansion of upper leaves is severely inhibited under limited B availability. In bor1-1, it is revealed that sugar composition of RG-II is not affected and proportion of borate dimerized RG-II is reduced to 40% whereas 90% of RG-II is crosslinked in wild type plants (Noguchi et al., 2003). These results support an idea that RG-II synthesis and B nutrient status do not affect each other, and further the local B concentration in the cells is one determinant for crosslinking of RG-II.
BOR2, the most similar to BOR1 in A. thaliana genome, is considered to be responsible for cellular distribution of B to RG-II in roots. Loss of function of BOR2 inhibits root cell elongation and decreases relative proportion of RG-II crosslinking in roots although the total B concentrations in roots were not significantly different from those of wild type plants under limited B conditions (Miwa et al., 2013). This finding suggests that BOR2 is involved in preferential distribution of B into RG-II at the cellular level under low B conditions. BOR2 appears to be predominately localized in plasma membrane and be recycled between plasma membrane and endosomes for polar localization in inner domain of plasma membrane. Although molecular mechanisms underlying B transport into RG-II through BOR2 are not yet determined, localization of BOR2 raises a possibility that incorporation of B into RG-II could be induced either in plasma membrane or in endosome/secretion vesicles.
As one possible hypothesis, when we assume that BOR2 localized in plasma membrane exports B into RG-II in apoplast, borate crosslinking of RG-II could occur beneath the plasma membrane in apoplast. In this case, direct or indirect interactions between BOR2 and RG-II monomer or between BOR2 and facilitators including GIPCs might be necessary for efficient delivery of B to RG-II monomer because exported boric acid/borate by BOR2 into apoplast could easily return back to cytosol due to the high permeability. Interaction between BOR2 and GIPCs present in plasma membrane for efficient RG-II dimerization would be an interesting hypothesis.
As the second possible hypothesis, BOR2 localized in endosomes/secretion vesicles could increase B concentrations in these vesicles to enhance the crosslinking reaction inside the vesicles, since BOR2 in the vesicles is likely able to transport B from cytosol into the vesicles. Among the BOR family in A. thaliana, BOR4, encoding a B exporter, is also localized to plasma membrane in roots but functions in B exclusion out of the cells against B toxicity when B is present in excess (Miwa et al., 2007, 2014). Overexpression of BOR4 confers high-B tolerance but results in higher sensitivity to B deficiency due to lower B concentrations in roots. Assuming that BOR4 may not be localized to endosomes, the finding possibly implies that localization of B exporters in endosomes/secretion vesicles is required for B delivery to RG-II by increasing B concentrations inside the vesicles. This second hypothesis supports a view suggested by Chormova et al. (2014) that borate crosslinking of RG-II occurs during RG-II synthesis in Golgi or secretion.
Orthologous genes to AtBOR1 have been increasingly identified in O. sativa (Nakagawa et al., 2007), in Triticum aestivum (Leaungthitikanchana et al., 2013), Vitis vinifera (Perez-Castro et al., 2012), and Z. mays (Chatterjee et al., 2014), suggesting that BOR1 genes are required for efficient B translocation to ensure sufficient supply for RG-II dimerization under B starvation in a wide range of plant species. In maize mutant named rotten ear, impaired development of tassels and ears was proven to be attributed to the point mutations in ZmBOR1/RTE (Chatterjee et al., 2014). Observation by TEM clarified that organization of cell walls was disrupted and cell adhesion was loosened in the ears of the rte mutant. Exogenous application of high B restored these adverse effects. Together with the study of tls1 maize mutants, these researches support that optimization of B transport by B transporters is required for normal development of inflorescence and fertility even in monocot species, which generally require lower amount of B compared to dicots because of lower amount of pectin in cell walls. In addition, OsBOR4, one of four BOR genes present in O. sativa, is found to be specifically expressed in pollen (Tanaka et al., 2013). Rice plants homozygous for Tos17 transposon insertion were obtained and they exhibited fertility comparable to wild type plants, however, occurrence of the mutant homozygous plants was lower than expected in the progeny derived from self-fertilization of the heterozygous mutant plants and germination rate of mutant pollens in pistils were lower than those of wild type plants. Assuming that high amount of B needs to accumulate in the growing tip of pollen tube (Iwai et al., 2006), B transporters locally deliver boric acid possibly into RG-II in pollen, resulting in regulation of germination and/or elongation of pollen, and consequent fertilization.
We still have a number of questions to address: How are the four side chains of RG-II synthesized and the structure is organized? Is there any enzyme that facilitates borate crosslinking? How does “borate crosslinked RG-II” regulate cell elongation? Does borate cross-linked RG-II not only determine cell wall properties but also function as a signaling component to regulate growth?
For gelation of pectin, Ca2+ bridges between HG polysaccharides might be sufficient. Why have plants evolved to utilize B, such a unique element, and RG-II for cell walls? Plants have developed defense systems against pathogens including fungi that are able to degrade HG by the activities of pectin methylesterase and endopolygalacturonase. Plant cell wall is the frontiers in the competition between plants and pathogens, and therefore plants have mechanisms in which oligo-galacturonide, degraded HG, functions as a signal molecule that represents disruption of cell walls. Since borate crosslinking has not been reported to be hydrolyzed by fungi, borate crosslinked RG-II could be free from the attack by fungi and prevent complete degradation of pectic polysaccharides. Evolutionary aspects in occurrence of cell wall degradation enzymes in fungi and novel components in plant cell wall should be focused.
Further identification of novel players for borate crosslinking of RG-II, such as GT, a membrane lipid and B transporters, will lead to understanding a whole figure of synthesis and physiological impacts of borate crosslinked RG-II in plants.
The authors declare that the research was conducted in the absence of any commercial or financial relationships that could be construed as a potential conflict of interest.
This work was supported by the Ministry of Education, Culture, Sports, Science, and Technology (MEXT), Japan (a Grant-in-Aid for Scientific Research on Innovative Areas grant number 25114502); and the Japan Science and Technology Agency (JST) (PRESTO).
Ahn, J. W., Verma, R., Kim, M., Lee, J. Y., Kim, Y. K., Bang, J. W., et al. (2006). Depletion of UDP-D-apiose/UDP-D-xylose synthases results in rhamnogalacturonan-II deficiency, cell wall thickening, and cell death in higher plants. J. Biol. Chem. 281, 13708–13716. doi: 10.1074/jbc.M512403200
Pubmed Abstract | Pubmed Full Text | CrossRef Full Text | Google Scholar
Akita, K., Ishimizu, T., Tsukamoto, T., Ando, T., and Hase, S. (2002). Successive glycosyltransfer activity and enzymatic characterization of pectic polygalacturonate 4-α-galacturonosyltransferase solubilized from pollen tubes of Petunia axillaris using pyridylaminated oligogalacturonates as substrates. Plant Physiol. 130, 374–379. doi: 10.1104/pp.005587
Pubmed Abstract | Pubmed Full Text | CrossRef Full Text | Google Scholar
Atmodjo, M. A., Hao, Z., and Mohnen, D. (2013). Evolving views of pectin biosynthesis. Annu. Rev. Plant Biol. 64, 747–779. doi: 10.1146/annurev-arplant-042811-105534
Pubmed Abstract | Pubmed Full Text | CrossRef Full Text | Google Scholar
Atmodjo, M. A., Sakuragi, Y., Zhu, X., Burrell, A. J., Mohanty, S. S., Atwood, J. A., et al. (2011). Galacturonosyltransferase (GAUT)1 and GAUT7 are the core of a plant cell wall pectin biosynthetic homogalacturonan: galacturonosyltransferase complex. Proc. Natl. Acad. Sci. U.S.A. 108, 20225–20230. doi: 10.1073/pnas.1112816108
Pubmed Abstract | Pubmed Full Text | CrossRef Full Text | Google Scholar
Blevins, D. G., and Lukaszewski, K. M. (1998). Boron in plant structure and function. Annu. Rev. Plant Biol. 49, 481–500. doi: 10.1146/annurev.arplant.49.1.481
Pubmed Abstract | Pubmed Full Text | CrossRef Full Text | Google Scholar
Bouton, S., Leboeuf, E., Mouille, G., Leydecker, M. T., Talbotec, J., Granier, F., et al. (2002). QUASIMODO1 encodes a putative membrane-bound glycosyltransferase required for normal pectin synthesis and cell adhesion in Arabidopsis. Plant Cell 14, 2577–2590. doi: 10.1105/tpc.004259
Pubmed Abstract | Pubmed Full Text | CrossRef Full Text | Google Scholar
Caffall, K. H., Pattathil, S., Phillips, S. E., Hahn, M. G., and Mohnen, D. (2009). Arabidopsis thaliana T-DNA mutants implicate GAUT genes in the biosynthesis of pectin and xylan in cell walls and seed testa. Mol. Plant 2, 1000–1014. doi: 10.1093/mp/ssp062
Pubmed Abstract | Pubmed Full Text | CrossRef Full Text | Google Scholar
Camacho-Cristóbal, J. J., Rexach, J., and González-Fontes, A. (2008). Boron in plants: deficiency and toxicity. J. Integr. Plant Biol. 50, 1247–1255. doi: 10.1111/j.1744-7909.2008.00742.x
Pubmed Abstract | Pubmed Full Text | CrossRef Full Text | Google Scholar
Chatterjee, M., Tabi, Z., Galli, M., Malcomber, S., Buck, A., Muszynski, M., et al. (2014). The boron efflux transporter ROTTEN EAR is required for maize inflorescence development and fertility. Plant Cell 26, 2962–2977. doi: 10.1105/tpc.114.125963
Pubmed Abstract | Pubmed Full Text | CrossRef Full Text | Google Scholar
Chormova, D., Messenger, D. J., and Fry, S. C. (2014). Boron bridging of rhamnogalacturonan-II, monitored by gel electrophoresis, occurs during polysaccharide synthesis and secretion but not post-secretion. Plant J. 77, 534–546. doi: 10.1111/tpj.12403
Pubmed Abstract | Pubmed Full Text | CrossRef Full Text | Google Scholar
Dell, B., and Huang, L. B. (1997). Physiological response of plants to low boron. Plant Soil 193, 103–120. doi: 10.1023/A:1004264009230
Delmas, F., Seveno, M., Northey, J. G., Hernould, M., Lerouge, P., Mccourt, P., et al. (2008). The synthesis of the rhamnogalacturonan II component 3-deoxy-D-manno-2-octulosonic acid (Kdo) is required for pollen tube growth and elongation. J. Exp. Bot. 59, 2639–2647. doi: 10.1093/jxb/ern118
Pubmed Abstract | Pubmed Full Text | CrossRef Full Text | Google Scholar
Deng, Y., Wang, W., Li, W. Q., Xia, C., Liao, H. Z., Zhang, X. Q., et al. (2010). MALE GAMETOPHYTE DEFECTIVE 2, encoding a sialyltransferase-like protein, is required for normal pollen germination and pollen tube growth in Arabidopsis. J. Integr. Plant Biol. 52, 829–843. doi: 10.1111/j.1744-7909.2010.00963.x
Pubmed Abstract | Pubmed Full Text | CrossRef Full Text | Google Scholar
Dumont, M., Lehner, A., Bouton, S., Kiefer-Meyer, M. C., Voxeur, A., Pelloux, J., et al. (2014). The cell wall pectic polymer rhamnogalacturonan-II is required for proper pollen tube elongation: implications of a putative sialyltransferase-like protein. Ann. Bot. 114, 1177–1188. doi: 10.1093/aob/mcu093
Pubmed Abstract | Pubmed Full Text | CrossRef Full Text | Google Scholar
Durbak, A. R., Phillips, K. A., Pike, S., O’Neill, M. A., Mares, J., Gallavotti, A., et al. (2014). Transport of boron by the tassel-less1 aquaporin is critical for vegetative and reproductive development in maize. Plant Cell 26, 2978–2995. doi: 10.1105/tpc.114.125898
Pubmed Abstract | Pubmed Full Text | CrossRef Full Text | Google Scholar
Egelund, J., Damager, I., Faber, K., Olsen, C. E., Ulvskov, P., and Petersen, B. L. (2008). Functional characterisation of a putative rhamnogalacturonan II specific xylosyltransferase. FEBS Lett. 582, 3217–3222. doi: 10.1016/j.febslet.2008.08.015
Pubmed Abstract | Pubmed Full Text | CrossRef Full Text | Google Scholar
Egelund, J., Petersen, B. L., Motawia, M. S., Damager, I., Faik, A., Olsen, C. E., et al. (2006). Arabidopsis thaliana RGXT1 and RGXT2 encode Golgi-localized (1,3)-alpha-D-xylosyltransferases involved in the synthesis of pectic rhamnogalacturonan-II. Plant Cell 18, 2593–2607. doi: 10.1105/tpc.105.036566
Pubmed Abstract | Pubmed Full Text | CrossRef Full Text | Google Scholar
Gilbert, L., Alhagdow, M., Nunes-Nesi, A., Quemener, B., Guillon, F., Bouchet, B., et al. (2009). GDP-D-mannose 3,5-epimerase (GME) plays a key role at the intersection of ascorbate and non-cellulosic cell-wall biosynthesis in tomato. Plant J. 60, 499–508. doi: 10.1111/j.1365-313X.2009.03972.x
Pubmed Abstract | Pubmed Full Text | CrossRef Full Text | Google Scholar
Hanaoka, H., Uraguchi, S., Takano, J., Tanaka, M., and Fujiwara, T. (2014). OsNIP3;1, a rice boric acid channel, regulates boron distribution and is essential for growth under boron-deficient conditions. Plant J. 78, 890–902. doi: 10.1111/tpj.12511
Pubmed Abstract | Pubmed Full Text | CrossRef Full Text | Google Scholar
Hongo, S., Sato, K., Yokoyama, R., and Nishitani, K. (2012). Demethylesterification of the primary wall by PECTIN METHYLESTERASE35 provides mechanical support to the Arabidopsis stem. Plant Cell 24, 2624–2634. doi: 10.1105/tpc.112.099325
Pubmed Abstract | Pubmed Full Text | CrossRef Full Text | Google Scholar
Ishii, T. (2002). A sensitive and rapid bioassay of homogalacturonan synthase using 2-aminobenzamide-labeled oligogalacturonides. Plant Cell Physiol. 43, 1386–1389. doi: 10.1093/Pcp/Pcf150
Pubmed Abstract | Pubmed Full Text | CrossRef Full Text | Google Scholar
Iwai, H., Hokura, A., Oishi, M., Chida, H., Ishii, T., Sakai, S., et al. (2006). The gene responsible for borate cross-linking of pectin Rhamnogalacturonan-II is required for plant reproductive tissue development and fertilization. Proc. Natl. Acad. Sci. U.S.A. 103, 16592–16597. doi: 10.1073/pnas.0605141103
Pubmed Abstract | Pubmed Full Text | CrossRef Full Text | Google Scholar
Kobayashi, M., Kouzu, N., Inami, A., Toyooka, K., Konishi, Y., Matsuoka, K., et al. (2011). Characterization of Arabidopsis CTP:3-deoxy-D-manno-2-octulosonate cytidylyltransferase (CMP-KDO synthetase), the enzyme that activates KDO during rhamnogalacturonan II biosynthesis. Plant Cell Physiol. 52, 1832–1843. doi: 10.1093/pcp/pcr120
Pubmed Abstract | Pubmed Full Text | CrossRef Full Text | Google Scholar
Kong, Y., Zhou, G., Abdeen, A. A., Schafhauser, J., Richardson, B., Atmodjo, M. A., et al. (2013). GALACTURONOSYLTRANSFERASE-LIKE5 is involved in the production of Arabidopsis seed coat mucilage. Plant Physiol. 163, 1203–1217. doi: 10.1104/pp.113.227041
Pubmed Abstract | Pubmed Full Text | CrossRef Full Text | Google Scholar
Kong, Y., Zhou, G., Yin, Y., Xu, Y., Pattathil, S., and Hahn, M. G. (2011). Molecular analysis of a family of Arabidopsis genes related to galacturonosyltransferases. Plant Physiol. 155, 1791–1805. doi: 10.1104/pp.110.163220
Pubmed Abstract | Pubmed Full Text | CrossRef Full Text | Google Scholar
Leaungthitikanchana, S., Fujibe, T., Tanaka, M., Wang, S., Sotta, N., Takano, J., et al. (2013). Differential expression of three BOR1 genes corresponding to different genomes in response to boron conditions in hexaploid wheat (Triticum aestivum L.). Plant Cell Physiol. 54, 1056–1063. doi: 10.1093/pcp/pct059
Pubmed Abstract | Pubmed Full Text | CrossRef Full Text | Google Scholar
Leonard, A., Holloway, B., Guo, M., Rupe, M., Yu, G., Beatty, M., et al. (2014). tassel-less1 encodes a boron channel protein required for inflorescence development in maize. Plant Cell Physiol. 55, 1044–1054. doi: 10.1093/pcp/pcu036
Pubmed Abstract | Pubmed Full Text | CrossRef Full Text | Google Scholar
Liu, X. L., Liu, L., Niu, Q. K., Xia, C., Yang, K. Z., Li, R., et al. (2011). MALE GAMETOPHYTE DEFECTIVE 4 encodes a rhamnogalacturonan II xylosyltransferase and is important for growth of pollen tubes and roots in Arabidopsis. Plant J. 65, 647–660. doi: 10.1111/j.1365-313X.2010.04452.x
Pubmed Abstract | Pubmed Full Text | CrossRef Full Text | Google Scholar
Miwa, K., Aibara, I., and Fujiwara, T. (2014). Arabidopsis thaliana BOR4 is upregulated under high boron conditions and confers tolerance to high boron. Soil Sci. Plant Nutr. 60, 349–355. doi: 10.1080/00380768.2013.866524
Miwa, K., Takano, J., Omori, H., Seki, M., Shinozaki, K., and Fujiwara, T. (2007). Plants tolerant of high boron levels. Science 318, 1417. doi: 10.1126/science.1146634
Pubmed Abstract | Pubmed Full Text | CrossRef Full Text | Google Scholar
Miwa, K., Wakuta, S., Takada, S., Ide, K., Takano, J., Naito, S., et al. (2013). Roles of BOR2, a boron exporter, in cross linking of rhamnogalacturonan II and root elongation under boron limitation in Arabidopsis. Plant Physiol. 163, 1699–1709. doi: 10.1104/pp.113.225995
Pubmed Abstract | Pubmed Full Text | CrossRef Full Text | Google Scholar
Molhoj, M., Verma, R., and Reiter, W. D. (2003). The biosynthesis of the branched-chain sugar D-apiose in plants: functional cloning and characterization of a UDP-D-apiose/UDP-D-xylose synthase from Arabidopsis. Plant J. 35, 693–703. doi: 10.1046/j.1365-313X.2003.01841.x
Pubmed Abstract | Pubmed Full Text | CrossRef Full Text | Google Scholar
Nakagawa, Y., Hanaoka, H., Kobayashi, M., Miyoshi, K., Miwa, K., and Fujiwara, T. (2007). Cell-type specificity of the expression of Os BOR1, a rice efflux boron transporter gene, is regulated in response to boron availability for efficient boron uptake and xylem loading. Plant Cell 19, 2624–2635. doi: 10.1105/tpc.106.049015
Pubmed Abstract | Pubmed Full Text | CrossRef Full Text | Google Scholar
Noguchi, K., Ishii, T., Matsunaga, T., Kakegawa, K., Hayashi, H., and Fujiwara, T. (2003). Biochemical properties of the cell wall in the Arabidopsis mutant bor1-1 in relation to boron nutrition. J. Soil Sci. Plant Nutr. 166, 175–178. doi: 10.1002/jpln.200390025
O’Neill, M. A., Eberhard, S., Albersheim, P., and Darvill, A. G. (2001). Requirement of borate cross-linking of cell wall rhamnogalacturonan II for Arabidopsis growth. Science 294, 846–849. doi: 10.1126/science.1062319
Pubmed Abstract | Pubmed Full Text | CrossRef Full Text | Google Scholar
O’Neill, M. A., Ishii, T., Albersheim, P., and Darvill, A. G. (2004). Rhamnogalacturonan II: structure and function of a borate cross-linked cell wall pectic polysaccharide. Annu. Rev. Plant Biol. 55, 109–139. doi: 10.1146/annurev.arplant.55.031903.141750
Pubmed Abstract | Pubmed Full Text | CrossRef Full Text | Google Scholar
O’Neill, M. A., Warrenfeltz, D., Kates, K., Pellerin, P., Doco, T., Darvill, A. G., et al. (1996). Rhamnogalacturonan-II, a pectic polysaccharide in the walls of growing plant cell, forms a dimer that is covalently cross-linked by a borate ester. J. Biol. Chem. 271, 22923–22930. doi: 10.1074/jbc.271.37.22923
Pubmed Abstract | Pubmed Full Text | CrossRef Full Text | Google Scholar
Orfila, C., Sorensen, S. O., Harholt, J., Geshi, N., Crombie, H., Truong, H. N., et al. (2005). QUASIMODO1 is expressed in vascular tissue of Arabidopsis thaliana inflorescence stems, and affects homogalacturonan and xylan biosynthesis. Planta 222, 613–622. doi: 10.1007/s00425-005-0008-z
Pubmed Abstract | Pubmed Full Text | CrossRef Full Text | Google Scholar
Pabst, M., Fischl, R. M., Brecker, L., Morelle, W., Fauland, A., Kofeler, H., et al. (2013). Rhamnogalacturonan II structure shows variation in the side chains monosaccharide composition and methylation status within and across different plant species. Plant J. 76, 61–72. doi: 10.1111/tpj.12271
Pubmed Abstract | Pubmed Full Text | CrossRef Full Text | Google Scholar
Pena, M. J., Zhong, R., Zhou, G. K., Richardson, E. A., O’Neill, M. A., Darvill, A. G., et al. (2007). Arabidopsis irregular xylem8 and irregular xylem9: implications for the complexity of glucuronoxylan biosynthesis. Plant Cell 19, 549–563. doi: 10.1105/tpc.106.049320
Pubmed Abstract | Pubmed Full Text | CrossRef Full Text | Google Scholar
Perez-Castro, R., Kasai, K., Gainza-Cortes, F., Ruiz-Lara, S., Casaretto, J. A., Pena-Cortes, H., et al. (2012). VvBOR1, the grapevine ortholog of AtBOR1, encodes an efflux boron transporter that is differentially expressed throughout reproductive development of Vitis vinifera L. Plant Cell Physiol. 53, 485–494. doi: 10.1093/pcp/pcs001
Pubmed Abstract | Pubmed Full Text | CrossRef Full Text | Google Scholar
Persson, S., Caffall, K. H., Freshour, G., Hilley, M. T., Bauer, S., Poindexter, P., et al. (2007). The Arabidopsis irregular xylem8 mutant is deficient in glucuronoxylan and homogalacturonan, which are essential for secondary cell wall integrity. Plant Cell 19, 237–255. doi: 10.1105/tpc.106.047720
Pubmed Abstract | Pubmed Full Text | CrossRef Full Text | Google Scholar
Reboul, R., Geserick, C., Pabst, M., Frey, B., Wittmann, D., Luetz-Meindl, U., et al. (2011). Down-regulation of UDP-glucuronic acid biosynthesis leads to swollen plant cell walls and severe developmental defects associated with changes in pectic polysaccharides. J. Biol. Chem. 286, 39982–39992. doi: 10.1074/jbc.M111.255695
Pubmed Abstract | Pubmed Full Text | CrossRef Full Text | Google Scholar
Seveno, M., Seveno-Carpentier, E., Voxeur, A., Menu-Bouaouiche, L., Rihouey, C., Delmas, F., et al. (2010). Characterization of a putative 3-deoxy-D-manno-2-octulosonic acid (Kdo) transferase gene from Arabidopsis thaliana. Glycobiology 20, 617–628. doi: 10.1093/glycob/cwq011
Pubmed Abstract | Pubmed Full Text | CrossRef Full Text | Google Scholar
Shorrocks, V. M. (1997). The occurrence and correction of boron deficiency. Plant Soil 193, 121–148. doi: 10.1023/A:1004216126069
Sterling, J. D., Atmodjo, M. A., Inwood, S. E., Kolli, V. S. K., Quigley, H. F., Hahn, M. G., et al. (2006). Functional identification of an Arabidopsis pectin biosynthetic homogalacturonan galacturonosyltransferase. Proc. Natl. Acad. Sci. U.S.A. 103, 5236–5241. doi: 10.1073/pnas.0600120103
Pubmed Abstract | Pubmed Full Text | CrossRef Full Text | Google Scholar
Sterling, J. D., Quigley, H. F., Orellana, A., and Mohnen, D. (2001). The catalytic site of the pectin biosynthetic enzyme α-1,4-galacturonosyltransferase is located in the lumen of the Golgi. Plant Physiol. 127, 360–371. doi: 10.1104/Pp.127.1.360
Pubmed Abstract | Pubmed Full Text | CrossRef Full Text | Google Scholar
Takano, J., Noguchi, K., Yasumori, M., Kobayashi, M., Gajdos, Z., Miwa, K., et al. (2002). Arabidopsis boron transporter for xylem loading. Nature 420, 337–340. doi: 10.1038/nature01139
Pubmed Abstract | Pubmed Full Text | CrossRef Full Text | Google Scholar
Takano, J., Tanaka, M., Toyoda, A., Miwa, K., Kasai, K., Fuji, K., et al. (2010). Polar localization and degradation of Arabidopsis boron transporters through distinct trafficking pathways. Proc. Natl. Acad. Sci. U.S.A. 107, 5220–5225. doi: 10.1073/pnas.0910744107
Pubmed Abstract | Pubmed Full Text | CrossRef Full Text | Google Scholar
Takano, J., Wada, M., Ludewig, U., Schaaf, G., Von Wiren, N., and Fujiwara, T. (2006). The Arabidopsis major intrinsic protein NIP5;1 is essential for efficient boron uptake and plant development under boron limitation. Plant Cell 18, 1498–1509. doi: 10.1105/tpc.106.041640
Pubmed Abstract | Pubmed Full Text | CrossRef Full Text | Google Scholar
Takano, J., Yamagami, M., Noguchi, K., Hayashi, H., and Fujiwara, T. (2001). Preferential translocation of boron to young leaves in Arabidopsis thaliana regulated by the BOR1 gene. Soil Sci. Plant Nutr. 47, 345–357. doi: 10.1080/00380768.2001.10408398
Tanaka, M., Wallace, I. S., Takano, J., Roberts, D. M., and Fujiwara, T. (2008). NIP6;1 is a boric acid channel for preferential transport of boron to growing shoot tissues in Arabidopsis. Plant Cell 20, 2860–2875. doi: 10.1105/tpc.108.058628
Pubmed Abstract | Pubmed Full Text | CrossRef Full Text | Google Scholar
Tanaka, N., Uraguchi, S., Saito, A., Kajikawa, M., Kasai, K., Sato, Y., et al. (2013). Roles of pollen-specific boron efflux transporter, OsBOR4, in the rice fertilization process. Plant Cell Physiol. 54, 2011–2019. doi: 10.1093/pcp/pct136
Pubmed Abstract | Pubmed Full Text | CrossRef Full Text | Google Scholar
Voxeur, A., Andre, A., Breton, C., and Lerouge, P. (2012). Identification of putative rhamnogalacturonan-II specific glycosyltransferases in Arabidopsis using a combination of bioinformatics approaches. PLoS ONE 7:e51129. doi: 10.1371/journal.pone.0051129
Pubmed Abstract | Pubmed Full Text | CrossRef Full Text | Google Scholar
Voxeur, A., and Fry, S. C. (2014). Glycosylinositol phosphorylceramides from Rosa cell cultures are boron-bridged in the plasma membrane and form complexes with rhamnogalacturonan II. Plant J. 79, 139–149. doi: 10.1111/tpj.12547
Pubmed Abstract | Pubmed Full Text | CrossRef Full Text | Google Scholar
Voxeur, A., Gilbert, L., Rihouey, C., Driouich, A., Rothan, C., Baldet, P., et al. (2011). Silencing of the GDP-D-mannose 3,5-epimerase affects the structure and cross-linking of the pectic polysaccharide rhamnogalacturonan II and plant growth in tomato. J. Biol. Chem. 286, 8014–8020. doi: 10.1074/jbc.M110.198614
Pubmed Abstract | Pubmed Full Text | CrossRef Full Text | Google Scholar
Wang, L., Wang, W., Wang, Y. Q., Liu, Y. Y., Wang, J. X., Zhang, X. Q., et al. (2013). Arabidopsis galacturonosyltransferase (GAUT) 13 and GAUT14 have redundant functions in pollen tube growth. Mol. Plant 6, 1131–1148. doi: 10.1093/mp/sst084
Pubmed Abstract | Pubmed Full Text | CrossRef Full Text | Google Scholar
Keywords: Arabidopsis thaliana, borate, glycosyltransferase, homogalacturonan, rhamnogalacturonan-II, pectin, primary cell wall, transporter
Citation: Funakawa H and Miwa K (2015) Synthesis of borate cross-linked rhamnogalacturonan II. Front. Plant Sci. 6:223. doi: 10.3389/fpls.2015.00223
Received: 16 February 2015; Accepted: 21 March 2015;
Published: 21 April 2015.
Edited by:
Masaru Fujimoto, The University of Tokyo, JapanReviewed by:
Aline Voxeur, Institut National de la Recherche Agronomique Versailles, FranceCopyright © 2015 Funakawa and Miwa. This is an open-access article distributed under the terms of the Creative Commons Attribution License (CC BY). The use, distribution or reproduction in other forums is permitted, provided the original author(s) or licensor are credited and that the original publication in this journal is cited, in accordance with accepted academic practice. No use, distribution or reproduction is permitted which does not comply with these terms.
*Correspondence: Kyoko Miwa, Division of Biosphere Science, Graduate School of Environmental Science, Hokkaido University, North-10, West-5, Kita-ku, Sapporo, Hokkaido 060-0810, JapanbWl3YWt5b2tvQGVlcy5ob2t1ZGFpLmFjLmpw
Disclaimer: All claims expressed in this article are solely those of the authors and do not necessarily represent those of their affiliated organizations, or those of the publisher, the editors and the reviewers. Any product that may be evaluated in this article or claim that may be made by its manufacturer is not guaranteed or endorsed by the publisher.
Research integrity at Frontiers
Learn more about the work of our research integrity team to safeguard the quality of each article we publish.