- Department of Biology, Memorial University of Newfoundland, St. John’s, NL, Canada
Rubisco is the most abundant protein on Earth that serves as the primary engine of carbon assimilation. It is characterized by a slow rate and low specificity for CO2 leading to photorespiration. We analyze here the challenges of operation of this enzyme as the main carbon fixation engine. The high concentration of Rubisco exceeds that of its substrate CO2 by 2–3 orders of magnitude; however, the total pool of available carbon in chloroplast, i.e., mainly bicarbonate, is comparable to the concentration of Rubisco active sites. This makes the reactant stationary assumption (RSA), which is essential as a condition of satisfying the Michaelis–Menten (MM) kinetics, valid if we assume that the delivery of CO2 from this pool is not limiting. The RSA is supported by active carbonic anhydrases (CA) that quickly equilibrate bicarbonate and CO2 pools and supply CO2 to Rubisco. While the operation of stromal CA is independent of light reactions, the thylakoidal CA associated with PSII and pumping CO2 from the thylakoid lumen is coordinated with the rate of electron transport, water splitting and proton gradient across the thylakoid membrane. At high CO2 concentrations, CA becomes less efficient (the equilibrium becomes unfavorable), so a deviation from the MM kinetics is observed, consistent with Rubisco reaching its Vmax at approximately 50% lower level than expected from the classical MM curve. Previously, this deviation was controversially explained by the limitation of RuBP regeneration. At low ambient CO2 and correspondingly limited capacity of the bicarbonate pool, its depletion at Rubisco sites is relieved in that the enzyme utilizes O2 instead of CO2, i.e., by photorespiration. In this process, CO2 is supplied back to Rubisco, and the chloroplastic redox state and energy level are maintained. It is concluded that the optimal performance of photosynthesis is achieved via the provision of continuous CO2 supply to Rubisco by carbonic anhydrases and photorespiration.
Introduction
Carbon fixation can be achieved by chemically simple processes, and it is enigmatic that it occurs via the complex mechanism mediated by a slow enzyme with dual specificity. Rubisco serves as the primary engine of carbon assimilation being the most abundant protein on Earth and characterized by a slow rate of catalysis (in higher plants the value of k is approx. 3 s-1) and low specificity for CO2 leading to photorespiration. As a simple alternative, the reduction of CO2 to formate which is then metabolized to carbohydrates and other compounds (C1-pathway of carbon assimilation) is possible. This mechanism, with no connection to the reductive photosynthetic carbon pathway (Calvin–Benson cycle), exists in some prokaryotes (Tagawa and Arnon, 1962). The reaction of carbohydrate synthesis from one-carbon compounds was first demonstrated in organic chemistry by Butlerow (1861); then Baeyer (1870) suggested formaldehyde as the primary product of CO2 fixation by plants. This hypothesis dominated before the discovery of the Calvin–Benson cycle in the 1940s (Benson and Calvin, 1950). Whereas it is now widely accepted that formate is not involved in the primary CO2 assimilation processes in plants, it may still have a role in side-reactions of photosynthesis and photorespiration, also including a possibility of a very minor CO2 fixation pathway via formate and formaldehyde (Kent, 1972; reviewed in Igamberdiev et al., 1999). However, this simple pathway did not acquire major significance during evolution and instead the enzyme Rubisco became the primary engine of carbon assimilation. In this paper, we demonstrate that by using Rubisco, which seems to be inefficient due to low turnover number and low specificity, living systems in fact exploit the mechanism that provides the most optimal parameters of carbon fixation. This is realized by coordinating CO2 assimilation with generation of reducing power and energy currency and with oxygen utilization.
Conditions for Michaelis–Menten Kinetics
It is widely assumed that all enzymes follow the Michaelis–Menten (MM) kinetics. The derivation of MM equation is usually based on the steady-state assumption (SSA) that the concentration of substrate (S) should be much higher than the concentration of enzyme (E), so practically all the enzyme during the reaction exists in the state of enzyme-substrate complex (ES). Recently it was shown that the SSA condition is not crucial, while essential is the reactant stationary assumption (RSA). It means that there is an initial transient phase, during which the initial substrate concentration remains approximately constant, while the ES complex concentration builds up (Schnell, 2014). This truly necessary condition does not require the restrictive limitation of choosing a substrate concentration that is much higher than the enzyme concentration in initial rate experiments. For the RSA to be valid, there must be only a negligible decrease in S during the initial transient phase. An auxiliary enzyme that supplies substrate from the reserve pool can provide this condition. This enzyme (called the buffering enzyme) should be fast as compared to the enzyme present in high concentration (called the engine enzyme; Igamberdiev and Kleczkowski, 2009). The combination of the fast (buffering) enzyme catalyzing the equilibrium reaction and the slow (engine) enzyme catalyzing the non-equilibrium reaction ensures that the total reaction rate is optimized via constant building up of the ES complex of the engine enzyme. This condition can be defined as the stable non-equilibrium principle following Bauer (1935).
Even at lower concentrations of substrate, the MM kinetics may be valid if the substrate is constantly delivered from its major reserve pool. The simplest way of such delivery occurs via a buffering enzyme. This was demonstrated earlier for ATP synthase, when its substrate (ADP) is delivered by the buffering enzyme adenylate kinase (Igamberdiev and Kleczkowski, 2003, 2006, 2015). Another example is glycine decarboxylase, when the buffering of its end products, NADH and CO2, by malate dehydrogenase and carbonic anhydrase (CA), respectively, is important for maintaining its maximal activity (Bykova et al., 2014). The same role of malate dehydrogenase has been established for the mitochondrial pyruvate dehydrogenase complex, which in plants has also the mechanism of substrate delivery via NAD-malic enzyme (Igamberdiev et al., 2014). For Rubisco, the condition of optimization of CO2 supply was outlined previously (Igamberdiev and Roussel, 2012), where it was assumed that neither SSA nor RSA conditions could be satisfied due to a very low CO2 concentration in chloroplast stroma as compared to Rubisco concentration.
In the current paper, it is suggested that the situation is not so far-reaching due to a high CA capacity making the MM kinetics generally valid for Rubisco, while the restrictions appear at low CO2 (the oxygenase reaction present) and high CO2 (CA equilibrium is less favorable) concentrations. The whole bicarbonate pool of chloroplast can be considered as a substrate for Rubisco, provided that CO2 is efficiently delivered to its active sites. This is reflected in Figure 1, which depicts the conditions of SSA, RSA, and MM, depending on CO2 and Rubisco concentrations. It shows also actual concentration values for Rubisco and CO2 in planta if we consider either only the dissolved molecular CO2 or the CO2 that can be delivered from the bicarbonate reserve pool. The kinetic analysis suggests that in the region where the RSA is valid but the SSA is not, the estimates of Vmax and Km are highly inflated (Hanson and Schnell, 2008). The increase in Vmax indicates that the rate of reaction can be increased significantly at the expense of the affinity to substrate; however, the conditions of the steady delivery of substrate by the buffering enzyme successfully overcome this limitation.
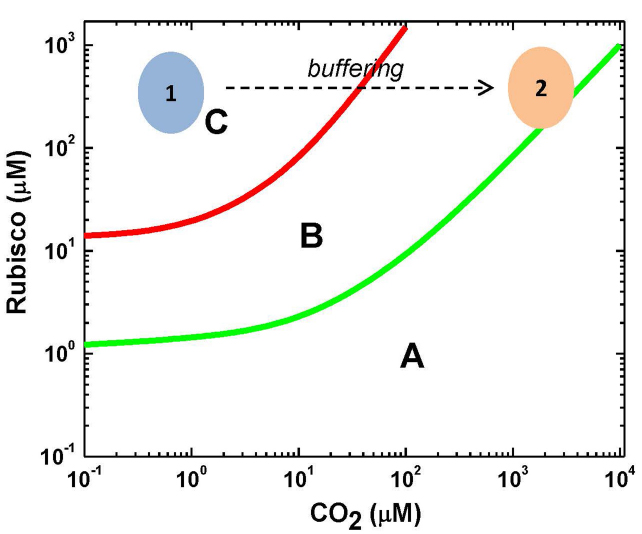
Figure 1. Limits of validity of SSA and RSA for the Rubisco reaction based on the approach developed in Hanson and Schnell (2008). Area (A): both the RSA and SSA are valid and the reaction obeys the MM equation. Area (B): the SSA is valid but the RSA is not. Applying the MM equation is possible provided that the decrease in substrate concentration is negligible during the initial transition. This can be achieved by thermodynamic buffering. Area (C): neither the QSSA nor the RSA is valid, substrate is rapidly depleted by enzyme and its continuous influx is necessary for steady reaction. The area 1 marks the in vivo condition if we assume that only CO2 is used as a substrate, while the area 2 marks the in vivo condition when we assume that the whole bicarbonate/CO2 pool is used as a substrate, provided that CO2 is efficiently delivered by the buffering enzyme carbonic anhydrase.
Carbonic Anhydrase and CO2 Delivery Capacity
The buffering of energy intermediates is the most widespread energy source in biological systems, and filling buffer reservoirs corresponds to the accumulation of free energy (Shnoll, 1979). Within the framework of the theory of dissipative structures (Prigogine, 1967), thermodynamic buffering (Stucki, 1980a) represents the basic regulatory principle for the maintenance of a stable far-from-equilibrium regime, where the production of energy is minimal. The bicarbonate buffering via CA operates effectively at pH values close to neutral and plays a significant role in many physiological processes—from carbon fixation in photosynthesis to respiration in animals (Henry, 1996). In the Rubisco reaction, CA will provide a continuous delivery of CO2 from the bicarbonate pool.
According to the Henderson–Hasselbalch equation, only 5–7% of CO2 is released from bicarbonate and carbonate at physiological pH (more with pH decrease). A facilitation of the equilibrium reaction between CO2 and bicarbonate by CA will promote a supply of CO2 to Rubisco. The large pH gradient between the cytosol and chloroplast stroma (which becomes higher in the light) results in a HCO3– concentration that is about five times higher in the stroma compared with the cytosol (Werdan and Heldt, 1972). The transition from darkness to light increases the HCO3– concentration of chloroplast stroma from 0.5 to 2–2.5 mM (Sicher, 1984). This makes the total concentration of carbon accessible to Rubisco approximately equal to the concentration of Rubisco active sites, resulting in the conditions where RSA is valid, provided that the delivery of CO2 from this pool is not limited. However, the data obtained with the stromal CA mutant indicate that the presence of stromal CA does not provide very significant facilitation of CO2 delivery from the stromal pool (Price et al., 1994). On the other hand, the reduction in chloroplast CA affects survival of plants at earlier stages of development (Ferreira et al., 2008). Also, the suppression of CA strongly affects photosynthetic rate of CO2 assimilation (Igamberdiev and Roussel, 2012). The importance of CA in the optimal Rubisco performance is also shown for cyanobacteria (Nishimura et al., 2014) and in photosynthetic bacteria (Dou et al., 2008), but in these organisms it can be explained by the existence of CA-based carbon concentration mechanism. A possible contribution of different CA isoforms to CO2 fixation by higher plants will be discussed below.
Evans (2009) estimated that, in the conditions of fully established CA equilibrium, the CO2 flux in the chloroplast stroma will increase by 26 times as compared if CO2 were the only diffusing substance. According to Tholen and Zhu (2011), the complete equilibration of CO2 and HCO3– occurs at CA concentration of 1 mM, while the concentration of CA in chloroplast stroma ranges between 0.04 and 0.69 mM, suggesting that the amount of CA may somewhat limit the conductance in the stroma. According to their estimations, the internal conductance of CO2 would decrease by more than 40% without CA, decreasing photosynthesis only by 7%. This small value, however, does not include the contribution of the thylakoidal CA that can supply CO2 to Rubisco depending on the electron transport rate (Shutova et al., 2008). This CA isoform was discovered in algae where it is considered to be most important for feeding CO2 to Rubisco (Hanson et al., 2003); recent studies indicate that it is present also in higher plants (Ignatova et al., 2011). This CA is also important for PSII operation because, simultaneously with CO2 production, it removes proton from the thylakoid lumen relieving inhibition of photosynthetic electron transport (Shutova et al., 2008). The primary contribution of the thylakoidal CA to CO2 delivery to Rubisco can explain why the rate of photosynthesis in the stromal CA mutant was not very significantly affected (Price et al., 1994). This explains also why the transgenic plants with impaired stromal CA provided no conclusive evidence for the important role of CA in photosynthesis. However, the fast delivery of CO2 at its higher concentration may be achieved mainly via the stromal CA operating at pH ∼8, because the CA equilibrium is less favorable in the thylakoid lumen at low pH values and not sufficient to feed Rubisco. Up to four separate CA activities associated with thylakoids have been identified (Rudenko et al., 2007).
The involvement of the thylakoidal CA in CO2 delivery to Rubisco implies that the operation of chloroplast electron transport not only supplies NADPH and ATP to the Calvin–Benson cycle, but also participates in pumping CO2 to Rubisco in the stroma through the CA mechanism. This mechanism is linked to the CA activity of PSII and correlates the intensity of chloroplast electron transport and photophosphorylation with the intensity of the Calvin–Benson cycle. Bicarbonate influx to the thylakoid, the CO2 supply to Rubisco, water splitting, and the build-up of proton gradient are all coupled via the CA activity of PSII (Figure 2). Recent findings show that bicarbonate acts as proton acceptor in photosynthetic water oxidation (Koroidov et al., 2014) and that the efficiency of water oxidation depends on the levels of inorganic carbon (Shevela et al., 2013). Bicarbonate is involved in transport of protons produced by water oxidation inside of photosystem II out into the lumen, resulting in a light-driven production of O2 and CO2 that can be delivered to Rubisco. The depletion of bicarbonate leads to a reversible down-regulation of O2 production (Koroidov et al., 2014). The dependence of PSII activity on HCO3– concentration also allows for sensing inorganic carbon level in the stroma and coordinating a feedback regulation of PSII with the Calvin–Benson cycle. These findings add bicarbonate to the regulatory network of oxygenic photosynthesis and confirm that CO2 is released in concordance with the operation of PSII, and that PSII together with the associated CA directly participates in CO2 production from bicarbonate.
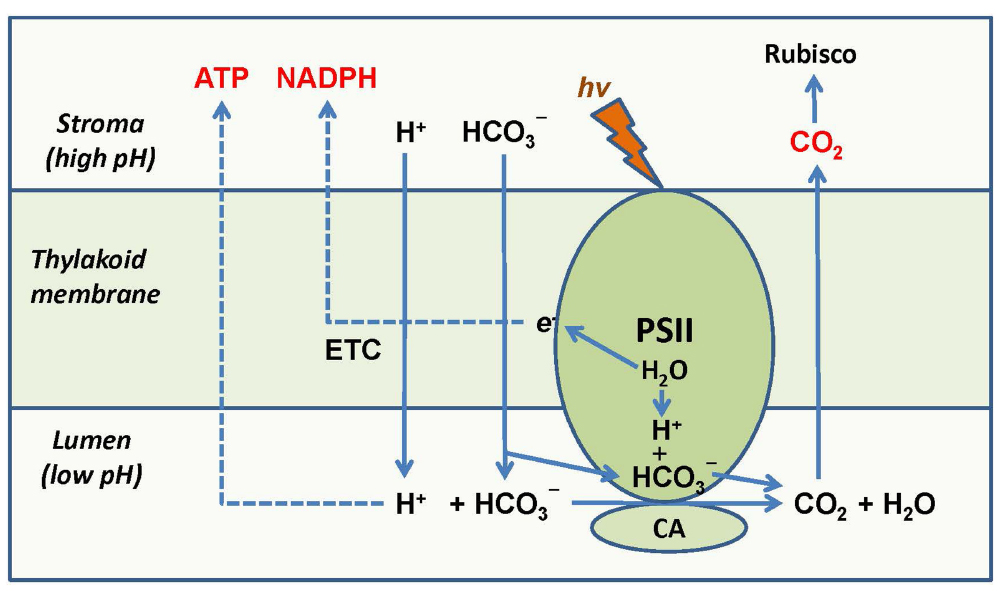
Figure 2. Coordination of supply of NADPH, ATP, and CO2 to Rubisco by PSII and its CA-associated activity. PSII supplies electrons to the chloroplast electron transport chain which results in NADP+ reduction and generation of proton gradient. The protons released during water splitting are accepted by bicarbonate anions forming CO2. A part of the proton gradient is used for bicarbonate transport in the lumen where it forms CO2 with the help of the PSII-associated CA. CO2 is thus supplied to the stroma and feeds Rubisco. Modified from Igamberdiev and Roussel (2012).
The thylakoidal CA contributes to the equilibrium of bicarbonate and CO2 and thus to the conversion of the bicarbonate pumped inside the thylakoid (by using the proton gradient formed during operation of the photosynthetic electron transport chain) to CO2 which escapes back to the stroma. This concentration mechanism was initially suggested by Fridlyand (1995). As it was mentioned earlier (Igamberdiev and Roussel, 2012), at high CO2 concentrations this mechanism will have a higher energy expenditure (dissipation of proton gradient) which may lead to a depletion of ATP needed for regeneration of the CO2 acceptor in the Calvin–Benson cycle. It thus explains the observed decrease in the rate of photosynthesis at oversaturating CO2 concentrations, e.g., in wheat plants (Fridlyand and Tsyuryupa, 1992) and in pea protoplasts (Riazunnisa et al., 2006). As the PSII-driven electron transport is a feedforward regulator of CO2 assimilation, the PSII-associated CA greatly amplifies the flux of CO2 available for carboxylation (Park et al., 1999). The stromal CA can also participate in supplying CO2 to Rubisco but it apparently lacks the necessary efficiency in CO2 concentration, therefore the thylakoidal CA can be considered as the main mechanism providing the continuous CO2 delivery to Rubisco.
Hanson et al. (2003) have shown that insufficient activity of the thylakoidal CA leads to a strong decrease in CO2 fixation. This can be compared with a much weaker effect on photosynthesis by decreasing stromal CA activity (Tholen and Zhu, 2011). The thylakoidal CA operates at low pH where higher portion of CO2 is present as a dissolved gas or H2CO3. This means that its efficiency in CO2 delivery may decrease already with a moderate increase of CO2 supply. The depletion of CO2 substrate at Rubisco site is similar to what Chance and Williams (1955) described for ATP synthase by introducing the term “State 4” to characterize the steady state with a vanishing flow ratio. In the case of the mitochondrial ATP synthase, State 4 condition is avoided in vivo due to the action of adenylate kinase equilibrating adenylates in the mitochondrial intermembrane space (Igamberdiev and Kleczkowski, 2003, 2006, 2015). The depletion of CO2 at the active sites of Rubisco is similar to State 4, and it is avoided by the buffering role of CA. This means that CA works as a thermodynamic buffer enzyme, which is able to effectively buffer the carboxylation potential to the value permitting optimal efficiency of Rubisco reaction. In fact, Rubisco is well optimized to changing CO2, O2, and thermal conditions in the subcellular environments (Tcherkez et al., 2006). The increased efficiency of photosynthesis can be likely achieved not through modulation of the Rubisco enzyme itself but rather via engineering of bicarbonate pumps and increasing their efficient coupling with the Rubisco reaction, e.g., by using transgenic plants expressing the genes associated with cyanobacterial carbon concentration mechanisms (Price et al., 2013; Zarzycki et al., 2013; McGrath and Long, 2014).
Rubisco Curve and its Interpretation
The dependence of Rubisco activity on CO2 in tissues and isolated chloroplasts is represented by a characteristic kinetic curve, in which the deviation from hyperbolic kinetics takes place upon the increase of CO2 concentration. Laisk (1985), Laisk and Oja (1998), and Ruuska et al. (1998) demonstrated a deviation from the MM curve by reaching a maximum velocity of Vmax that ranges from 25 to 50% of the transitional maximum velocity (VCmax) that could be achieved in the absence of such deviation. This means that the rate of steady state reaction deviates from that of the transitional state before CO2 concentration reaches its constant level defined in frames of the MM kinetics. In order to explain the unusual shape of Rubisco curve, it has been suggested that the limitation comes from regeneration of RuBP (Laisk, 1985; Laisk and Oja, 1998; Laisk et al., 2009). This explanation was questioned by Farazdaghi (2011): according to his views, the whole RuBP regeneration process never affects directly the steady state rate of Rubisco reaction but instead the inhibition of Rubisco by the immediate product of reaction (PGA) is involved. The main problem in this interpretation is the lack of experimental data supporting such inhibitory mechanism.
The dependence of kcat of Rubisco in planta (as measured in intact leaves) on photosynthetic electron transport (Eichelmann et al., 2009) is firmly established; however, the final explanation is not given. There is no direct evidence that it occurs via PGA accumulation. Since the rate of CO2 supply via the thylakoidal CA associated with PSII is directly dependent on electron transport, its intensity can determine the maximum rate of Rubisco, which can vary by one order of magnitude depending on the electron transport rate (Eichelmann et al., 2009). It is shown that the thylakoid lumen-localized CA limits CO2 supply to Rubisco, while the pool of RuBP is not depleted (Hanson et al., 2003). The limitation of CO2 supply from thylakoid can occur not only due to its dependence on electron transport but also because, at high CO2, the CA equilibrium becomes less favorable, whereas the CO2 concentration is still far below the concentration of Rubisco active sites. The mechanism of CO2 supply would prevent the overproduction of PGA above the capacity of its utilization in the Calvin–Benson cycle; moreover at high CO2 its production may be kept below such capacity due to earlier saturation of Rubisco curve, which becomes the cause rather than the consequence of the observed shape of Rubisco curve.
The involvement of buffering activities for removal of product of enzyme complexes has been shown for glycine decarboxylase complex (Bykova et al., 2014) and for pyruvate dehydrogenase complex (Igamberdiev et al., 2014). In both cases, the buffering enzymes establish rapid equilibrium of the products of enzymatic reaction relieving inhibition by the product at the active site. For these two enzymes, malate dehydrogenase establishes NADH/NAD+ equilibrium, favoring low concentration of NADH that is a strong inhibitor of both enzymes. In the case of glycine decarboxylase, CA may be another important buffering enzyme, converting the formed in the reaction CO2 to bicarbonate. In the case of Rubisco, the suggested limitation by the product PGA (Farazdaghi, 2011) is less evident, although the antisense reduction of glyceraldehyde phosphate dehydrogenase activity also limits the flux through Rubisco (Price et al., 1995), and the reaction of PGA conversion to glyceraldehyde-3-phosphate is displaced strongly toward the product formation, depending on ATP/ADP ratio in the stroma (Fridlyand and Scheibe, 1999). More important is the equilibration of glyceraldehyde-3-phosphate and subsequent substrates in further reactions equilibrated by buffering enzymes of the Calvin–Benson cycle (Igamberdiev and Kleczkowski, 2011). Thus, without the denial of possible Rubisco limitation by PGA, we are more in favor of limitation by CO2, which can take place both at the level of Rubisco activation by CO2 binding and at the level of Rubisco catalysis.
Considering the source of CO2 as bicarbonate buffer, we can, following (Stucki, 1980a,b) and his theory of thermodynamic buffering (reviewed in Igamberdiev and Kleczkowski, 2009), instead of the dependence of maximum velocity from substrate concentration, consider the dependence of flow ratio j on force ratio x (Stucki, 1980a):
This dependence will be expressed by the degree of coupling q, the parameter which was considered by Stucki (1980a) as constant. However, in the case of Rubisco it will have higher value at low CO2 concentration due to CA equilibrium strongly displaced toward CO2, while at high CO2 concentration its value will be lower due to less favorable equilibrium of CA for CO2 supply to Rubisco. This means that at the force ratio approaching zero (the change from transitional to steady state) the flow ratio will be lower than that achieved at higher degree of coupling that occurs in the transitional state. This means that the dependence of flow through Rubisco (assimilation of CO2) will follow the hyperbolic kinetics at low CO2 concentration (low force of CO2 supply to Rubisco with high coupling) which then will be saturated at significantly lower rate than that following from MM kinetics due to lower coupling at the high force of CO2 supply.
In the conclusion of this section, it should be stated that the Rubisco curve is not a classical MM curve; however, it coincides with the MM curve when the delivery of CO2 from the CO2/bicarbonate pool is not limited by the buffering enzyme (CA). The limitation comes when CO2 concentration becomes high and CA equilibrium turns to be less efficient to produce CO2 from bicarbonate. The dependence of Rubisco reaction from CO2 is the dependence of flow ratio (Rubisco reaction) on force ratio (delivery of CO2). While at low CO2 concentration the coupling coefficient q of CA approaches almost 1, upon CO2 increase CA becomes less efficient and the coupling between CO2 and its delivery to Rubisco drops down. Figure 3 shows the dependence of flow ratio on force ratio at two different degrees of coupling (0.99 and 0.7) and when the coupling coefficient continuously decreases from 0.99 to 0.7. As a result, we obtain a curve which is saturated at lower level of flow rate than it is expected from the MM equation, as observed in the actual case of Rubisco in planta. This shape of curve simply means that the lower value of saturating Rubisco velocity than the Vmax value expected from the MM kinetics occurs due to a decreasing capacity of CO2 supply by CA at increased CO2 concentrations. The mechanism of CO2 supply by CA that pumps it from the thylakoid lumen can efficiently deliver CO2 to Rubisco, but its efficiency decreases with the increase of CO2 concentration.
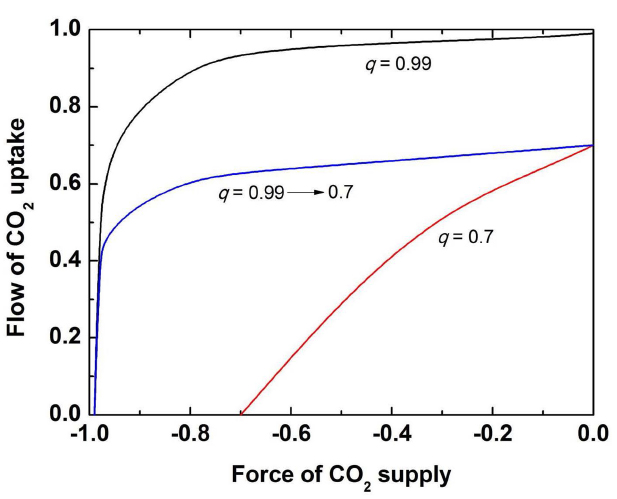
Figure 3. Dependence of the flow rate (utilization of CO2 by Rubisco) on force rate (the level of saturation by CO2) at different values of the coupling parameter q as indicated in the figure (high degree of coupling, q = 0.99; low degree of coupling, q = 0.7; the decreasing degree of coupling from 0.99 to 0.7 which approximates to the actual Rubisco curve).
One of the important features of Rubisco is its carbon isotope effect. 13C carbon isotope discrimination values are stable for concrete Rubiscos and differ depending on Rubisco type and evolutionary position of organism (von Caemmerer et al., 2014). According to the classical theory, the Rayleigh effect (Rayleigh, 1896) should take place, affecting the discrimination value at low CO2. The significant constancy of Rubisco carbon isotope effect may indirectly indicate that CO2 at Rubisco sites is never depleted because it is delivered by the buffering enzyme CA. At the rate of delivery determined only by the rapid equilibrium achieved via CA, the constant 13C fractionation values will represent the in vivo property of Rubisco under the continuous flux of CO2. Variation in 13C discrimination depending on CO2 depletion may take place in the conditions of low CA activity or its inhibition, e.g., it was shown that carbon isotope fractionation by Rubisco is lower if the stromal CA is reduced by 92% (Williams et al., 1996), confirming that CA indeed prevents CO2 depletion at Rubisco active sites.
The Role of Photorespiration in CO2 Supply to Rubisco
While the mechanism of control of Rubisco function via CO2 buffering by CA turns the whole bicarbonate pool to a substrate with high degree of coupling, at low ambient CO2 the bicarbonate/CO2 pool in C3 plants is not renewed sufficiently fast upon depletion. In those conditions, Rubisco load by the substrate remains insufficient to provide the turnover of the Calvin–Benson cycle to consume the reductive power and energy from light reactions. A lower activation state of Rubisco at low CO2 can partially contribute to the overall balance of redox and energy but the most important tool in these conditions becomes the second mechanism of Rubisco optimization, which is its oxygenase reaction. At low CO2 concentrations, even at high degree of CA coupling, the flow through Rubisco is low, since CO2 delivery remains restricted even at the high degree of coupling between CA and Rubisco leading to a decrease of the reaction rate. This results in displacement of the balance between the electron transport rate and CO2 fixation. The CO2 load of carbon assimilation that is attributed to carboxylation at Rubisco site is not constant but fluctuates depending on CO2 supply. Therefore a device is sought which can compensate the fluctuations of the load in order to minimize the deviations from conductance matching and hence the deviations from optimal efficiency of carboxylation in vivo.
In the conditions of low ambient CO2, the oxygenase reaction of Rubisco is needed to compensate fluctuations of the load. Depending of the ambient O2 and CO2 concentrations, the Rubisco kinetics is described by the two mirror curves producing the same optimal flow, i.e., the flux via activated Rubisco is apparently constant at different CO2 concentrations (André, 2011). The oxygenase reaction of Rubisco takes an alternative substrate (O2) and provides the CO2 production in the photorespiratory sequence of reactions. This restricts the variation of CO2 and O2 concentrations within certain limits and keeps the flux through Rubisco constant (Roussel and Igamberdiev, 2011). At low CO2, the supply of CO2 to Rubisco becomes uncoupled from the activity of PSII and the generation of NADPH and ATP exceeds the capacity of the Calvin–Benson cycle. The use of oxygen keeps the flux through Rubisco steady by initiating a metabolic pathway that serves as a major sink of reducing power and ATP.
An increase in photorespiration reduces net photosynthesis but contributes to the maintenance of chloroplast CO2 concentration (Tholen and Zhu, 2011). The mechanism of delivery of substrate from the products of reaction is well explored in nature. The pair of flavin-containing oxidase and catalase in peroxisomes represents such example (Igamberdiev and Kleczkowski, 2009). By returning half of the oxygen consumed in the flavin-dependent reaction, catalase not only detoxifies hydrogen peroxide but also prevents depletion of oxygen at the site of flavin-containing oxidase. Photorespiration produces CO2 in the quantity of 25% of the phosphoglycolate carbon. Oxidation of glyoxylate by the flavin-dependent glycolate oxidase is fast and non-limited by redox level, as this could be in the case of glycolate dehydrogenase (Igamberdiev and Kleczkowski, 2009). The mitochondrial form of CA may contribute to efficient reutilization of the photorespiratory CO2 (Bykova et al., 2014). The intercompartmental pattern of the photorespiratory pathway promotes metabolite channeling, contributes to the control of carbon flux routes through the metabolic network (Sweetlove and Fernie, 2013) and generates the oscillatory regime between the reactions of carboxylation and oxygenation (Roussel et al., 2007; Roussel and Igamberdiev, 2011).
Thus, the role of photorespiration consists not only in the maintenance of redox and energy balance of photosynthetic cells of C3 plants by utilization of the excess of NADPH and ATP, but also in preventing CO2 depletion at Rubisco sites. Photorespiration leads to the maintenance of the balance between CO2 load and consumption of NADPH and ATP, so the processes become well equilibrated. In C4 plants, which remain phenotypically less plastic (Sage and McKown, 2006), the role of photorespiration becomes minimal; however, it may still play a role in bundle sheath cells (Yoshimura et al., 2004) where all photorespiratory CO2 is efficiently captured. Photorespiration may also be a factor that prevented depletion of CO2 concentration in the atmosphere (Igamberdiev and Lea, 2006) in the same way as it prevents its depletion in photosynthetic cells. Engineering of plants with a high capacity of bicarbonate pump can also result in more efficient refixation of photorespiratory CO2; however, modulation of the photorespiratory pathway itself could lead to the changes in the coordination between Rubisco activity, electron transport rate and cellular redox balance, and therefore may not be beneficial for the overall photosynthetic performance (de Carvalho et al., 2011).
Conclusion
Rubisco operates at much higher concentration than its substrate CO2; however, chloroplasts have bicarbonate pool which concentration is comparable to the concentration of Rubisco and increases upon illumination. The delivery of CO2 from this pool to Rubisco active sites is a major prerequisite of its stable operation. While the stromal CA has a limited capacity for provision of the optimal performance of Rubisco, the mechanism involving the PSII-associated CA activity coordinated with water splitting, chloroplast electron transport, and ATP synthesis may possess a higher capacity. At low ambient CO2, this mechanism cannot produce enough CO2 to utilize all the reducing power generated in light reactions, and the oxygenase reaction of Rubisco represents a sink for reducing power and energy and results in photorespiratory supply of CO2 to keep Rubisco functioning. The flux through the activated Rubisco is apparently steady and relatively independent of different CO2 concentrations (considering that CO2 can be substituted by O2). At low CO2, its supply is limited by the photorespiratory feedback, while at high CO2 it is limited by the capacity of the thylakoidal CO2 pump. This is summarized in Figure 4. Rubisco operates at lower CO2 concentrations than its protein concentration, while the homeostatic equilibration of CO2 supply from the bicarbonate pool controls the metabolic flux through the enzyme and coordinates it with NADPH and ATP produced in light reactions. By using Rubisco, which originally seemed to be inefficient having low catalytic constant and low specificity, living systems in fact exploit the mechanism that aims to achieve the optimal parameters of carbon fixation. They do so by coordinating CO2 assimilation rate, via buffering the bicarbonate pool, with generation of reducing power and energy currency and with oxygen utilization. This mechanism underlines the importance of the strategy for improving photosynthesis via engineering of bicarbonate pumps and increasing their efficient coupling with the reaction of Rubisco.
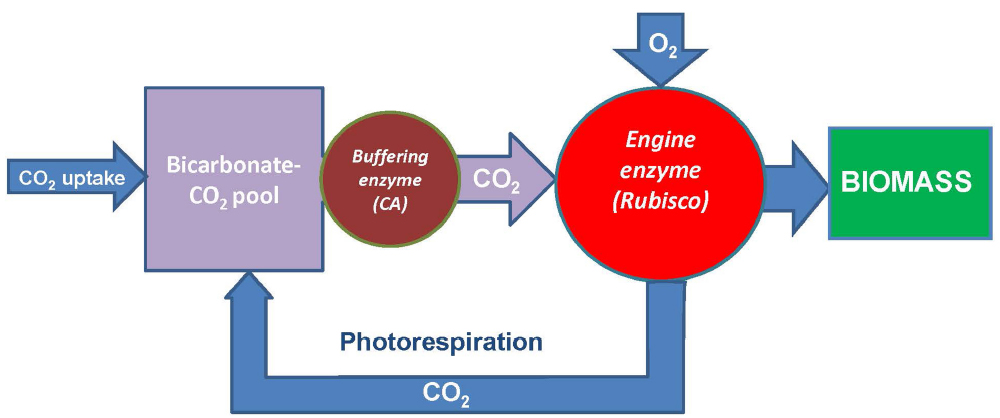
Figure 4. General scheme showing operation of Rubisco as the engine for generating biomass from CO2. The source of CO2 is a bicarbonate pool fed from the atmosphere and buffered by carbonic anhydrase (CA) serving as a pump for Rubisco. The latter is an engine producing biomass and at the same time generating a feedback (photorespiration) via its oxygenase reaction to feed the bicarbonate pool in the conditions of insufficient CO2 supply. Modified from Igamberdiev and Roussel (2012).
Conflict of Interest Statement
The author declares that the research was conducted in the absence of any commercial or financial relationships that could be construed as a potential conflict of interest.
Acknowledgments
The discussion of the ideas of the paper with Dr. Leszek A. Kleczkowski (Umeå University, Sweden) and Dr. Marc R. Roussel (University of Lethbridge, Canada) is greatly acknowledged. This work was supported by the National Science and Engineering Research Council of Canada.
Abbreviations
CA, carbonic anhydrase; MM, Michaelis–Menten; PGA, phosphoglyceric acid; RuBP, ribulose-1,5-bisphosphate.
References
André, M. J. (2011). Modelling 18O2 and 16O2, unidirectional fluxes in plants: I. regulation of pre-industrial atmosphere. Biosystems 103, 239–251. doi: 10.1016/j.biosystems.2010.10.004
Pubmed Abstract | Pubmed Full Text | CrossRef Full Text | Google Scholar
Baeyer, A. D. (1870). Ueber die wasserentziehung und ihre bedeutung für das pflanzenleben und die gärung. Ber. Dtsch. Chem. Ges. 3, 63–75.
Bauer, E. S. (1935). Theoretical Biology. Moscow: VIEM. (Republished with partial English translation in 1982 by Akadémiai Kiadó, Budapest).
Benson, A. A., and Calvin, M. (1950). Carbon dioxide fixation by green plants. Annu. Rev. Plant Physiol. 1, 25–42. doi: 10.1146/annurev.pp.01.060150.000325
Butlerow, A. (1861). Bildung einer zuckerartigen Substanz durch Synthese. Ann. Chem. Pharm. 44, 295–298.
Bykova, N. V., Møller, I. M., Gardeström, P., and Igamberdiev, A. U. (2014). The function of glycine decarboxylase complex is optimized to maintain high photorespiratory flux via buffering of its reaction products. Mitochondrion 19, 357–364. doi: 10.1016/j.mito.2014.01.001
Pubmed Abstract | Pubmed Full Text | CrossRef Full Text | Google Scholar
Chance, B., and Williams, G. R. (1955). Respiratory enzymes in oxidative phosphorylation. III. The steady state. J. Biol. Chem. 217, 409–427.
de Carvalho, J. F. C., Madgwick, P. J., Powers, S. J., Keys, A. J., Lea, P. J., and Parry, M. A. J. (2011). An engineered pathway for glyoxylate metabolism in tobacco plants aimed to avoid the release of ammonia in photorespiration. BMC Biotechnol. 11:111. doi: 10.1186/1472-6750-11-111
Pubmed Abstract | Pubmed Full Text | CrossRef Full Text | Google Scholar
Dou, Z., Heinhorst, S., Williams, E. B., Murin, C. D., Shively, J. M., and Cannon, G. C. (2008). CO2 fixation kinetics of Halothiobacillus neapolitanus mutant carboxysomes lacking carbonic anhydrase suggest the shell acts as a diffusional barrier for CO2. J. Biol. Chem. 283, 10377–10384. doi: 10.1074/jbc.M709285200
Pubmed Abstract | Pubmed Full Text | CrossRef Full Text | Google Scholar
Eichelmann, H., Talts, E., Oja, V., Padu, E., and Laisk, A. (2009). Rubisco in planta kcat is regulated in balance with photosynthetic electron transport. J. Exp. Bot. 60, 4077–4088. doi: 10.1093/jxb/erp242
Pubmed Abstract | Pubmed Full Text | CrossRef Full Text | Google Scholar
Evans, J. R. (2009). Potential errors in electron transport rates calculated from chlorophyll fluorescence as revealed by a multilayer leaf model. Plant Cell Physiol. 50, 698–706. doi: 10.1093/pcp/pcp041
Pubmed Abstract | Pubmed Full Text | CrossRef Full Text | Google Scholar
Farazdaghi, H. (2011). The single-process biochemical reaction of Rubisco: a unified theory and model with the effects of irradiance, CO2, and rate-limiting step on the kinetics of C3 and C4 photosynthesis from gas exchange. Biosystems 103, 265–284. doi: 10.1016/j.biosystems.2010.11.004
Pubmed Abstract | Pubmed Full Text | CrossRef Full Text | Google Scholar
Ferreira, F. J., Guo, C., and Coleman, J. R. (2008). Reduction of plastid-localized carbonic anhydrase activity results in reduced Arabidopsis seedling survivorship. Plant Physiol. 147, 585–594. doi: 10.1104/pp.108.118661
Pubmed Abstract | Pubmed Full Text | CrossRef Full Text | Google Scholar
Fridlyand, L. E. (1995). “On the possibility of existence of specific CO2 concentration mechanism in chloroplasts of C3 plants,” in Photosynthesis: From Light to Biosphere, Vol. 5, ed. P. Mathis (Dordrecht: Kluwer), 559–562.
Fridlyand, L. E., and Scheibe, R. (1999). Regulation of the Calvin cycle for CO2 fixation as an example for general control mechanisms in metabolic cycles. Biosystems 51, 79–93. doi: 10.1016/S0303-2647(99)00017-9
Pubmed Abstract | Pubmed Full Text | CrossRef Full Text | Google Scholar
Fridlyand, L. E., and Tsyuryupa, S. N. (1992). Inhibition of photosynthesis by supraoptimal concentrations of CO2 and possible mechanisms of this phenomenon. Soviet Plant Physiol. 39, 504–507.
Hanson, D. T., Franklin, L. A., Samuelsson, G., and Badger, M. R. (2003). The Chlamydomonas reinhardtii cia3 mutant lacking a thylakoid lumen-localized carbonic anhydrase is limited by CO2 supply to Rubisco and not photosystem II function in vivo. Plant Physiol. 132, 2267–2275. doi: 10.1104/pp.103.023481
Pubmed Abstract | Pubmed Full Text | CrossRef Full Text | Google Scholar
Hanson, S. M., and Schnell, S. (2008). Reactant stationary approximation in enzyme kinetics. J. Phys. Chem. A 112, 8654–8658. doi: 10.1021/jp8026226
Pubmed Abstract | Pubmed Full Text | CrossRef Full Text | Google Scholar
Henry, R. P. (1996). Multiple roles of carbonic anhydrase in cellular transport and metabolism. Annu. Rev. Physiol. 58, 523–538. doi: 10.1146/annurev.physiol.58.1.523
Pubmed Abstract | Pubmed Full Text | CrossRef Full Text | Google Scholar
Igamberdiev, A. U., Bykova, N. V., and Kleczkowski, L. A. (1999). Origins and metabolism of formate in higher plants. Plant Physiol. Biochem. 37, 503–513. doi: 10.1016/S0981-9428(00)80102-3
Igamberdiev, A. U., and Kleczkowski, L. A. (2003). Membrane potential, adenylate levels and Mg2+ are interconnected via adenylate kinase equilibrium in plant cells. Biochim. Biophys. Acta 1607, 111–119. doi: 10.1016/j.bbabio.2003.09.005
Pubmed Abstract | Pubmed Full Text | CrossRef Full Text | Google Scholar
Igamberdiev, A. U., and Kleczkowski, L. A. (2006). Equilibration of adenylates in the mitochondrial intermembrane space maintains respiration and regulates cytosolic metabolism. J. Exp. Bot. 57, 2133–2141. doi: 10.1093/jxb/erl006
Pubmed Abstract | Pubmed Full Text | CrossRef Full Text | Google Scholar
Igamberdiev, A. U., and Kleczkowski, L. A. (2009). Metabolic systems maintain stable non-equilibrium via thermodynamic buffering. Bioessays 31, 1091–1099. doi: 10.1002/bies.200900057
Pubmed Abstract | Pubmed Full Text | CrossRef Full Text | Google Scholar
Igamberdiev, A. U., and Kleczkowski, L. A. (2011). Optimization of CO2 fixation in photosynthetic cells via thermodynamic buffering. Biosystems 103, 224–229. doi: 10.1016/j.biosystems.2010.10.001
Pubmed Abstract | Pubmed Full Text | CrossRef Full Text | Google Scholar
Igamberdiev, A. U., and Kleczkowski, L. A. (2015). Optimization of ATP synthase function in mitochondria and chloroplasts via the adenylate kinase equilibrium. Front. Plant Sci. 6:10. doi: 10.3389/fpls.2015.00010
Pubmed Abstract | Pubmed Full Text | CrossRef Full Text | Google Scholar
Igamberdiev, A. U., and Lea, P. J. (2006). Land plants equilibrate O2 and CO2 concentrations in the atmosphere. Photosynth. Res. 87, 177–194. doi: 10.1007/s11120-005-8388-2
Pubmed Abstract | Pubmed Full Text | CrossRef Full Text | Google Scholar
Igamberdiev, A. U., and Roussel, M. R. (2012). Feedforward non-Michaelis–Menten mechanism for CO2 uptake by Rubisco: contribution of carbonic anhydrases and photorespiration to optimization of photosynthetic carbon assimilation. Biosystems 107, 158–166. doi: 10.1016/j.biosystems.2011.11.008
Pubmed Abstract | Pubmed Full Text | CrossRef Full Text | Google Scholar
Igamberdiev, A. U., Lernmark, U., and Gardeström P. (2014). Activity of the mitochondrial pyruvate dehydrogenase complex in plants is stimulated in the presence of malate. Mitochondrion 19, 184–190. doi: 10.1016/j.mito.2014.04.006
Pubmed Abstract | Pubmed Full Text | CrossRef Full Text | Google Scholar
Ignatova, L. K., Rudenko, N. N., Mudrik, V. A., Fedorchuk, T. P., and Ivanov, B. N. (2011). Carbonic anhydrase activity in Arabidopsis thaliana thylakoid membrane and fragments enriched with PSI or PSII. Photosynth. Res. 110, 89–98. doi: 10.1007/s11120-011-9699-0
Pubmed Abstract | Pubmed Full Text | CrossRef Full Text | Google Scholar
Kent, S. S. (1972). Photosynthesis in the higher plant Vicia faba. II. The non-Calvin cycle origin of acetate and its metabolic relationship to the photosynthetic origin of formate. J. Biol. Chem. 247, 7293–7302.
Koroidov, S., Shevela, D., Shutova, T., Samuelsson, G., and Messinger, J. (2014). Mobile hydrogen carbonate acts as proton acceptor in photosynthetic water oxidation. Proc. Natl. Acad. Sci. U.S.A. 111, 6299–6304. doi: 10.1073/pnas.1323277111
Pubmed Abstract | Pubmed Full Text | CrossRef Full Text | Google Scholar
Laisk, A. (1985). “Kinetics of photosynthetic CO2 uptake in C3 plants,” in Kinetics of Photosynthetic Carbon Metabolism, eds J. Viil, G. Grishina, and A. Laisk (Tallinn: Valgus Press), 21–34.
Laisk, A., Eichelman, H., and Oja, V. (2009). “Leaf C3 photosynthesis in silico,” in Photosynthesis in Silico: Understanding Complexity from Molecules to Ecosystems, eds A. Laisk, L. Nedbal, and Govindjee (Dordrecht: Springer), 295–322.
Laisk, A., and Oja, V. (1998). Dynamics of Leaf Photosynthesis: Rapid Response Measurements and Their Interpretations. Melbourne: CSIRO Publishing.
McGrath, J. M., and Long, S. P. (2014). Can the cyanobacterial carbon-concentrating mechanism increase photosynthesis in crop species? A theoretical analysis. Plant Physiol. 164, 2247–2261. doi: 10.1104/pp.113.232611
Pubmed Abstract | Pubmed Full Text | CrossRef Full Text | Google Scholar
Nishimura, T., Yamaguchi, O., Takatani, N., Maeda, S. I., and Omata, T. (2014). In vitro and in vivo analyses of the role of the carboxysomal β-type carbonic anhydrase of the cyanobacterium Synechococcus elongatus in carboxylation of ribulose-1,5-bisphosphate. Photosynth. Res. 121, 151–157. doi: 10.1007/s11120-014-9986-7
Pubmed Abstract | Pubmed Full Text | CrossRef Full Text | Google Scholar
Park, Y. I., Karlsson, J., Rojdestvenski, I., Pronina, N., Klimov, V., Oquist, G., et al. (1999). Role of a novel photosystem II-associated carbonic anhydrase in photosynthetic carbon assimilation in Chlamydomonas reinhardtii. FEBS Lett. 444, 102–105. doi: 10.1016/S0014-5793(99)00037-X
Price, G. D., Evans, J. R., von Caemmerer, S., Yu, J. W., and Badger, M. R. (1995). Specific reduction of chloroplast glyceraldehyde-3-phosphate dehydrogenase activity in ribulose bisphosphate regeneration in transgenic tobacco plants. Planta 195, 369–378. doi: 10.1007/BF00202594
Pubmed Abstract | Pubmed Full Text | CrossRef Full Text | Google Scholar
Price, G. D., Pengelly, J. J., Forster, B., Du, J., Whitney, S. M., von Caemmerer, S., et al. (2013). The cyanobacterial CCM as a source of genes for improving photosynthetic CO2 fixation in crop species. J. Exp. Bot. 64, 753–768. doi: 10.1093/jxb/ers257
Pubmed Abstract | Pubmed Full Text | CrossRef Full Text | Google Scholar
Price, G. D., von Caemmerer, S., Evans, J. R., Yu, J. W., Lloyd, J., Oja, V., et al. (1994). Specific reduction of chloroplast carbonic-anhydrase activity by antisense rna in transgenic tobacco plants has a minor effect on photosynthetic CO2 assimilation. Planta 193, 331–340. doi: 10.1007/BF00201810
Rayleigh, J. W. S. (1896). Theoretical considerations respecting the separation of gases by diffusion and similar processes. Philos. Mag. J. Sci. 42, 493–498. doi: 10.1080/14786449608620944
Riazunnisa, K., Padmavathi, L., Bauwe, H., and Raghavendra, A. S. (2006). Markedly low requirement of added CO2 for photosynthesis by mesophyll protoplasts of pea (Pisum sativum): possible roles of photorespiratory CO2 and carbonic anhydrase. Physiol. Plant. 128, 763–772. doi: 10.1111/j.1399-3054.2006.00803.x
Roussel, M. R., and Igamberdiev, A. U. (2011). Dynamics and mechanisms of oscillatory photosynthesis. Biosystems 103, 230–238. doi: 10.1016/j.biosystems.2010.07.020
Pubmed Abstract | Pubmed Full Text | CrossRef Full Text | Google Scholar
Roussel, M. R., Ivlev, A. A., and Igamberdiev, A. U. (2007). Oscillations of the internal CO2 concentration in tobacco leaves transferred to low CO2. J. Plant Physiol. 164, 1188–1196. doi: 10.1016/j.jplph.2006.08.004
Pubmed Abstract | Pubmed Full Text | CrossRef Full Text | Google Scholar
Rudenko, N. N., Ignatova, L. K., and Ivanov, B. N. (2007). Multiple sources of carbonic anhydrase activity in pea thylakoids: soluble and membrane-bound forms. Photosynth. Res. 91, 81–89. doi: 10.1007/s11120-007-9148-2
Pubmed Abstract | Pubmed Full Text | CrossRef Full Text | Google Scholar
Ruuska, S. A., Andrews, T. J., Badger, M. R., Hudson, G. S., Laisk, A., Price, G. D., et al. (1998). The interplay between limiting processes in C3 photosynthesis studied by rapid response gas exchange using transgenic tobacco impaired in photosynthesis. Aust. J. Plant Physiol. 25, 859–870. doi: 10.1071/PP98079
Sage, R. F., and McKown, A. D. (2006). Is C4 photosynthesis less phenotypically plastic than C3 photosynthesis? J. Exp. Bot. 57, 303–317. doi: 10.1093/jxb/erj040
Pubmed Abstract | Pubmed Full Text | CrossRef Full Text | Google Scholar
Shevela, D., Nöring, B., Koroidov, S., Shutova, T., Samuelsson, G., and Messinger, J. (2013). Efficiency of photosynthetic water oxidation at ambient and depleted levels of inorganic carbon. Photosynth. Res. 117, 401–412. doi: 10.1007/s11120-013-9875-5
Pubmed Abstract | Pubmed Full Text | CrossRef Full Text | Google Scholar
Schnell, S. (2014). Validity of the Michaelis–Menten equation—steady-state or reactant stationary assumption: that is the question. FEBS J. 281, 464–472. doi: 10.1111/febs.12564
Pubmed Abstract | Pubmed Full Text | CrossRef Full Text | Google Scholar
Shutova, T., Kenneweg, H., Buchta, J., Nikitina, J., Terentyev, V., Chernyshov, S., et al. (2008). The photosystem II-associated Cah3 in Chlamydomonas enhances the O2 evolution rate by proton removal. EMBO J. 27, 782–791. doi: 10.1038/emboj.2008.12
Pubmed Abstract | Pubmed Full Text | CrossRef Full Text | Google Scholar
Sicher, R. C. (1984). Characteristics of light-dependent inorganic carbon uptake by isolated spinach chloroplasts. Plant Physiol. 74, 962–966. doi: 10.1104/pp.74.4.962
Pubmed Abstract | Pubmed Full Text | CrossRef Full Text | Google Scholar
Stucki, J. W. (1980a). The thermodynamic buffer enzymes. Eur. J. Biochem. 109, 257–267. doi: 10.1111/j.1432-1033.1980.tb04791.x
Pubmed Abstract | Pubmed Full Text | CrossRef Full Text | Google Scholar
Stucki, J. W. (1980b). The optimal efficiency and the economic degrees of coupling of oxidative phosphorylation. Eur. J. Biochem. 109, 269–283. doi: 10.1111/j.1432-1033.1980.tb04792.x
Pubmed Abstract | Pubmed Full Text | CrossRef Full Text | Google Scholar
Sweetlove, L. J., and Fernie, A. R. (2013). The spatial organization of metabolism within the plant cell. Annu. Rev. Plant Biol. 64, 723–746. doi: 10.1146/annurev-arplant-050312-120233
Pubmed Abstract | Pubmed Full Text | CrossRef Full Text | Google Scholar
Tagawa, K., and Arnon, D. J. (1962). Ferredoxins as electron carriers in photosynthesis and in the biological production and consumption of hydrogen gas. Nature 195, 537–543. doi: 10.1038/195537a0
Pubmed Abstract | Pubmed Full Text | CrossRef Full Text | Google Scholar
Tcherkez, G. G., Farquhar, G. D., and Andrews, T. J. (2006). Despite slow catalysis and confused substrate specificity, all ribulose bisphosphate carboxylases may be nearly perfectly optimized. Proc. Natl. Acad. Sci. U.S.A. 103, 7246–7251. doi: 10.1073/pnas.0600605103
Pubmed Abstract | Pubmed Full Text | CrossRef Full Text | Google Scholar
Tholen, D., and Zhu, X.-G. (2011). The mechanistic basis of internal conductance: a theoretical analysis of mesophyll cell photosynthesis and CO2 diffusion. Plant Physiol. 156, 90–105. doi: 10.1104/pp.111.172346
Pubmed Abstract | Pubmed Full Text | CrossRef Full Text | Google Scholar
von Caemmerer, S., Tazoe, Y., Evans, J. R., and Whitney, S. M. (2014). Exploiting transplastomically modified Rubisco to rapidly measure natural diversity in its carbon isotope discrimination using tuneable diode laser spectroscopy. J. Exp. Bot. 65, 3759–3767. doi: 10.1093/jxb/eru036
Pubmed Abstract | Pubmed Full Text | CrossRef Full Text | Google Scholar
Werdan, K., and Heldt, H. W. (1972). Accumulation of bicarbonate in intact chloroplasts following a pH gradient. Biochim. Biophys. Acta 283, 430–441. doi: 10.1016/0005-2728(72)90260-5
Williams, T. G., Flanagan, L. B., and Coleman, J. R. (1996). Photosynthetic gas exchange and discrimination against 13CO2 and C18O16O in tobacco plants modified by an antisense construct to have low chloroplastic carbonic anhydrase. Plant Physiol. 112, 319–326. doi: 10.1104/pp.112.1.319
Pubmed Abstract | Pubmed Full Text | CrossRef Full Text | Google Scholar
Yoshimura, Y., Kubota, F., and Ueno, O. (2004). Structural and biochemical bases of photorespiration in C4 plants: quantification of organelles and glycine decarboxylase. Planta 220, 307–317. doi: 10.1007/s00425-004-1335-1
Pubmed Abstract | Pubmed Full Text | CrossRef Full Text | Google Scholar
Zarzycki, J., Axen, S. D., Kinney, J. N., and Kerfeld, C. A. (2013). Cyanobacterial-based approaches to improving photosynthesis in plants. J. Exp. Bot. 64, 787–798. doi: 10.1093/jxb/ers294
Pubmed Abstract | Pubmed Full Text | CrossRef Full Text | Google Scholar
Keywords: bicarbonate pool, carbonic anhydrases, chloroplasts, Michaelis–Menten kinetics, photorespiration, reactant stationary approximation, Rubisco
Citation: Igamberdiev AU (2015) Control of Rubisco function via homeostatic equilibration of CO2 supply. Front. Plant Sci. 6:106. doi: 10.3389/fpls.2015.00106
Received: 01 December 2014; Accepted: 09 February 2015;
Published online: 26 February 2015.
Edited by:
Lee Sweetlove, University of Oxford, UKReviewed by:
Katja Baerenfaller, Swiss Federal Institute of Technology Zürich, SwitzerlandNicholas Smirnoff, Exeter University, UK
Copyright © 2015 Igamberdiev. This is an open-access article distributed under the terms of the Creative Commons Attribution License (CC BY). The use, distribution or reproduction in other forums is permitted, provided the original author(s) or licensor are credited and that the original publication in this journal is cited, in accordance with accepted academic practice. No use, distribution or reproduction is permitted which does not comply with these terms.
*Correspondence: Abir U. Igamberdiev, Department of Biology, Memorial University of Newfoundland, 232 Elizabeth Avenue, St. John’s, NL A1B 3X9, Canada email: igamberdiev@mun.ca